- 1Department of Materials Science and Engineering, The Pennsylvania State University, University Park, PA, United States
- 2Department of Chemical Engineering, The Pennsylvania State University, University Park, PA, United States
- 3Department of Chemistry, The Pennsylvania State University, University Park, PA, United States
Single ion conducting gel polymer electrolytes (GPEs) are characterized as having a certain amount of ionic liquid or solvent incorporated into a single ion-conducting polymer matrix and may afford the advantages of high conductivity and low electrolyte polarization under battery operation. Single ion conducting polymers often suffer from low conductivity due to their reliance on polymer segmental motion to achieve sufficient ion mobility. However, by incorporating specific solvents into a single ion conducting matrix, mobility of the polymer can be enhanced while still maintaining the advantages of single ion conduction. Although many of the solvents used to swell GPEs are mixtures of flammable organic solvents (such as dimethyl carbonate), there are many potential non-reactive, low vapor pressure solvents that could effectively solvate alkali-ion based GPEs and plasticize the polymer matrix to enhance ion conductivity. Adipate-based solvents are a group of non-volatile plasticizers with low viscosities and low vapor pressures at room temperature derived from adipic acid. The ester groups in these solvents may effectively solvate alkali ions such as Na+, leading to higher conductivity, while circumventing issues of flammability found in current alkali-ion conducting electrolytes. This study investigates the properties of sodium-ion conducting GPEs that have been swollen with varying adipate-based solvents and the subsequent dielectric response from the solvent addition. Dielectric relaxation spectroscopy was used to characterize the Na+ conductivity, static dielectric constant, ion-conducting content, and mobility of the membranes before and after the non-volatile solvent uptake. Understanding this relationship will pave the path toward safer, more efficient solid-state polymer electrolytes for battery applications.
Introduction
From portable electronics to hybrid vehicles, alkali-ion batteries are a ubiquitous and vital part of today’s technology-driven world. The advancement of the lithium-ion battery has sparked research and development of new kinds of alkali-ion and alkaline earth-based batteries such as those driven by Na+, K+, or Mg2+ ion transport (Slater et al., 2013; Yoo et al., 2013; Yabuuchi et al., 2014; Jian et al., 2015; Choi and Aurbach, 2016). These nascent technologies have shown great promise in delivering high energy density, fast discharge rates, and high cyclability, all while addressing the emerging issue of lithium scarcity (Komaba et al., 2011; Saravanan et al., 2013; Wen et al., 2014; Kundu et al., 2015; Lee et al., 2017). While battery technology has been flourishing over the last three decades, there are still some serious safety concerns that must be addressed. One key materials challenge is developing an electrolyte to replace traditional liquid-based electrolytes in batteries, which are often prone to leakage and flammability (Balakrishnan et al., 2006; Tagawa and Brodd, 2009; Fleischhammer and Doering, 2018; Kerner et al., 2016; Hartnig and Schmidt, 2018). One way to tackle this issue is to employ solid-state polymer or polymer-based electrolytes. Gel polymer electrolytes (GPEs), in particular, have been gaining momentum as an optimum way to combine the diffusive properties of liquids with the mechanical properties of a solid, serving as a safe and attractive alternative to traditional liquid electrolytes (Balakrishnan et al., 2006; Hartnig and Schmidt, 2018; Liang et al., 2018).
GPEs are fabricated by employing a plasticizer or solvent mixture within an ion-conducting polymer matrix. The segmental motion of the polymer matrix allows the ionic species to move between coordination sites (either on one chain or between adjacent chains), while the solvent further promotes mobility of the ionic species leading to enhanced conduction. Additionally, the solvent can increase the dielectric constant of the material to promote ion dissociation. Because the solvent is incorporated within the polymer matrix, GPEs exist in the semi solid-state, thereby avoiding the leakage and flammability issues found with traditional liquid electrolytes. Polymer matrixes such as poly(ethylene oxide), poly(vinylidene fluoride) (PVDF), and poly(acrylonitrile) have been extensively explored for GPE applications due to their low glass transition temperatures and because the electron donating groups (−O−, −NH2) in these materials can coordinate with alkali cations to enhance ion-conduction. Ion conductivity in GPEs can be further improved by incorporating the right type of solvent into the polymer matrix. It is necessary to choose solvents with high permittivity (ɛ > 15) to dissolve alkali metal-based salts in high concentration, low viscosity (≤1 cP) to assure that the transport of ions is not impeded, and a high flashpoint to avoid issues with flammability in device usage (Song et al., 1999; Stephan, 2006; Pandey, 2008; Mustarelli, 2011; Long et al., 2016; Arya and Sharma, 2017; Hartnig and Schmidt, 2018; Leuthner, 2018). Solvents employed in GPEs can increase ionic conductivity by either softening the polymer matrix and lowering the glass transition temperature or improving dissociation of the ionic species and increasing the amount of mobile charge carriers (Arya and Sharma, 2017; Choi and Colby, 2017).
GPEs are typically produced by solution casting, in which the polymer matrix and electrolytic species are dissolved into an appropriate solvent. This solution is then cast onto a substrate where the solvent is subsequently removed through heat or vacuum, and a GPE film is formed (Long et al., 2016; Safa et al., 2016; Liang et al., 2018). However, in recent years there has been a great interest in investigating environmentally friendly methods of producing solid polymer electrolytes, without the use of organic solvents. Photopolymerization is an attractive, in situ method of efficiently mass producing mechanically robust, free-standing GPEs. In this method, a curable monomer, liquid electrolyte, and photo-initiators are placed in a lithium-ion cell and cured under ultraviolet (UV) radiation to form a polymer network in which the liquid electrolyte solidifies within the gaps of the polymer matrix. Monomers with vinyl and oxide groups are needed to induce polymerization and promote conduction of the ion conducting species (Seo et al., 2016; Capparelli et al., 2018). This method has been successful in both lithium and sodium-ion conducting UV-cured GPEs (Safa et al., 2016; Bella et al., 2017; Dam et al., 2019; Jin et al., 2019; Wang et al., 2019; Ford et al., 2020).
GPEs of lithium-containing salts have been extensively studied due to the high energy density of lithium ion batteries, but in recent years there has been a push to further research on sodium ion-conducting electrolytes (due to the low cost and processing of sodium salts) with new kinds of specific solvents to enhance conductivity. For sodium-ion based electrolytes, solvents that contain carbonate oxygens or ester groups are typically employed because the oxygen atom in these groups can coordinate with the ionic species, thereby promoting conduction (Xu, 2004; Xu, 2014). For this reason, carbonate-based solvents such as propylene carbonate (PC) and ethylene carbonate have been proven to coordinate with Na+ and heighten conduction in Na+ electrolyte applications. By utilizing appropriate solvents that have low flash points and the ability to coordinate with Na+, it may be possible to attain a new generation of safer, efficient solvents for forthcoming battery technologies.
However, despite the great strides made in understanding the structure-conductivity relationships of sodium-ion conducting GPEs, there have been relatively few studies on single-ion conducting GPEs. Most Na+-conducting GPEs consist of a salt that has been dissolved into a polymer matrix, allowing for mobility of both ionic species of the salt, which may lower overall conduction of the target ion and lead to undesirable electrolyte polarization in a device. The benefit of utilizing single-ion conductors is that only one of the ionic species is free for transport, which may yield a more efficient electrolyte. In this paper, we have investigated how carbonate and ester-based solvents such as PC, dimethyl adipate, and diethyl 4-oxopimelate, as well as glycerol impact the conductivity profile and polymer dynamics of single-ion Na+-conducting photopolymerized GPE membranes. We have employed dielectric relaxation spectroscopy (DRS) to characterize the membranes and have proposed mechanisms by which the solvents interact with the Na+ using Fourier‐transform infrared spectroscopy (FTIR). Thermal gravimetric analysis (TGA) was employed to quantify solvent retention in the GPEs during DRS. We have found that all plasticizers investigated improved the conductivity of the Na+ GPE base membrane, and that using glycerol as a plasticizer yielded a nearly two order of magnitude improvement in conductivity. The solvents selected all have a flashpoint above 100°C, in order to address the growing concern of flammability in electrolytic devices. The insights from this work will assist in developing a deeper understanding of ion transport in single-ion conducting GPEs and ultimately help pave the way toward improving conductivity in next-generation polymer electrolytes.
Experimental
Materials
The materials used for the photocurable resin were commercially available and in line with our group’s prior work (Seo et al., 2016; Capparelli et al., 2018). The resin consisted of poly(ethylene glycol) diacrylate (PEGDA, oligomer, Mn 700), diurethane dimethacrylate (DUDMA, oligomer, Mn 471), dipentaerythritol penta-/hexa-acrylate (cross-linker), and 2-Acrylamido-2-methyl-1-propanesulfonic acid sodium salt solution (Na+ AMPS−). 1 wt% phenylbis(2,4,6-trimethylbenzoyl)- phosphine oxide (Irgacure 819, initiator), 1 wt% 1-hydroxycyclohexyl phenyl ketone (Irgacure 184, initiator), and 0.02 wt% Sudan I (UV absorber) were added to the resin to induce photocuring. Sudan I was purchased from Acros Organics (Waltham, MA, United States), while all the other chemicals were obtained from Sigma-Aldrich (St. Louis, MO, United States). Figure 1 shows the chemical structure of PEGDA, DUDMA, and the Na+ AMPS− salt.
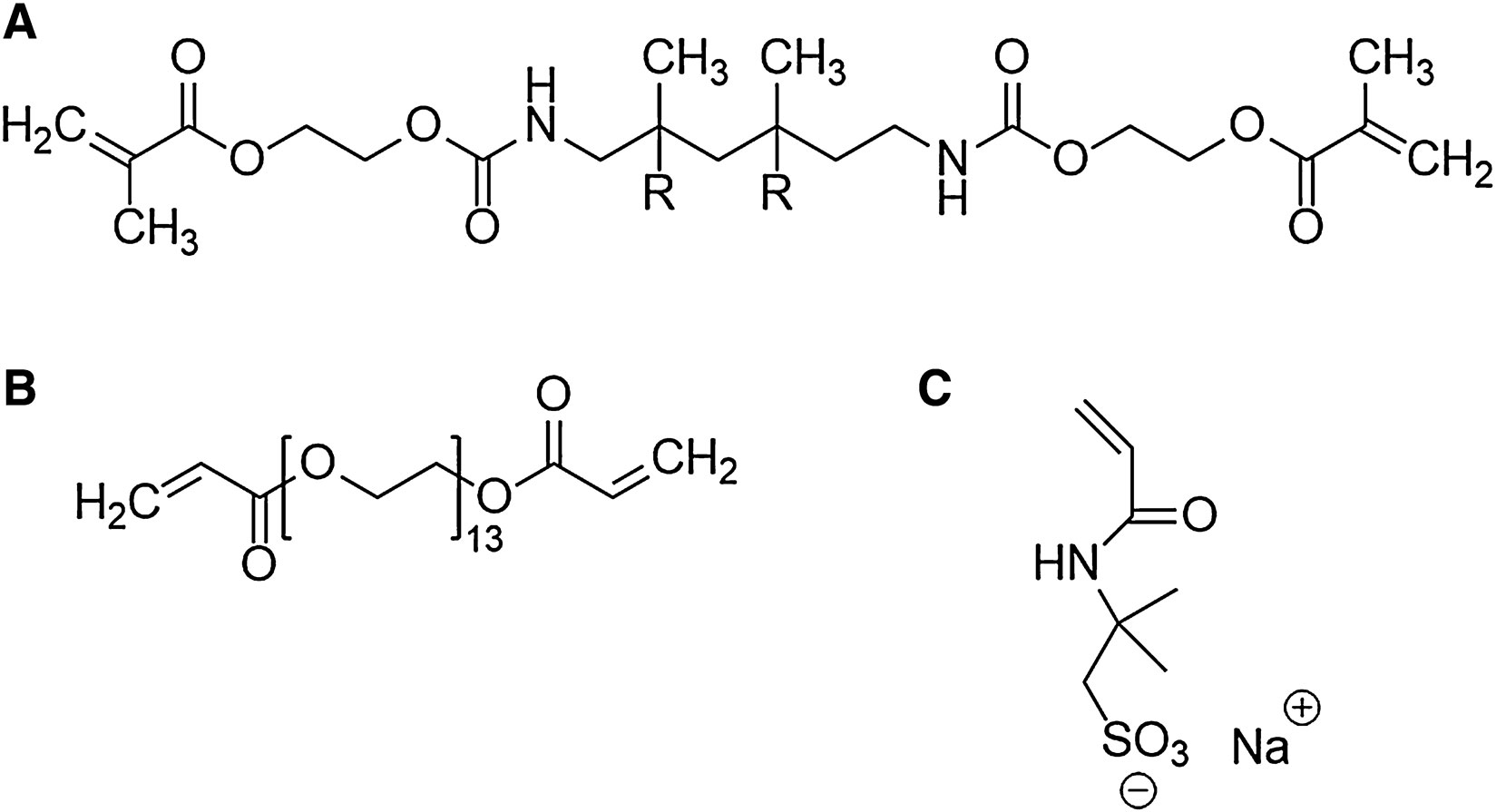
FIGURE 1. Chemical structures of the oligomers and ionic species used in this study: (A) diurethane dimethacrylate, (B) polyethylene glycol diacrylate, and (C) 2-Acrylamido-2-methyl-1-propanesulfonic acid sodium salt.
The electrolyte formulation was prepared by mixing all materials (oligomers, crosslinker, ionic species, plasticizers, initiators, and UV absorber) at the desired ratio in an amber glass jar to prevent unwanted curing from natural light. Dimethyl adipate, PC, glycerol, and diethyl 4-oxopimelate were added to the membrane as plasticizers in a 1:1 molar ratio of plasticizer to ionic group. The initiators and UV absorber were added to the resin at 1 wt% and 0.02 wt%, respectively. Supplementary Table S1 includes the mole fraction and molecular weights of the oligomers (PEGDA and DUDMA), ionic species (Na+ AMPS−), plasticizers, and cross-linker used to create the GPE membrane. The resins were stirred using a magnetic stirrer for at least 12 h and stored in amber glass vials until use. Resins were used for printing within 3 days of formulation. The GPEs were printed by casting 0.5 ml of resin onto a glass plate using a syringe and flattened using a custom-made doctor blade with a thickness of 0.05 mm. The GPEs were cured by placing the glass plate with the resin into a UV curing oven (UVP Ultraviolet Crosslinker, Upland, CA, United States) for 10 min. Upon curing, the membranes were removed from the glass plate using a metal spatula and rinsed with methanol or acetone to remove any uncured resin from the sample. Figure 2 shows the finished free-standing GPE upon completion of photopolymerization and the subsequent chemical structure.
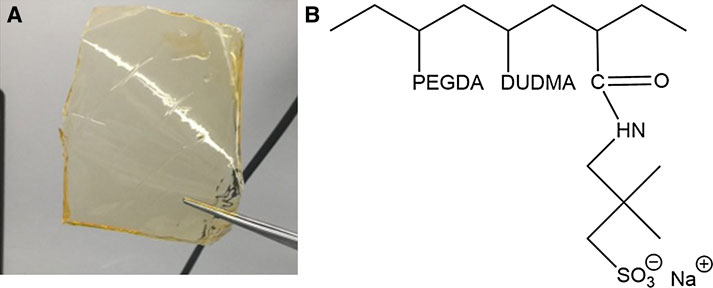
FIGURE 2. (A) Free-standing Na+ gel polymer electrolyte membrane upon completion of UV-curing. (B) Chemical structure of photopolymerized non-solvated Na+ gel polymer electrolyte.
Characterization
All FTIR spectra of the photopolymerized membranes were obtained using a Bruker Vertex 70 FTIR spectrometer (Bruker, Billerica, MA, United States) equipped with a liquid nitrogen-cooled wide band mercury-cadmium-telluride detector. The membranes were directly placed on a ZnSe crystal and analyzed using the HorizonTM multiple reflection attenuated total reflection accessory (Harrick Scientific Products, Inc., Pleasantville, NY, United States) under a dry air purge at ambient temperature. ZnSe crystals were cleaned with 2-butanone between measurements to prevent contaminates in the spectra. The spectra were signal averaged over 400 scans at a resolution of 4 cm−1. All spectra were recorded and analyzed using Bruker OPUS 6.5 software.
Dielectric Relaxation Spectroscopy
Free-standing samples were placed in between a 10 and 20 mm diameter freshly polished brass electrode to form a parallel plate capacitor cell. The sample was squeezed to its thickness of 0.05 mm and placed in the Novocontrol Technologies BDS1400 preparation chamber (Montabaur, Germany), heated under vacuum (∼10−2 Torr). Dielectric measurements were performed using a Novocontrol Technologies Alpha High Resolution Broadband Dielectric/Impedance Spectrometer (Montabaur, Germany) with 0.1 V excitation and no bias in a dry nitrogen environment. Samples were heated above 100°C for 15 min until the conductivity became constant from loss of water, and the spectra were obtained on cooling. Data was collected in isothermal frequency sweeps of 10−1–107 Hz from 120 to 25°C in steps of 5°C using a Quatro temperature control unit.
Results and Discussion
Table 1 shows the range of solvents used in this study and their chemical structures. PC was chosen due to its high dielectric constant, low flashpoint, low vapor pressure, and ability to form solvation complexes with alkali metal ions, thereby improving ionic mobility (Côté et al., 1996; Nasirzadeh et al., 2005; Shakourian-Fard et al., 2015) For this reason, PC is of great interest for use in single-ion conducting polymers(Shakourian-Fard et al., 2015; Flores et al., 2017). Lian et al. reported ionic conductivities of 10–6 to 10–5 S/cm−1 for a series of polyvinyl formal-based Li+ single ion conducting membranes that were plasticized with PC (Lian et al., 2014). Pan et al. have used PC in a series of poly(vinylidene fluoride-co-hexafluoropropylene)-based single ion conductors and have obtained a room temperature ionic conductivity of 0.104 mS/cm−1(Pan et al., 2016). Due to the ability of PC to coordinate with alkali ions and improve ionic conductivity, it is of great interest in this study as a control solvent.
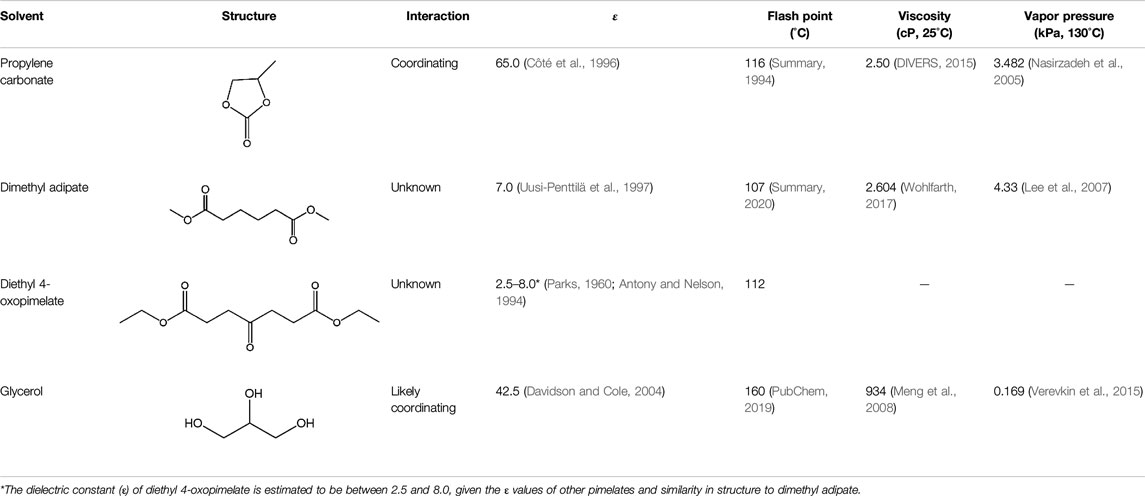
TABLE 1. Physical properties of the solvents investigated in this study and their proposed interaction with sodium ions.
Linear carbonates such as dimethyl carbonate (DMC) are also often employed as solvents in solid polymer electrolytes due to their low viscosity and large electrochemical stability window. DMC has been successfully utilized as a plasticizer in a poly(vinylidene fluoride)-based single-ion conducting GPE in a prior study by Wang et al. (2014). Unfortunately, the low flashpoint of DMC (17°C) can pose a serious safety hazard to consumers and thus limits its widespread use in commercial applications. Adipic-based linear esters such as dimethyl adipate and diethyl 4-oxopimelate have not yet been investigated for electrolytic applications but were chosen for this study due to their low viscosities and vapor pressures (Lee et al., 2007; Wohlfarth, 2017; Summary, 2020). Additionally, the low flashpoint and moderate dielectric constant of these materials may allow for potential usage in future electrolyte applications (Uusi-Penttilä et al., 1997; Lee et al., 2007).
Finally, glycerol boasts a high dielectric constant and flashpoint, making it an ideal potential plasticizer for single-ion conducting applications (Davidson and Cole, 2004; PubChem, 2019). Despite its high viscosity (934 cP), it has been proven to form coordinating complexes with Na+, which may allow for heightened ionic conductivity (Wiens et al., 2014; DIVERS, 2015). Iwaki, et al. have used glycerol as a plasticizer for non-single ion conducting GPEs, and found improvement in conductivity of the plasticized samples (Iwaki et al., 2012). Because this plasticizer is a protic material, it cannot be used in traditional Na+ or Li+ electrolytes, due to the potential hazard of hydrogen gas formation. However, the ability to form coordination spheres with Na+ may be a promising path toward higher ionic conductivity and if the issue of hydrogen gas formation can be addressed, it may be a potential plasticizer for future electrolytic applications.
Fourier-Transform Infrared Spectroscopy
FTIR spectra were collected for each membrane sample to confirm the presence of the plasticizer in the GPE matrix. Additionally, a membrane with no solvent, made under the same conditions, was analyzed to ensure the peaks observed in the FTIR spectra were due to the presence of the plasticizer and not from the membrane material (Figure 3A).
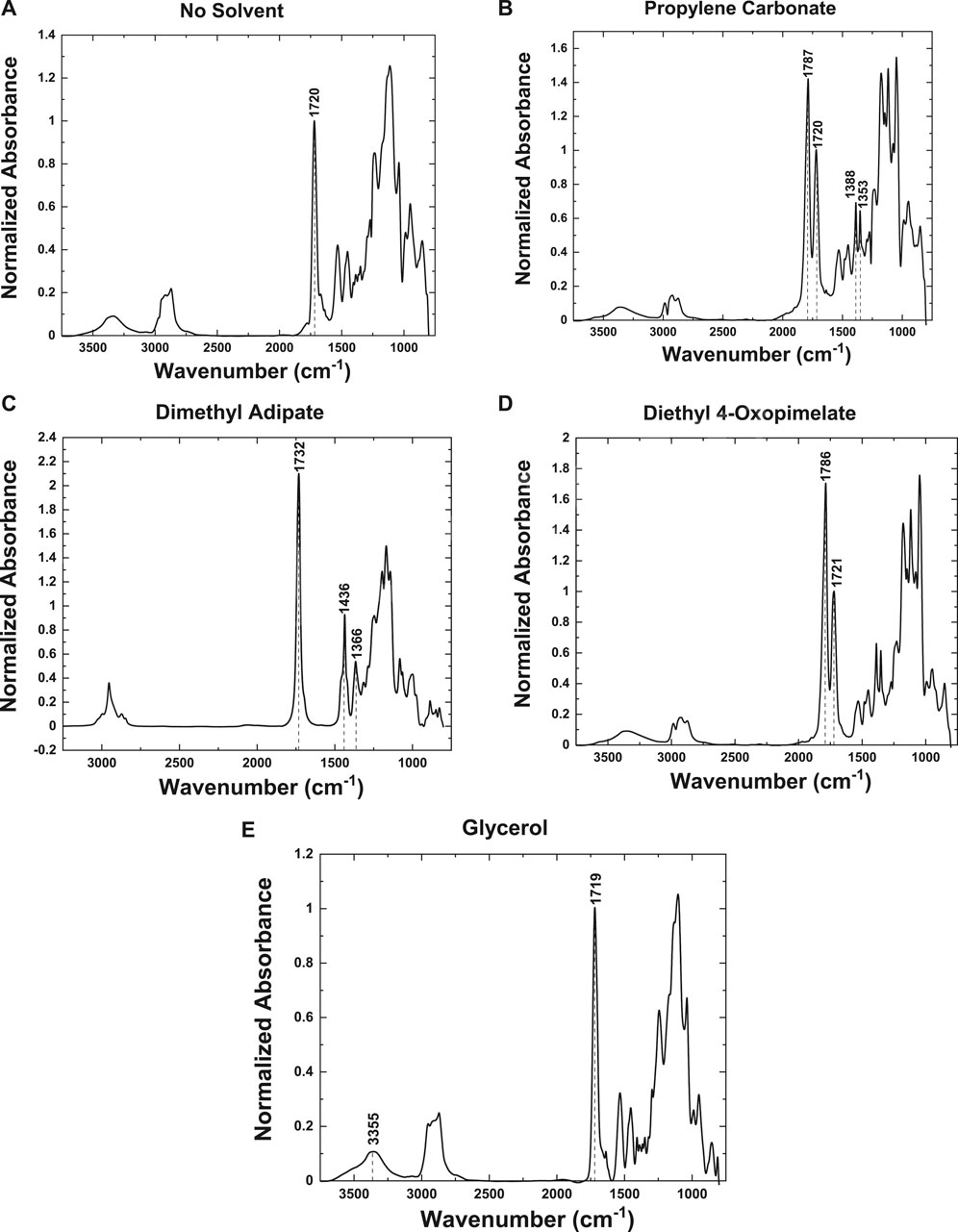
FIGURE 3. FTIR spectra of membranes with the following plasterers: (A) no solvent plasticizer, (B) propylene carbonate, (C) dimethyl adipate, (D) diethyl 4-oxopimelate, and (E) glycerol.
All spectra were normalized to the symmetric stretching mode (ν(s)C=O) at 1,720 cm−1, which is representative of the PEGDA and DUDMA in the membrane (Figure 3A). In Figure 3B, the membrane with the PC plasticizer showed a two intense peaks at 1,787 and 1,720 cm−1 from the symmetric stretch of the carbonyl group (ν(s)C=O) (Ikezawa and Ariga, 2007). The peak at 1,787 cm−1 is most likely due to the PC coordinating with the Na+ ions in the membrane (Wang et al., 2015). This membrane also showed a distinct peak at 1,353 cm−1, corresponding to the symmetric stretch of the ring (ν(s)C-C) and methyl group (ν(s)CH3) (Ikezawa and Ariga, 2007). A distinct peak was also observed at 1,388 cm−1 due to the bending of the methyl group (δ(s)CH3) and ether within the ring (ω O-CH2), thus indicating the presence of the solvent in the membrane (Ikezawa and Ariga, 2007). The membrane using dimethyl adipate as the plasticizer (Figure 3C) presented a distinct narrow peak at 1,436 cm−1, corresponding to the bending mode of the methyl group (δ(s)CH3), and a peak at 1,366 cm−1, corresponding to the bending modes of the C-H bonds within the ring (δ(s)C-H) (Vuksanović et al., 2015). It also presented an intense peak at 1,732 cm−1 from the symmetric stretch of the ether group (ν(s)C=O) (Vuksanović et al., 2015). The frequency shift is due to the Na+ ions in the membrane coordinating with the oxygen from the ether group (Vuksanović et al., 2015; Wang et al., 2015). The membrane with the diethyl 4-oxopimelate plasticizer, as seen in Figure 3D, exhibited an intense peak at 1,721 cm−1, due to the symmetric stretching of the carbonyl group (ν(s)C=O). And it also presented an intense peak at 1,786 cm−1, which is most likely due to the coordination of the Na+ ions in the membrane (Wang et al., 2015). As seen in Figure 3E, the membrane containing glycerol as the plasticizer has few peaks unique from that of the membrane material. However, the normalized intensity of the peak found at 3,355 cm−1, which is indicative of the symmetric stretching of the OH groups (ν(s)OH), has a higher intensity of that of the membrane (Figure 3A) (Ahmed et al., 2010). Hence, demonstrating the presence of glycerol in the membrane.
Ionic Conductivity
Figure 4 shows the ionic conductivity data for the Na+-conducting GPEs membranes as a function of temperature. All solvents were incorporated into the polymer matrix at a 1:1 molar ratio of solvent to ionic group. The GPE samples were heated from 30 to 125°C, cooled from 125 to 30°C, and then heated from 30 to 130°C. Data for Figure 4 was taken from the second heating cycle and Table 2 shows the full heating and cooling cycles to illustrate the complete thermal history of the samples. It is difficult to comment on if the conductivity increased from specific solvents due to higher amounts of solvent retention (Supplementary Figures S1–S4), or on the solvating ability of the specific solvent. But as a whole, all the investigated solvents improved the conductivity of the unsolvated Na+ base membrane. Solvents incorporated into GPEs can increase ionic conductivity by either softening the polymer matrix and lowering the glass transition temperature or by improving dissociation of the ionic species and increasing the amount of mobile charge carriers (Arya and Sharma, 2017; Choi and Colby, 2017). Prior studies by Iwaki et al. have indicated that glycerol can be a successful plasticizer in sodium alginate based GPEs (Iwaki et al., 2012). This may be because the hydroxyl groups in the glycerol act as ligands and form coordination complexes with Na+. Miroshnikov et al. have used solid-state Nuclear Magnetic Resonance (NMR) alongside Density Functional Theory (DFT) computational methods to study coordination interactions of Na+ and found that Na+ forms coordination spheres with hydroxyl functional groups, which was also confirmed by Morisaki et al. through atomic bombardment mass spectroscopy (Morisaki et al., 2002; Miroshnikov et al., 2019). Prior molecular dynamic simulation studies by Wiens et al. have indicated that the hydroxyl groups of glycerol cluster around sodium and promote ionization while photoionization experiments have confirmed the formation of glycerol-Na+ complexes (Wiens et al., 2014; Alexander et al., 2020). The facile formation of Na+ -glycerol solvation structures from the hydroxyl groups in the glycerol as well as the high flashpoint and low vapor pressure of glycerol may explain the heightened conductivity of the glycerol-solvated membranes compared to the unsolvated membranes.
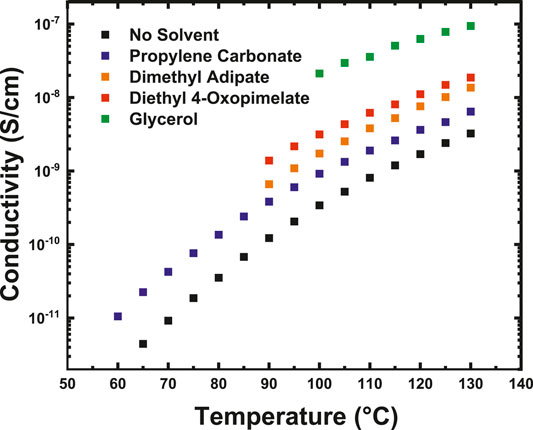
FIGURE 4. Conductivity of the solvated sodium-ion conducting membranes as a function of temperature taken from dielectric relaxation spectroscopy.
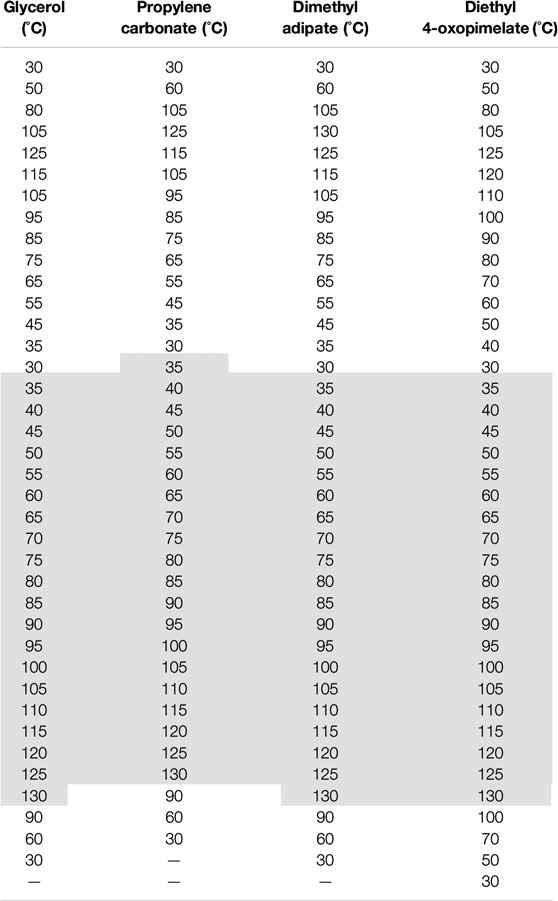
TABLE 2. Full temperature cycle for dielectric relaxation spectroscopy experiments conducted on the solvated membranes in this study. The dark gray shaded region indicates the heating cycle where the conductivity data was taken from.
The linear diesters investigated in this study also showed an improvement in conductivity of the Na+ base membrane. Typically, linear carbonates are employed as solvents in alkali-ion electrolytes, because the carbonate oxygen or ester groups in these groups dissociate the cation from its salt while softening the polymer matrix, thereby improving conductivity (Xu, 2004; Xu, 2014). The additional oxygen in diethyl 4-oxopimelate as compared to dimethyl adipate may allow for higher dissociation of Na+, leading to the higher conductivity.
Finally, the membranes solvated with PC also showed a modest increase in conductivity compared to the Na+ base membrane. PC is often employed as a solvent in traditional alkali- ion liquid electrolytes due to its low viscosity and high dielectric constant (Côté et al., 1996). Ponrouch et al. has conducted a series of experimental tests to study the conductivity of sodium-ion carbonate-based electrolytes and has found favorable room temperature conductivity (10–2 S/cm−1) by using an electrolytic mixture of ethylene carbonate, PC, and DMC (Ponrouch et al., 2013). This high room temperature conductivity is likely due to the ability of PC to dissociate and coordinate with the sodium-ion, promoting ion mobility. Indeed, several molecular dynamic simulations and density functional theory calculation studies have found the successful formation of sodium-ion PC complexes (Shakourian-Fard et al., 2015; Flores et al., 2017). This has also been confirmed experimentally by Geng et al. using Raman spectroscopy (Geng et al., 2019). Because PC exhibits a high dielectric constant, it was expected that incorporation of this solvent would increase the conductivity of the base membrane by several orders of magnitude. It is possible that the low increase in conductivity of the PC membranes stemmed from solvent evaporation during membrane processing. If a way of preserving solvent in the membrane during the measurement is cultivated, it may be possible to improve the conductivity by several orders of magnitude.
Solvent Retention
In order to further study the impact of solvent retention, the changes in ionic conductivity for each of the membranes taken from the heating and cooling cycle were plotted as a function of temperature.
Figure 5A shows the ionic conductivity of the unsolvated membrane as a function of frequency at 125, 115, 105, and 95°C for the first cooling and second heating cycle (shown in Table 2). Because there is no solvent present in the unsolvated membrane, there was no solvent evaporation and hence no change in ionic conductivity between the heating and cooling cycles. One of the overall drawbacks of GPEs is the potential for loss of solvent during heating, which may impact performance (Mustarelli, 2011). Figure 5B shows the conductivity profile of the membrane solvated with PC, which show some solvent evaporation between the heating and cooling cycle. This may be why the ionic conductivity of the PC solvated membranes shown in Figure 5 is moderate despite its high dielectric constant. Figures 5C,D shows the conductivity profile of the membranes solvated with dimethyl adipate and diethyl 4-oxopimelate, respectively. As with PC, there is some loss of solvent which may correspond with lowering in ionic conductivity during thermal cycling. Finally, Figure 5E shows the conductivity profile of the membrane solvated with glycerol. Although there was solvent loss between the heating and cooling cycle, as seen in Figure 4, the glycerol solvated membrane exhibited the highest conductivity of all the membrane studied.
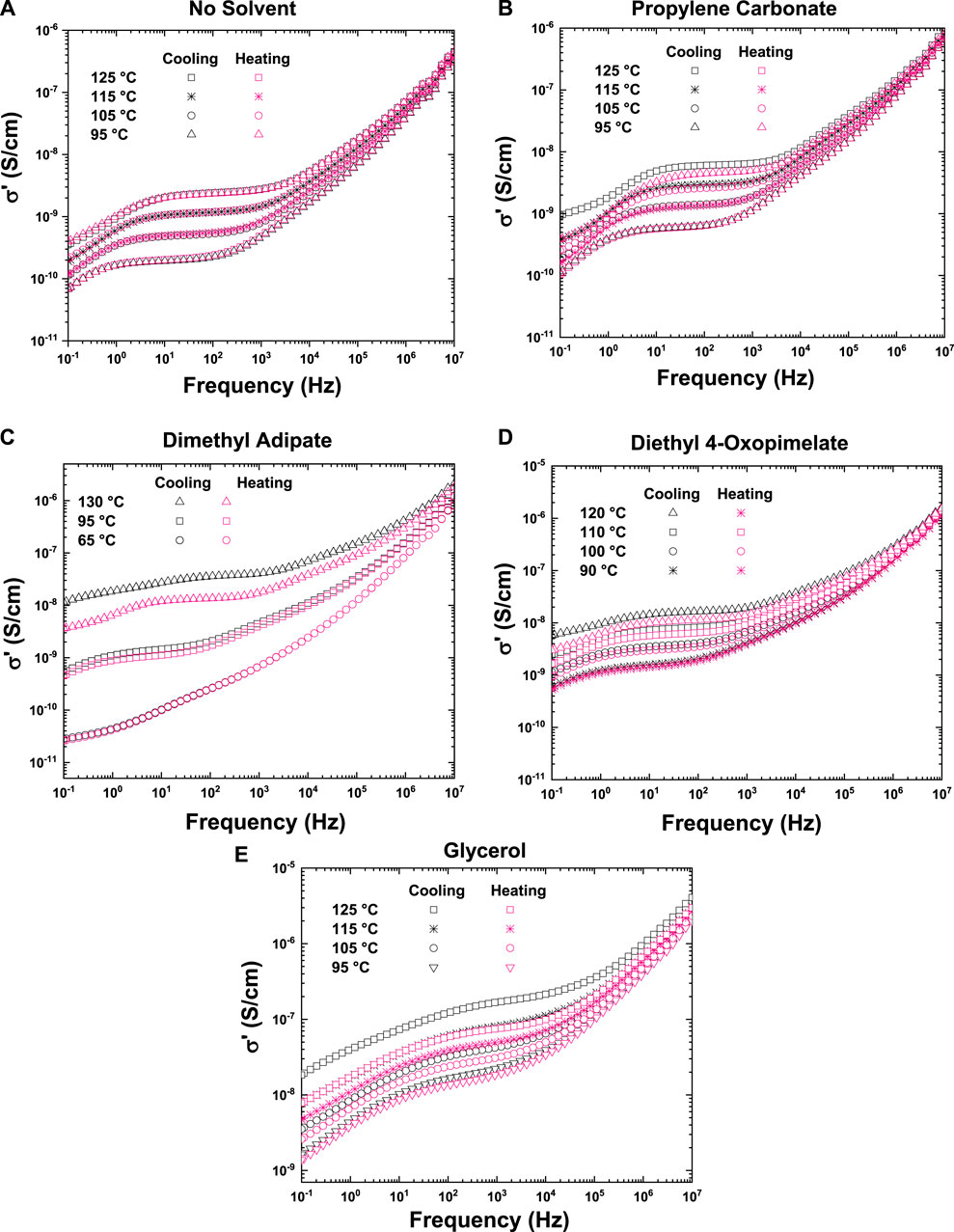
FIGURE 5. Conductivity profile as a function of frequency taken from heating and cooling cycle for unsolvated membrane (A), propylene carbonate solvated membrane (B), dimethyl adipate solvated membrane (C), diethyl 4-oxopimelate solvated membrane (5 days), and glycerol solvated membrane (E).
TGA was used to further study solvent retention in the GPE membranes. By emulating the full temperature cycle performed in the DRS measurements, it was possible quantify solvent loss for the dimethyl adipate, diethyl 4-oxpimelate, and PC-solvated membrane. The dimethyl adipate solvated membrane retained 45 wt% of the initial solvent while the diethyl 4-oxopimelate membrane retained 33 wt% of the solvent (Supplementary Figure S2). With a starting 1:1 molar ratio of solvent to mobile ion, the loss of solvent would indicate that there are more ions in the materials than solvent as the DRS experiments proceeded. TGA was also used to analyze solvent retention in the PC and glycerol-solvated membranes (Supplementary Figure S3). The PC-solvated membrane appears to have lost most of the solvent by the end of the first cooling cycle, which would explain the low ionic conductivity measured from DRS. The glycerol solvated membrane is estimated to have retained 40 wt% of the solvent (Supplementary Figures S3, S4). If a way of preserving solvent in the membrane during the measurement is cultivated, it may be possible to further improve the conductivity of GPEs by several orders of magnitude.
Electrode Polarization Analysis
DC conduction in single-ion conducting polymers can be expressed by:
in which e is charge, µ is ion mobility and p is the number density of ions that are participating in conduction (Wang S. et al., 2011). The electrode polarization model allows the ionic conductivity to be separated into contributions from number density of conducting ions and conducting ion mobility as a function of temperature (Fragiadakis et al., 2009; Lee et al., 2010; Wang W. et al., 2011; Tudryn et al., 2012; Wang and Colby, 2013). Electrode polarization occurs when the mobile ions have enough time to polarize at the interface of the electrodes. This polarization yields an increase in the dielectric constant (from higher capacitance due to the storing of counterions at the electrode surface) and a decrease in the conductivity (due to polarized ions reducing the field experienced by mobile ions) (Ishai et al., 2013). The time scale for conduction when counter ion motion becomes diffusive can be expressed by:
with εs denoted as the static dielectric constant before the onset of electrode polarization and ε0 is the vacuum permittivity. When electrode polarization occurs and the mobile ions start to polarize, the time scale for electrode polarization can be described as follows:
in which εEP is the permittivity upon completion of electrode polarization. The MacDonald and Coelho model then treat electrode polarization as a Debye relaxation with loss tangent (Macdonald, 1953; Coelho, 1983; Fragiadakis et al., 2008):
To this end, τσ and τEP are fitting parameters to then determine εs and εEP. These values can then be used to determine the number density of simultaneously conduction ions (p) and the resulting mobility (µ):
where
Increasing the dielectric constant of a system may be accomplished by incorporating plasticizers with high dielectric constants into the polymer matrix (Liang et al., 2012) Figure 6 shows the static dielectric constant (εs) for each membrane, determined by fitting the measured DC conductivity and τσ at each temperature to Eq. 2. At 85°C, εs of the unsolvated membrane was 5.28, and upon incorporation of the coordinating solvents, εs improved to 8.7 for PC and 39.0 for glycerol. εs values obtained for both the glycerol and PC membranes stayed consistent across the measured temperature range. The improvement in εs in the glycerol-solvated membrane compared to the unsolvated membrane is likely due to the higher dielectric constant of glycerol (42.5 at 25°C) (Davidson and Cole, 2004), while the moderate improvement in εs in PC membranes despite the high dielectric constant of PC (65.0) likely originates from solvent evaporation effects (Supplementary Figure S3). Addition of dimethyl adipate and diethyl 4-oxopimelate increased εs from 5.28 to 65.6 and 45.2, respectively, at 85°C. εs changed by 57.5 over a span of 50°C for the dimethyl adipate solvated membranes and by 29 over a span of 55°C for the diethyl 4-oxopimelate solvated membranes. Both membranes solvated with the linear diesters also showed a linear increase of εs with decreasing temperature, which has been previously seen for other single-ion conducting ionomer systems and is attributed to thermal randomization of dipoles (Fragiadakis et al., 2008; Wang and Colby, 2013; Choi et al., 2014a). It is possible that the large increase in εs of the linear diester-solvated membranes compared to the other studied solvated membranes is due to increased solvation of the ion pair and the subsequent higher dipole moment that follows. Choi et al. have found that incorporating a crown ether into a Li+ conducting ionomer increased the dielectric constant of the system by lengthening the distance between the ion pair from the addition of the solvent, thereby increasing the dipole moment (Choi and Colby, 2017). It is possible that the incorporation of larger linear diester-based solvents increases εs more than coordinating solvents due to higher ion pair solvation and higher enhancement of the dipole moment.
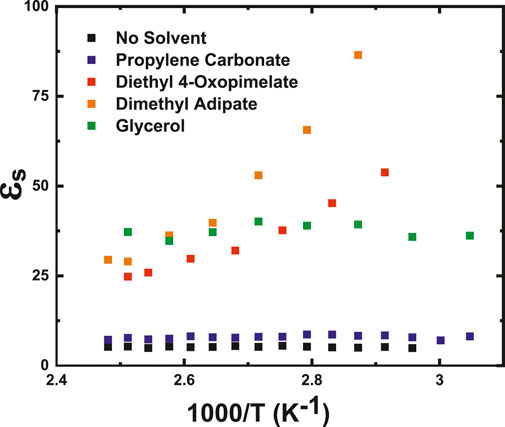
FIGURE 6. Static dielectric constant as a function of temperature for membranes solvated with propylene carbonate, glycerol, dimethyl adipate, and diethyl 4-oxopimelate obtained from fits to the electrode polarization model.
Figure 7 shows the number density of conducting ions as a function of temperature, calculated from Eq. 5. The temperature dependence of the number density of conducting ions for these membranes can be fit to the Arrhenius equation:
where p∞ is the conducting ion concentration as T approaches ∞ and
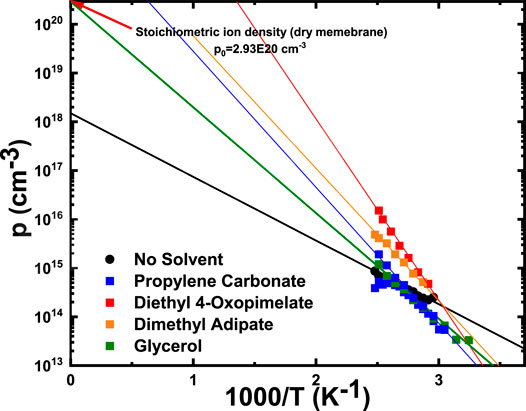
FIGURE 7. Conducting ion concentration as a function of temperature. Solid lines are Arrhenius fits to Eq. 7 with fitting parameters
Finally, it is necessary to discuss the impact of ion aggregation on conducting ion content. Increasing temperature can lead to loss of solvent, which leads to higher formation of aggregates and impact ion conducting content. Dimethyl adipate, diethyl 4-oxopimelate, and PC-solvated membranes exhibited p∞ larger than the stoichiometric ion density (p0), which is non-realistic. This is likely due to the solvent loss during measurement. Lower conductivity was observed at the same temperature after heating and cooling the sample, suggesting solvent evaporation during the measurement at different temperatures. Evaporation of solvents led to a decreased in mobile ion density (ions would aggregate more with less solvation). The data for these membranes were taken from the first cooling cycle after stabilizing the membrane at 130°C. As temperature decreased, the sample contained less and less plasticizer, as it kept evaporating throughout the measurement. As such, the calculated ion content would be lower than the one expected without constant plasticizer loss during the measurement. The overall result is a steeper slope that lead to the extrapolated p∞>p0.
Figure 8 shows the mobility of the simultaneously conducting sodium ions as a function of temperature, determined from the electrode polarization (EP) model. The ion mobilities were also fit to the Vogel-Fulcher-Tammann (VFT) expression:
where µ∞ is the high temperature limit of the mobility, T0 is the Vogel temperature, and D is the strength parameter. These fitting parameters are listed in greater detail in Table 4. The unsolvated membrane, glycerol, and dimethyl adipate membranes exhibited VFT-like behavior, implying that conductivity is coupled to segmental motion. This behavior has been observed among single-ion conducting ionomer systems.(Fragiadakis et al., 2008; Fragiadakis et al., 2009; Choi et al., 2012; Tudryn et al., 2012; Wang and Colby, 2013; Choi et al., 2014a; Choi et al., 2014b; Phys et al., 2015; Choi and Colby, 2017). However, VFT dependence was non-existent for the PC and diethyl 4-oxopimelate membranes, implying possible decoupling of ion motion from segmental relaxation (Tudryn et al., 2012). It is difficult to definitively comment on whether the mobility increased from specific solvents due to higher amounts of solvent retention (Supplementary Figures S2–S4), or on the solvating ability of the solvent. But as a whole, below 100°C, incorporation of solvent improved ionic mobility of all of the solvated membranes. This is likely because addition of these solvents improved flexibility of the polymer matrix thereby increasing the mobility of the sodium ions. Of all the studied membranes, glycerol, and PC yielded the highest improvement in mobility. Glycerol improved the mobility of the unsolvated membrane by 100 times at 100°C while PC improved mobility by 3 times at 100°C. Dimethyl adipate yielded a smaller increase in mobility (2.3 times at 100°C), while diethyl 4-oxopimelate only improved mobility at temperatures under 100°C.

TABLE 3. Dielectric properties of solvents and solvated gel polymer electrolyte membranes determined from electrode polarization analysis.
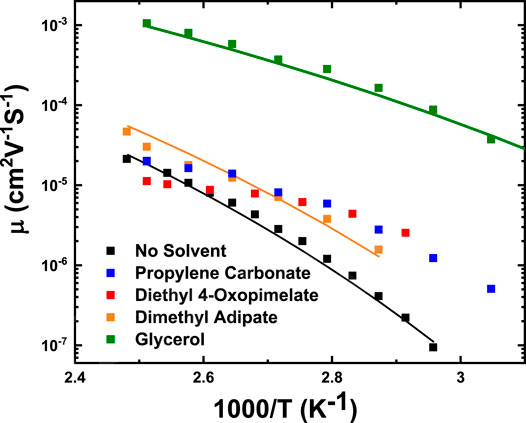
FIGURE 8. Mobility of conducting ions as a function of temperature calculated from Eq. 6. Solid lines are fits to Vogel-Fulcher-Tammann equation, with fitting parameters T0, D, and µ∞ listed in Table 4.
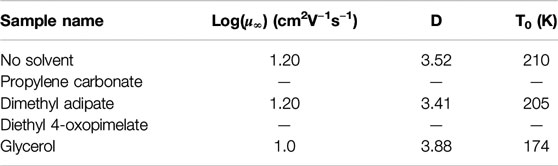
TABLE 4. Fitting parameters of the Vogel-Fulcher-Tammann temperature dependence and mobility of conducting ions.
The glass transition temperature (Tg) can be estimated by (Matsuoka, 1997):
Given T0 decreased for all solvated membranes, incorporation of these solvents likely lowered the Tg of the system and increased chain flexibility, which may be further confirmed using dynamic mechanical analysis.
Investigating the impact of solvents on ionic mobility in single-ion conducting polymers has been a topic of great interest in several research groups (Choi et al., 2014a; Phys et al., 2015; Phys et al., 2016). Typically, solvents that are larger and bulkier lead to lower binding energy between the conducting cation and solvent molecule, which give rise to higher mobility. In our case, the sodium ions solvated by a smaller coordinating solvent such as glycerol exhibited higher mobility than those solvated by the larger diester-based solvents.
The lower mobility of the linear diester solvated membranes may be because the complexes formed between the sodium ion and dimethyl adipate and diethyl 4-oxopimelate are bulkier and less mobile than the complexes formed between the sodium ion and glycerol. The lower mobility of the dimethyl adipate and diethyl 4-oxopimelate membranes is also congruent with the higher εs, which may be due to the enhanced dipole moment that comes from increasing the distance between the Na+ and anionic sulfonate group tethered to the polymer chain. However, further testing must be done to ascertain the reasoning behind the lower mobility for the sodium ion and linear diester-based solvent systems.
Conclusions
In this paper, we have investigated how solvents such as PC, dimethyl adipate, diethyl 4-oxopimelate, and glycerol impact the conductivity profile and polymer dynamics of single-ion Na+ conducting photopolymerized GPE membranes. By employing FTIR, we have proposed mechanisms by which the solvent molecules coordinate with the Na+, which likely impacts the subsequent increase in conductivity of the solvated membranes. DRS was used to characterize the conductivity of the membranes, while the electrode polarization model was utilized to obtain the static dielectric constant and to deconvolute conductivity into contributions from ion conducting content and mobility. We have found that all plasticizers investigated improved the conductivity, static dielectric constant, and mobility of the Na+ GPE base membrane and that using glycerol as a plasticizer yielded a nearly two order of magnitude improvement in conductivity. While it is difficult to ascertain whether the mobility increased due to solvent retention (Supplementary Figures S2–S4), or on the unique solvating ability of the solvent, it was demonstrated that all of the solvents incorporated yielded an increase in ionic conductivity of the non-solvated membrane. This increase is likely from coordination mechanisms between the solvents and Na+, as shown in FTIR. If a method of preserving solvent in the membrane during the measurement can be cultivated, it may be possible to further improve the conductivity of single-ion conducting GPE membranes. The solvents selected all have a flashpoint above 100°C, in order to address the growing concern of flammability in electrolytic devices. The comparisons from this work will assist in developing a deeper understanding of solvent-ion interactions in single-ion conducting GPEs and ultimately help pave the way toward improving conductivity in next-generation polymer electrolytes.
Data Availability Statement
The raw data supporting the conclusions of this article will be made available by the authors, without undue reservation.
Author Contribution
PK conducted and designed all experiments except for FTIR and wrote the entire manuscript (except for the FTIR section). WM conducted the conducting ion content/ion mobility analysis. SS conducted the FTIR experiments and wrote the FTIR section of the manuscript. JS conducted the TGA experiments. CF aided in initial experimental design and setup. CC provided important aid with initial UV curing experiments. RH reviewed conducting ion content/ion mobility analysis. MH provided guidance, suggestions, and insight throughout entire experimental process.
Conflict of Interest
The authors declare that the research was conducted in the absence of any commercial or financial relationships that could be construed as a potential conflict of interest.
Acknowledgments
MH acknowledges the Penn State Materials Research Institute and Penn State Institutes for Energy and the Environment for infrastructure support. The authors also thank U. Hyeok Choi for meaningful discussions regarding material morphology and property relationships and Zitan Huang for discussions regarding solvent retention.
Supplementary Material
The Supplementary Material for this article can be found online at: https://www.frontiersin.org/articles/10.3389/fenrg.2020.569387/full#supplementary-material
References
Ahmed, M. K., McLeod, M. P., Nézivar, J., and Giuliani, A. W. (2010). Fourier transform infrared and near-infrared spectroscopic methods for the detection of toxic Diethylene Glycol (DEG) contaminant in glycerin based cough syrup. Spectroscopy 24 (6), 601–608. doi:10.3233/SPE-2010-048210.1155/2010/608749
Alexander, W. A., Wiens, J. P., Minton, T. K., and Nathanson, G. M. (2020). Reactions of solvated electrons initiated by sodium atom ionization at the vacuum-liquid interface. Science 335 (6072), 1072–1075. doi:10.1126/science.1215956
Antony, A. A., and Nelson, R. D. (1994). Chapter II: tables of dielectric constants, dipole moments, and dielectric relaxation times. Princeton, NJ: Frick Chemical Laboratory. New York, NY. IEEE.
Arya, A., and Sharma, A. L. (2017). Polymer electrolytes for lithium ion batteries : a critical study. Ionics 23, 497–540. doi:10.1007/s11581-016-1908-6
Balakrishnan, P. G., Ramesh, R., and Prem Kumar, T. (2006). Safety mechanisms in lithium-ion batteries. J. Power Sources 155, 401–414. doi:10.1016/j.jpowsour.2005.12.002
Bella, F, Colò, F, Nair, J. R., and Gerbaldi, C. (2017). Light-cured polymer electrolytes for safe, low-cost and sustainable sodium-ion batteries. J. Power Sources 365, 293–302. doi:10.1016/j.jpowsour.2017.08.079
Capparelli, C., Fernandez Pulido, C. R., Wiencek, R. A., and Michael A. Hickner, (2018). Resistance and permselectivity of 3D printed micropatterned anion exchange membranes. ACS Appl. Mater. Interfaces 11, 26298–26306. doi:10.1021/acsami.8b04177
Choi, J. W., and Aurbach, D. (2016). Promise and reality of post-lithium-ion batteries with high energy densities. Nat. Rev. Mater 1, 16013. doi:10.1038/natrevmats.2016.13
Choi, U. H., and Colby, R. H. (2017). The role of solvating 12-crown-4 plasticizer on dielectric constant and ion conduction of poly(ethylene oxide) single-ion conductors. Macromolecules 50 (14), 5582–5591. doi:10.1021/acs.macromol.7b00467
Choi, U. H., Lee, M., Wang, S., and Liu, W. (2012). Ionic conduction and dielectric response of poly(imidazolium acrylate) ionomers. Macromolecules 45, 3974–3985. doi:10.1021/ma202784e
Choi, U. H., Liang, S., Reilly, M. V. O., Winey, K. I., Runt, J., and Colby, R. H. (2014a). Influence of solvating plasticizer on ion conduction of polysiloxane single-ion conductors. Macromolecules 47, 3145–3153. doi:10.1021/ma500146v
Choi, U. H., Ye, Y., Salas, D., Liu, W., Winey, K. I., Elabd, Y. A., et al. (2014b). Dielectric and viscoelastic responses of imidazolium-based ionomers with different counterions and side chain lengths. Macromolecules 47 (2), 777–790. doi:10.1021/ma402263y
Coelho, R. (1983). Sur la relaxation d'une charge d'espace. Rev. Phys. Appl. 18 (3), 137–146. doi:10.1051/rphysap:01983001803013700
Côté, J. F., Brouillette, D., Desnoyers, J. E., Rouleau, J. F., St-Arnaud, J. M., and Perron, G. (1996). Dielectric constants of acetonitrile, γ-butyrolactone, propylene carbonate, and 1,2-dimethoxyethane as a function of pressure and temperature. J. Solution Chem. 25 (12), 1163–1173. doi:10.1007/bf00972644
Dam, T., Jena, S. S., and Ghosh, A. (2019). Ion dynamics, rheology and electrochemical performance of UV cross-linked gel polymer electrolyte for Li-ion battery. J. Appl. Phys. 126 (10), 105104. doi:10.1063/1.5112149
Davidson, D. W., and Cole, R. H. (2004). Dielectric relaxation in glycerol, propylene glycol, and n-Propanol. J. Chem. Phys. 19, 1484. doi:10.1063/1.1748105
DIVERS (2015). Viscosity, Surface tension, specific density and molecular weight of selected liquids print this sort order. Divers 7 (19), 1–5.
Fleischhammer, M., and Doering, H. (2018). “Chemical safety,” in Lithium-Ion Batteries: Basics and Applications. Editor, R. Korthauer (Berlin, Germany: Springer), 263–275. doi:10.1007/978-3-662-53071-9
Flores, E., Åvall, G., Jeschke, S., and Johansson, P. (2017). Solvation structure in dilute to highly concentrated electrolytes for lithium-ion and sodium-ion batteries. Electrochim. Acta 233, 134–141. doi:10.1016/j.electacta.2017.03.031
Ford, H. O., Cui, C., and Schaefer, J. L. (2020). Comparison of single-ion conducting polymer gel electrolytes for sodium, potassium, and calcium Batteries: influence of polymer chemistry, cation identity, charge density, and solvent on conductivity. Batteries 6, 11. doi:10.3390/batteries6010011
Fragiadakis, D., Dou, S., Colby, R. H., and Runt, J. (2008). Molecular mobility, ion mobility, and mobile ion concentration in poly(ethylene oxide)-based polyurethane ionomers. Macromolecules 41 (15), 5723–5728. doi:10.1021/ma800263b
Fragiadakis, D., Dou, S., Colby, R. H., and Runt, J. (2009). Molecular mobility and Li+ conduction in polyester copolymer ionomers based on poly(ethylene oxide). J. Chem. Phys. 130 (6), 064907. doi:10.1063/1.3063659
Geng, C., Buchholz, D., Kim, G. T., Carvalho, D. V., Zhang, H., Chagas, L. G., et al. (2019). Influence of salt concentration on the properties of sodium‐based electrolytes. Small Methods 3 (4), 1800208–1800209. doi:10.1002/smtd.201800208
Hartnig, C., and Schmidt, M. (2018). “Electrolytes and conducting salts,” in Lithium-Ion Batteries: Basics and Applications. Berlin, Germany: Springer, 59–74.
Ikezawa, Y., and Ariga, T. (2007). In situ FTIR spectra at the Cu electrode/propylene carbonate solution interface. Electrochim. Acta 52 (7), 2710–2715. doi:10.1016/j.electacta.2006.09.050
Ishai, P. B., Talary, M. S., Caduff, A., Levy, E., and Feldman, Y. (2013). Electrode polarization in dielectric measurements : a review. Meas. Sci. Technol. 24 (10). doi:10.1088/0957-0233/24/10/102001
Iwaki, Y. O., Escalona, M. H., Briones, J. R., and Pawlicka, A. (2012). Sodium alginate-based ionic conducting membranes. Mol. Cryst. Liq. Cryst. 554, 221–231. doi:10.1080/15421406.2012.634329
Jian, Z., Luo, W., and Ji, X. (2015). Carbon electrodes for K-ion batteries. J. Am. Chem. Soc. 137 (36), 11566–11569. doi:10.1021/jacs.5b06809
Jin, L., Ahmed, F., Ryu, T., Yoon, S., Zhang, W., Lee, Y., et al. (2019). Highly conductive and flexible gel polymer electrolyte with bis (fluorosulfonyl) imide lithium salt via UV curing for Li-ion batteries. Membranes 9, 139. doi:10.3390/membranes9110139
Kerner, M., Lim, D.-H., Jeschke, S., Rydholm, T., Ahn, J.-H., and Scheers, J. (2016). Towards more thermally stable Li-ion battery electrolytes with salts and solvents sharing nitrile functionality. J. Power Sources 332, 204–212. doi:10.1016/j.jpowsour.2016.09.101
Klein, R. J., Zhang, S., Dou, S., Jones, B. H., Colby, R. H., and Runt, J. (2006). Modeling electrode polarization in dielectric spectroscopy: ion mobility and mobile ion concentration of single-ion polymer electrolytes. J. Chem. Phys. 124 (14), 144903. doi:10.1063/1.2186638
Komaba, S., Ishikawa, T., Yabuuchi, N., Murata, W., Ito, A., and Ohsawa, Y. (2011). Fluorinated ethylene carbonate as electrolyte additive for rechargeable Na batteries. ACS Appl. Mater. Interfaces 3, 4165–4168. doi:10.1021/am200973k
Kundu, D., Talaie, E., Duffort, V., and Nazar, L. F. (2015). The emerging chemistry of sodium ion batteries for electrochemical energy storage. Angew. Chem. Int. Ed. (54), 3431–3448. doi:10.1002/anie.201410376
Lee, M., Choi, U. H., Colby, R. H., and Gibson, H. W. (2010). Ion conduction in imidazolium acrylate ionic liquids and their polymers. Chem. Mater. 22 (21), 5814–5822. doi:10.1021/cm101407d
Lee, M., Hong, J., Lopez, J., Sun, Y., Feng, D., Lim, K., et al. (2017). High-performance sodium-organic battery by realizing four-sodium storage in disodium rhodizonate. Nat. Energy 2, 861. doi:10.1038/s41560-017-0014-y
Lee, M.-J., Lai, C.-H., Wang, T.-B., and Lin, H.-M. (2007). Vapor−Liquid equilibrium of mixtures containing adipic acid, glutaric acid, dimethyl adipate, dimethyl glutarate, methanol, and water. J. Chem. Eng. Data 52 (4), 1291–1296. doi:10.1021/je700027n
Leuthner, S. (2018). “Lithium-ion battery overview,” in Lithium-Ion Batteries: Basics and Applications. Editor Korthauer, R. (Berlin, Germany: Springer), 13–19.
Lian, F., Guan, H, Wen, Y, and Pan, X. (2014). Polyvinyl formal based single-ion conductor membranes as polymer electrolytes for lithium ion batteries J. Membrane Sci. 469, 67–72. doi:10.1016/j.memsci.2014.05.065
Liang, S., Choi, U. H., Liu, W., Runt, J., and Colby, R. H. (2012). Synthesis and lithium ion conduction of polysiloxane single-ion conductors containing novel weak-binding borates. Chem. Mater. 24 (12), 2316–2323. doi:10.1021/cm3005387
Liang, S., Yan, W., Wu, X., Zhang, Y., Zhu, Y., Wang, H., et al. (2018). Gel polymer electrolytes for lithium ion batteries: fabrication, characterization and performance. Solid State Ion. 318, 2–18. doi:10.1016/j.ssi.2017.12.023
Long, L., Wang, S., Xiao, M., and Meng, Y. (2016). Polymer electrolytes for lithium polymer batteries. J. Mater. Chem. 4 (26), 10038–10069. doi:10.1039/C6TA02621D
Macdonald, J. R. (1953). Theory of ac space-charge polarization effects in photoconductors, semiconductors, and electrolytes. Phys. Rev. 92 (1), 4–17. doi:10.1103/PhysRev.92.4
Matsuoka, S. (1997). Entropy, free volume, and cooperative relaxation. J. Res. Natl. Inst. Stand. Technol. 102 (2), 213. doi:10.6028/jres.102.017
Meng, X., Zheng, P., Wu, J., and Liu, Z. (2008). Density and viscosity measurements of diethyl adipate from (303 to 373) K and up to 20 MPa. J. Chem. Eng. Data 53 (7), 1474–1478. doi:10.1021/je700740e
Miroshnikov, M., Kato, K., Babu, G., Kumar, N., Mahankali, K., Hohenstein, E., et al. (2019). Nature-derived sodium-ion battery: mechanistic insights into Na-ion coordination within sustainable molecular cathode materials. ACS Appl. Energy Mater. 2 (12), 8596–8604. doi:10.1021/acsaem.9b01526
Morisaki, N., Kobayashi, H., Yamamura, Y., Morisaki, M., Nagasawa, K., and Hashimoto, Y. (2002). Coordination of sodium cation to an oxygen function and olefinic double bond to form molecular adduct ion in fast atom bombardment mass spectrometry. Chem. Pharm. Bull. 50 (7), 935–940. doi:10.1248/cpb.50.935
Mustarelli, P., and Quartarone, E. (2011). Electrolytes for solid-state lithium rechargeable batteries: recent advances and perspectives. Chem. Soc. Rev. 40, 2525–2540. doi:10.1039/c0cs00081g
Nasirzadeh, K., Neueder, R., and Kunz, W. (2005). Vapor pressures of propylene carbonate and N,N-dimethylacetamide. J. Chem. Eng. Data 50 (1), 26–28. doi:10.1021/je049950g
Pan, Q., Chen, Y., Zhang, Y., Zeng, D., Sun, Y., and Cheng, H. (2016). A dense transparent polymeric single ion conductor for lithium ion batteries with remarkable long-term stability. J. Power Sources 336, 75–82. doi:10.1016/j.jpowsour.2016.10.033
Pandey, G. P, and Agrawal, R. C. A. (2008). Solid polymer electrolytes: materials designing and all-solid-state battery applications: an overview. J. Phys. D 41 (22), 223001. doi:10.1088/0022-3727/41/22/223001
Parks, A. M. (1960). “The electrical properties of some solid organic esters,” in Conference on electrical insulation, Washington, DC, October 17–19, 1960, 169–176.
Phys, J. C., Colby, R. H., and Runt, J. (2015). Modeling electrode polarization in dielectric spectroscopy : ion mobility and mobile ion concentration of single-ion polymer electrolytes Modeling electrode polarization in dielectric spectroscopy : ion mobility and mobile ion concentration of single-ion. J. Chem. Phys. 124, 144903. doi:10.1063/1.2186638
Phys, J. C., Fragiadakis, D., Dou, S., Colby, R. H., and Runt, J. (2009). Molecular mobility and conduction in polyester copolymer ionomers based on poly (ethylene oxide). J. Chem. Phys 130, 064907. doi:10.1063/1.3063659
Ponrouch, A., Dedryvère, R., Monti, D., Demet, A. E., Ateba Mba, J. M., Croguennec, L., et al. (2013). Towards high energy density sodium ion batteries through electrolyte optimization. Energy Environ. Sci. 6 (8), 2361–2369. doi:10.1039/c3ee41379a
Safa, M., Chamaani, A., Chawla, N., and El-Zahab, B. (2016). Polymeric ionic liquid gel electrolyte for room temperature lithium battery applications. Electrochim. Acta 213, 587–593. doi:10.1016/j.electacta.2016.07.118
Saravanan, K., Mason, C. W., Rudola, A., Wong, K. H., and Balaya, P. (2013). The first report on excellent cycling stability and superior rate capability of Na3V2(PO4)3for sodium ion batteries. Adv. Energy Mater. 3, 444–450. doi:10.1002/aenm.201200803
Seo, J., Kushner, D. I., and Hickner, M. A. (2016). 3D printing of micropatterned anion exchange membranes. ACS Appl. Mater. Interfaces 8 (26), 16656–16663. doi:10.1021/acsami.6b03455
Shakourian-Fard, M., Kamath, G., Smith, K., Xiong, H., and Sankaranarayanan, S. K. R. S. (2015). Trends in Na-ion solvation with alkyl-carbonate electrolytes for sodium-ion batteries: insights from first-principles calculations. J. Phys. Chem. C 119 (40), 22747–22759. doi:10.1021/acs.jpcc.5b04706
Slater, M. D., Kim, D., Lee, E., and Johnson, C. S. (2013). Sodium-ion batteries. Adv. Funct. Mater. 23 (8), 947–958. doi:10.1002/adfm.201200691
Song, J. Y., Wang, Y. Y., and Wan, C. C. (1999). Review of gel-type polymer electrolytes for lithium-ion batteries. J. Power Sources 77 (2), 183–197. doi:10.1016/S0378-7753(98)00193-1
Stephan, A. M. (2006). Review on gel polymer electrolytes for lithium batteries. Eur. Polym. J. 42 (1), 21–42. doi:10.1016/j.eurpolymj.2005.09.01710.1016/j.eurpolymj.2006.02.006
Summary C (2020). Dimethyl adipate. Available at: https://pubchem.ncbi.nlm.nih.gov/compound/12329#section=Melting-Point (Accessed April 12, 2020).
Tagawa, K., and Brodd, R. J. (2009). Production processes for fabrication of lithium-ion batteries, 181–194. doi:10.1007/978-0-387-34445-4
Tudryn, G. J., Reilly, M. V. O., Dou, S., King, D., Winey, K, Runt, J, et al. (2012). Molecular mobility and cation conduction in polyether − ester − sulfonate copolymer ionomers. Macromolecules 45, 3962–3973. doi:10.1021/ma202273j
Uusi-Penttilä, M. S., Richards, R. J., Torgerson, B. A., and Berglund, K. A. (1997). Spectroscopically determined dielectric constants for various esters. Ind. Eng. Chem. Res. 36 (2), 510–512. doi:10.1021/ie960532h
Verevkin, S. P., Zaitsau, D. H., Emel’Yanenko, V. N., and Zhabina, A. A. (2015). Thermodynamic properties of glycerol: experimental and theoretical study. Fluid Phase Equilibr. 397, 87–94. doi:10.1016/j.fluid.2015.03.038
Vuksanović, J. M., Radović, I. R., Šerbanović, S. P., and Kijevčanin, M. L. (2015). Experimental investigation of interactions and thermodynamic properties of poly(ethylene glycol) 200/400 + dimethyl adipate/dimethyl phthalate binary mixtures. J. Chem. Eng. Data 60 (6), 1910–1925. doi:10.1021/acs.jced.5b00156
Wang, J. H., and Colby, R. H. (2013). Exploring the role of ion solvation in ethylene oxide based single-ion conducting polyanions and polycations. Soft Matter 9, 10275–10286. doi:10.1039/c3sm51460a
Wang, J.-H. H., Yang, C. H.-C., Masser, H., Shiau, H.-S., O’Reilly, M. V., Winey, K. I., et al. (2015). Ion states and transport in styrenesulfonate methacrylic PEO9 random copolymer ionomers. Macromolecules 48 (19), 7273–7285. doi:10.1021/acs.macromol.5b01524
Wang, S., Liu, W., and Colby, R. H. (2011). Counterion dynamics in polyurethane-carboxylate ionomers with ionic liquid counterions. Chem. Mater. 23, 1862–1873. doi:10.1021/cm103548t
Wang, S., Wei, C., Ding, W., Zou, L., Gong, Y., Liu, Y., et al. (2019). High-voltage sulfolane plasticized UV-curable gel polymer electrolyte. Polymers 11 (8), 1306–1312. doi:10.3390/polym11081306
Wang, W., Tudryn, G. J., Colby, R. H., and Winey, K. I. (2011). Thermally driven ionic aggregation in poly(ethylene oxide)-based sulfonate ionomers. J. Am. Chem. Soc. 133 (28), 10826–10831. doi:10.1021/ja201405v
Wang, X., Liu, Z., Kong, Q., Jiang, W., Yao, J., Zhang, C., et al. (2014). A single-ion gel polymer electrolyte based on polymeric lithium tartaric acid borate and its superior battery performance. Solid State Ion. 262, 747–753. doi:10.1016/j.ssi.2013.09.007
Wen, Y., He, K., Zhu, Y., Han, F., Xu, Y., Matsuda, I., et al. (2014). Expanded graphite as superior anode for sodium-ion batteries. Nat. Commun. 5, 1–10. doi:10.1038/ncomms5033
Wiens, J. P., Nathanson, G. M., Alexander, W. A., Minton, T. K., Lakshmi, S., and Schatz, G. C. (2014). Collisions of sodium atoms with liquid glycerol: insights into solvation and ionization. J. Am. Chem. Soc. 136 (8), 3065–3074. doi:10.1021/ja4106144
Wohlfarth, C. (2017). “Viscosity of dimethyl adipate,” in Viscosity of Pure Organic Liquids and Binary Liquid Mixtures. Editor, M. D. Lechner (Berlin, Germany: Springer), 320. doi:10.1007/978-3-662-49218-5_293
Xu, K. (2004). Nonaqueous liquid electrolytes for lithium-based rechargeable batteries.Chem. Rev. 104, 4303–4417. doi:10.1021/cr030203g
Xu, K. (2014). Electrolytes and interphases in Li-ion batteries and beyond. Chem. Rev. 114, 11503–11618. doi:10.1021/cr500003w
Yabuuchi, N., Kubota, K., Dahbi, M., and Komaba, S. (2014). Research development on sodium-ion batteries. Chem. Rev. 114, 11636–11682. doi:10.1021/cr500192f
Keywords: single ion conductors, gel polymer electrolytes, coordinating solvents, sodium-ion electrolyte, ionomers, solid polymer electrolyte
Citation: Kuray P, Mei W, Sheffield SE, Sengeh J, Pulido CRF, Capparelli C, Hickey RJ and Hickner MA (2020) Ion Transport in Solvated Sodium-Ion Conducting Gel Polymer Electrolytes. Front. Energy Res. 8:569387. doi: 10.3389/fenrg.2020.569387
Received: 03 June 2020; Accepted: 31 August 2020;
Published: 08 October 2020.
Edited by:
Tomonori Saito, Oak Ridge National Laboratory (DOE), United StatesReviewed by:
U. Hyeok Choi, Inha University, South KoreaMinjae Lee, Kunsan National University, South Korea
Xi Chen, Oak Ridge National Laboratory (DOE), United States
Copyright © 2020 Kuray, Mei, Sheffield, Pulido, Capparelli, Hickey and Hickner. This is an open-access article distributed under the terms of the Creative Commons Attribution License (CC BY). The use, distribution or reproduction in other forums is permitted, provided the original author(s) and the copyright owner(s) are credited and that the original publication in this journal is cited, in accordance with accepted academic practice. No use, distribution or reproduction is permitted which does not comply with these terms.
*Correspondence: Michael A. Hickner, bWFoNDlAcHN1LmVkdQ==