- 1Laboratorio di Fisiologia delle Alghe e delle Piante, Dipartimento di Scienze della Vita e dell’Ambiente, Università Politecnica delle Marche, Ancona, Italy
- 2CIRCC, Università di Bari, Bari, Italy
The need to reduce the CO2 footprint of human activities calls for the utilization of new means of production and new sources of products. Microalgae are a very promising source of a large variety of products, from fuels to chemicals for multiple industrial applications (e.g., dyes, pharmaceutical products, cosmetics, food and feed, new materials for high tech manufacture), and for processes such as wastewater treatment. Algae, as photosynthetic organisms, use light to energize the synthesis of organic matter and differently from most terrestrial plants, can be cultured on land that is not used for crop production. We describe the main factors contributing to microalgae productivity in artificial cultivation systems and discuss the research areas that still need investigation in order to pave the way to the generation of photosynthetic cell factories. We shall comment on the main caveats of the possible mode of improving photosynthetic efficiency and to optimize the partitioning of fixed C to products of commercial relevance. We address the problem of the selection of the appropriate strain and of the consequences of their diverse physiology and culture conditions for a successful commercial application. Finally, we shall provide state of the art information on cell factories chassis by means of synthetic biology approaches to produce chemicals of interest.
Introduction
Climate changes presently occurring on Earth point toward the need to reduce the CO2 anthropogenic emissions and call for the utilization of renewable sources of products, especially of fuels. CO2 accounts for about 76% of total greenhouse gases which are emitted for the most part (72%) by the energy production sector (International Energy Agency, 2019). Global CO2 emissions in 2019 flattened at around 33 Gt and the reason has been ascribed to clean energy transition happening in the power sector (International Energy Agency, 2020a). Among renewable sources of energy, biomass (that includes agriculture and forest residues, energy crops, and algae) contains stored energy from the sun.
Sunlight is an almost limitless source of energy, with about 100000 TW y–1 reaching our Planet. It is a massive amount compared to our current energy consumption of about 15 TW y–1, and to its forecasted increase to about 45 TW y–1 by the end of this century (Barber, 2009; Benedetti et al., 2018). Even though photosynthetic organisms are already able to store about 100 TW y–1, land plants primarily store the energy as lignocellulose, a biopolymer which is not easily exploited as renewable feedstock (Barber, 2009; Aro, 2016). Yet, half of Earth photosynthesis is run by algae in aquatic environments. Conversely to land plants, algal cells do not contain lignin, and the photosynthetically fixed carbon (C) is readily recycled in the ecosystems through the food web (Barber, 2009). Algae display other advantages for industrial applications with respect to land plants. They show higher growth rates, all their biomass is photosynthetically active, and they photosynthesize all year around, leading to about twice as much projected yield per acre with respect to land plants (Chisti, 2007; Clarens et al., 2010, International Energy Agency, 2017). Further, they do not compete for arable land with edible plants, and, in the case of marine species, they avoid the use of drinkable water, highly valuable features considering the forecasted worldwide population increase and future food demand.
Algae as oxygenic phototrophs include, in a broad definition, both prokaryotic and eukaryotic organisms (Raven and Giordano, 2014). In eukaryotic algae two major plastid lineages are observed. The green lineage includes mainly Chlorophyta (green algae) species. The red lineage encloses Rhodophyta (red algae) and several phylogenetic groups originated from secondary endosymbiotic events, like diatoms (Bacillariophyta) (Raven and Giordano, 2014). Microalgae show a wide range of morphologies and cell sizes (1 μm -1 mm) (Giordano and Wang, 2018). They live in marine, freshwater and terrestrial environments, colonizing even habitats characterized by extreme conditions (Raven and Giordano, 2014; de Vargas et al., 2015). They also display a wide range of metabolic diversity, representing a valuable natural source of multiple compounds (Brodie et al., 2017), from biofuels to pharmaceutical products, cosmetics, food and feed, new materials for high tech manufacture (Table 1). Carbohydrate-rich microalgal feedstock is also a suitable substrate for fermentative processes to synthesize fine chemicals (such as succinic acid and lactic acid) (Wang et al., 2013; Lee et al., 2017). Further, life cycle analysis and product environmental footprint assessed that microalgae-based products are sustainable, clean and contribute to waste valorization (Dietrich et al., 2017).
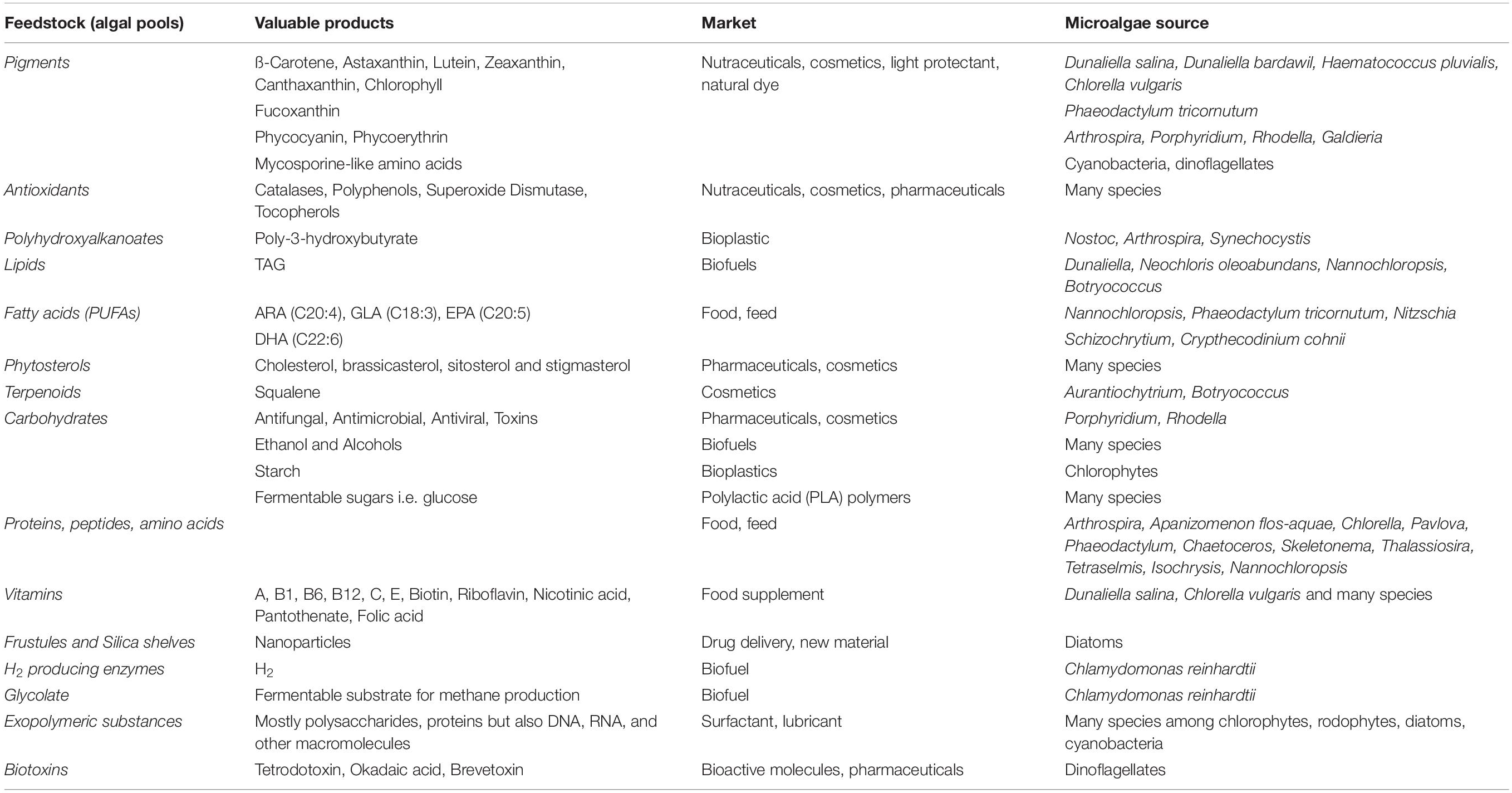
Table 1. Major natural products from microalgae (Gallardo-Rodríguez et al., 2012; Borowitzka, 2013; Enzing et al., 2014; Giordano and Wang, 2018; Kamalanathan and Quigg, 2019 and references there in; Taubert et al., 2019).
Although microalgae have been commercially cultured for over 40 years, their biomass is still quite scant on the market, nowadays ranging around 13600 t y–1, which corresponds to about 27200 t y–1 of CO2 (International Energy Agency, 2017; Morales et al., 2018). Currently, the lacunose understanding of the biological constraints on algal photosynthesis and growth, particularly in large-scale production plants, hampers a cost-effective exploitation of algal biomass as a new mean for CO2 capture into bioenergy feedstock and as a cheap source of commercial products. Thus, future efforts shall avail a deeper comprehension of physiological and environmental factors controlling microalgal resource allocation to the multiple metabolic pathways. In this way, beside selecting the best natural CO2 fixers and producers of fine chemicals, we shall also design photosynthetic living factories converting sunlight and inorganic (or recycled) nutrients into valuable biomolecules, at costs which make the biological production system economically viable.
Although technological advances in large scale production plants are also necessary for a sustainable algae-based industry, in this review we will focus on the key biological factors limiting microalgae photosynthetic efficiency and physiological processes associated to their productivity. We will outline how the interplay between algal genotypes and resource availability affects the biomass quality. We shall address open challenges and possible solutions to achieve higher product yields.
Main Factors Affecting Microalgae Co2 Fixation in Nature
Light Capture and Conversion Into Chemical Energy
Photosynthetic organisms currently convert into biomass about 0.1% of the sunlight energy reaching Earth (Barber, 2009; Benedetti et al., 2018). Photosynthetic efficiency increases to 1%, or seldomly to 3%, under controlled growth conditions (Zhu et al., 2008; Melis, 2009; Cotton et al., 2015), while the theoretical maximum of oxygenic photosynthesis energy conversion into biomass is estimated to be about 10-12% (Zhu et al., 2008; Melis, 2009; Blankenship et al., 2011; Peers, 2014).
To convert sunlight into biomass, oxygenic photosynthetic organisms first capture light by means of light harvesting pigments. Energy is then transferred to the photosystem (PS) reaction center (RC), where charge separation occurs. Electrons move along the thylakoid multiprotein complexes PSII, cytb6f, PSI, to generate reductants and ATP synthase produces ATP. Reducing power and ATP are then used by several metabolic reactions (see Figure 1 for further details).
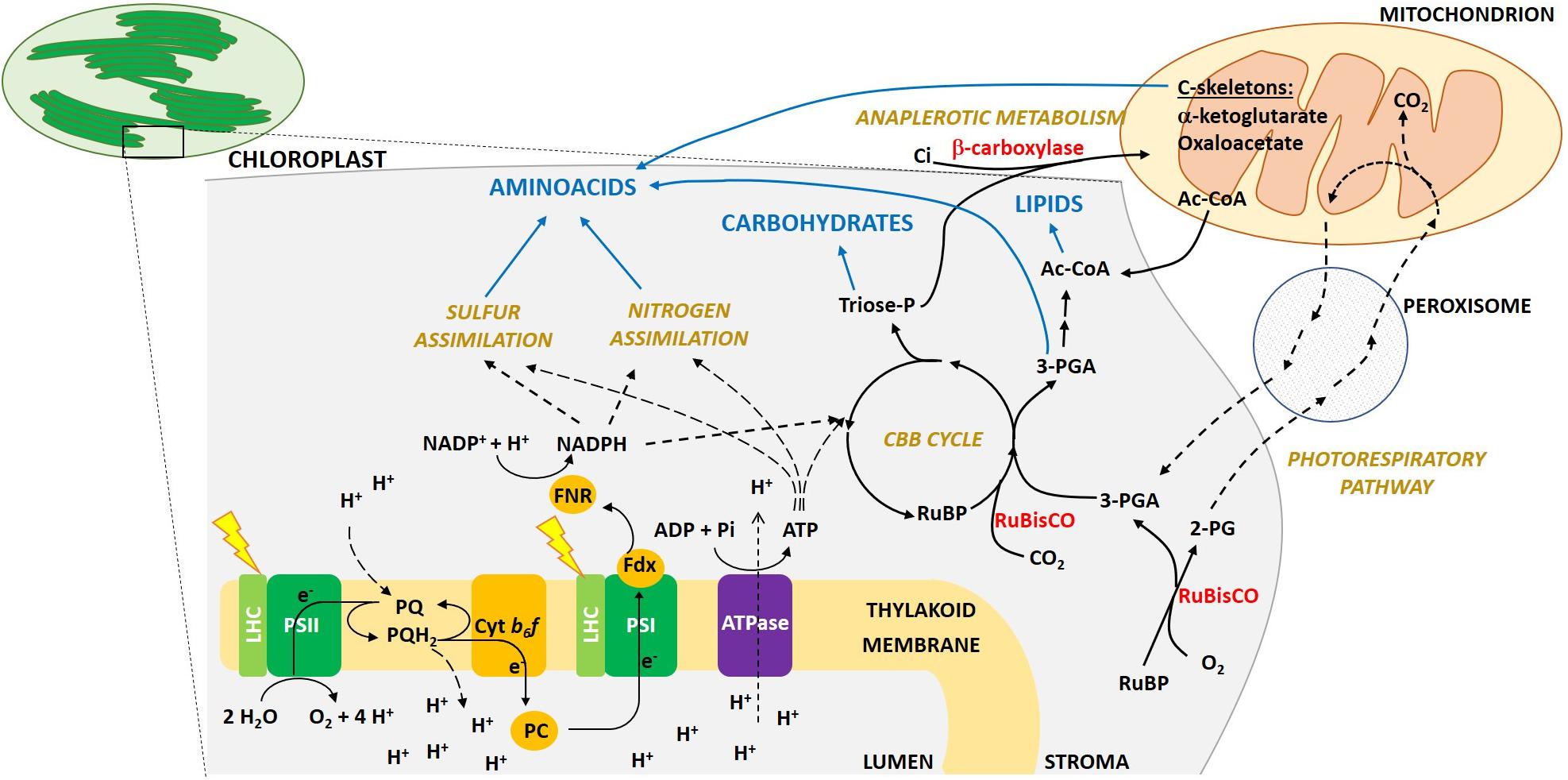
Figure 1. Schematic overview of photosynthesis light reactions, C fixation and allocation into macromolecule pools in eukaryotic algae. The main protein complexes involved in photosynthetic light capture and electron transport are located in the thylakoid membranes inside the chloroplast (PSII, Photosystem II; LHC, Light Harvesting complexes; cytb6f, cytochrome b6f; PSI, Photosystem I; ATPase, ATP synthase). The absorption of light energy at PSII and PSI drives the linear electron transport from water to NADPH. Electrons extracted from water at the luminal side of PSII, thanks to the membrane soluble electron carrier plastochinol (PQ/PQH2, plastoquinone/plastoquinol), move to the cytb6f, which then transfers an electron to plastocyanin (PC), a luminal protein. PC reaches PSI, which then reduces Ferredoxin (Fdx) on its stromal side. Fdx interacts with Ferredoxin NADPH reductase (FNR) to finally reduce NADP+ to NADPH. Regulatory proteins involved in the dissipation as heat of the absorbed exceeding energy (e.g., LHCSR protein), or involved in alternative electron transport routes (e.g., FLV, PGRL1, NDH-1), are not included in the picture. In parallel to electron transport, a proton gradient is generated across the thylakoid membrane, which is used by the ATP synthase to produce ATP. ATP and reducing power generated during electron transport are then used for C fixation in the Calvin-Benson-Bassham (CBB) cycle, as well as for N and S assimilation and other metabolic pathways. Only the main metabolic intermediates and enzymes are shown. Rubisco enzyme catalyzes the carboxylation of Ribulose-1,5-bisphosphate (RuBP), using CO2 and producing two molecules of 3-phosphoglycerate (3-PGA). 3-PGA is then reduced to triose phosphates sugars (Triose-P; GAP and DHAP). Both 3-PGA and triose-P can be allocated into carbohydrates, lipids and amino acids synthesis, or used to regenerate RuBP in the regeneration phase of the CBB cycle. C-skeletons are also imported from mitochondria, mainly in the form of α-ketoglutarate, oxaloacetate or Acetyl-CoA. Note that even if here we are focusing mainly in photosynthesis and we depict macromolecules synthesis only in the chloroplast stroma, in the cell multiple pathways exist to metabolize sugars, lipids and amino acids also in other cellular compartments. Rubisco, as detailed in the text, also catalyzes an oxygenation reaction, producing from RuBP and molecular oxygen a molecule of 3-PGA, which enters the CBB cycle, and a molecule of 2-phosphoglycolate (2-PG). The latter is first dephosphorylated to glycolate in the chloroplast stroma. The glycolate is then exported from the chloroplast and secreted from the cell or enters a dedicated salvage pathway, the photorespiration, which in eukaryotic cells involves several reactions taking place also in peroxisomes and mitochondria. Part of the C is lost as CO2 (25%), while the reaming part is converted back to 3-PGA and metabolized as above.
Already during the so-called light reactions of photosynthesis several factors contribute to the reduced final yield (Figure 2).
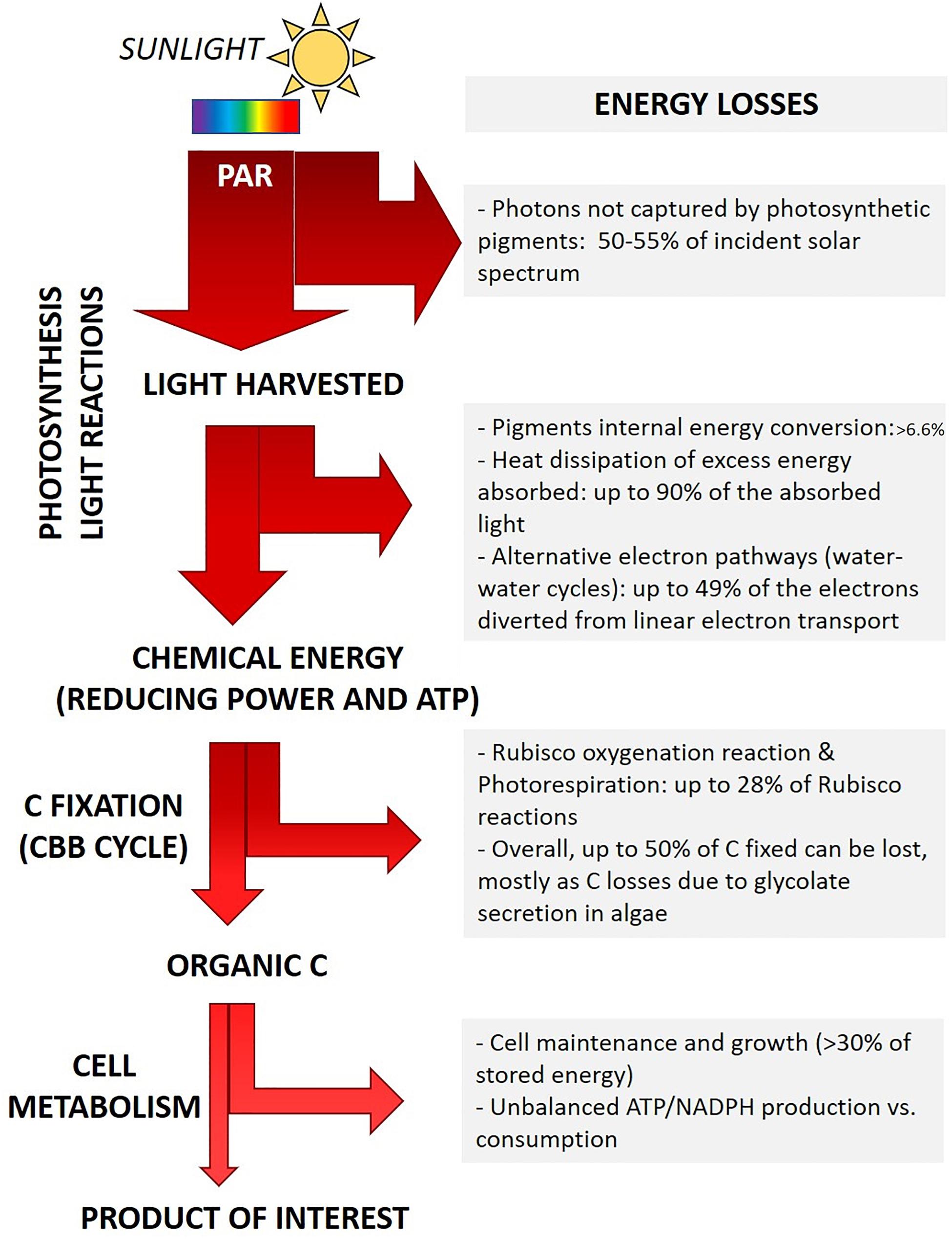
Figure 2. Outline of main energy losses during light energy conversion into biomass. Major biological processes responsible for energy loss are summarized, the corresponding estimated amount of energy dissipated is also outlined (Tabita et al., 2008; Zhu et al., 2008; Blankenship et al., 2011; Wilhelm and Selmar, 2011; Peers, 2014). The actual fraction of energy loss depends on the microalgal genotype and on the specific growth conditions (light intensity and quality, temperature, nutrients concentration and chemical form), as detailed in the text.
(i) Energy loss due to light quality. Some energy losses are intrinsic to the light-harvesting pigments. Each pigment is capable of capturing specific light wavelengths of the visible spectrum, each corresponding to the energy required to promote an electron from the ground state to different excited states. E.g., in chlorophylls, red photons promote electrons to the lowest singlet excited state, while more energetic blue photons span the energy gap to higher excited states. In order to drive photochemistry, pigment molecules first require the internal conversion of such higher excited states to the lowest singlet excited state. The extra energy of high energy photons (e.g., blue photons) is lost as heat before energy transfer and charge separation may occur (Barber, 2009; Johnson, 2016; Figure 2). All species performing oxygenic photosynthesis accumulate chlorophyll a (Chl a) as the main pigment. Primary accessory pigments vary according to the phylogenetic groups and range among Chl b (green algae), Chl c (e.g., diatoms) or phycobilins (cyanobacteria and red algae). Multiple carotenoids involved both in light harvesting and in photoprotection are also synthesized. Only the fraction of incident sunlight which can be absorbed by photosynthetic cells is “photosynthetically active” (Photosynthetically Active Radiation - PAR, 400–700 nm), whereas almost half of the solar irradiance spectrum cannot be captured and it is not energizing photosynthesis (Cardona et al., 2018; Figure 2). Rarely, Chl d or Chl f are also synthesized, expanding PAR to the far-red region (till 750 nm) (Cardona et al., 2018; Nürnberg et al., 2018). Introducing pathways to synthesize such far-red absorbing pigments has been suggested as a tool to widen the range of captured light (Cardona et al., 2018). The use of strictly red and far-red light sources would reduce this kind of light energy loss, yet this approach has the drawback to require artificial light sources (Cotton et al., 2015). Recently, new materials able to convert unabsorbed photons with higher energy (e.g., green photons) into red photons have also been developed, possibly contributing to reduce the fraction of unexploited light energy in industrial applications (Ooms et al., 2016).
(ii) Energy loss due to light intensity. In the Nature, the ability to harvest as much sunlight energy as possible, even exceeding cell metabolic demand, is likely to provide a competitive advantage to individuals, as this behavior minimizes the energy harvested by nearby competitors and thus their growth (Melis, 2009). Further, natural light intensity frequently fluctuates from being limiting to in excess. Photosynthetic organisms thus evolved the ability to fine tune the amount of energy spent in photochemistry or dissipated. Energy dissipation is minimal when light irradiance is low and all available energy drives photosynthesis. Conversely, under saturating illumination, the fraction of energy and/or electrons in excess compared to the metabolic demand is safely dissipated (Peers et al., 2009; Peltier et al., 2010; Wilhelm et al., 2014; Allahverdiyeva et al., 2015; Lepetit et al., 2017). Microalgae display different amplitude and combination of molecular mechanisms to dissipate excess energy, according to their phylogenetic group and habitat (i.e., growth conditions) (Peers et al., 2009; Peltier et al., 2010; Gerotto and Morosinotto, 2013; Meneghesso et al., 2016). Nature-driven evolution of the light harvesting regulation is essential for photosynthetic cells to cope with natural light variations (Wilhelm and Selmar, 2011; Niyogi and Truong, 2013). However, it turns detrimental for the productivity of photosynthetic organisms in commercial application, as it leads to the dissipation of most of the energy harvested, up to 80-90%, in saturating light conditions (Wilhelm and Selmar, 2011; Peers, 2014; Figure 2). Noteworthy, in natural environments light saturation of photosynthesis usually occurs at around 10-20% of full sunlight intensity (Melis, 2009; Peers, 2014).
A similar situation occurs in dense microalgal cultures such as those in artificial cultivation systems, where cells of the external layer experience over-saturating illumination and dissipate most of the energy or, in the worst case, suffer of photoinhibition. On the contrary, the inner layer of cells is subjected to light limitation (Melis, 2009; Simionato et al., 2013).
Inorganic Carbon Capture and Conversion
CO2 in the atmosphere is nowadays above 400 ppm, while future scenarios assume a peak of 750 ppm by the end of this century, according to the Fifth Assessment Report (AR5) of the International Panel of Climate Change [IPCC] (2014). Due to anthropogenic emissions, a net flux of CO2 from the atmosphere has been reaching the oceans thanks to CO2 dissolution and biological fixation (Falkowski and Raven, 2007). The former mechanism depends on atmospheric CO2 pressure, temperature, salinity and pH. At the usual pH of seawater, around 8.0–8.3, the dissolved inorganic carbon (DIC) is mainly in the form of HCO3–, so that the equilibrium concentration of CO2 ranges between 10 and 20 μM in present oceans (Falkowski and Raven, 2007).
Among DIC species, only CO2 is the chemical form of inorganic carbon (Ci) fixed by Ribulose-1,5-bisphosphate Carboxylase/Oxygenase (Rubisco) enzyme into carbohydrates. Rubisco is the major carboxylase on Earth and, likely, the most common enzyme in the biosphere (Ellis, 1979). Carboxylation of the pentose phosphate sugar ribulose-1,5-bisphosphate (RuBP) by Rubisco produces two 3-phosphoglicerate (3-PGA) molecules (Figure 1). Despite being so widespread in oxygenic photosynthetic organisms, Rubisco is a quite inefficient enzyme (Falkowski and Raven, 2007). The reaction has a slow turnover rate (i.e., a low Kcatc) and it needs relatively high concentrations of CO2 (elevated Michaelis-Menten constant, Km, for CO2). In addition, O2 is a competitive inhibitor of the carboxylation reaction. When oxygenation of RuBP occurs, a molecule of phosphoglycolate (2-PG) is produced together with one of 3-PGA (Figure 1). The latter enters the Calvin-Benson-Bassham (CBB) cycle, while 2-PG is a toxic compound and not a common metabolic intermediate (Hagemann et al., 2016). The 2-PG phosphate is first recovered by hydrolysis and the glycolate is excreted in variable quantity from the cell (Raven et al., 2000). The glycolate retained inside the cell enters a dedicated salvage pathway, the photorespiration, accomplished in the chloroplast, peroxisome and mitochondrion of eukaryotic algae (see Hagemann et al., 2016 for evolutive details). In this pathway, glycolate is metabolized and up to 75% of the carbon is recovered in the form of 3-PGA, whereas the remaining 25% is lost as CO2 (Figure 1). Rubisco oxygenation side-reaction thus impacts photosynthetic organisms’ final productivity in two ways (Figure 2). First, due to the high concentration and solubility of O2, the amount of C lost because of 2-PG formation can be appreciable and decreases photosynthetic efficiency in C fixation. Second, photorespiration increases the energetic cost associated with photosynthesis (Raven et al., 2000).
In the course of evolution, Rubisco CO2/O2 selectivity factor (τ = VmaxCO2 KmO2/VmaxO2 KmCO2) showed a tendency to increase as compared to the value in the more primitive cyanobacteria, partially mitigating the impact of oxygenation (Table 2; Tabita et al., 2008). Half-saturation constant for CO2 (Km for CO2) also decreased. However, the maximal reaction rate (Kcatc) became lower (Table 2). The highest known value of τ is 238 and it is found in red algae (Raven et al., 2000). Diatoms show instead a greater variation in τ and KmCO2 values which do not follow the evolutive trend (Table 2; Young and Hopkinson, 2017).
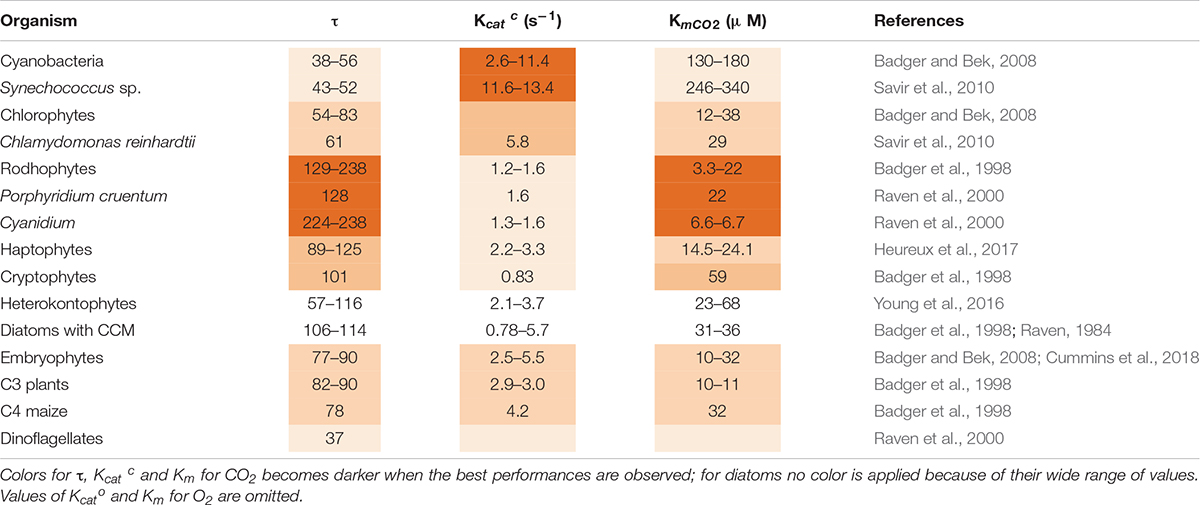
Table 2. Forms and catalytic properties of Rubisco in different taxa: functional diversities and specific values are highlighted.
No Rubisco with zero oxygenation activity has evolved in Nature, possibly due to intrinsic fragility of the active site. Nevertheless, microalgae achieved a strong reduction in Rubisco oxygenation by evolving the so-called CO2 concentrating mechanisms (CCMs) to actively pump CO2 at the Rubisco active site, in an energy-dependent manner (Giordano et al., 2005). Conversely to the so-called “biochemical” CCM, like C4 or CAM metabolism in plants, in algae HCO3– is not first incorporated into organic intermediates and then released as CO2 nearby Rubisco (Raven, 1997a, 2010; Giordano et al., 2005). Taking advantage of the different forms of Ci dissolved in water, microalgal “biophysical” CCM uses CO2 channels and HCO3– membrane transporters to accumulate Ci, carbonic anhydrases (CAs) to allow the rapid conversion of HCO3– to CO2 or vice versa following the equilibrium and acidic compartments (primarily the thylakoid lumen) to favor HCO3– conversion to CO2 next to the Rubisco. The additional occurrence of Rubisco-containing microcompartments, the carboxysomes in cyanobacteria and the pyrenoids in eukaryotic algae, further facilitates the constitution of high CO2 concentrations and, at the same time, limits CO2 leakage through the outward diffusion.
Although widespread in microalgae, CCMs show species-specific features, which result in different energy requirement to effectively concentrate CO2 nearby Rubisco. More efficient biophysical CCMs have been observed in species where Rubisco selectivity is lower, like cyanobacteria, but also green algae (Giordano et al., 2005) and some diatoms (Hopkinson et al., 2011, 2016; Young et al., 2016). In the latter, CCMs are indeed quite diverse among species and unique, suggesting a co-evolution between Rubisco properties and CO2 concentrating strategies (Tachibana et al., 2011; Matsuda et al., 2017; Young and Hopkinson, 2017). So far, CCMs with lower energy cost (calculated as mol photons absorbed per mol of Ci converted into one mol C in carbohydrate, assuming no leakage of CO2 in CCMs) take advantage of HCO3– entry in the thylakoid lumen driven by the proton gradient generated during photosynthesis (Raven et al., 2014). Luminal CAs, like Cah3 found in the green alga Chlamydomonas reinhardtii (Karlsson et al., 1998) and Θ-CA in the diatom Phaeodactylum tricornutum (Kroth et al., 2008; Kikutani et al., 2016; Matsuda et al., 2017), then speed up the equilibration of HCO3– and CO2, causing the CO2 diffusion out of the thylakoid into the surrounding pyrenoid, where Rubisco is localized (Raven, 1997b).
In addition to the reaction of oxygenation, the very low value of Rubisco catalytic rate (Kcatc) (2–4 C s–1) is likewise a major barrier to enhance C assimilation, except in cyanobacteria whose Kcatc is around 12 C s–1 (Table 2). A higher Kcatc would reduce the amount of Rubisco required by the cell to sustain a certain growth rate, improving nitrogen (N) use efficiency of Rubisco itself and of the additional proteins involved in its assembly and activation (Raven et al., 2014). In fact, when low temperature slows down enzymatic reactions and also Rubisco’s Kcatc is decreased, algae respond by increasing Rubisco abundance per cell (Young et al., 2015). Since accumulating Rubisco is costly, algae may alternatively adopt the strategy of increasing the abundance of CCM components instead of the Rubisco enzyme at low temperature (Andersson, 2008).
Metabolic Fluxes of C
The 3-PGA produced by Rubisco can be allocated as such to other metabolic pathways like fatty acid synthesis, but it is typically reduced to the triose-phosphate glyceraldehyde-3-phosphate (GAP) in the reduction phase of CBB cycle and, hence, used by the cell (Figure 1). Some GAP molecules are further processed in the CBB cycle to regenerate the starting substrate RuBP. Other molecules are the net production of C fixation and are directed to the synthesis of monosaccharides and storage carbohydrates, which are then used in cell growth, respiration and synthesis of the other cell organic compounds (Figure 1).
Storage carbohydrates differ among species. Diatoms, under nutrient-replete conditions, store sugars in vacuoles as the β-1,3-glucan polymer (chrysolaminarin) during the day, catabolizing it in the dark (Granum et al., 2002; Caballero et al., 2016). Carbohydrates are also involved in extracellular polymeric substances production (Granum et al., 2002). In green algae (like in plants) carbohydrates accumulate in the form of starch crystalline granules within the plastid, whereas red algal starch is cytosolic and is known as floridean starch (Patron and Keeling, 2005). Cyanobacteria, as most bacteria, accumulate glycogen or poly3-hydroxybutrate (PHB) or other polyhydroxyalkanoates (PHAs) as energy stores (Murphy and Vance, 1999). PHAs are being considered promising candidates for sustainable polymer production as an alternative to conventional plastics (Luengo et al., 2003); PHAs-derived bioplastics would be completely and quickly bio-degraded by a variety of microorganisms into CO2 and water (Table 1).
Cyanobacteria can allocate C also to neutral lipids, which are accumulated as small droplets (30-300 nm) close to the cell or thylakoid membrane (Peramuna and Summers, 2014). Many eukaryotes, instead, form lipid bodies of triacylglycerides (TAGs) that can range from 0.1 to 50 μm in size (Murphy and Vance, 1999; Figure 3). The accumulation of large lipid bodies is favored by spatial constraints: lipids accumulate easier than other C reservoirs when space is limiting due to a lower hydration (Palmucci et al., 2011). TAG synthesis starts in plastids from the carboxylation of acetyl-CoA by acetyl-CoA carboxylase (ACCase) to produce malonyl-CoA (Martins et al., 2013; Wichmann et al., 2020). As described in Figure 3, acyl chains are synthesized by various enzymes localized in different subcellular compartments. The final steps of acyl elongation, desaturation and insertion in TAGs occur in the endoplasmic reticulum (Figure 3B). Microalgae, particularly genera as Schizochrytium, Crypthecodium, Nannochloropsis and Phaeodactylum (Enzing et al., 2014), are well known to synthesize long and very long chain polyunsaturated fatty acids (LC-PUFA). LC-PUFAs consist of 20-22 C and have a high nutraceutical value as they include, for example, Eicosapentanoic acid (EPA or 20:5n-3) and Docosahexaenoic acid (DHA or 22:6n-3) (Table 1 and Figure 3). In some microalgae, as Botryococcus braunii, free fatty acids are substrates for the synthesis of alkanes (Wichmann et al., 2020). Further, in eukaryotic algae, the cytosolic mevalonate (MVA) pathway and the plastidic 2-C-methyl-d-erythritol 4-phosphate (MEP) pathway generate isopentenyl pyrophosphate (IPP) and dimethylallyl pyrophosphate (DMAPP), metabolic precursors of terpenoids (Figure 3; Wichmann et al., 2020).
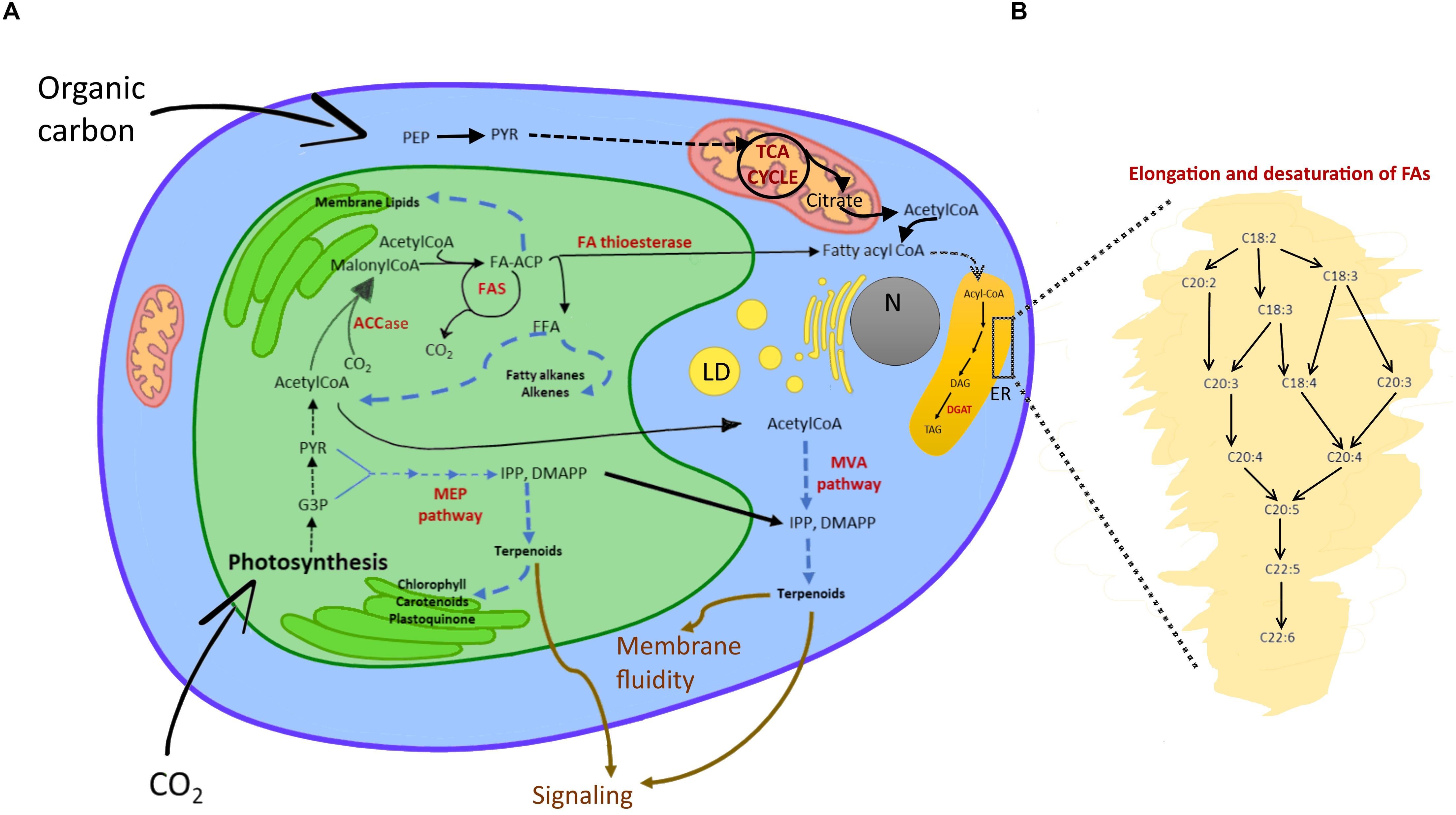
Figure 3. Lipid metabolism in eukaryotic algae. (A) Schematic view of fatty acid and isoprenoid biosynthesis. In the chloroplast, Acetyl-CoA from the triose phosphates pool, or imported as acetate from cytosol and mitochondria to the plastid, is carboxylated by ACCase to malonyl-CoA (Martins et al., 2013; Wichmann et al., 2020). The malonyl group is transferred to an ACP. Malonyl-ACP undergoes a series of condensation reactions with acetyl-CoA, catalyzed by a β-ketoacyl-ACP synthase (KAS). Thanks to the FAS multi-subunit complex, saturated C16:0- and C18:0-ACP are synthetized. The acyl groups are then either retained in the plastids to be incorporated into membrane lipids or they are hydrolyzed from ACP by specific fatty acyl-ACP thioesterases that release FFAs. C16:0- and C18:0-ACPs can be desaturated into C18:1 before being hydrolyzed. FFA are exported to the cytosol and esterified to CoA by a long-chain acyl-CoA synthase located in the outer envelope of the plastid to form acyl-CoAs (Martins et al., 2013). By analogy with higher plants, it is predicted that these extraplastidic acyl-CoA esters are then transferred to the endoplasmic reticulum or acylated onto a phospholipid backbone before further participation in the synthesis of membrane lipids or storage TAGs (see Sayanova et al., 2017 for details). Enzymatic reactions are indicated by solid arrows, series of reactions by dashed arrows, pathway names in bold black, intermediates in black, main enzymes in red, functions of products in brown. Abbreviations: LD, lipid droplet; N, nucleus; ER, endoplasmic reticulum; MEP, 2-C-methyl-D-erythriol 4-phosphate; MAV, mevalonate, ACCase, acetyl-CoA carboxylase; ACP, acyl carrier protein; FAS, fatty acid synthase; DGAT, diacylglycerol acyltransferase; DAG, diacylglycerol; FFA, free fatty acid; G3P, glyceraldehyde-3-phosphate; PYR, pyruvate; TAG, triacylglycerol. (B) Enlarged ER segment detailing elongation and desaturation of algal fatty acids.
The removal of C-intermediates from the Krebs cycle in the mitochondria, as oxaloacetate and 2-oxoglutarate (Figure 1), allows to synthesize various molecules like aspartate and glutamate, which are then substrates for the synthesis of related amino acids and pyrimidines. Anaplerotic reactions replenish the Krebs cycle (Figure 1): PEP carboxylase, PYR carboxylase and PEPC carboxykinase β-carboxylate 3 C compounds, either phosphoenolpyruvate (PEP) or pyruvate (PYR), using CO2 or HCO3–. About 5% of the fixed C in algae is fixed via anaplerotic fixation (Raven and Farquhar, 1990).
Nutrient Requirement and Allocation Into Macromolecules
The average macronutrients elemental stoichiometry of microalgae, under resource-replete conditions and optimal environmental parameters (usually adopted during the first phase of biomass production in industrial cultivation plants), is C124N16S1.3P1 (Ho et al., 2003; Quigg et al., 2010; Giordano, 2013). Such stoichiometry is quite conserved among microalgal species, whereas the stoichiometry of micronutrients like Fe, Zn, Mn, Cu, Mo shows higher variability.
The actual elemental stoichiometry depends on both genotype and environmental conditions, like nutrient availability, light, temperature, salinity. E.g., species belonging to the red algae lineage, like diatoms, display a higher S cell quota (and thus a lower C:S ratio) than species from the green lineage (Norici et al., 2005). The assimilation of N in the form of NO3–, due to the N oxidation number of + 5, requires more energy than the assimilation of N as NH4+ (N oxidation number -3); thus, the available chemical source of N constrains C:N ratio and growth when energy is limiting (Norici et al., 2002; Ruan et al., 2017; Ruan and Giordano, 2017).
C, N and S are all assimilated through energy-demanding reductive pathways into macromolecular pools. C, the most abundant element in algal cells (36–65% of dry matter), is allocated into proteins, carbohydrates and lipids. Proteins are also the primary functional reservoir of cellular N (Figure 1). Remarkably, 15-25% of total cellular N is allocated to proteins involved in the light reactions of photosynthesis and 5-10% to the Rubisco protein, depending on the growth light (Li et al., 2015). Essential amino acids (cysteine and methionine) and glutathione are sinks of cellular S (Giordano and Raven, 2014). Phospholipids and nucleic acids are the major functional reservoirs of cellular P. In some algae, polyphosphates are additional P stores resulting from luxury uptake, a process which may divert the elemental composition from the cell essential requirement when energy and resources are available in excess (Giordano and Ratti, 2013).
Under replete nutrient supply, microalgae contain the following macromolecular composition (expressed as percentage of dry weight): from 27 to 43% proteins, from 12 to 21% lipids, from 12 to 23% carbohydrates, 8–27% ash, 5–6% nucleic acids, about 1% chl a (Finkel et al., 2016). Carotenoid content ranges between 0.1–0.2% of dry weight; however, β-carotene can increase up to 14% of dry weight in Dunaliella (Spolaore et al., 2006; Becker, 2007). Vitamins B1, B2, B3, B6, B12, E, K, and D are also present in traces (Becker, 2007; Kamalanathan and Quigg, 2019; Bacchetti et al., 2020).
Similarly to elemental stoichiometry, also macromolecular composition varies according to phylogenetic groups (Finkel et al., 2016; Figure 4A) and environment. It reflects fundamental cellular properties in terms of structural and functional organization, like primary storage pools (some species are well-recognized as oleaginous for their strategy to accumulate lipids instead of carbohydrates), light harvesting apparatus, cell wall. Notably, each macromolecular pool is characterized by a different cost in terms of chemical energy (ATP equivalents). Allocating C to lipids has a major cost compared to the one of carbohydrate synthesis (Montechiaro and Giordano, 2010; Palmucci et al., 2011). Protein pool is costly as it requires the assimilation of different elements, C, N and S, into amino acids (Giordano and Raven, 2014). Energy investment in the three macromolecular pools has been calculated in Figure 4B according to their abundance. The “cheapest” biomass appears to be that of diatoms. Compared to the other groups, diatoms are characterized by a higher ash content and a lower amount of proteins and carbohydrates (Figure 4A; Finkel et al., 2016). Consistently, unlike other algae (e.g., green algae), diatoms do not possess cellulosic cell wall, but they harbor a silica shell named frustule which makes Si a macronutrient for this algal group. Further, they display a different C allocation and regulation of the C metabolism, resulting in enriched lipid fraction (Wagner et al., 2017).
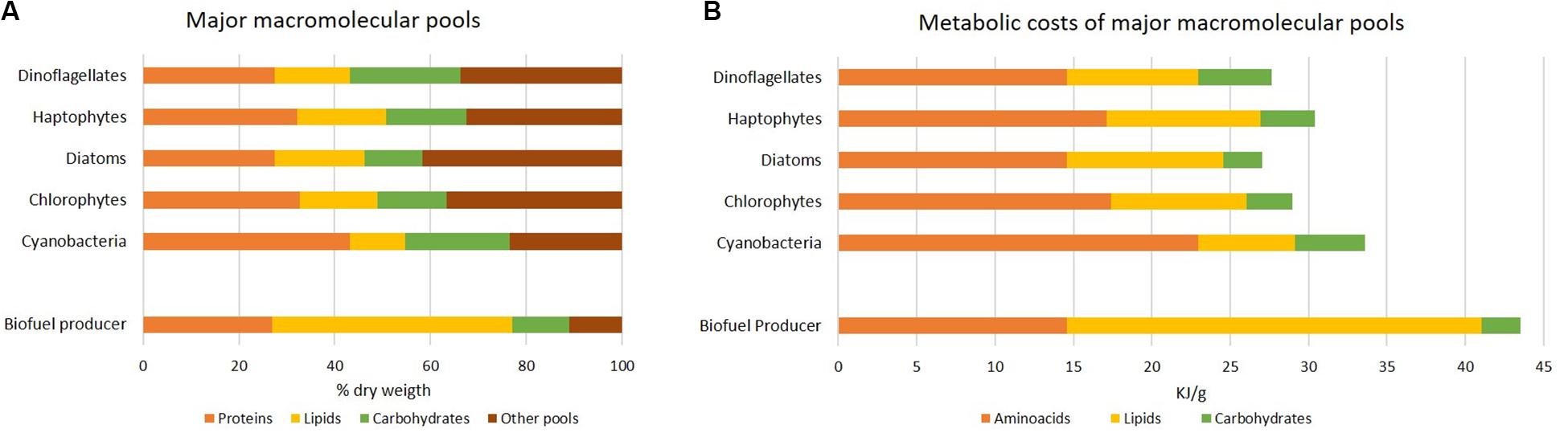
Figure 4. Metabolic cost of macromolecular pools in different group of microalgae. (A) Average macromolecular composition in different algal phyla, as percentage of dry weight under nutrient-sufficient exponential growth; all data except for the “biofuel producer” are according to Finkel et al. (2016). The biofuel producer is suggested as a cell containing the lowest protein and carbohydrate percentage and a lipid percentage suitable for biofuel production in exponentially growing algae. (B) Estimate of the energy investment (kJ g–1) by algal phyla according to data reported in (A). The hydrolysis of 1 ATP was assumed to yield 55 kJ mol–1 and the transfer of 2e– was assumed to correspond to 4 ATP equivalents (Giordano and Raven, 2014). Budgets of ATP equivalents per mole are 11, 15, 41 and 33 corresponding to the assimilation of CO2 into carbohydrates, CO2 into lipids, nitrate into glutamate and sulfate into cysteine (the latter two as proxy for proteins), respectively (Palmucci et al., 2011; Giordano and Raven, 2014); molecular weight of macromolecules used in calculations are according to Geider and La Roche (2002) and Sterner and Elser (2002). Note that we are presenting only the costs of amino acids, lipids and carbohydrates pools.
High lipid content is a desirable trait for industrial purposes as neutral lipids (TAGs) can be transesterified to fatty acid methyl esters to obtain biodiesel. In oleaginous species, the lipid content can increase up to 77% of dry weight by altering the available C:N ratio in growth media (Chisti, 2007; Li et al., 2008; Sun et al., 2019); in fact, C is largely allocated into lipid stores when N is depleted and protein synthesis is stopped. However, during severe elemental imbalance and lipid accumulation, cell growth is hampered, representing a major issue in developing economically viable biofuel producers.
An ideal biofuel producer would thus allocate a major C quota to lipids already during exponential growth phase, without nutritional stress, and show remarkable rates of biomass production. As proposed in Figure 4, an ideal biofuel producer would harbor protein and carbohydrate contents as low as in the diatom example, but a lipid content as high as 50% of dry weight. The energy cost associated to the intended composition is 1.3-1.6 times higher than what required for the average composition of existing algae (Figure 4). In line with a higher biomass cost, many known oleaginous species, like B. braunii, are characterized by a poor growth rate; when a major energy quota is allocated to lipid storage, energy is likely diverted from the cellular quota reserved for growth (Giordano and Wang, 2018).
Energetic constraints to biomass composition become relevant when energy availability limits growth (Wagner et al., 2006; Jakob et al., 2007; Nogueira et al., 2015; Ruan and Giordano, 2017; Ruan et al., 2017; Alboresi et al., 2016). This is often the case in dense commercial cultures, where light is limiting. A domesticated biofuel producer strain shall therefore present an improved energy utilization through genetic modifications.
Open Challenges Toward Enhanced Co2 Fixation in Microalgae: Building Living Factories
The impact of above described biological constraints on microalgal composition and growth has been weighed in Table 3, which focuses on biofuel production. Input data are representative of species, cultivation systems and climatic conditions showing different lipid content and biomass productivity, then used to calculate the biodiesel productivity. The most productive species/plant still requires roughly half of the Italian area (corresponding to the entire Italian arable land) to fulfill 9% of the energy demand by 2030 in the transport sector, the estimated goal for biofuel quota according to the Sustainable Development Scenario (International Energy Agency, 2020b). If vast areas are required, climatic parameters also come into play when assessing the potential final yield, as exemplified by T. suecica cultivation plants in Tuscany or Tunisia, showing almost 40% less biomass yield in the former than in the latter (Table 3).
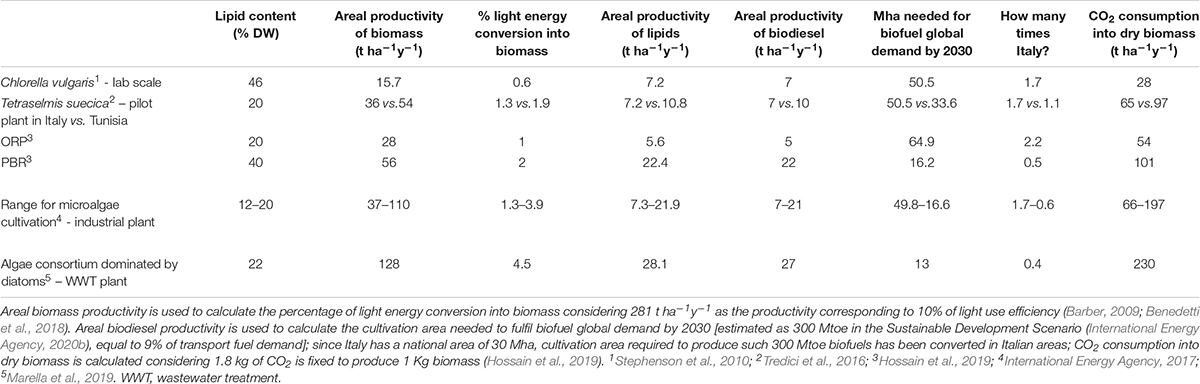
Table 3. Lipid content, areal biomass and lipid productivity, projected biodiesel productivity (assuming 96% recovery of lipids through direct transesterification; Lepage and Roy, 1984) for microalgae cultured in different climatic conditions, cultivation systems, laboratory or pilot or industrial scale.
It follows that current assessment will not allow a fast transition to clean sustainable energy. Yet, theoretical estimates of maximum light conversion into biomass production (10-12%) (Zhu et al., 2008; Barber, 2009; Melis, 2009; Blankenship et al., 2011; Peers, 2014) are still quite distant from the actual values (Table 3).
A major goal for the scientific community is thus to significantly increase the efficiency of light energy conversion into biomass, allowing the use of photosynthetic cells as cost-effective factories. Multiple challenges need to be overcome: (i) deepening our knowledge of the physiological constraints cells experience as cultivation systems get larger; (ii) combining production of exploitable biomass with waste valorization, which results in important cost reduction; (iii) improving the light use efficiency and rewiring cell metabolism to the desired product.
Screening of natural variants, optimization of culturing conditions and new ad hoc solutions by means of synthetic biology approaches are pivotal to overcome these issues and to reach significant impacts in terms of renewable biomass feedstock for energy conversion and captured CO2 emissions.
Strain Selection, Microbial Consortia and Optimization of Nutrient Resources
To date, research in the applied field of algal research has more often focused on a limited number of so-called model species, as Synechocycstis and Synechococcous for cyanobacteria, C. reinhardtii for green algae, P. tricornutum for diatoms, since their genomes are sequenced and several molecular tools are available or rapidly developing. At the same time, algae-based industry has focused on a few robust algal strains, like Arthrospira platensis and Dunaliella salina, primarily selected for “health-foods” and antioxidants production (see Table 1). Further, their ability to thrive at extreme pH (A. platensis) and salinity (D. salina) avoids easy contamination by wild algal strains, grazers, and pathogens even if cultured in open ponds. It has been estimated that 10 to 30% of annual production in open ponds is lost due to pond contamination (Richardson et al., 2014). Closed cultivation systems as PBRs, on the other hand, guarantee greater crop protection and allow a better control of the growth environment (Table 4); it is therefore easier to reach higher biomass yield and to direct algal C allocation to target compounds with respect to cultivation in open ponds (Tables 3, 4). However, PBRs pose other challenges (Table 4). They require higher energy input. Photosynthetic build-up of O2 is another disadvantage of PBRs. If no O2 degassing system is present, dissolved O2 can reach 250% of saturation, much higher than expected at air equilibrium. This leads to inhibition of Rubisco, to ROS formation, and it hinders microalgae growth (Raso et al., 2012; Bilanovic et al., 2016).
Suitable microalgae for industrial production should thus own high growth rates, natural ability to tolerate extreme conditions required by scale-up or specific industrial application, high productivity for native or heterologous products (Picardo et al., 2013; Giordano et al., 2015b).
Besides monospecific cultures, more and more evidences are pointing toward the advantages of using consortia of algal species or of algae and bacteria (Newby et al., 2016 and references there in). Polycultures enclosing both photosynthetic and heterotrophic species provide a more stable crop, protected from grazing and infection losses (Corcoran and Boeing, 2012), and a greater potential for overyielding and C fixation (Shurin et al., 2014; Newby et al., 2016). It is noteworthy that, in Table 3, the diatom-dominated consortium reached the highest areal biomass productivity as compared to the other monoculture systems. This most likely relies on the functional complementary of species, as for nutrient and light utilization. Biomass quality may also improve in the polyculture relative to the one of most productive monocultures: e.g., higher lipid production of highly diverse algal communities compared to that of the respective monocultures under similar growth conditions (Stockenreiter et al., 2012).
One of the basic principles of microbial ecology is that species diversity promotes ecosystem productivity and stability (Cardinale et al., 2011). This principle applies also to traditional wastewater treatment plants, which are robust and large open systems that rely on many species of different microorganisms naturally assembled to remove nutrients from wastewaters (Brenner et al., 2008). When selecting species for polyculture, thus, functional richness is the primary goal (Newby et al., 2016). For example, in N limited growth media, N2 can be biologically fixed by diazotrophic cyanobacteria, allowing N to become available to symbiotic algae (Stockenreiter and Litchman, 2019). The described strategy based on functional complementarity of the mixed strains reduces nutrient demand and associated costs in a large-scale cultivation plant (Stockenreiter and Litchman, 2019). Alternatively, the chief commercial method to produce N fertilizers by fixing atmospheric nitrogen into ammonia, is the highly energy demanding Haber–Bosch process (Travis, 1993). Non-photosynthetic bacteria may contribute to functional consortia also providing vitamins, phytohormones like indole-3-acetic acid, siderophores involved in Fe solubility (for detailed literature see in Newby et al., 2016).
The valorization of wastewaters as sustainable source of nutrients (P,N, trace metals) for algal cultivation, while achieving water treatment demand, is a promising path since it can strongly reduce biomass production cost and environmental impact; the entire production chain can meet sustainable and circular bioeconomy criteria. Growth on wastewaters imposes mixotrophy and heterotrophy nutrition for algae. Beside autotrophic nutrition in the presence of inorganic C sources and light, microalgae can act as heterotrophs and use either soluble organic carbon (such as glucose, acetate, and glycerol; osmotrophy) or particulate organic carbon (phagotrophy) (Selosse et al., 2017) as energy and carbon source in the dark, possibly resulting in high biomass density. Mixotrophic cultivation of microalgae is a combination of photoautotrophy and heterotrophy to use both inorganic and organic carbon substrates in the presence of light. Mixotrophic nutritional regime cannot be applied indistinctively to all microalgae. Mixotrophy is more frequent in eukaryotic algae which originated themselves from events of phagotrophy, as secondary and tertiary endosymbiotic events (Beardall and Raven, 2016); similarly, it has been shown that the presence of specific membrane transporters and enzymes of carbohydrate metabolism are usually involved in the ability to grow in soluble organic matter rich media (Barbier et al., 2005). Thus, mixotrophy enhances the biomass productivity only in specific genotypes. For example, glycerol as C source and NH4+ as N source enhanced growth of P. tricornutum and increased the productivity of highly valuable omega-3 LC-PUFAs, such as EPA, by a factor of 10 when compared with photoautotrophic control conditions (Ceron et al., 2000; Villanova et al., 2017). In Galdiera sp., higher biomass and PUFA production, despite a lower concentration of lipids, were observed under mixotrophic and heterotrophic conditions with respect to autotrophic conditions; also, the lipid profile was affected by nutritional regime (López et al., 2019).
In parallel with the use of wastewaters for algal cultivation, the use of flue gases has been studied to mitigate greenhouse gas emission and temporarily sequester CO2 into biomass. Flue gases may contain pCO2 values from 3 to 25% of its volume (Packer, 2009); when directly bubbled in algal cultures, flue gases cause growth medium acidification, which can be reduced by adding a pH buffer. Not all algae are suited for CO2 bioremediation since they cannot equally tolerate high CO2 concentrations: for example, D. salina is CO2-sensitive and inhibited at 10% CO2 while Chlorococcum littorale is extremely CO2-tolerant and grows rapidly at CO2 concentrations up to 60% (Kodama et al., 1993; Sergeenko et al., 2000; Muradyan et al., 2004). High CO2 tolerance requires the ability to maintain intracellular pH homeostasis by down regulating CAs and the CCMs (Borowitzka, 2016). It is worth mentioning acidophilic and acidotolerant microalgae which lack CCMs (Diaz and Maberly, 2009) have also been tested for cultivation in plants fed on flue gases (Eibl et al., 2014; Nagappan et al., 2020; De Farias-Neves et al., 2019).
Noteworthy, the ability to cope with up to 20% or more CO2 may not imply a higher growth rate. Since a higher growth rate is consequent to an increased nutrient use efficiency, bubbling cultures with CO2 stimulates growth if C is the limiting nutrient, as it might be the case in CCM lacking species (Beardall and Giordano, 2002; Raven et al., 2011, 2012; Venuleo et al., 2018), or if a decreased cost for CCM allows a higher energy investment into making new cells (Raven et al., 2014; Li et al., 2015). Moreover, when CO2 is bubbled into the culture, it may elicit a nutritional (C/N) imbalance leading to a change in biomass quality (Beardall and Giordano, 2002; Giordano and Ratti, 2013; Palmucci et al., 2011; Raven et al., 2011, 2012).
Designing Ad hoc Bio-Factories for Fuels and High-Value Chemicals
The above described strain selection and optimization of culturing condition are pivotal to make the best possible use of available energy and resources according to target products. However, as suggested by the biofuel yields exemplified in Table 3, it is unlikely that such approach alone will achieve significant stocks and allow a major breakthrough in most applications. Genetic manipulation shall thus provide additional ad hoc solutions to optimize energy harvesting and utilization, and to rewire cell metabolism toward specific products (Figure 5).
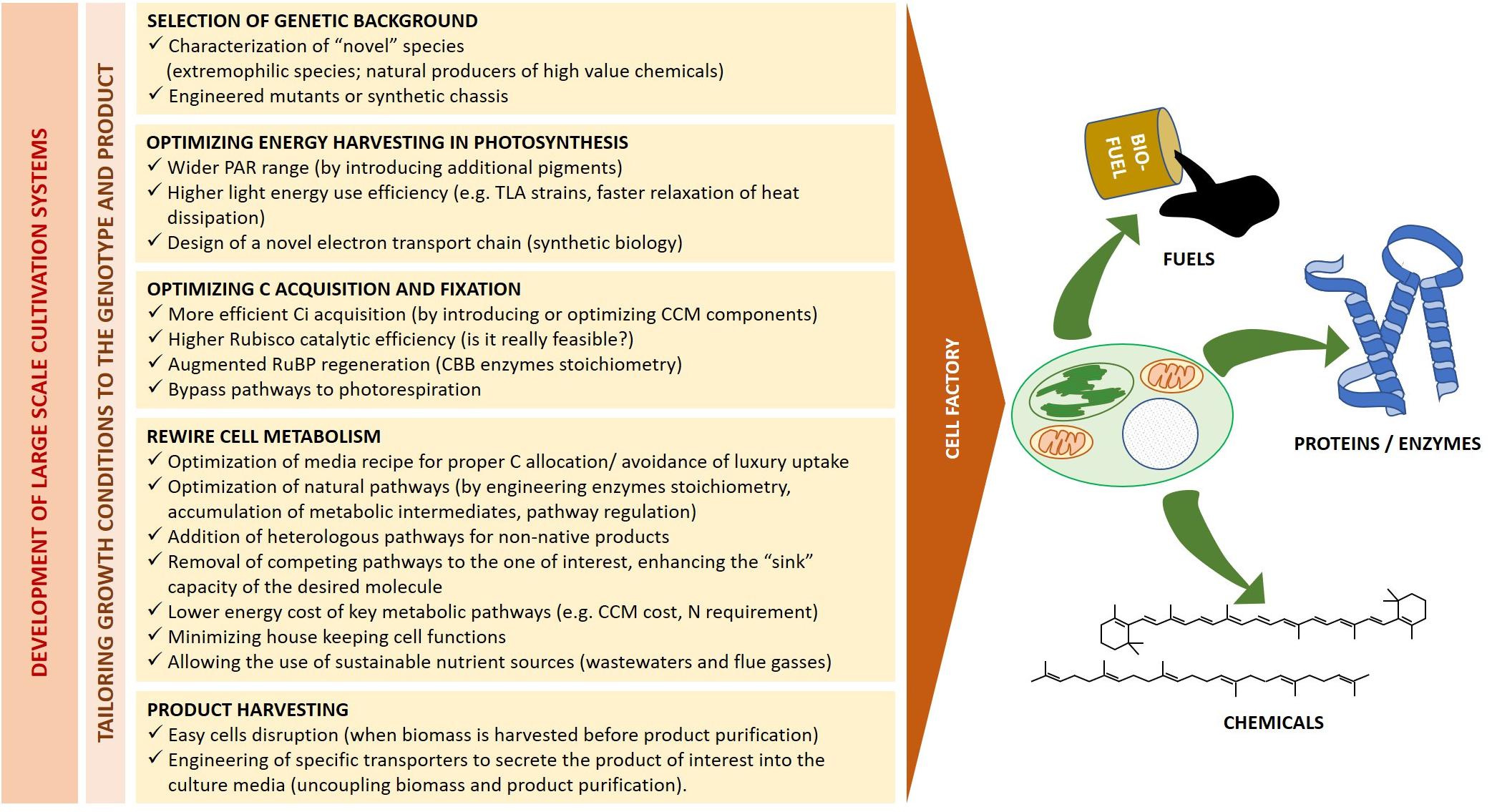
Figure 5. Microalgae as cell factories. Heading to a sustainable industrial production, the bioreactor design and growth conditions must be tailored to the selected genetic background and final product of interest. The major biological traits which require simultaneous optimization for fuel and high-value chemicals production from living factories are outlined.
Synthetic biology is a recent discipline which deals with the application of engineering principles to biology. It aims both at simplifying complex systems and at constructing novel ones by combining individual parts from multiple biological sources, introducing functions not previously held by the host (Kliebenstein, 2014; Liu and Steward, 2015; Shih, 2018; Luan and Lu, 2018). One of the most powerful application of plant synthetic biology is thus to design “domesticated” photosynthetic cells for a continuous production of biofuels and high-value compounds, starting from sunlight and inorganic or recycled nutrients. To this goal, microalgae metabolic engineering shall be applied at multiple levels, from enhancing the catalytic efficiency of native enzymes to introducing novel functions, like product secretion, which will ease the harvesting of commercial product (Figure 5).
However, in order to extensively apply this approach to photosynthetic organisms, several tools still need to be fully developed to manipulate microalgal cells in a predictable way.
Molecular Biology Tools: State-of-the-Art and Open Challenges
Both prokaryotic and eukaryotic microalgae are suitable candidates as producing platforms: their different features are critical for accumulation of different kinds of product. Cyanobacteria naturally accumulate bioplastic precursors (Table 1; Luengo et al., 2003) and are good hosts for small molecules not requiring eukaryotic post-translational modifications. Conversely, eukaryotic algae are valuable when such modifications (e.g., glycosylation) are essential, or when product compartmentalization is necessary. Eukaryotic algae are also a precious source of several secondary metabolites (Wijffels et al., 2013), and include some well-known oleaginous species, as P. tricornutum or N. gaditana.
The ability to change, remove or introduce gene(s) of interest in a programmable way, and to achieve a stable phenotype of the engineered strains over time, is pivotal to industrial application of photosynthetic bio-factories (Patel et al., 2019; Pérez et al., 2019). Molecular tools are better established for cyanobacteria than for eukaryotic algae, but nuclease-based genome editing tools like Clustered Regulatory Interspaced Short Palindromic Repeats (CRISPR)/Cas9 and Transcription Activator-Like Effector Nucleases (TALEN) are rapidly developing and applied to algal species of different phylogeny and commercial value (Doron et al., 2016; Nymark et al., 2016; Shin et al., 2016; Kroth et al., 2018; Verruto et al., 2018; Ortega-Escalante et al., 2019; Pérez et al., 2019; Ng et al., 2020). Yet, genetic manipulation of microalgae, particularly eukaryotic ones, still encounters several challenges. They are mostly related to the low efficiency of the transformation protocols and to the low and unstable expression levels of transgenes. Such weaknesses have been mainly ascribed to positional effects of gene integration and/or gene silencing. Secondary off-target mutations in edited strains, even when targeted genome editing has been performed with nucleases (Patel et al., 2019), are an undesirable aspect of algae transformation. To minimize issues due to off-target mutations, ribonucleoproteins (RNP-) based methods for CRISPR/Cas gene editing have been developed in substitution to vector-mediated protocols (Patel et al., 2019).
The selection of genetic backgrounds which minimizes gene silencing is a tool to achieve a stable expression of transgenes. Mutants of the model green alga C. reinhardtii that showed increased nuclear transgene expression, called UVM4 and UVM11, have been obtained with random UV mutagenesis (Neupert et al., 2009). Knowledge on molecular mechanisms underlying gene expression regulation shall be deepened to fully overcome drawbacks due to low heterologous gene expression, avoiding at the same time labor intensive screening of random mutants. The optimization of cis regulatory elements like promoters, ribosome binding sites (RBS), UTR sequences, and the optimization of heterologous sequence codon usage according to the selected cell chassis, have also been recognized as a key feature for successful transgene expression (Rasala et al., 2011; Jinkerson and Jonikas, 2015; Till et al., 2020); specifically in eukaryotic cells, mimicking a native nuclear gene sequence as accurately as possible, by interrupting the transgene coding sequence with introns, shall also contribute to a stable transgene expression (Baier et al., 2018). Finally, efforts have been made to identify and characterize the best genomic environment where to introduce transgenes, the so-called neutral sites, loci in which gene integration is not compromising other cell functions (Ng et al., 2015; Pinto et al., 2015; Kroth et al., 2018).
Once productive genome edited microalgae are obtained and ready to be exploited in large scale industrial plants, the biosafety issue must be timely addressed by specific agencies (Spicer and Molnar, 2018): both potential harm to human and animal health and potential risk for the environment (i.e., vertical or horizontal gene transfer, strain competition with wild-type strain) are to be assessed (Henley et al., 2013). Although genetically modified algae are predicted not to have fitness advantages in nature, safety measures to avoid the spill over of modified strains must be regulated and applied especially in outdoor cultivation systems (Wijffels et al., 2013; Henley et al., 2013; Spicer and Molnar, 2018).
Cellular Targets to Enhance Bio-Factories Productivity
All biological processes described in section 2, from light harvesting to metabolic energy consumption, are characterized by substantial energy losses (Figure 2 and Table 5) and are potential targets for improving energy use efficiency.
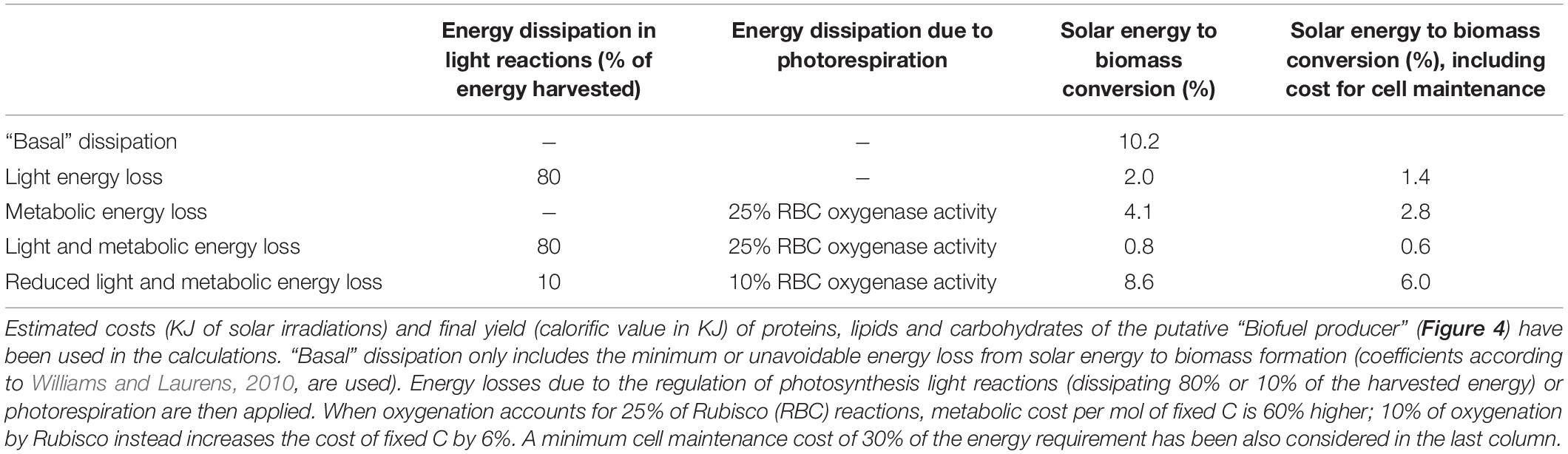
Table 5. Examples of efficiency of solar energy conversion into biomass, in the absence or presence of different sources of energy dissipation.
Adjusting the efficiency of photosynthetic light reactions will rise the amount of energy available for cell metabolism. A key strategy to improve light conversion into chemical energy deals with modulation of light harvesting and photoprotection. They are both very dynamic processes which readily respond to changes in light intensity, and account for substantial energy dissipation when light irradiation exceeds the photosynthesis saturation point (Figure 2 and Table 5; Peltier et al., 2010; Wilhelm and Selmar, 2011; Peers, 2014).
The reduction of light harvesting capacity is a valuable strategy, which has already been tested in strains with genetically Truncated Light harvesting Antennae (TLA). TLA approach reduces the light harvesting cross-section in photosynthetic cells and potentially triplicates the productivity of plants and algae (Melis, 2009; Kirst et al., 2017). In a dense microalgal culture, TLA phenotype diminishes the energy harvested by the external layer of cells which are directly exposed to intense illumination. Therefore, their need for energy dissipation and risk of photoinhibition are reduced, while their photosynthetic efficiency enhanced. TLA phenotype also favor the diffusion and homogeneity of light into the culture. This increases light availability for the inner layer of cells that instead, in the case of WT cultures, suffer light limitation and reduced growth. TLA benefits were demonstrated in different species, where TLA cultures showed up to 1.5 times the growth and productivity of WT (Nakajima et al., 2001; Polle et al., 2003; Mussgnug et al., 2007; Perrine et al., 2012; Kirst et al., 2014; Shin et al., 2017). However, TLA advantages have been proved under specific growth conditions (i.e., high light) which accentuate TLA positive traits. Conversely, when limiting light was supplied, TLA growth was reduced compared to the WT one (Nakajima et al., 2001; Perrine et al., 2012; Shin et al., 2017). In addition, some TLA mutants showed null advantages in their productivity, irrespective of the condition tested (Page et al., 2012; Nymark et al., 2019). Diverging phenotypes among TLA mutants have been ascribed to undesirable side effects of the mutation or to an unbalanced photoprotection caused by the altered antenna system (de Mooij et al., 2015; Nymark et al., 2019). In TLA Synechocystis cells, the reduced size of phycobilisomes was also shown to have a wide impact on cell proteome (Liberton et al., 2017). Remarkably, despite TLA approach still faces challenges to be solved in future studies, TLA approach has a further central advantage on resource use efficiency: as it reduces cellular N quota invested in light harvesting proteins, more energy can be allocated to other metabolic pathways (Figures 4, 5).
A further approach to optimize light harvesting capacity deals with modulation of photoprotection. Photoprotection results from an intricate network of processes regulating photosynthetic electron transport. It is essential to cope with variable and excess light: the light reactions promptly respond to any burst of higher illumination, increasing the amount of energy captured by the antenna system and electron transport. Conversely, the metabolic reactions are not equally fast to respond to increased energy availability. This creates a dangerous imbalance between production and utilization of ATP and reductants, leading to over-reduction of electron transport chain and photoinhibition. Photoprotection mechanisms thus provide the cell safe valves to dissipate these bursts of excess energy. If fully abolished, the cells will be prone to reactive oxygen species (ROS) formation, photoinhibition, and eventually cell damage when light is absorbed in excess (Wilhelm and Selmar, 2011; Niyogi and Truong, 2013; Gerotto et al., 2016). Thus, a valuable option to optimize photoprotection processes targets their relaxation kinetics. It is estimated that the sustained energy quenching upon a switch from high to low light accounts for about 20% reduction in crop yield (Taylor and Long, 2017): their activation/deactivation kinetic in response to light intensity fluctuations is a possible target of improvement. Validation of this hypothesis has been obtained in tobacco, where the simultaneous over-expression of three key proteins regulating heat dissipation of absorbed excess energy (i.e., PSBS; VDE; ZE) resulted in 9% increased C fixation rates and an average of 15% increase plant dry weight with respect to WT. This was due to a faster deactivation of the heat dissipation mechanisms when plants were moved from strong to limiting light (Kromdijk et al., 2016). A similar approach can be transferred to algae expecting analogous potential benefits. For example, in PBRs, culture mixing leads microalgae to experience a fluctuating light regime: cells are alternatively exposed to strong illumination in the external layer or to limiting light in the internal layer. Thus, the activation/deactivation timescale of photoprotection mechanisms shall fit with the PBR mixing kinetic, in order to protect cells from bursts of high light when cells are in the external layer, while relaxing fast when cells are back to the inner section of the PBR (Sforza et al., 2012; Simionato et al., 2013).
Conversely to light harvesting system and photoprotection processes, photosystem RCs are highly conserved among oxygenic photosynthetic organisms and they are already extremely efficient machines (0.8 and 1 electron/photon for PSII and PSI, respectively) (Caffarri et al., 2014; Raven et al., 2014; Romero et al., 2017). Thus, instead of modifying PSI and PSII, synthetic biology shall design novel architectures of the electron transport chain, in which protein complexes from multiple natural sources are merged together to improve photosynthetic performance (Ort et al., 2015; Pérez et al., 2019). For example, the introduction of bacterial type reaction centers holding bacteriochlorophyll pigments will allow PAR to be expanded up to 1100 nm. It requires a complete redesign of the electron transport chain, thus relying on extensive application of synthetic biology. The modified oxygenic photosynthesis is suggested to potentially double the efficiency of its electron transport (Ort et al., 2015).
Once light energy is temporary stored as chemical energy, ATP and NADPH are used to fix C. Rubisco enzyme causes a considerable loss in energy conversion due to its competitive oxygenase activity: when 25% of the reactions catalyzed by Rubisco use molecular oxygen as substrates rather than CO2, the energy requirement for mol of C fixed increases by 60% compared to the sole carboxylation activity (Table 5). So far attempts to engineer the enzymatic properties of Rubisco by enhancing carboxylation kinetic while limiting oxygenation reactions have failed, despite a massive number of studies focused on the characterization of Rubisco crystal structures in different cyanobacterial, algal and plant species and a detailed knowledge on the catalytic reaction mechanisms both for carboxylation and oxygenation (Taylor et al., 2001; Andersson, 2008; Tcherkez, 2013, 2016). Thus, if Rubisco has already been “optimized” by the Nature during photosynthetic life evolution (Tcherkez et al., 2006; Savir et al., 2010; Kerfeld, 2015), it will possibly remain a major source of energy loss. What can research still pursue? Following a synthetic biology approach, the “best” available natural option of Rubisco (i.e., showing the highest Kcatc, as in cyanobacteria, or the best τ, as in red algae, Table 2) may be combined with the most energy-saving and efficient CCM set up (as in some green algae and diatoms) in the cell chassis. Another suggested solution to improve Rubisco catalytic efficiency, particularly under fluctuating irradiation, deals with engineering Rubisco activase, a Rubisco regulatory protein which facilitates the release of inhibitors from Rubisco active site in an ATP-dependent manner (Carmo-Silva et al., 2015). Moreover, being Rubisco a relatively big and highly expressed enzyme (Ellis, 1979), the removal of high-N content amino acids such as arginine from its sequence may represent a way to increase N and energy use efficiency (Figure 4).
RuBP regeneration is also recognized as a main bottleneck reducing CBB cycle efficiency (Falkowski and Raven, 2007). A path to improve C fixation thus relies on optimization of CBB enzymes’ stoichiometry to ease the regeneration of RuBP. Mutants harboring modified amounts of Rubisco, sedoheptulose-1,7-bisphosphatase, fructose-1,6-bisphosphatase (the latter two as a bifunctional enzyme in cyanobacteria), fructose 1,6-bisphosphate aldolase or transketolase, have been obtained both in eukaryotic algae and cyanobacteria (Fang et al., 2012; Ogawa et al., 2015; Liang and Lindblad, 2016; Yang et al., 2017; De Porcellinis et al., 2018). As described above for TLA mutants, manipulated abundance of CBB enzymes led to variable results, according to the species and to the specific enzyme under investigation. Yet, these mutants often displayed increased photosynthesis and growth. For example, overexpression of sedoheptulose-1,7-bisphosphatase or sedoheptusole-1,7/fructose-1,6-bisphosphatase increased glycerol accumulation in Dunaliella bardawil and wax esters in Euglena gracilis (Fang et al., 2012; Ogawa et al., 2015). In Synechococcus, the overexpression of the bifunctional enzyme led to a large adjustment of C metabolism, enhancing photosynthetic C fixation while decreasing respiration (De Porcellinis et al., 2018). These findings prove that modifying the expression of specific CBB enzymes shall contribute to an improved C fixation and cell productivity.
Another attracting option to enhance C fixation efficiency relies on engineering or replacing the photorespiratory pathway, in order to metabolize glycolate produced by Rubisco with lower energy cost and resource consumption. A first functional synthetic metabolic bypass to photorespiration has been successfully introduced in Synechococcus elongatus PCC7942. Six heterologous genes of the C fixing 3-hydroxypropionate pathway from Chloroflexus aurantiacus, a thermophilic anoxygenic phototroph, were expressed in S. elongatus (Shih et al., 2014). Next steps on the same path rely on further engineering of cell metabolism to allocate the metabolic intermediates generated by the bypass pathway to the production of industrially relevant molecules (Shih et al., 2014).
More ambitious routes would be to import oxygen-insensitive pathways for the key reaction of C fixation to bypass Rubisco altogether. The possibility to introduce (at least in addition to carboxylation) CO2 reductive pathways into photosynthetic cells has also been considered. Verifying the actual feasibility and potential of these synthetic pathways in vivo is a major task for the future (Bar-Even et al., 2010; Bar-Even, 2018; Cotton et al., 2018).
For some specific purposes, a fully opposite option can be envisioned, which takes advantage of Rubisco oxygenation side reaction instead of trying to limit it. Under specific growth conditions, glycolate is a main sink of fixed C and a very high amount of glycolate is produced and secreted by C. reinhardtii cells. Glycolate can be recovered from the culture media and used as a substrate for other biotechnological applications, as methane production (Taubert et al., 2019; Table 1).
In parallel with maximizing biomass productivity, several research groups worldwide are constructing genetically modified strains with the goal of improving the yield of natural products or introducing the ability to synthesize non-native molecules (examples in Table 6; Case and Atsumi, 2016; Sun et al., 2019; Wichmann et al., 2020). Multiple strategies are under investigation to enhance the accumulation of biofuel feedstocks like alcohols or TAG. They range from overexpression of native or heterologous enzymes of the fatty acid biosynthetic pathway, like diacylglycerol acyltransferase (DGAT) enzyme; increased accumulation of metabolic intermediates used for lipids synthesis, like boosting acetyl-CoA supply; disruption of competing pathways; up to modulation of transcription factors accumulation (Sun et al., 2019; Figure 3 and Table 6).
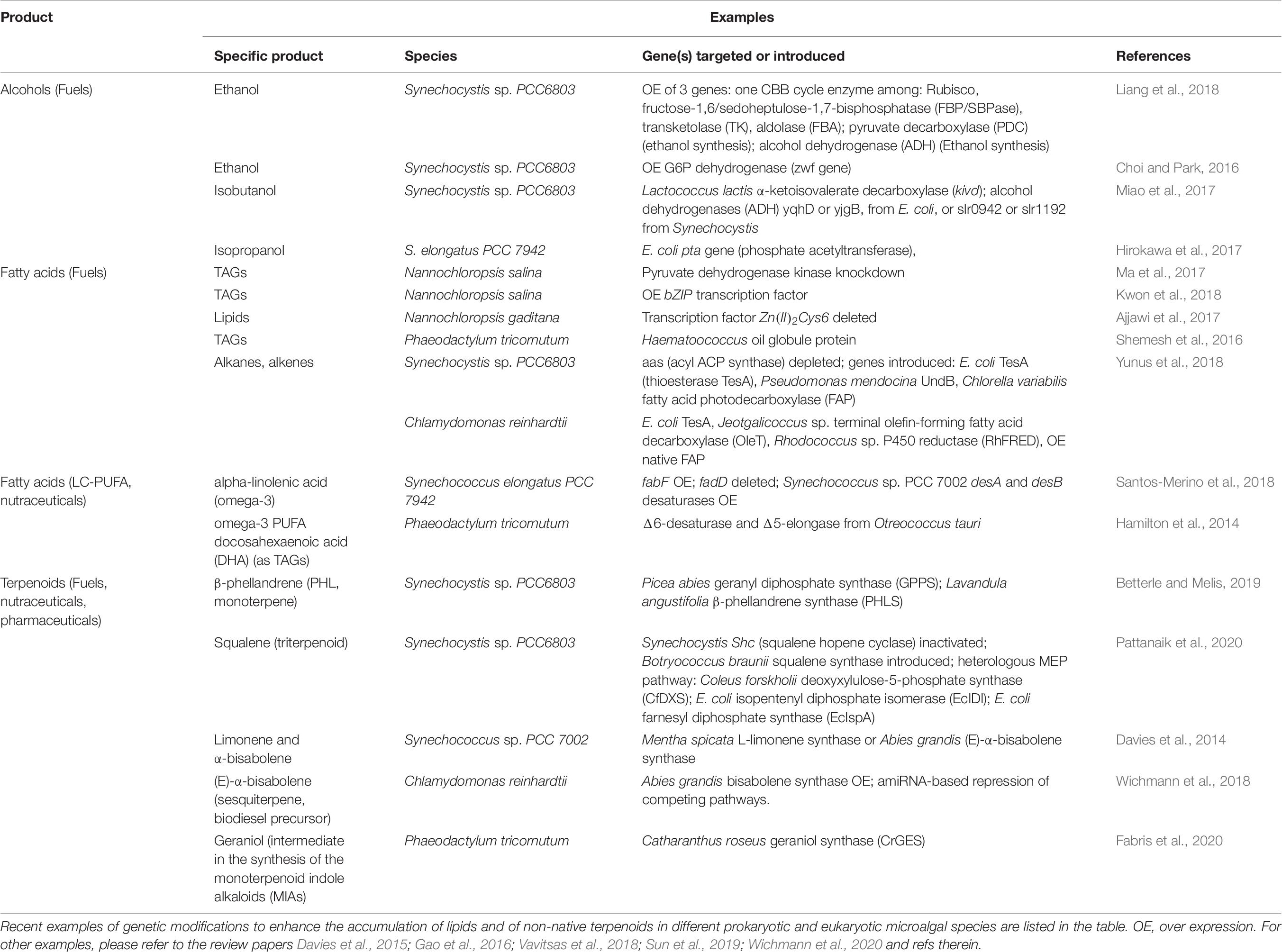
Table 6. Examples of metabolic engineering for bio-fuel precursors and high-value molecules production.
Terpenoids, also known as terpenes or isoprenoids, are another valuable class of hydrocarbon-derived molecules for industrial purposes. They are naturally produced by photosynthetic cells in the form of pigments, plant hormones and species-specific secondary metabolites with defensive role or mediating interactions with the environment (Figure 3; Pichersky and Raguso, 2018). They include thousands of chemicals with a wide range of human applications, from fuels, to pharmaceuticals (e.g., artemisinin has anti-malarial properties), to food and cosmetic additives, as menthol, limonene and squalene (Davies et al., 2015; Vavitsas et al., 2018; Wichmann et al., 2020). However, terpenoids are usually accumulated in low amount, harnessing an economically viable extraction from their natural producer. Currently, some terpenoids are produced in heterotrophic hosts like E. coli and S. cerevisiae, which require multiple genetic modification to establish the biosynthetic pathway. Conversely, microalgae already hold core terpenoid biosynthetic pathways for production of primary molecules like pigments, being a valuable host for heterologous production of non-native plant terpenes (Vavitsas et al., 2018; Wichmann et al., 2020; Table 6 lists some examples).
In addition to hydrocarbons, microalgae, particularly green algae, have been used as valuable hosts for heterologous protein productions. Proteins expressed in C. reinhardtii, but also Dunaliella salina, D. tertiolecta and Chlorella ellipsoidea include antibodies, immonotoxins, enzymes and subunits of vaccines (for a review see Rasala and Mayfield, 2015), further demonstrating the potential of microalgae as an important source of fuels, food, feed and pharmaceutical products.
Working on a Whole Cell Physiology Level: Source/Sink Balance, -Omic Sciences and Computational Simulations
The above-mentioned examples and findings on the genetic engineering of single processes in photosynthetic cells show a few folds impact on algal productivity. According to the estimations on the energy conversion efficiency and CO2 sequestration reported in Tables 3, 5, this increase is still not enough to reach a major productivity breakthrough in multiple applications. Further, many studies highlight important side effects on other cell functions. This can be due to secondary effects of the mutations, but also to unpredicted interactions among physiological processes, leading e.g., to C allocation into different ready-to-use or storage macromolecular pools, luxury uptake of nutrients, functional and compositional homeostasis (see review Giordano, 2013 for details on homeostasis; Giordano et al., 2015a; Finkel et al., 2016; Ruan et al., 2018; Giordano and Wang, 2018). Our lacunose knowledge on cell strategies regarding homeostasis, acclimation and adaptation responses, even in the case of extensively studied species (e.g., diatoms), is still a major issue in the manipulation of photosynthetic cells (Wagner et al., 2017).
It is thus clear that any attempt to improve CO2 fixation and biomass quality should be based on the holistic view of cell physiology, by simultaneously adapting (i.e., balancing) the activity and the regulation of multiple cellular functions.
First, chemical energy (ATP) and reductants produced by photosynthesis light reaction must be carefully tied with the energy cost of C fixation and other metabolic pathways (Figures 1, 4) (Cotton et al., 2015; Giordano and Wang, 2018). Remarkably, the source/sink balance is not the only way in which photosynthetic light reactions and cell metabolism are interdependent. The redox poise in chloroplast stroma generated by the electron transport, in the form of reduced ferredoxin or NADPH, is also a crucial regulatory signal for several metabolic pathways through redox regulation of multiple enzymes by the thioredoxin system (Kikutani et al., 2012; Morisse et al., 2014; Nikkanen and Rintamäki, 2014).
Further, any change driven by metabolic engineering design must also be carefully customized to the selected genetic background and the specific growth environment cells will experience in large scale production, to avoid downstream issues during the scale up of the process, which is currently among the biggest obstacles to economically viable bio-factories (Borowitzka, 2016; Newby et al., 2016).
Even though some common features of bio-factory strains can be outlined, like the ability to thrive in extreme environment to limit contaminations, a fast growth rate, a high cell density, a high biomass productivity, it is conceivable that multiple ad hoc cell chassis should be designed according to each specific purpose, to minimize all the bottlenecks of each specific production system.
Rapidly developing -omic sciences, i.e., transcriptomic, proteomic and metabolomic, shall support a comprehensive understanding of cell physiology and of the consequences of each targeted mutation on cell functions. Such a holistic view of cell functioning shall then drive the selection of additional processes which require metabolic energy optimization or which can be minimized to save metabolic energy (Figure 5). Performing direct validation of the productivity and the drawbacks of different metabolic variants with experimental studies on living cells is a labor-intensive and time-consuming procedure. Mathematical models which mimic the consequences of a mutation in silico are thus extremely relevant to develop a successful, wide-ranging, metabolic engineering approach to design photosynthetic cells as a function of the selected species and growth environments.
Biochemical simulations for photosynthetic C assimilation started in the 80s (Farquhar et al., 1980, 2001), then being constantly improved and expanded. Available models now include reactions from multiple pathways, like CBB cycle, photorespiration, starch and sucrose synthesis, as a function of nutrient availability (Zhu et al., 2007, 2013). Given the high metabolic cost of N assimilation into amino acids (Figure 4), a further advantage of computational modeling is, for example, that a constant N quota can be allocated to the whole set of enzymes considered. This will avoid additional energy investment of the cell in N acquisition, while optimizing enzymes relative stoichiometry (Zhu et al., 2007). Similar computational predictions are recently under development also for economically relevant algal species. They simulate the complex network of photosynthetic light reactions and their regulation, as well as algal metabolic reactions in different environmental conditions, like illumination regime, nutrient and CO2 availability (Chang et al., 2011; Du et al., 2018; Perin et al., 2019; Fachet et al., 2020; Toyoshima et al., 2020). Remarkably, robust mathematical models rely on a deep understanding of the cell physiology, to include as many parameters as possible in the simulation. This multidisciplinary approach shall allow to design living factory where all the house-keeping functions are present but minimized and most of the energy is directed to the commercial products (Table 5 and Figure 5).
Conclusion and Perspectives
Photosynthetic organisms that are able to fix more CO2 with less resources will be of paramount relevance to face major global challenges regarding climate change, sustainable management of natural resources, food and energy demand. Among photosynthetic organisms, microalgae have gained the highest attention due to several unique capabilities, that make them outcompete higher plants when considering their exploitation in the bio-based industry.
The microalgae evolutive history results in a variety of genotypes, wide functional diversity and metabolic flexibility, which play a crucial role in determining resource demand and use efficiency. At the same time, metabolic flexibility and homeostasis in response to external perturbations are also responsible for the fact that cultures may easily diverge from the intended biomass quality. These factors are often underestimated when selecting candidates and setting up production plants. The only chance to reach stable quality and meaningful amount of the products is to consider such physiological complexity. Further, in order to reach a clean transition toward renewable energy and circular economy, cell factories should approach the maximal theoretical value for light energy conversion into biomass. Valuable strategies leading to an economically sustainable algal cultivation are currently under research and enclose: selection of robust strains and consortia as natural producers of high-value molecules along with the implementation of nutrient recycling from wastewaters or flue gases; characterization of cell physiological responses in different environments; optimization of molecular tools for predictable genetic manipulation, metabolic design, computational modeling; selection of functional “building blocks” from different biological sources to be reassembled in optimized synthetic cell factories. Future efforts shall eventually combine a careful selection of the most appropriate genetic background, culturing conditions and ad hoc genetic engineering, to significantly improve photosynthetic cells bio-commodities productivity.
Author Contributions
MG conceived the outline of the manuscript. CG and AN wrote the manuscript and generated the figures. All authors contributed to the article and approved the submitted version.
Conflict of Interest
The authors declare that the research was conducted in the absence of any commercial or financial relationships that could be construed as a potential conflict of interest.
Acknowledgments
The authors acknowledge the financial support from Università Politecnica delle Marche (Ricerca Scientifica di Ateneo, UNIVPM 2019, to AN, and “Progetto Strategico di Ateneo”, 2017, to MG).
References
Ajjawi, I., Verruto, J., Aqui, M., Soriaga, L. B., Coppersmith, J., Kwok, K., et al. (2017). Lipid production in Nannochloropsis gaditana is doubled by decreasing expression of a single transcriptional regulator. Nat. Biotechnol. 35, 647–652. doi: 10.1038/nbt.3865
Alboresi, A., Perin, G., Vitulo, N., Diretto, G., Block, M., Jouhet, J., et al. (2016). Light remodels lipid biosynthesis in Nannochloropsis gaditana by modulating carbon partitioning between organelles. Plant Physiol. 171, 2468–2482. doi: 10.1104/pp.16.00599
Allahverdiyeva, Y., Suorsa, M., Tikkanen, M., and Aro, E.-M. (2015). Photoprotection of photosystems in fluctuating light intensities. J. Exp. Bot. 66, 2427–2436. doi: 10.1093/jxb/eru463
Andersson, I. (2008). Catalysis and regulation in Rubisco. J. Exp. Bot. 59, 1555–1568. doi: 10.1093/jxb/ern091
Aro, E.-M. (2016). From first generation biofuels to advanced solar biofuels. Ambio 45, S24–S31. doi: 10.1007/s13280-015-0730-0
Bacchetti, T., Annibaldi, A., Comitini, F., Ciani, M., Damiani, E., Norici, A., et al. (2020). “Alternative ingredients for feed and food,” in The First Outstanding 50 Years of “Università Politecnica delle Marche”, eds S. Longhi et al. (Cham: Springer). doi: 10.1007/978-3-030-33832-9_34
Badger, M. R., Andrews, T. J., Whitney, S. M., Ludwig, M., Yellowlees, D. C., Leggat, W., et al. (1998). The diversity and coevolution of Rubisco, plastids, pyrenoids, and chloroplast-based CO2-concentrating mechanisms. Can. J. Bot. 76, 1052–1071. doi: 10.1139/b98-074
Badger, M. R., and Bek, E. J. (2008). Multiple Rubisco forms in Proteobacteria: their functional significance in relation to CO2 acquisition by CBB cycle. J. Exp. Bot. 59, 1525–1541. doi: 10.1093/jxb/erm297
Baier, T., Wichmann, J., Kruse, O., and Lauersen, K. J. (2018). Intron-containing algal transgenes mediate efficient recombinant gene expression in the green microalga Chlamydomonas reinhardtii. Nucleic Acids Res. 46, 6909–6919. doi: 10.1093/nar/gky532
Barber, J. (2009). Photosynthetic energy conversion: natural and artificial. Chem. Soc. Rev. 38, 185–196. doi: 10.1039/b802262n
Barbier, G., Oesterhelt, C., Larson, M. D., Halgren, R. G., Wilkerson, C., Garavito, R. M., et al. (2005). Comparative genomics of two closely related unicellular thermo-acidophilic red algae, Galdieria sulphuraria and Cyanidioschyzon merolae, reveals the molecular basis of the metabolic flexibility of Galdieria sulphuraria and significant differences in carbohydrate metabolism of both algae. Plant Physiol. 137, 460–474. doi: 10.1104/pp.104.051169
Bar-Even, A. (2018). Daring metabolic designs for enhanced plant carbon fixation. Plant Sci. 273, 71–83.
Bar-Even, A., Noor, E., Lewis, N. E., and Milo, R. (2010). Design and analysis of synthetic carbon fixation pathways. Proc. Natl. Acad. Sci. U.S.A. 107, 8889–8894. doi: 10.1073/pnas.0907176107
Beardall, J., and Giordano, M. (2002). Ecological implications of microalgal and cyanobacterial CO2 concentrating mechanisms, and their regulation. Funct. Plant Biol. 29, 335–347. doi: 10.1071/PP01195
Beardall, J., and Raven, J. A. (2016). “Carbon acquisition by microalgae,” in The physiology of microalgae. Developments in Applied Phycology 6, eds M. A. Borowitzka, J. Beardall, and J. A. Raven (Cham: Springer), 89–99. doi: 10.1007/978-3-319-24945-2_4
Becker, E. W. (2007). Micro-algae as a source of protein. Biotechnol. Adv. 25, 207–210. doi: 10.1016/j.biotechadv.2006.11.002
Benedetti, M., Vecchi, V., Barera, S., and Dall’Osto, L. (2018). Biomass from microalgae: the potential of domestication towards sustainable biofactories. Microb. Cell Fact. 17:173.
Betterle, N., and Melis, A. (2019). Photosynthetic generation of heterologous terpenoids in cyanobacteria. Biotechnol. Bioeng. 116, 2041–2051. doi: 10.1002/bit.26988
Bilanovic, D., Holland, M., Starosvetsky, J., and Armon, R. (2016). Co-cultivation of microalgae and nitrifiers for higher biomass production and better carbon capture. Bioresour. Technol. 220, 282–288. doi: 10.1016/j.biortech.2016.08.083
Blankenship, R. E., Tiede, D. M., Barber, J., Brudvig, G. W., Fleming, G., Ghirardi, M., et al. (2011). Comparing Photosynthetic and the Potential for Improvement. Science 332, 805–810. doi: 10.1126/science.1200165
Borowitzka, M. A. (2013). High-value products from microalgae—Their development and commercialisation. J. Appl. Phycol. 25, 743–756. doi: 10.1007/s10811-013-9983-9
Borowitzka, M. A. (2016). “Algal physiology and large-scale outdoor cultures of microalgae,” in The Physiology of Microalgae. Developments in Applied Phycology 6, eds M. A. Borowitzka, J. Beardall, and J. A. Raven (Cham: Springer), 600–652. doi: 10.1007/978-3-319-24945-2_23
Brenner, K., You, L., and Arnold, F. H. (2008). Engineering microbial consortia: a new frontier in synthetic biology. Trends Biotechnol. 26, 483–489. doi: 10.1016/j.tibtech.2008.05.004
Brodie, J., Chan, C. X., De Clerck, O., Cock, J. M., Coelho, S. M., Gachon, C., et al. (2017). The algal revolution. Trends Plant Sci. 22, 726–738. doi: 10.1016/j.tplants.2017.05.005
Caballero, M. A., Jallet, D., Shi, L., Rithner, C., Zhang, Y., and Peers, G. (2016). Quantification of chrysolaminarin from the model diatom Phaeodactylum tricornutum. Algal Res. 20, 180–188. doi: 10.1016/j.algal.2016.10.008
Caffarri, S., Tibiletti, T., Jennings, R. C., and Santabarbara, S. (2014). A comparison between plant photosystem I and photosystem II architecture and functioning. Curr. Protein Pept. Sci. 15, 296–331. doi: 10.2174/1389203715666140327102218
Cardinale, B. J., Matulich, K. L., Hooper, D. U., Byrnes, J. E., Duffy, E., Gamfeldt, L., et al. (2011). The functional role of producer diversity in ecosystems. Am. J. Bot. 98, 572–592. doi: 10.3732/ajb.1000364
Cardona, T., Shao, S., and Nixon, P. J. (2018). Enhancing photosynthesis in plants: the light reactions. Essays Biochem. 62, 85–94. doi: 10.1042/EBC20170015
Carmo-Silva, E., Scales, J. C., Madgwick, P. J., and Parry, M. A. J. (2015). Optimizing Rubisco and its regulation for greater resource use efficiency. Plant Cell Environ. 38, 1817–1832. doi: 10.1111/pce.12425
Case, A. E., and Atsumi, S. (2016). Cyanobacterial chemical production. J. Biotechnol. 231, 106–114. doi: 10.1016/j.jbiotec.2016.05.023
Ceron, G. M. C., Fernandez, S. J. M., Molina, G. E., and Garcia, C. F. (2000). Mixotrophic growth of Phaeodactylum tricornutum on glycerol: growth rate and fatty acid profile. J. Appl. Phycol. 12, 239–248. doi: 10.1023/A:1008123000002
Chang, R. L., Ghamsari, L., Manichaikul, A., Hom, E. F. Y., Balaji, S., Fu, W., et al. (2011). Metabolic network reconstruction of Chlamydomonas offers insight into light-driven algal metabolism. Mol. Syst. Biol. 7:518. doi: 10.1038/msb.2011.52
Chisti, Y. (2007). Biodiesel from microalgae. Biotechnol. Adv. 25, 294–306. doi: 10.1016/j.biotechadv.2007.02.001
Choi, Y.-N., and Park, J. M. (2016). Enhancing biomass and ethanol production by increasing NADPH production in Synechocystis sp. PCC 6803. Bioresource Technol. 213, 54–57. doi: 10.1016/j.biortech.2016.02.056
Clarens, A. F., Resurreccion, E. P., White, M. A., and Colosi, L. M. (2010). Environmental life cycle comparison of algae to other bioenergy feedstocks. Environ. Sci. Technol. 44, 1813–1819. doi: 10.1021/es902838n
Corcoran, A. A., and Boeing, W. J. (2012). Biodiversity increases the productivity and stability of phytoplankton communities. PLoS One 7:e49397. doi: 10.1371/journal.pone.0049397
Cotton, C. A., Edlich-Muth, C., and Bar-Even, A. (2018). Reinforcing carbon fixation: CO2 reduction replacing and supporting carboxylation. Curr. Opin. Biotechnol. 49, 49–56. doi: 10.1016/j.copbio.2017.07.014
Cotton, C. A. R., Douglass, J. S., De Causmaecker, S., Brinkert, K., Cardona, T., Fantuzzi, A., et al. (2015). Photosynthetic constraints on fuel from microbes. Front. Bioeng. Biotechnol. 3:36. doi: 10.3389/fbioe.2015.00036
Cummins, P. L., Kannappan, B., and Gready, J. E. (2018). Directions for optimization of photosynthetic carbon fixation: RuBisCO’s efficiency may not be so constrained after all. Front. Plant Sci. 9:183. doi: 10.3389/fpls.2018.00183
Davies, F. K., Jinkerson, R. E., and Posewitz, M. C. (2015). Toward a photosynthetic microbial platform for terpenoid engineering. Photosynth. Res. 123, 265–284. doi: 10.1007/s11120-014-9979-6
Davies, F. K., Work, V. H., Beliaev, A. S., and Posewitz, M. C. (2014). Engineering limonene and bisabolene production in wild type and a glycogen-deficient mutant of Synechococcus sp. PCC 7002. Front. Bioeng. Biotechnol. 2:21. doi: 10.3389/fbioe.2014.00021
De Farias-Neves, F., Hoinaski, L., Rubi-Rörig, L., Bianchini-Derner, R., and de Melo-Lisboa, H. (2019). Carbon biofixation and lipid composition of an acidophilic microalga cultivated on treated wastewater supplied with different CO2 levels. Environ. Technol. 40, 3308–3317. doi: 10.1080/09593330.2018.1471103
de Mooij, T., Janssen, M., and Cerezo-Chinarro, O. (2015). Antenna size reduction as a strategy to increase biomass productivity: a great potential not yet realized. J. Appl. Phycol. 27, 1063–1077. doi: 10.1007/s10811-014-0427-y
De Porcellinis, A. J., Nørgaard, H., Furelos Brey, L. M., Erstad, S. M., Jones, P. R., Heazlewood, J. L., et al. (2018). Overexpression of bifunctional fructose-1,6-bisphosphatase/sedoheptulose-1,7-bisphosphatase leads to enhanced photosynthesis and global reprogramming of carbon metabolism in Synechococcus sp. PCC 7002. Metab. Eng. 47, 170–183. doi: 10.1016/j.ymben.2018.03.001
de Vargas, C., Audic, S., Henry, N., Decelle, J., Mahe, F., Logares, R., et al. (2015). Eukaryotic plankton diversity in the sunlit ocean. Science 348:1261605. doi: 10.1126/science.1261605
Diaz, M. M., and Maberly, S. C. (2009). Carbon-concentrating mechanisms in acidophilic algae. Phycologia 48, 77–85. doi: 10.2216/08-08.1
Dietrich, K., Dumont, M. J., Del Rio, L. F., and Orsat, V. (2017). Producing PHAs in the bioeconomy – Towards a sustainable bioplastic. Sustain. Prod. Consum. 9, 58–70. doi: 10.1016/j.spc.2016.09.001
Doron, L., Segal, N., and Shapira, M. (2016). Transgene expression in microalgae—From tools to applications. Front. Plant Sci. 7:505. doi: 10.3389/fpls.2016.00505
Du, W., Jongbloets, J. A., van Boxtel, C., Pineda Hernández, H., Lips, D., Oliver, B. G., et al. (2018). Alignment of microbial fitness with engineered product formation: obligatory coupling between acetate production and photoautotrophic growth. Biotechnol. Biofuels 11:38.
Eibl, J. K., Corcoran, J. D., Senhorinho, G. A., Zhang, K., Hosseini, N. S., Marsden, J., et al. (2014). Bioprospecting for acidophilic lipid-rich green microalgae isolated from abandoned mine site water bodies. AMB Expr. 4:7. doi: 10.1186/2191-0855-4-7
Enzing, C., Ploeg, M., Barbosa, M., and Sijtsma, L. (2014). Microalgae-Based Products for the Food and Feed Sector: An Outlook for Europe. JRC Scientific and Policy Reports. Report No. EUR 26255 EN. Brussels: European Union. doi: 10.2791/3339
Fabris, M., George, J., Kuzhiumparambil, U., Lawson, C. A., Jaramillo-Madrid, A. C., Abbriano, R. M., et al. (2020). Extrachromosomal genetic engineering of the marine diatom Phaeodactylum tricornutum enables the heterologous production of monoterpenoids. ACS Synth. Biol. 9, 598–612. doi: 10.1021/acssynbio.9b00455
Fachet, M., Witte, C., Flassig, R. J., Rihko-struckmann, L. K., Mckie-krisberg, Z., Polle, J. E. W., et al. (2020). Reconstruction and analysis of a carbon-core metabolic network for Dunaliella salina. BMC Bioinformatics 21:1. doi: 10.1186/s12859-019-3325-0
Falkowski, P., and Raven, J. A. (2007). Aquatic Photosynthesis 488, 2nd Edn. Princeton, NJ: Princeton University Press.
Fang, L., Lin, H. X., Low, C. S., Wu, M. H., Chow, Y., et al. (2012). Expression of the Chlamydomonas reinhardtii Sedoheptulose-1, 7-bisphosphatase in Dunaliella bardawil leads to enhanced photosynthesis and increased glycerol production. Plant Biotechnol. J. 10, 1129–1135. doi: 10.1111/pbi.12000
Farquhar, G. D., von Caemmerer, S., and Berry, J. A. (1980). A biochemical model of photosynthetic CO2 assimilation in leaves of C3 species. Planta 149, 78–90. doi: 10.1007/BF00386231
Farquhar, G. D., von Caemmerer, S., and Berry, J. A. (2001). Models of photosynthesis. Plant Physiol. 125, 42–45. doi: 10.1104/pp.125.1.42
Finkel, Z. V., Follows, M. J., Liefer, J. D., Brown, C. M., Benner, I., and Irwin, A. J. (2016). Phylogenetic diversity in the macromolecular composition of microalgae. PLoS One 11:e0155977. doi: 10.1371/journal.pone.0155977
Gallardo-Rodríguez, J., Sanchez-Mirón, A., García-Camacho, F., López-Rosales, L., Chisti, Y., and Molina-Grima, E. (2012). Bioactives from microalgal dinoflagellates. Biotechnol. Adv. 30, 1673–1684. doi: 10.1016/j.biotechadv.2012.07.005
Gao, X., Sun, T., Pei, G., Chen, L., and Zhang, W. (2016). Cyanobacterial chassis engineering for enhancing production of biofuels and chemicals. Appl. Microbiol. Biotechnol. 100, 3401–3413. doi: 10.1007/s00253-016-7374-2
Geider, R., and La Roche, J. (2002). Redfield revisited: variability of C:N:P in marine microalgae and its biochemical basis. Eur. J. Phycol. 37, 1–17. doi: 10.1017/S0967026201003456
Gerotto, C., Alboresi, A., Meneghesso, A., Jokel, M., Suorsa, M., Aro, E.-M., et al. (2016). Flavodiiron proteins act as safety valve for electrons in Physcomitrella patens. Proc. Natl. Acad. Sci. U.S.A. 113, 12322–12327. doi: 10.1073/pnas.1606685113
Gerotto, C., and Morosinotto, T. (2013). Evolution of photoprotection mechanisms upon land colonization: evidence of PSBS-dependent NPQ in late Streptophyte algae. Physiol. Plant. 149, 583–598. doi: 10.1111/ppl.12070
Giordano, M. (2013). Homeostasis: an underestimated focal point of ecology and evolution. Plant Sci. 211, 92–101. doi: 10.1016/j.plantsci.2013.07.008
Giordano, M., Beardall, J., and Raven, J. A. (2005). CO2 concentrating mechanisms in algae: mechanisms, environmental modulation, and evolution. Annu. Rev. Plant Biol. 56, 99–131. doi: 10.1146/annurev.arplant.56.032604.144052
Giordano, M., Norici, A., and Beardall, J. (2015a). Impact of inhibitors of amino acid, protein, and RNA synthesis on C allocation in the diatom Chaetoceros muellerii: a FTIR approach. Algae 32, 161–170. doi: 10.4490/algae.2017.32.6.6
Giordano, M., Palmucci, M., and Norici, A. (2015b). Taxonomy and growth conditions concur to determine the energetic suitability of algal fatty acid complements. J. Appl. Phycol. 27, 1401–1413. doi: 10.1007/s10811-014-0457-5
Giordano, M., and Ratti, S. (2013). The biomass quality of algae used for CO2 sequestration is highly species-specific and may vary over time. J. Appl. Phycol. 25, 1431–1434. doi: 10.1007/s10811-012-9966-2
Giordano, M., and Raven, J. A. (2014). Nitrogen and sulfur assimilation in plants and algae. Aquat. Bot. 118, 45–61. doi: 10.1016/j.aquabot.2014.06.012
Giordano, M., and Wang, Q. (2018). “Microalgae for industrial purposes,” in Biomass and Green Chemistry: Building a renewable Pathway, ed. S. Vaz (Cham: Springer). doi: 10.1007/978-3-319-66736-2_6
Granum, E., Kirkvold, S., and Myklestad, S. M. (2002). Cellular and extracellular production of carbohydrates and amino acids by the marine diatom Skeletonema costatum: diel variations and effects of N depletion. Mar. Ecol. Prog. Ser. 242, 82–93. doi: 10.3354/meps242083
Hagemann, M., Kern, R., Maurino, V. G., Hanson, D. T., Weber, A. P. M., Sage, R. F., et al. (2016). Evolution of photorespiration from cyanobacteria to land plants, considering protein phylogenies and acquisition of carbon concentrating mechanisms. J. Exp. Bot. 67, 2963–2976. doi: 10.1093/jxb/erw063
Hamilton, M., Haslam, R., Napier, J., and Sayanova, O. (2014). Metabolic engineering of microalgae for enhanced production of omega-3 long chain polyunsaturated fatty acids. Metab. Eng. 22, 3–9. doi: 10.1016/j.ymben.2013.12.003
Henley, W. J., Litaker, R. W., Novoveská, L., Duke, C. S., Quemada, H. D., and Sayre, R. T. (2013). Initial risk assessment of genetically modified (GM) microalgae for commodity-scale biofuel cultivation. Algal Res. 2, 66–77. doi: 10.1016/j.algal.2012.11.001
Heureux, A. M. C., Young, J. N., Whitney, S. M., Eason-Hubbard, M. R., Lee, R. B. Y., Sharwood, R. E., et al. (2017). The role of Rubisco kinetics and pyrenoid morphology in shaping the CCM of haptophyte microalgae. J. Exp. Bot. 68, 3959–3969. doi: 10.1093/jxb/erx179
Hirokawa, Y., Dempo, Y., Fukusaki, E., and Hanai, T. (2017). Metabolic engineering for isopropanol production by an engineered cyanobacterium, Synechococcus elongatus PCC 7942, under photosynthetic conditions. J. Biosci. Bioeng. 12, 39–45. doi: 10.1016/j.jbiosc.2016.07.005
Ho, T.-Y., Quigg, A., Finkel, Z. V., Allen, J. M., Wyman, K., Falkowski, P. G., et al. (2003). Elemental composition of some marine phytoplankton. J. Phycol. 39, 1145–1159. doi: 10.1111/j.0022-3646.2003.03-090.x
Hopkinson, B. M., Dupont, C. L., Allen, A. E., and Morel, F. M. M. (2011). Efficiency of the CO2-concentrating mechanism of diatoms. Proc. Natl. Acad. Sci. U.S.A. 108, 3830–3837. doi: 10.1073/pnas.1018062108
Hopkinson, B. M., Dupont, C. L., and Matsuda, Y. (2016). The physiology and genetics of CO2 concentrating mechanisms in model diatoms. Curr. Opin. Plant Biol. 31, 51–57. doi: 10.1016/j.pbi.2016.03.013
Hossain, N., Zaini, J., and Indra Mahlia, T. M. (2019). Life cycle assessment, energy balance and sensitivity analysis of bioethanol production from microalgae in a tropical country. Renew. Sustain. Energy Rev. 115:109371. doi: 10.1016/j.rser.2019.109371
International Energy Agency (2017). State of Technology Review on Algae Bioenergy. Available online at: https://www.ieabioenergy.com/wp-content/uploads/2016/01/Laurens-Algae-Bioenergy-Report-IEA-webinar-170124-final-rev1.pdf (accessed March 10, 2020).
International Energy Agency (2020a). Global CO2 Emissions in 2019. Available online at: https://www.iea.org/articles/global-co2-emissions-in-2019 (accessed March 10, 2020).
International Energy Agency (2020b). Transport Biofuels -Analyses. Available online at: https://www.iea.org/reports/transport-biofuels (accessed June 24, 2020).
International Panel of Climate Change [IPCC] (2014). Synthesis Report. Contribution of Working Groups I, II and III to the Fifth Assessment Report of the Intergovernmental Panel on Climate Change [Core Writing Team, eds R. K. Pachauri and L. A. Meyer (Geneva: IPCC).
Jakob, T., Wagner, H., Stehfest, K., and Wilhelm, C. (2007). A complete energy balance from photons to new biomass reveals a light- and nutrient-dependent variability in the metabolic costs of carbon assimilation. J. Exp. Bot. 58, 2101–2112. doi: 10.1093/jxb/erm084
Jinkerson, R. E., and Jonikas, M. C. (2015). Molecular techniques to interrogate and edit the Chlamydomonas nuclear genome. Plant J. 82, 393–412. doi: 10.1111/tpj.12801
Kamalanathan, M., and Quigg, A. (2019). Physiological Limitations and Solutions to Various Applications of Microalgae,” in Microalgae. Available online at: https://www.intechopen.com/books/microalgae-from-physiology-to-application/physiological-limitations-and-solutions-to-various-applications-of-microalgae (accessed January 29, 2020).
Karlsson, L., Clarke, A. K., Chen, Z.-Y., Hugghins, S. Y., and Park, Y. I. (1998). A novel α-type carbonic anhydrase associated with the thylakoid membrane in Chlamydomonas reinhardtii is required for growth at ambient CO2. EMBO J. 17, 1208–1216. doi: 10.1093/emboj/17.5.1208
Kerfeld, C. A. (2015). Plug-and-play for improving primary productivity. Am. J. Bot. 102, 1949–1950. doi: 10.3732/ajb.1500409
Kikutani, S., Nakajima, K., Nagasato, C., Tsuji, Y., Miyatake, A., and Matsuda, Y. (2016). Thylakoid luminal u-carbonic anhydrase critical for growth and photosynthesis in the marine diatom Phaeodactylum tricornutum. Proc. Natl Acad. Sci. U.S.A. 113, 9828–9833. doi: 10.1073/pnas.1603112113
Kikutani, S., Tanaka, R., Yamazaki, Y., Hara, S., Hisabori, T., Kroth, P. G., et al. (2012). Redox regulation of carbonic anhydrases via thioredoxin in chloroplast of the marine diatom Phaeodactylum tricornutum. J. Biol. Chem. 287, 20689–20700. doi: 10.1074/jbc.M111.322743
Kirst, H., Formighieri, C., and Melis, A. (2014). Maximizing photosynthetic efficiency and culture productivity in cyanobacteria upon minimizing the phycobilisome light-harvesting antenna size. Biochim. Biophys. Acta Bioenerg. 1837, 1653–1664. doi: 10.1016/j.bbabio.2014.07.009
Kirst, H., Gabilly, S. T., Niyogi, K. K., Lemaux, P. G., and Melis, A. (2017). Photosynthetic antenna engineering to improve crop yields. Planta 245, 1009–1020. doi: 10.1007/s00425-017-2659-y
Kliebenstein, D. J. (2014). Synthetic biology of metabolism: using natural variation to reverse engineer systems. Curr. Opin. Plant Biol. 19, 20–26. doi: 10.1016/j.pbi.2014.03.008
Kodama, M., Ikemoto, H., and Miyachi, S. (1993). A new species of highly CO2-tolerant fast-growing marine microalga for high-density cultivation. J. Mar. Biotechnol. 1, 21–25.
Kromdijk, J., Glowacka, K., Leonelli, L., Gabilly, S. T., Iwai, M., Niyogi, K. K., et al. (2016). Improving photosynthesis and crop productivity by accelerating recovery from photoprotection. Science 354, 857–861. doi: 10.1126/science.aai8878
Kroth, P. G., Bones, A. M., Daboussi, F., Ferrante, M. I., Jaubert, M., Kolot, M., et al. (2018). Genome editing in diatoms: achievements and goals. Plant Cell Rep. 37, 1401–1408.
Kroth, P. G., Chiovitti, A., Gruber, A., Martin-Jezequel, V., Mock, T., Parker, M. S., et al. (2008). A model for carbohydrate metabolism in the diatom Phaeodactylum tricornutum deduced from comparative whole genome analysis. PLoS One 3:e1426. doi: 10.1371/journal.pone.0001426
Kwon, S., Kang, N. K., Koh, H. G., Shin, S.-E., Lee, B., Jeong, B., et al. (2018). Enhancement of biomass and lipid productivity by overexpression of a bZIP transcription factor in Nannochloropsis salina. Biotechnol. Bioeng. 115, 331–340. doi: 10.1002/bit.26465
Lee, T., Tseng, Y., Cheng, C., Chen, Y., Lin, C., Su, H., et al. (2017). Characterization of a heat-tolerant Chlorella sp. GD mutant with enhanced photosynthetic CO2 fixation efficiency and its implication as lactic acid fermentation feedstock. Biotechnol. Biofuels 10:214. doi: 10.1186/s13068-017-0905-y
Lepage, G., and Roy, C. C. (1984). Improved recovery of fatty acid through direct transesterification without prior extraction or purification. J. Lipid Res. 25, 1391–1396.
Lepetit, B., Gélin, G., Lepetit, M., Sturm, S., Vugrinec, S., Rogato, A., et al. (2017). The diatom Phaeodactylum tricornutum adjusts nonphotochemical fluorescence quenching capacity in response to dynamic light via fine-tuned Lhcx and xanthophyll cycle pigment synthesis. New Phytol. 214, 205–218. doi: 10.1111/nph.14337
Li, G., Brown, C. M., Jeans, J. A., Donaher, N. A., McCarthy, A., and Campbell, D. A. (2015). The nitrogen costs of photosynthesis in a diatom under current and future pCO2. New Phytol. 205, 533–543. doi: 10.1111/nph.13037
Li, Y., Horsman, M., Wang, B., Wu, N., and Lan, C. Q. (2008). Effects of nitrogen sources on cell growth and lipid accumulation of green alga Neochloris oleoabundans. Appl. Microbiol. Biotechnol. 81, 629–636. doi: 10.1007/s00253-008-1681-1
Liang, F., Englund, E., Lindberg, P., and Lindblad, P. (2018). Engineered cyanobacteria with enhanced growth show increased ethanol production and higher biofuel to biomass ratio. Metab. Eng. 46, 51–59. doi: 10.1016/j.ymben.2018.02.006
Liang, F., and Lindblad, P. (2016). Effects of overexpressing photosynthetic carbon flux control enzymes in the cyanobacterium Synechocystis PCC 6803. Metab. Eng. 38, 56–64. doi: 10.1016/j.ymben.2016.06.005
Liberton, M., Chrisler, W. B., Nicora, C. D., Moore, R. J., Smith, R. D., Koppenaal, D. W., et al. (2017). Phycobilisome truncation causes widespread proteome changes in Synechocystis sp. PCC 6803. PLoS One 12:e0173251. doi: 10.1371/journal.pone.0173251
Liu, W., and Steward, C. N. J. (2015). Plant synthetic biology. Trends Plant Sci. 20, 309–317. doi: 10.1016/j.tplants.2015.02.004
López, G., Yate, C., Ramos, F. A., Cala, M. P., Restrepo, S., and Baena, S. (2019). Production of polyunsaturated fatty acids and lipids from autotrophic, mixotrophic and heterotrophic cultivation of Galdieria sp. strain USBA-GBX-832. Sci. Rep. 9:10791. doi: 10.1038/s41598-019-46645-3
Luan, G., and Lu, X. (2018). Tailoring cyanobacterial cell factory for improved industrial properties. Biotechnol. Adv. 36, 430–442. doi: 10.1016/j.biotechadv.2018.01.005
Luengo, J. M., García, B., Sandoval, A., Naharro, G., and Olivera, E. A. R. (2003). Bioplastics from microorganisms. Curr. Opin. Microbiol. 6, 251–260. doi: 10.1016/S1369-5274(03)00040-7
Ma, X., Yao, L., Yang, B., Lee, Y. K., Chen, F., and Liu, J. (2017). RNAi-mediated silencing of a pyruvate dehydrogenase kinase enhances triacylglycerol biosynthesis in the oleaginous marine alga Nannochloropsis salina. Sci. Rep. 7:11485. doi: 10.1038/s41598-017-11932-4
Marella, T. K., Datta, A., Patil, M. D., Dixit, S., and Tiwari, A. (2019). Biodiesel production through algal cultivation in urban wastewater using algal floway. Bioresour. Technol. 280, 222–228.
Martins, D. A., Custódio, L., Barreira, L., Pereira, H., Ben-Hamadou, R., Varela, J., et al. (2013). Alternative sources of n-3 long-chain polyunsaturated fatty acids in marine microalgae. Mar. Drugs 11, 2259–2281. doi: 10.3390/md11072259
Matsuda, Y., Hopkinson, B. M., Nakajima, K., Dupont, C. L., and Tsuji, Y. (2017). Mechanisms of carbon dioxide acquisition and CO2 sensing in marine diatoms: a gateway to carbon metabolism. Philos. Trans. R. Soc. B 372:20160403. doi: 10.1098/rstb.2016.0403
Melis, A. (2009). Solar energy conversion efficiencies in photosynthesis: minimizing the chlorophyll antennae to maximize efficiency. Plant Sci. 177, 272–280. doi: 10.1016/j.plantsci.2009.06.005
Meneghesso, A., Simionato, D., Gerotto, C., La Rocca, N., Finazzi, G., and Morosinotto, T. (2016). Photoacclimation of photosynthesis in the Eustigmatophycean Nannochloropsis gaditana. Photosynth. Res. 129, 291–305. doi: 10.1007/s11120-016-0297-z
Miao, R., Liu, X., Englund, E., Lindberg, P., and Lindblad, P. (2017). Isobutanol production in Synechocystis PCC 6803 using heterologous and endogenous alcohol dehydrogenases. Metab. Eng. Commun. 5, 45–53. doi: 10.1016/j.meteno.2017.07.003
Montechiaro, F., and Giordano, M. (2010). Compositional homeostasis of the dinoflagellate Protoceratium reticulatum grown at three different pCO2. J. Plant Physiol. 167, 110–113. doi: 10.1016/j.jplph.2009.07.013
Morales, M., Sanchez, L., and Revah, S. (2018). The impact of environmental factors on carbon dioxide fixation by microalgae. FEMS Microbiol. Lett. 365:fnx262. doi: 10.1093/femsle/fnx262
Morisse, S., Michelet, L., Bedhomme, M., Marchand, C. H., Calvaresi, M., Trost, P., et al. (2014). Thioredoxin-dependent redox regulation of chloroplastic phosphoglycerate kinase from Chlamydomonas reinhardtii. J. Biol. Chem. 289, 30012–30024. doi: 10.1074/jbc.M114.597997
Muradyan, E. A., Klyachko-Gurvich, G. L., Tsoglin, L. N., Sergeyenko, T. V., and Pronina, N. A. (2004). Changes in lipid metabolism during adaptation of the Dunaliella salina photosynthetic apparatus to high CO2 concentration. Russ. J. Plant Physiol. 51, 53–62. doi: 10.1023/B:RUPP.0000011303.11957.48
Murphy, D. J., and Vance, J. (1999). Mechanisms of lipid-body formation. Trends Biochem. Sci. 24, 109–115. doi: 10.1016/S0968-0004(98)01349-8
Mussgnug, J. H., Thomas-Hall, S., Rupprecht, J., Foo, A., Klassen, V., McDowall, A., et al. (2007). Engineering photosynthetic light capture: impacts on improved solar energy to biomass conversion. Plant Biotechnol. J. 5, 802–814. doi: 10.1111/j.1467-7652.2007.00285.x
Nagappan, S., Tsai, P., Devendran, S., Alagarsamy, V., and Ponnusamy, V. K. (2020). Enhancement of biofuel production by microalgae using cement flue gas as substrate. Environ. Sci. Pollut. Res. 27, 17571–17586. doi: 10.1007/s11356-019-06425-y
Nakajima, Y., Tsuzuki, M., and Ueda, R. (2001). Improved productivity by reduction of the content of light-harvesting pigment in Chlamydomonas perigranulata. J. Appl. Phycol. 13, 95–101.
Neupert, J., Karcher, D., and Bock, R. (2009). Generation of Chlamydomonas strains that efficiently express nuclear transgenes. Plant J. 57, 1140–1150. doi: 10.1111/j.1365-313X.2008.03746.x
Newby, D. T., Mathews, T. J., Pate, R. C., Huesemann, M. H., Lane, T. W., Wahlen, B. D., et al. (2016). Assessing the potential of polyculture to accelerate algal biofuel production. Algal Res. 19, 264–277. doi: 10.1016/j.algal.2016.09.004
Ng, A. H., Berla, B. M., and Pakrasi, H. B. (2015). Fine-Tuning of photoautotrophic protein production by combining promoters and neutral sites in the cyanobacterium Synechocystis sp. Appl. Environ. Microbiol. 81, 6857–6863.
Ng, I., Keskin, B. B., and Tan, S. (2020). A critical review of genome editing and synthetic biology applications in metabolic engineering of microalgae and cyanobacteria. Biotechnol. J. 15:1900228. doi: 10.1002/biot.201900228
Nikkanen, L., and Rintamäki, E. (2014). Thioredoxin-dependent regulatory networks in chloroplasts under fluctuating light conditions. Philos. Trans. R. Soc. Lond. B. Biol. Sci. 369:20130224. doi: 10.1098/rstb.2013.0224
Niyogi, K. K., and Truong, T. B. (2013). Evolution of flexible non-photochemical quenching mechanisms that regulate light harvesting in oxygenic photosynthesis. Curr. Opin. Plant Biol. 16, 307–314. doi: 10.1016/j.pbi.2013.03.011
Nogueira, D. P. K., Silva, A. F., Araújo, O. Q. F., and Chaloub, R. M. (2015). Impact of temperature and light intensity on triacylglycerol accumulation in marine microalgae. Biomass Bioenerg. 72, 280–287. doi: 10.1016/j.biombioe.2014.10.017
Norici, A., Dalsass, A., and Giordano, M. (2002). Role of phosphoenolpyruvate carboxylase in anaplerosis in the green microalga Dunaliella salina cultured under different nitrogen regimes. Physiol. Plant. 116, 186–191. doi: 10.1034/j.1399-3054.2002.1160207.x
Norici, A., Hell, R., and Giordano, M. (2005). Sulfur and primary production in aquatic environments: an ecological perspective. Photosynth. Res. 86, 409–417. doi: 10.1007/s11120-005-3250-0
Nürnberg, D. J., Morton, J., Santabarbara, S., Telfer, A., Joliot, P., Antonaru, L. A., et al. (2018). Photochemistry beyond the red limit in chlorophyll f–containing photosystems. Science 360, 1210–1213. doi: 10.1126/science.aar8313
Nymark, M., Sharma, A. K., Sparstad, T., Bones, A. M., and Winge, P. (2016). A CRISPR/Cas9 system adapted for gene editing in marine algae. Sci. Rep. 6:24951. doi: 10.1038/srep24951
Nymark, M., Volpe, C., Hafskjold Grønbech, C. M., Kirst, H., Serif, M., Vadstein, O., et al. (2019). Loss of ALBINO3b insertase results in truncated light-harvesting antenna in diatoms. Plant Physiol. 181, 1257–1276. doi: 10.1104/pp.19.00868
Ogawa, T., Tamoi, M., Kimura, A., Mine, A., Sakuyama, H., Yoshida, E., et al. (2015). Enhancement of photosynthetic capacity in Euglena gracilis by expression of cyanobacterial fructose-1,6-/sedoheptulose-1,7-bisphosphatase leads to increases in biomass and wax ester production. Biotechnol. Biofuels 8:80.
Ooms, M. D., Dinh, C. T., Sargent, E. H., and Sinton, D. (2016). Photon management for augmented photosynthesis. Nat. Commun. 7:12699. doi: 10.1038/ncomms12699
Ort, D. R., Merchant, S. S., Alric, J., Barkan, A., Blankenship, R. E., and Bock, R. (2015). Redesigning photosynthesis to sustainably meet global food and bioenergy demand. Proc. Natl. Acad. Sci. U.S.A. 112, 8529–8536. doi: 10.1073/pnas.1424031112
Ortega-Escalante, J. A., Jasper, R., and Miller, S. M. (2019). CRISPR/Cas9 mutagenesis in Volvox carteri. Plant J. 97, 661–672. doi: 10.1111/tpj.14149
Packer, M. (2009). Algal capture of carbon dioxide; biomass generation as a tool for greenhouse gas mitigation with reference to New Zealand energy strategy and policy. Energy Policy 37, 3428–3437. doi: 10.1016/j.enpol.2008.12.025
Page, L. E., Liberton, M., and Pakrasi, H. B. (2012). Phycobilisome antenna truncation reduces photoautotrophic productivity in Synechocystis sp. PCC 6803, a cyanobacterium. Appl. Environ. Microbiol. 165, 705–714.
Palmucci, M., Ratti, S., and Giordano, M. (2011). Ecological and evolutionary implications of carbon allocation in marine phytoplankton as a function of nitrogen availability: a Fourier transform infrared spectroscopy approach. J. Phycol. 47, 313–323. doi: 10.1111/j.1529-8817.2011.00963.x
Patel, V. K., Soni, N., Prasad, V., Saper, A., Dasgupta, S., and Bhadra, B. (2019). CRISPR – Cas9 System for genome engineering of photosynthetic microalgae. Mol. Biotechnol. 61, 541–561.
Patron, N. J., and Keeling, P. J. (2005). Common evolutionary origin of starch biosynthetic enzymes in green and red algae. J. Phycol. 41, 1131–1141. doi: 10.1111/j.1529-8817.2005.00135.x
Pattanaik, B., Englund, E., Nolte, N., and Lindberg, P. (2020). Introduction of a green algal squalene synthase enhances squalene accumulation in a strain of Synechocystis sp. PCC 6803. Metab. Eng. Commun. 10:e00125. doi: 10.1016/j.mec.2020.e00125
Peers, G. (2014). Increasing algal photosynthetic productivity by integrating ecophysiology with systems biology. Trends Biotechnol. 32, 551–555. doi: 10.1016/j.tibtech.2014.09.007
Peers, G., Truong, T. B., Ostendorf, E., Busch, A., Elrad, D., Grossman, A. R., et al. (2009). An ancient light-harvesting protein is critical for the regulation of algal photosynthesis. Nature 462, 518–521. doi: 10.1038/nature08587
Peltier, G., Tolleter, D., Billon, E., and Cournac, L. (2010). Auxiliary electron transport pathways in chloroplasts of microalgae. Photosynth. Res. 106, 19–31.
Peramuna, A., and Summers, M. L. (2014). Composition and occurrence of lipid droplets in the cyanobacterium Nostoc punctiforme. Arch. Microbiol. 196, 881–890. doi: 10.1007/s00203-014-1027-6
Pérez, A. A., Chen, Q., Pineda Hernandez, H., Branco, dos Santos, F., and Hellingwerf, K. J. (2019). On the use of oxygenic photosynthesis for the sustainable production of commodity chemicals. Physiol. Plant. 166, 413–427. doi: 10.1111/ppl.12946
Perin, G., Bellan, A., Bernardi, A., Bezzo, F., and Morosinotto, T. (2019). The potential of quantitative models to improve microalgae photosynthetic efficiency. Physiol. Plant. 166, 380–391. doi: 10.1111/ppl.12915
Perrine, Z., Negi, S., and Sayre, R. T. (2012). Optimization of photosynthetic light energy utilization by microalgae. Algal Res. 1, 134–142. doi: 10.1016/j.algal.2012.07.002
Picardo, M. C., de Medeiros, J. L., Monteiro, J. G. M., Chaloub, R. M., Giordano, M., de Queiroz, O., et al. (2013). A methodology for screening of microalgae as a decision making tool for energy and green chemical process applications. Clean Technol. Environ. Policy 15, 275–291. doi: 10.1007/s10098-012-0508-z
Pichersky, E., and Raguso, R. A. (2018). Why do plants produce so many terpenoid compounds? New Phytol. 220, 692–702. doi: 10.1111/nph.14178
Pinto, F., Pacheco, C. C., Oliveira, P., Montagud, A., Landels, A., Couto, N., et al. (2015). Improving a Synechocystis -based photoautotrophic chassis through systematic genome mapping and validation of neutral sites. DNA Res. 22, 425–437. doi: 10.1093/dnares/dsv024
Polle, J. E. W., Kanakagiri, S., and Melis, A. (2003). tla1, a DNA insertional transformant of the green alga Chlamydomonas reinhardtii with a truncated light-harvesting chlorophyll antenna size. Planta 217, 49–59.
Quigg, A., Irwin, A. J., and Finkel, Z. V. (2010). Evolutionary inheritance of elemental stoichiometry in phytoplankton. Proc. R. Soc. B 278, 526–534. doi: 10.1098/rspb.2010.1356
Rasala, B. A., and Mayfield, S. P. (2015). Photosynthetic biomanufacturing in green algae; production of recombinant proteins for industrial, nutritional, and medical uses. Photosynth. Res. 123, 227–239. doi: 10.1007/s11120-014-9994-7
Rasala, B. A., Muto, M., Sullivan, J., and Mayfield, S. P. (2011). Improved heterologous protein expression in the chloroplast of Chlamydomonas reinhardtii through promoter and 5’ untranslated region optimization. Plant Biotechnol. J. 9, 674–683. doi: 10.1111/j.1467-7652.2011.00620.x
Raso, S., Genugten, B., Vermuë, M., and Wijffels, R. (2012). Effect of oxygen concentration on the growth of Nannochloropsis sp. at low light intensity. J. Appl. Phycol. 24, 863–871. doi: 10.1007/s10811-011-9706-z
Raven, J. A. (1997a). Inorganic carbon acquisition by marine autotrophs. Adv. Bot. Res. 27, 85–209. doi: 10.1016/S0065-2296(08)60281-5
Raven, J. A. (1997b). CO2 concentrating mechanisms: a direct role for thylakoid lumen acidification? Plant Cell Environ. 20, 147–154. doi: 10.1046/j.1365-3040.1997.d01-67.x
Raven, J. A. (2010). Inorganic carbon acquisition by eukaryotic algae: four current questions. Photosynth. Res. 106, 123–134. doi: 10.1007/s11120-010-9563-7
Raven, J. A., Beardall, J., and Giordano, M. (2014). Energy costs of carbon dioxide concentrating mechanisms in aquatic organisms. Photosynth. Res. 121, 111–124.
Raven, J. A., and Farquhar, G. D. (1990). The influence of N metabolism and organic acid synthesis on the natural abundance of C isotopes in plants. New Phytol. 116, 505–529. doi: 10.1111/j.1469-8137.1990.tb00536.x
Raven, J. A., and Giordano, M. (2014). Algae. Curr. Biol. 24, R590–R595. doi: 10.1016/j.cub.2014.05.039
Raven, J. A., Giordano, M., Beardall, J., and Maberly, S. C. (2011). Algal and aquatic plant carbon concentrating mechanisms in relation to environmental change. Photosynth. Res. 109, 281–296. doi: 10.1007/s11120-011-9632-6
Raven, J. A., Giordano, M., Beardall, J., and Maberly, S. C. (2012). Algal evolution in relation to atmospheric CO2: carboxylases, carbon-concentrating mechanisms and carbon oxidation cycles. Philos. Trans. R Soc. B 367, 493–507. doi: 10.1098/rstb.2011.0212
Raven, J. A., Kübler, J. E., and Beardall, J. (2000). Put out the light and then put out the light. J. Mar. Biol. Assoc. U.K. 80, 1–25. doi: 10.1017/S0025315499001526
Richardson, J. W., Johnson, M. D., Zhang, X., Zemke, P., Chen, W., and Hu, Q. (2014). A financial assessment of two alternative cultivation systems and their contributions to algae biofuel economic viability. Algal Res. 4, 96–104.
Romero, E., Novoderezhkin, V. I., and Van Grondelle, R. (2017). Quantum design of photosynthesis for bio-inspired solar-energy conversion. Nature 543, 355–365. doi: 10.1038/nature22012
Ruan, Z., and Giordano, M. (2017). The use of NH4+ rather than NO3- affects cell stoichiometry, C allocation, photosynthesis and growth in the cyanobacterium Synechococcus sp. UTEX LB 2380, only when energy is limiting. Plant Cell Environ. 40, 227–236. doi: 10.1111/pce.12858
Ruan, Z., Prášil, O., and Giordano, M. (2018). The phycobilisomes of Synechococcus sp. are constructed to minimize nitrogen use in nitrogen-limited cells and to maximize energy capture in energy-limited cells. Environ. Exp. Bot. 150, 152–160. doi: 10.1016/j.envexpbot.2018.01.015
Ruan, Z., Raven, J. A., and Giordano, M. (2017). In Synechococcus sp. competition for energy between assimilation and acquisition of C and those of N only occurs when growth is light limited. J. Exp. Bot. 68, 3829–3839. doi: 10.1093/jxb/erx074
Santos-Merino, M., Garcillán-Barcia, M. P., and de la Cruz, F. (2018). Engineering the fatty acid synthesis pathway in Synechococcus elongatus PCC 7942 improves omega-3 fatty acid production. Biotechnol. Biofuels 11:239. doi: 10.1186/s13068-018-1243-4
Savir, Y., Noor, E., Milo, R., and Tlusty, T. (2010). Cross-species analysis traces adaptation of Rubisco toward optimality in a low-dimensional landscape. Proc. Natl. Acad. Sci. U.S.A. 107, 3475–3480. doi: 10.1073/pnas.0911663107
Sayanova, O., Mimouni, V., Ulmann, L., Morant-Manceau, A., Pasquet, V., Schoefs, B., et al. (2017). Modulation of lipid biosynthesis by stress in diatoms. Philos. Trans. R. Soc. B 372:20160407. doi: 10.1098/rstb.2016.0407
Selosse, M. A., Charpin, M., and Not, F. (2017). Mixotrophy everywhere on land and in water: the grand écart hypothesis. Ecol. Lett. 20, 246–263. doi: 10.1111/ele.12714
Sergeenko, T. V., Muradyan, E. A., Pronina, N. A., Klyachko-Gurich, G. L., Mishina, I. M., and Tsoglin, L. N. (2000). The effect of extremely high CO2 concentration on the growth and biochemical composition of microalgae. Russ. J. Plant Physiol. 47, 632–638.
Sforza, E., Simionato, D., Giacometti, G. M., Bertucco, A., and Morosinotto, T. (2012). Adjusted light and dark cycles can optimize photosynthetic efficiency in algae growing in photobioreactors. PLoS One 7:e38975. doi: 10.1371/journal.pone.0038975
Shemesh, Z., Leu, S., Khozin-Goldberg, I., Didi-Cohen, S., Zarka, A., and Boussiba, S. (2016). Inducible expression of Haematococcus oil globule protein in the diatom Phaeodactylum tricornutum: association with lipid droplets and enhancement of TAG accumulation under nitrogen starvation. Algal Res. 18, 321–331. doi: 10.1016/j.algal.2016.07.002
Shih, P. M. (2018). Towards a sustainable bio-based economy: redirecting primary metabolism to new products with plant synthetic biology. Plant Sci. 273, 84–91. doi: 10.1016/j.plantsci.2018.03.012
Shih, P. M., Zarzycki, J., Niyogi, K. K., and Kerfeld, C. A. (2014). Introduction of a synthetic CO2-fixing photorespiratory bypass into a cyanobacterium. J. Biol. Chem. 289, 9493–9500. doi: 10.1074/jbc.C113.543132
Shin, S., Lim, J., Koh, H. G., Kim, E. K., Kang, N. K., Jeon, S., et al. (2016). CRISPR/Cas9-induced knockout and knock-in mutations in Chlamydomonas reinhardtii. Sci. Rep. 6:27810. doi: 10.1038/srep27810
Shin, W., Lee, B., Kang, N. K., Kim, Y., and Jeong, W. (2017). Complementation of a mutation in RP43 causing partial truncation of light-harvesting chlorophyll antenna in Chlorella vulgaris. Sci. Rep. 7:1929.
Shurin, J. B., Mandal, S., and Abbott, R. L. (2014). Trait diversity enhances yield in algal biofuel assemblages. J. Appl. Ecol. 51, 603–611. doi: 10.1111/1365-2664.12242
Simionato, D., Basso, S., Giacometti, G. M., and Morosinotto, T. (2013). Optimization of light use efficiency for biofuel production in algae. Biophys. Chem. 182, 71–78. doi: 10.1016/j.bpc.2013.06.017
Spicer, A., and Molnar, A. (2018). Gene editing of microalgae: scientific progress and regulatory challenges in Europe. Biology 7:21. doi: 10.3390/biology7010021
Spolaore, P., Joannis-Cassan, C., Duran, E., and Isambert, A. (2006). Commercial applications of microalgae. J. Biosci. Bioeng. 101, 87–96. doi: 10.1263/jbb.101.87
Stephenson, A. I., Dennis, J. S., Howe, C. J., Scott, S. A., and Smith, A. G. (2010). Influence of nitrogen-limitation regime on the production by Chlorella vulgaris of lipids for biodiesel feedstocks. Biofuels 1, 47–58.
Sterner, R. W., and Elser, J. J. (2002). Ecological Stoichiometry: The Biology of Elements from Molecules to the Biosphere. Princeton, NJ: Princeton University.
Stockenreiter, M., Graber, A., Haupt, F., and Stibor, H. (2012). The effect of species diversity on lipid production by micro-algal communities. J. Appl. Phycol. 24, 45–54. doi: 10.1007/s10811-010-9644-1
Stockenreiter, M., and Litchman, E. (2019). Nitrogen-fixer enhances lipid yields in algal polycultures. Algal Res. 44:101676. doi: 10.1016/j.algal.2019.101676
Sun, X.-M., Ren, L.-J., Zhao, Q.-Y., Ji, X.-J., and Huang, H. (2019). Enhancement of lipid accumulation in microalgae by metabolic engineering. Biochim. Biophys. Acta 1864, 552–566. doi: 10.1016/j.bbalip.2018.10.004
Tabita, F. R., Hanson, T. E., Satagopan, S., Witte, B. H., and Kreel, N. E. (2008). Phylogenetic and evolutionary relationships of Rubisco and the Rubisco-like proteins and the functional lessons provided by diverse molecular forms. Philos. Trans. R Soc. Lond. B Biol. Sci. 363, 2629–2640. doi: 10.1098/rstb.2008.0023
Tachibana, M., Allen, A. E., Kikutani, S., Endo, Y., Bowler, C., and Matsuda, Y. (2011). Localization of putative carbonic anhydrases in two marine diatoms, Phaeodactylum tricornutum and Thalassiosira pseudonana. Photosynth. Res. 109, 205–221. doi: 10.1007/s11120-011-9634-4
Taubert, A., Jakob, T., and Wilhelm, C. (2019). Glycolate from microalgae: an efficient carbon source for biotechnological applications. Plant Biotechnol. J. 1, 1538–1546. doi: 10.1111/pbi.13078
Taylor, S. H., and Long, S. P. (2017). Slow induction of photosynthesis on shade to sun transitions in wheat may cost at least 21% of productivity. Philos. Trans. R. Soc. B Biol. Sci. 372:20160543. doi: 10.1098/rstb.2016.0543
Taylor, T. C., Backlund, A., Bjorhall, K., Spreitzer, R. J., and Andersson, I. (2001). First crystal structure of Rubisco from a green alga, Chlamydomonas reinhardtii. J. Biol. Chem. 276, 48159–48164. doi: 10.1074/jbc.M107765200
Tcherkez, G. (2013). Modelling the reaction mechanism of ribulose-1, 5- bisphosphate carboxylase/oxygenase and consequences for kinetics parameters. Plant Cell Environ. 36, 1586–1596. doi: 10.1111/pce.12066
Tcherkez, G. (2016). The mechanism of Rubisco-catalysed oxygenation. Plant Cell Environ. 39, 983–997. doi: 10.1111/pce.12629
Tcherkez, G. G. B., Farquhar, G. D., and Andrews, T. J. (2006). Despite slow catalysis and confused substrate specificity, all ribulose bisphosphate carboxylases may be nearly perfectly optimized. Proc. Natl. Acad. Sci. U.S.A. 103, 7246–7251. doi: 10.1073/pnas.0600605103
Till, P., Toepel, J., Buhler, B., Mach, R. L., and Mach-Aigner, A. R. (2020). Regulatory systems for gene expression control in cyanobacteria. Appl. Microbiol. Biotechnol. 104, 1977–1991. doi: 10.1007/s00253-019-10344-w
Toyoshima, M., Toya, Y., and Shimizu, H. (2020). Flux balance analysis of cyanobacteria reveals selective use of photosynthetic electron transport components under different spectral light conditions. Photosynth. Res. 143, 31–43. doi: 10.1007/s11120-019-00678-x
Travis, T. (1993). The Haber-Bosch process—exemplar of 20th-century chemical industry. Chem. Ind. 15, 581–585.
Tredici, M. R., Rodolfi, L., Biondi, N., Bassi, N., and Sampietro, G. (2016). Techno-economic analysis of microalgal biomass production in a 1-ha Green Wall Panel (GWP®) plant. Algal Res. 19, 253–263.
Vavitsas, K., Fabris, M., and Vickers, C. E. (2018). Terpenoid metabolic engineering in photosynthetic microorganisms. Genes 9:520. doi: 10.3390/genes9110520
Venuleo, M., Prásil, O., and Giordano, M. (2018). Life at elevated CO2 modifies the cell composition of Chromera velia (Chromerida). Eur. J. Phycol. 53, 58–66. doi: 10.1080/09670262.2017.1376255
Verruto, J., Francis, K., Wang, Y., Low, M. C., Greiner, J., Tacke, S., et al. (2018). Unrestrained markerless trait stacking in Nannochloropsis gaditana through combined genome editing and marker recycling technologies. Proc. Natl. Acad. Sci. U.S.A. 115, 7015–7022. doi: 10.1073/pnas.1718193115
Villanova, V., Fortunato, A. E., Singh, D., Dal, Bo, D., Conte, M., et al. (2017). Investigating mixotrophic metabolism in the model diatom Phaeodactylum tricornutum. Philos. Trans. R. Soc. B 372:20160404. doi: 10.1098/rstb.2016.0404
Wagner, H., Jakob, T., Fanesi, A., and Wilhelm, C. (2017). Towards an understanding of the molecular regulation of carbon allocation in diatoms: the interaction of energy and carbon allocation. Philos. Trans. R. Soc. Lond. B. Biol. Sci. 372:20160410. doi: 10.1098/rstb.2016.0410
Wagner, H., Jakob, T., and Wilhelm, C. (2006). Balancing the energy flow from captured light to biomass under fluctuating light conditions. New Phytol. 169, 95–108. doi: 10.1111/j.1469-8137.2005.01550.x
Wang, C., Thygesen, A., Liu, Y., Li, Q., Yang, M., Dang, D., et al. (2013). Bio-oil based biorefinery strategy for the production of succinic acid. Biotechnol. Biofuels 6:74. doi: 10.1186/1754-6834-6-74
Wichmann, J., Baier, T., Wentnagel, E., Lauersen, K. J., and Kruse, O. (2018). Tailored carbon partitioning for phototrophic production of (E)-α-bisabolene from the green microalga Chlamydomonas reinhardtii. Metab. Eng. 45, 211–222. doi: 10.1016/j.ymben.2017.12.010
Wichmann, J., Lauersen, K. J., and Kruse, O. (2020). Green algal hydrocarbon metabolism is an exceptional source of sustainable chemicals. Curr. Opin. Biotechnol. 61, 28–37. doi: 10.1016/j.copbio.2019.09.019
Wijffels, R. H., Kruse, O., and Hellingwerf, K. J. (2013). Potential of industrial biotechnology with cyanobacteria and eukaryotic microalgae. Curr. Opin. Biotechnol. 24, 405–413. doi: 10.1016/j.copbio.2013.04.004
Wilhelm, C., Jungandreas, A., Jakob, T., and Goss, R. (2014). Light acclimation in diatoms: from phenomenology to mechanisms. Mar. Genomics 16, 5–15. doi: 10.1016/j.margen.2013.12.003
Wilhelm, C., and Selmar, D. (2011). Energy dissipation is an essential mechanism to sustain the viability of plants: the physiological limits of improved photosynthesis. J. Plant Physiol. 168, 79–87. doi: 10.1016/j.jplph.2010.07.012
Williams, P. J. B., and Laurens, L. M. L. (2010). Microalgae as biodiesel and biomass feedstocks: review and analyses of the biochemistry, energetics and economics. Energy Environ. Sci. 3, 554–590.
Yang, B., Liu, J., Ma, X., Guo, B., Liu, B., Wu, T., et al. (2017). Genetic engineering of the Calvin cycle toward enhanced photosynthetic – CO2 fixation in microalgae. Biotechnol. Biofuels 10:229.
Young, J. N., Goldman, J. A., Kranz, S. A., Tortell, P. D., and Morel, F. M. (2015). Slow carboxylation of Rubisco constrains the rate of carbon fixation during Antarctic phytoplankton blooms. New Phytol. 205, 172–181. doi: 10.1111/nph.13021
Young, J. N., Heureux, A. M., Sharwood, R. E., Rickaby, R. E., Morel, F. M., and Whitney, S. M. (2016). Large variation in the Rubisco kinetics of diatoms reveals diversity among their carbon-concentrating mechanisms. J. Exp. Bot. 67, 3445–3456. doi: 10.1093/jxb/erw163
Young, J. N., and Hopkinson, B. M. (2017). The potential for co-evolution of CO2-concentrating mechanisms and Rubisco in diatoms. J. Exp. Bot. 68, 3751–3762. doi: 10.1093/jxb/erx130
Yunus, I. S., Wichmann, J., Wordenweber, R., Lauersen, K. J., Kruse, O., and Jones, P. R. (2018). Synthetic metabolic pathways for photobiological conversion of CO2 into hydrocarbon fuel. Metabolic Eng. 49, 201–211. doi: 10.1016/j.ymben.2018.08.008
Zhu, X., Long, S. P., and Ort, D. R. (2008). What is the maximum efficiency with which photosynthesis can convert solar energy into biomass? Curr. Opin. Biotechnol. 19, 153–159. doi: 10.1016/j.copbio.2008.02.004
Zhu, X., Wang, Y. U., Ort, D. R., and Long, S. P. (2013). e-photosynthesis: a comprehensive dynamic mechanistic model of C3 photosynthesis: from light capture to. Plant Cell Environ. 36, 1711–1727. doi: 10.1111/pce.12025
Keywords: microalgae, photosynthesis, Rubisco, C allocation, lipid metabolism, polycultures, metabolic engineering, cell factory
Citation: Gerotto C, Norici A and Giordano M (2020) Toward Enhanced Fixation of CO2 in Aquatic Biomass: Focus on Microalgae. Front. Energy Res. 8:213. doi: 10.3389/fenrg.2020.00213
Received: 10 April 2020; Accepted: 05 August 2020;
Published: 16 September 2020.
Edited by:
Michele Aresta, IC2R Ltd., ItalyReviewed by:
Wei Liu, Molecule Works Inc., United StatesJames Landon, University of Kentucky, United States
Copyright © 2020 Gerotto, Norici and Giordano. This is an open-access article distributed under the terms of the Creative Commons Attribution License (CC BY). The use, distribution or reproduction in other forums is permitted, provided the original author(s) and the copyright owner(s) are credited and that the original publication in this journal is cited, in accordance with accepted academic practice. No use, distribution or reproduction is permitted which does not comply with these terms.
*Correspondence: Alessandra Norici, YS5ub3JpY2lAdW5pdnBtLml0
†Deceased