- 1National Joint Engineering Research Center for Abrasion Control and Molding of Metal Materials, School of Materials Science and Engineering, Henan University of Science and Technology, Luoyang, China
- 2School of Food and Drug, Luoyang Normal University, Luoyang, China
- 3Provincial and Ministerial Co-Construction of Collaborative Innovation Center for Non-Ferrous Metal New Materials and Advanced Processing Technology, Henan Key Laboratory of Non-Ferrous Materials Science & Processing Technology, Henan University of Science and Technology, Luoyang, China
In recent years, aqueous rechargeable zinc-ion batteries (AZIBs) have attracted more and more attention not only because they use earth-abundant metals but also due to their improved safety and high volumetric energy density. As one type of promising electrode material for AZIBs, manganese-based materials are receiving considerable attention owing to their high theoretical specific capacity, non-toxicity, and low cost. This mini review summarizes the recent advances on the application of manganese-based materials (manganese oxide, manganate, and their composites) in AZIBs. In addition, the methods to enhance their zinc-ion storage properties are summarized and discussed, such as morphology engineering, doping, as well as compositing with other materials. Ultimately, some personal prospects for future research of the manganese-based materials for AZIBs are also proposed.
Introduction
In recent years, overconsumption of fossil fuels has caused many problems, such as global warming and environmental deterioration, which have been paid more and more attention by people all over the world (Liu et al., 2016, 2019c,d; He et al., 2017; Ma et al., 2017; Chen et al., 2019a; Guo et al., 2019c; Pan et al., 2020). Renewable energies like solar and wind energy are regarded as clean and sustainable ways to solve these issues. However, the intermittent nature of solar and wind energies greatly hinder their further development (Wang et al., 2019c; Xiao et al., 2019; Yuan et al., 2019; Zhao X. et al., 2019; Cheng et al., 2020; Ma J. Q. et al., 2020). Fortunately, electrochemical energy storage (EES) devices are regarded as a promising solution which could store electric energy obtained from renewable energies (Ding et al., 2018; Chen et al., 2019b; Li Y. et al., 2019; Li J. et al., 2020; Li Y. et al., 2020; Zhang et al., 2019; Ma J. Y. et al., 2020; Wang R. et al., 2020; Wang W. et al., 2020). Among various EES devices, lithium-ion batteries have been intensively investigated and used in many applications such as portable electronics, electrical vehicles, and smart grids due to their high energy density, long cycle life, and environmental benignity (Wang F. et al., 2018; Wang F. et al., 2020; Wang G. et al., 2020; Hao et al., 2019; Liu et al., 2019f; Wu et al., 2019; Zhao Q. et al., 2019; Gao G. J. et al., 2020; Song et al., 2020; Zou et al., 2020). Nevertheless, the increasing concerns about limited lithium resources, high cost, and safety issue strongly limit their further development for large-scale applications (Liu et al., 2018; Ma X. D. et al., 2019; Wang et al., 2019d; Yixuan et al., 2020; Yu et al., 2020; Zhang et al., 2020). In addition, the other alkali metals, Na and K, are extremely reactive and the introduced organic electrolytes are flammable, which bring great security risks for sodium-ion and potassium-ion batteries (Song et al., 2018; Ding et al., 2019; Hua et al., 2019). Therefore, it is urgent to explore alternative battery systems with low cost, high safety, and long cycle life.
Aqueous rechargeable zinc-ion batteries (AZIBs), in particular, comprise of a zinc metal anode, the aqueous electrolyte in majority, and a cathode for accommodation of Zn ions. Significantly, it differs from the traditional alkaline Zn battery (such as Zn-Mn or Ni-Zn battery) which is based on dissolution/precipitation reactions at the Zn anode and H+ intercalation/extraction reactions at the cathode. Also, it is also distinguished from other batteries with Zn anode but no intercalation of Zn ions in cathode reactions (Kordesh and Weissenbacher, 1994; Song et al., 2018). Nowadays, AZIBs have attracted more and more attention not only because they use earth-abundant metals but also due to their improved safety and high volumetric energy density (Chen et al., 2017; Song et al., 2018). As a typical type of cathode materials for AZIBs, manganese-based materials are receiving considerable attention in recent years owing to their high theoretical specific capacity, non-toxicity, and low cost (Figures 1A,B). For instance, Wu et al. (2018) recently reported an article about α-MnO2 coated with graphene as a high-performance cathode material for AZIBs. By a simple hydrothermal method, Zhu et al. (2018) fabricated a flower-like Mn3O4 and used it as a cathode material of AZIBs. And Wan and Niu (2019) reviewed the design strategies of vanadium-based materials for AZIBs. However, there is still a lack of review to exclusively cover the state-of-the-art developments of manganese-based materials for AZIBs.
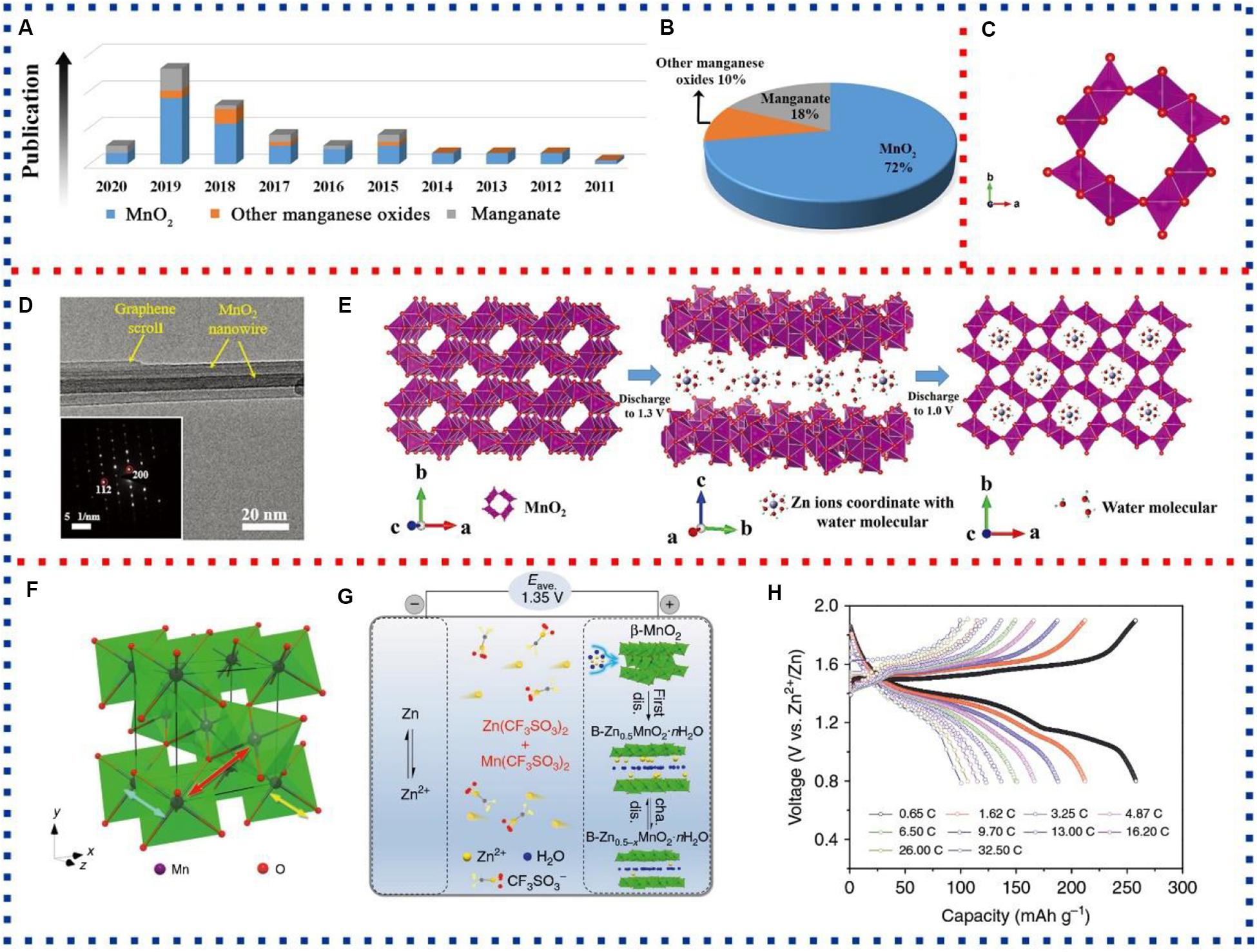
Figure 1. (A) The statistical table of manganese-based materials reported in aqueous rechargeable zinc-ion batteries (AZIBs). (B) The percentage of different manganese-based materials reported in AZIBs. (C) Schematic diagram of α-MnO2 crystal structure. (D) Transmission electron microscopy (TEM) and corresponding selected area electron diffraction (SAED) of α-MnO2/graphene scrolls (MGS). (E) Schematic diagram of zinc ions inserting into the MGS electrode in two steps. (F) Schematic diagram of β-MnO2 crystal structure. (G) Schematic diagram of the Zn/β-MnO2 batteries with β-MnO2 as cathode, Zn(CF3SO3)2 add Mn(CF3SO3)2 as electrolyte. (H) Discharge/charge profiles of Zn/β-MnO2 batteries. (C) Reprinted with permission (Gao X. et al., 2020). Copyright 2020, WILEY-VCH. (D,E) Reprinted with permission (Wu et al., 2018). Copyright 2018, WILEY-VCH. (F–H) Reprinted with permission (Zhang et al., 2017). Copyright 2017, Springer Nature.
Herein, we will give an overview on manganese-based materials, mainly including manganese oxide, manganate, as well as their composite materials, and their application in the field of electrode materials for AZIBs. Their micro/nanostructures and electrochemical properties are systematically summarized. Furthermore, some reasonable suggestions to promote future breakthroughs were also presented.
Manganese-Based Materials for Aqueous Rechargeable Zinc-Ion Batteries
The electrochemical performances of manganese-based materials, such as manganese oxide, manganate, and their composites, as cathode materials for AZIBs are summarized in Table 1. The zinc-ion storage properties of manganese-based materials combined with carbon-based materials are significantly superior than those of pure manganese-based materials.
Manganese Oxide
Since manganese has a variety of valence states, it could form a series of manganese oxides, such as MnO2 (Alfaruqi et al., 2016), Mn3O4 (Zhu et al., 2018), etc. Due to their special structure, they could be used as cathode materials for AZIBs (Khamsanga et al., 2019; Palaniyandy et al., 2019).
Manganese Dioxide
Manganese dioxide has a variety of crystal structures, such as α-MnO2 (Wu et al., 2018), β-MnO2 (Zhang et al., 2017), γ-MnO2 (Alfaruqi et al., 2015c), δ-MnO2 (Khamsanga et al., 2019), etc., which are composed of the basic structural unit MnO6 octahedral into chain/tunnel/layered structure by sharing angle/edge and have been well applied in AZIBs (Song et al., 2018; Tang et al., 2019). However, the low electronic conductivity of MnO2 and large volume change of electrode during Zn2+ insertion/extraction process hinder the further development of MnO2 as the cathode material for AZIBs (Tang et al., 2019). At present, many researchers are devoted to improve the electronic conductivity of MnO2 and the cycling stability of MnO2-based AZIBs (Wu et al., 2018; Tang et al., 2019).
α-MnO2, which have stable 2 × 2 tunnel structure, has received considerable attention in recent years as a cathode material for AZIBs (Figure 1C; Palaniyandy et al., 2019; Gao X. et al., 2020). In 2009, α-MnO2 was first applied as a cathode material for AZIBs, and specific capacity reached 210 mAh g–1 at 0.2 A g–1 (Xu et al., 2009). Pan et al. (2016) added MnSO4 into ZnSO4 electrolyte to suppress the dissolution of Mn2+ and stabilize the electrode. During the discharging process, Zn2+ and H+ co-inserted into α-MnO2, generating ZnSO4[Zn(OH)2]3.xH2O and MnOOH, from which Zn2+ and H+ could reversibly extract in the charging process. And these Zn/α-MnO2 batteries show excellent rate capability and high capacity retention of 92% after 5,000 cycles. To further enhance the zinc-ion storage properties of α-MnO2, Wu et al. (2018) fabricated α-MnO2 nanowires coated with graphene (MGS) by hydrothermal method (Figure 1D). The graphene not only increases the electronic conductivity of the material but also effectively inhibits the erosion of the electrolyte to electrode. During discharge and charging, the insertion of Zn2+ takes place in two steps, First, Zn2+ inserted into water layers and formed zinc-buserite, then continued to be inserted into 2 × 2 tunnels of α-MnO2 (Figure 1E). These detailed studies provide a good theoretical basis for α-MnO2 in AZIBs.
Compared with other manganese dioxide phases, β-MnO2 has the narrowest tunnel and minimal channel. The 1 × 1 tunnel (2.3 × 2.3 Å) of the β-MnO2 hardly incorporates Zn2+ inserting (Figure 1F; Devaraj and Munichandraiah, 2008; Islam et al., 2017a; Zhang et al., 2017). However, β-MnO2 has high thermodynamic stability. Therefore, it has also been reported as a cathode material for AZIBs. For instance, Islam et al. (2017a) synthesized β-MnO2 nanorods exposed to (101) plane by a microwave-assisted hydrothermal method and used them as cathode materials for AZIBs. By a combination of in situ synchrotron, ex situ X-ray diffraction (XRD), and other techniques, the Zn-ion storage mechanism has revealed that the reaction mechanism of the β-MnO2 nanorod electrode in the Zn cell proceeded via a combination of solid solution and conversion reactions, in which Zn ion intercalated into the β-MnO2 framework, followed by the formation of Zn-inserted phases along with the precipitation of ZnSO4⋅3Zn(OH)2.5H2O on the electrode surface. And the electrode shows a high discharge capacity of 270 mA h g–1 at 0.1 A g–1. Similarly, Zhang et al. (2017) synthesized β-MnO2 nanorods by a hydrothermal method and took 3 M Zn(CF3SO3)2 with 0.1 M Mn(CF3SO3)2 as electrolyte. As shown in Figure 1G, during the initial discharge, a phase transformed from tunneled structure to layered structure (Zn-buserite) and followed by reversible Zn2+ (de)intercalation in the H2O-containing Zn-buserite framework. Mn2+ dissolution can be effectively inhibited by the addition of Mn(CF3SO3)2 in which the amorphous MnOx in situ generated on the electrode surface. The electrode showed excellent rate performance, which maintained 100 mAh g–1, even the current density reached 32.5 C (Figure 1H).
Comprised of randomly arranged 1 × 1 (size ∼ 2.3 × 2.3 Å, pyrolusite) and 1 × 2 (size ∼2.3 × 4.6 Å, ramsdellite) tunnels, γ-MnO2 is suitable for Zn2+ intercalation/deintercalation. In 2003, Kumar and Sampath (2003) first applied γ-MnO2 as the positive electrode for AZIBs. Subsequently, various γ-MnO2 were reported and used as cathode materials for AZIBs. For example, Alfaruqi et al. (2015c) prepared tunnel mesoporous γ-MnO2 by simple redox reaction. As shown in Supplementary Figure S1C, during the discharge process, the tunnel-type γ-MnO2 transformed to three phases (ZnMn2O4, γ-ZnxMnO2, and L-ZnyMnO2), which was accompanied that Mn4+ was reduced to Mn3+ and Mn2+, respectively.
δ-MnO2 is considered to be a favorable host for Zn2+ due to its unique layered structure (the interlayer spacing can reach up to 7.0 Å) (Corpuz et al., 2019; Guo et al., 2019b). Compared with α-MnO2, β-MnO2, and γ-MnO2, another advantage of δ-MnO2 is that there is no phase transition during Zn2+ insertion or extraction, which could prevent the capacity fading effectively. However, unstable structure during the cycling limits its further application. At present, refine grain (Han et al., 2017; Guo et al., 2019a), synthetized special morphology (Alfaruqi et al., 2015a; Guo et al., 2018), and add structural stabilizer (Kao-ian et al., 2019) are common ways to improve the performance of δ-MnO2. For instance, Guo et al. (2019a) synthesized δ-MnO2 nanosheets with a thickness of 2–4 nm by reduction of KMnO4. During the initial discharge process, H+ inserted into the δ-MnO2, followed by co-insertion of Zn2+ and H+. Ultrathin nanosheets shortened the diffusion path of Zn2+ and H+ and improved the ion diffusion coefficient. The Zn/δ-MnO2 cells still showed 86 mAh g–1 at 0.5 A g–1, which show excellent cycle stability.
ε-MnO2 with face-shared MnO6 and YO6 octahedra (Y denotes vacancy) structure was also reported in AZIBs (Sun et al., 2017; Song et al., 2018). For instance, Sun et al. (2017) deposited MnO2 on the carbon fiber paper with no binder is required (Supplementary Figure S1E). They first reported that during the discharge process, H+ and Zn2+ inserting corresponded to the generating of MnOOH and ZnMn2O4, respectively, in AZIBs.
Other manganese dioxides, such as λ-MnO2 and todorokite-type MnO2, have also been reported in AZIBs (Lee et al., 2013; Yuan et al., 2014). In 2014, Yuan et al. (2014) removed Li from LiMn2O4 with H2SO4 and synthesized λ-MnO2. The Zn/λ-MnO2 cell displayed 442.6 mAh g–1 at 13.8 mA g–1. Lee et al. (2013) turned Na-birnessite into Mg-containing solution and synthesized Mg1.8Mn6O12.4.8H2O with todorokite-type structure. The cell could deliver specific capacity of 108 mAh g–1 at 0.5 C (1 C = 255 mA g–1).
Other Manganese Oxides
In addition to manganese dioxide, other manganese oxides such as Mn3O4 (Hao et al., 2018; Zhu et al., 2018), Mn2O3 (Jiang et al., 2017), and others (Fu et al., 2018) are also widely studied in AZIBs.
Mn2O3 is an alternative cathode material for AZIBs. In 2017, Jiang et al. (2017) first reported α-Mn2O3 as the cathode material of AZIBs. The α-Mn2O3 has a bixbyite structure. In this structure, the Mn3+ ions are in octahedral coordination and each oxygen ion is surrounded by four Mn ions. During the discharge process, the Mn3+ of Mn2O3 was reduced to Mn2+, accompanied the generation of ZnxMn2O3 without other phases generated. Based on this property, after 2,000 cycles, the α-Mn2O3 displays a reversible discharge capacity of 38 mAh g–1 at 2 A g–1.
Mn3O4 has a mixed valence state of +2 and +3, in which Mn2+ occupies the tetrahedral gap and Mn3+ occupies the octahedral gap. Inspired by multiple Mn valences may contribute to the diffusivity and migration of Zn2+ (Zhang et al., 2016; Ma J. L. et al., 2020). Zhu et al. (2018) synthesized a flower-like Mn3O4 on stainless steel welded mesh (SSWM@Mn3O4) via a one-step hydrothermal method (Supplementary Figures S1A,B). They used ex situ XRD and ex situ scanning electron microscope to reveal the structure and morphology evolution of SSWM@Mn3O4 electrode. During the discharge process, Mn3+ is reduced to Mn2+ and exists in the form of MnO after discharge. The ZnSO4 and H2O in the aqueous electrolyte react with the sequent OH– ions to form large flake-like ZnSO4[Zn(OH)2]3.5H2O. Due to the intimate contact between the material and current collector, the SSWM@Mn3O4 electrode shows great stability with higher than 100 mAh g–1 capacity at 0.1 A g–1 after 500 cycles.
In addition to the manganese oxides described above, some non-stoichiometric manganese oxides were also reported in AZIBs. For instance, Fu et al. (2018) synthesized N-doped carbon porous MnOx nanorods (MnOx@N-C) by using metal organic framework (MOF) materials. Amorphous carbon and onion-like nitrogen-doped carbon on the surface formed an electronic conductive network, which not only improves the electron conductivity but also alleviates the volume change caused by the insertion or extraction of Zn2+. Benefiting from the special structure, the electrode still could deliver 305 mAh g–1 after 600 cycles at 0.5 A g–1.
Manganate
Manganate is an important component of manganese-based materials. Some manganates are investigated as cathode materials for AZIBs, such as LiMn2O4 (Wu et al., 2015), ZnMn2O4 (Zhang et al., 2016; Wu et al., 2017; Chen et al., 2019c), KMn8O16 (Cui et al., 2018), and their derivatives (Tao et al., 2020).
Benefiting from the low cost, non-toxicity, easy fabrication, and high safety, LiMn2O4 has been well studied as a positive electrode material for lithium-ion batteries. Recently, this material has been also reported in AZIBs. For instance, Wu et al. (2015) investigated the effect of thiourea as the electrolyte additive on the electrochemical performance of AZIBs, using LiMnO4 as a cathode material. Similarly, LiMnO4 was also applied as a cathode material for AZIBs by Hoang et al. (2017) to study the suppression of zinc dendrite growth, in which pyrazole was used as an additive for the electrolyte.
Zhang et al. (2016) fabricated non-stoichiometric ZnMn2O4/carbon composite and applied it as a cathode material for AZIBs. They found the unoccupied octahedral sites (8c) of the ZnMn2O4 have cation vacancies, which can provide additional pathways for Zn2+ migration (Supplementary Figure S1F). During the discharge process, Zn2+ inserted to the Zn-O tetrahedron sites, while the charge process is opposite (Supplementary Figure S1D). Benefiting from the oxygen vacancy caused by cationic vacancies, the ZnMn2O4/carbon electrode shows remarkable performance of 150 mAh g–1 at 50 mA g–1 and an unprecedented capacity retention of 94% after 500 cycles at 0.5 A g–1 (Supplementary Figure S1G). Later, Cui et al. (2018) synthesized KMn8O16 microspheres by the hydrothermal method. During the initial charge process, partial K+ extracted from KMn8O16. And in the following charge/discharge cycles, Zn2+ inserted or extracted from the electrode reversibly. The Zn/KMn8O16 cell delivered 77.0 mAh g–1 after 100 cycles and exhibited low self-discharge, indicating stable electrochemical performance.
Conclusion and Outlook
In summary, AZIBs have attracted considerable attention in recent years mainly due to their high safety, low cost, and high volumetric energy density. This mini review basically summarized the application of manganese-based materials, including manganese oxide, manganate, and their composite materials in AZIBs. Although tunnel or layered structures of manganese-based materials provide a path for Zn2+ insertion/extraction, the poor ionic and electrical conductivity restricts their further application. In this regard, it is particularly important to shorten the transport path of zinc ions and composite with materials with a high electronic conductivity. Hence, some aspects could be focused on to further improve the electrochemical performances of manganese-based AZIBs. And also some problems still exist and need to be resolved for their further development.
(1) MnO2 with different structures such as α, β, γ, δ, etc., has been well investigated as a cathode material for AZIBs. The mechanism of insertion or extraction properties of Zn2+ and their phase transformation during cycling are not clear enough and still need to be explored. In operando techniques, such as in situ X-ray technique, in situ electron microscopy, and in situ scanning probe technology, as well as synchron X-ray-based techniques, are very helpful to obtain time-dependent information. So, more research using in operando techniques needs to be conducted to get insight and understanding of the zinc-ion storage mechanism of manganese-based materials for AZIBs.
(2) Poor conductivity of MnO2 and volume change caused by phase transformation often lead to poor rate and cycling performances of the Zn/MnO2 batteries. Special MnO2 morphologies such as nanospheres, core-shell structures, or nanowires growing on a conductive substrate such as carbon cloth/paper or stainless steel mesh will effectively solve these issues. Therefore, this research direction may deserve further study.
(3) Other manganese oxides (mainly includes Mn3O4, Mn2O3, and MnOx) and manganate (such as LiMn2O4, ZnMn2O4, and KMn8O16) have also been reported for AZIBs, but compared to MnO2, more work on related materials as well as their zinc-ion storage mechanism should be further explored.
We hope this review is helpful and can inspire more innovative ideas for manganese-based or even transition metal-oxide materials as cathode materials for AZIBs.
Author Contributions
WZ, YL, and SW conceived the idea. YL and XZ wrote the draft. All authors contributed to the writing, discussion, and revision of the final version of the manuscript.
Funding
This work was supported by the Program for Changjiang Scholars and Innovative Research Team in University (IRT_16R21), the Chinese 02 Special Fund (2017ZX02408003), Open Fund of National Joint Engineering Research Center for Abrasion Control and Molding of Metal Materials (HKDNM201807), the Key Science and Technology Program of Henan Province (Grant No. 172102310551), Science Foundation for Youths of Henan University of Science and Technology (2013QN006), the Student Research Training Plan of Henan University of Science and Technology (2019031), and the National Undergraduate Innovation Entrepreneurship Training Program (202010464031).
Conflict of Interest
The authors declare that the research was conducted in the absence of any commercial or financial relationships that could be construed as a potential conflict of interest.
Supplementary Material
The Supplementary Material for this article can be found online at: https://www.frontiersin.org/articles/10.3389/fenrg.2020.00195/full#supplementary-material
References
Alfaruqi, M. H., Gim, J., Kim, S., Song, J., Duong Tung, P., Jo, J., et al. (2015a). A layered delta-MnO2 nanoflake cathode with high zinc-storage capacities for eco-friendly battery applications. Electrochem. Commun. 60, 121–125. doi: 10.1016/j.elecom.2015.08.019
Alfaruqi, M. H., Gim, J., Kim, S., Song, J., Jo, J., Kim, S., et al. (2015b). Enhanced reversible divalent zinc storage in a structurally stable alpha-MnO2 nanorod electrode. J. Power Sources 288, 320–327. doi: 10.1016/j.jpowsour.2015.04.140
Alfaruqi, M. H., Islam, S., Gim, J., Song, J., Kim, S., Duong Tung, P., et al. (2016). A high surface area tunnel-type alpha-MnO2 nanorod cathode by a simple solvent-free synthesis for rechargeable aqueous zinc-ion batteries. Chem. Phys. Lett. 650, 64–68. doi: 10.1016/j.cplett.2016.02.067
Alfaruqi, M. H., Islam, S., Mathew, V., Song, J., Kim, S., Duong Pham, T., et al. (2017). Ambient redox synthesis of vanadium-doped manganese dioxide nanoparticles and their enhanced zinc storage properties. Appl. Surf. Sci. 404, 435–442. doi: 10.1016/j.apsusc.2017.02.009
Alfaruqi, M. H., Islam, S., Putro, D. Y., Mathew, V., Kim, S., Jo, J., et al. (2018). Structural transformation and electrochemical study of layered MnO2 in rechargeable aqueous zinc-ion battery. Electrochim. Acta 276, 1–11. doi: 10.1016/j.electacta.2018.04.139
Alfaruqi, M. H., Mathew, V., Gim, J., Kim, S., Song, J., Baboo, J. P., et al. (2015c). Electrochemically induced structural transformation in a gamma-MnO2 cathode of a high capacity zinc-ion battery system. Chem. Mater. 27, 3609–3620. doi: 10.1021/cm504717p
Chen, H. P., Liu, J. Q., Liu, P., Wang, Y. J., Xiao, H. Y., Yang, Q. F., et al. (2019a). Carbon-confined magnesium hydride nano-lamellae for catalytic hydrogenation of carbon dioxide to lower olefins. J. Catal. 379, 121–128. doi: 10.1016/j.jcat.2019.09.022
Chen, L., Chen, L., Zhai, W., Li, D., Lin, Y., Guo, S., et al. (2019b). Tunable synthesis of LixMnO2 nanowires for aqueous Li-ion hybrid supercapacitor with high rate capability and ultra-long cycle life. J. Power Sources 413, 302–309. doi: 10.1016/j.jpowsour.2018.12.026
Chen, L., Yang, Z., Qin, H., Zeng, X., and Meng, J. (2019c). Advanced electrochemical performance of ZnMn2O4/N-doped graphene hybrid as cathode material for zinc ion battery. J. Power Sources 425, 162–169. doi: 10.1016/j.jpowsour.2019.04.010
Chen, L.-N., Yan, M.-Y., Mei, Z.-W., and Mai, L.-Q. (2017). Research progress and prospect of aqueous zinc ion battery. J. Inorg. Mater. 32, 225–234. doi: 10.15541/jim20160192
Cheng, Y., Xiao, X., Pan, K. M., and Pang, H. (2020). Development and application of self-healing materials in smart batteries and supercapacitors. Chem. Eng. J. 380:122565. doi: 10.1016/j.cej.2019.122565
Corpuz, R. D., De Juan, L. M. Z., Praserthdam, S., Pomprasertsuk, R., Yonezawa, T., Mai Thanh, N., et al. (2019). Annealing induced a well-ordered single crystal delta-MnO2 and its electrochemical performance in zinc-ion battery. Sci. Rep. 9:15107. doi: 10.1038/s41598-019-51692-x
Cui, J., Wu, X., Yang, S., Li, C., Tang, F., Chen, J., et al. (2018). Cryptomelane-type KMn8O16 as potential cathode material - for aqueous zinc ion battery. Front. Chem. 6:352. doi: 10.3389/fchem.2018.00352
Deng, Z., Huang, J., Liu, J., Ren, L., Zhu, L., Xiao, X., et al. (2019). beta-MnO2 nanolayer coated on carbon cloth as a high-activity aqueous zinc-ion battery cathode with high-capacity and long-cycle-life. Mater. Lett. 248, 207–210. doi: 10.1016/j.matlet.2019.04.036
Devaraj, S., and Munichandraiah, N. (2008). Effect of crystallographic structure of MnO2 on its electrochemical capacitance properties. J. Phys. Chem. C 112, 4406–4417. doi: 10.1021/jp7108785
Ding, H. B., Zhang, Q. F., Liu, Z. M., Wang, J., Ma, R. F., Fan, L., et al. (2018). TiO2 quantum dots decorated multi-walled carbon nanotubes as the multifunctional separator for highly stable lithium sulfur batteries. Electrochim. Acta 284, 314–320. doi: 10.1016/j.electacta.2018.07.167
Ding, J., Zhang, H., Zhou, H., Feng, J., Zheng, X., Zhong, C., et al. (2019). Sulfur-grafted hollow carbon spheres for potassium-ion battery anodes. Adv. Mater. 31:1900429. doi: 10.1002/adma.201900429
Fu, Y., Wei, Q., Zhang, G., Wang, X., Zhang, J., Hu, Y., et al. (2018). High-performance reversible aqueous Zn-ion battery based on porous MnOx nanorods coated by MOF-derived N-doped carbon. Adv. Energy Mater. 8:1801445. doi: 10.1002/aenm.201801445
Gao, G. J., Feng, W. J., Su, W. X., Wang, S. J., Chen, L. J., Li, M. M., et al. (2020). Preparation and modification of MIL-101(Cr) metal organic framework and its application in lithium-sulfur batteries. Int. J. Electrochem. Sci. 15, 1426–1436. doi: 10.20964/2020.02.26
Gao, X., Wu, H., Li, W., Tian, Y., Zhang, Y., Wu, H., et al. (2020). H+-insertion boosted alpha-MnO2 for an aqueous Zn-Ion battery. Small 16:1905842. doi: 10.1002/smll.201905842
Gou, L., Xue, D., Mou, K.-L., Zhao, S.-P., Wang, Y., Fan, X.-Y., et al. (2019). alpha-MnO2@In2O3 nanotubes as cathode material for aqueous rechargeable Zn-Ion battery with high electrochemical performance. J. Electrochem. Soc. 166, A3362–A3368. doi: 10.1149/2.0801914jes
Guo, C., Liu, H., Li, J., Hou, Z., Liang, J., Zhou, J., et al. (2019a). Ultrathin delta-MnO2 nanosheets as cathode for aqueous rechargeable zinc ion battery. Electrochim. Acta 304, 370–377. doi: 10.1016/j.electacta.2019.03.008
Guo, C., Zhou, Q., Liu, H., Tian, S., Chen, B., Zhao, J., et al. (2019b). A case study of beta-, and delta-MnO2 with different crystallographic forms on ion-storage in rechargeable aqueous zinc ion battery. Electrochim. Acta 324:134867. doi: 10.1016/j.electacta.2019.134867
Guo, X., Li, J., Jin, X., Han, Y., Lin, Y., Lei, Z., et al. (2018). A hollow-structured manganese oxide cathode for stable Zn-MnO2 batteries. Nanomaterials 8:301. doi: 10.3390/nano8050301
Guo, X., Zhou, J., Bai, C., Li, X., Fang, G., and Liang, S. (2020). Zn/MnO2 battery chemistry with dissolution-deposition mechanism. Mater. Today Energy 16:100396. doi: 10.1016/j.mtener.2020.100396
Guo, X. T., Zhang, Y. Z., Zhang, F., Li, Q., Anjum, D. H., Liang, H. F., et al. (2019c). A novel strategy for the synthesis of highly stable ternary SiOx composites for Li-ion-battery anodes. J. Mater. Chem. A 7, 15969–15974. doi: 10.1039/c9ta04062e
Han, M., Huang, J., Liang, S., Shan, L., Xie, X., Yi, Z., et al. (2020). Oxygen defects in β-MnO2 enabling high-performance rechargeable aqueous zinc/manganese dioxide battery. iScience 23:100797. doi: 10.1016/j.isci.2019.100797
Han, S.-D., Kim, S., Li, D., Petkov, V., Yoo, H. D., Phillips, P. J., et al. (2017). Mechanism of Zn insertion into nanostructured delta-MnO2: a nonaqueous rechargeable Zn metal battery. Chem. Mater. 29, 4874–4884. doi: 10.1021/acs.chemmater.7b00852
Hao, J., Mou, J., Zhang, J., Dong, L., Liu, W., Xu, C., et al. (2018). Electrochemically induced spinel-layered phase transition of Mn3O4 in high performance neutral aqueous rechargeable zinc battery. Electrochim. Acta 259, 170–178. doi: 10.1016/j.electacta.2017.10.166
Hao, X. G., Zhao, Q., Su, S. M., Zhang, S. Q., Ma, J. B., Shen, L., et al. (2019). Constructing multifunctional interphase between Li1.4Al0.4Ti1.6(PO4)3and Li Metal by magnetron sputtering for highly stable solid-state lithium metal batteries. Adv. Energy Mater. 9:1901604. doi: 10.1002/aenm.201901604
He, T. T., Wang, W., Chen, W., Chen, D. M., and Yang, K. (2017). Influence of In and Sn compositions on the reactivity of Al-Ga-In-Sn alloys with water. Int. J. Hydrogen Energy 42, 5627–5637. doi: 10.1016/j.ijhydene.2016.11.112
Hoang, T. K. A., The Nam Long, D., Cho, J. H., Su, J. Y. J., Lee, C., Lu, C., et al. (2017). Sustainable gel electrolyte containing pyrazole as corrosion inhibitor and dendrite suppressor for aqueous Zn/LiMn2O4 battery. ChemSusChem 10, 2816–2822. doi: 10.1002/cssc.201700441
Hua, Y., Li, X. X., Chen, C. Y., and Pang, H. (2019). Cobalt based metal-organic frameworks and their derivatives for electrochemical energy conversion and storage. Chem. Eng. J. 370, 37–59. doi: 10.1016/j.cej.2019.03.163
Huang, J., Wang, Z., Hou, M., Dong, X., Liu, Y., Wang, Y., et al. (2018). Polyaniline-intercalated manganese dioxide nanolayers as a high-performance cathode material for an aqueous zinc-ion battery. Nat. Commun. 9:2906. doi: 10.1038/s41467-018-04949-4
Islam, S., Alfaruqi, M. H., Mathew, V., Song, J., Kim, S., Kim, S., et al. (2017a). Facile synthesis and the exploration of the zinc storage mechanism of beta-MnO2 nanorods with exposed (101) planes as a novel cathode material for high performance eco-friendly zinc-ion batteries. J. Mater. Chem. A 5, 23299–23309. doi: 10.1039/c7ta07170a
Islam, S., Alfaruqi, M. H., Song, J., Kim, S., Duong Tung, P., Jo, J., et al. (2017b). Carbon-coated manganese dioxide nanoparticles and their enhanced electrochemical properties for zinc-ion battery applications. J. Energy Chem. 26, 815–819. doi: 10.1016/j.jechem.2017.04.002
Jiang, B., Xu, C., Wu, C., Dong, L., Li, J., and Kang, F. (2017). Manganese sesquioxide as cathode material for multivalent zinc ion battery with high capacity and long cycle life. Electrochim. Acta 229, 422–428. doi: 10.1016/j.electacta.2017.01.163
Kao-ian, W., Pornprasertsuk, R., Thamyongkit, P., Maiyalagan, T., and Kheawhom, S. (2019). Rechargeable zinc-ion battery based on choline chloride-urea deep eutectic solvent. J. Electrochem. Soc. 166, A1063–A1069. doi: 10.1149/2.0641906jes
Khamsanga, S., Pornprasertsuk, R., Yonezawa, T., Mohamad, A. A., and Kheawhom, S. (2019). delta-MnO2 nanoflower/graphite cathode for rechargeable aqueous zinc ion batteries. Sci. Rep. 9:8441. doi: 10.1038/s41598-019-44915-8
Kordesh, K., and Weissenbacher, M. (1994). Rechargeable alkaline manganese dioxide/zinc batteries. J. Power Sources 51, 61–78. doi: 10.1016/0378-7753(94)01955-X
Kumar, G. G., and Sampath, S. (2003). Electrochemical characterization of poly(vinylidenefluoride)-zinc triflate gel polymer electrolyte and its application in solid-state zinc batteries. Solid State Ion. 160, 289–300. doi: 10.1016/s0167-2738(03)00209-1
Lee, J., Ju, J. B., Cho, W. I., Cho, B. W., and Oh, S. H. (2013). Todorokite-type MnO2 as a zinc-ion intercalating material. Electrochim. Acta 112, 138–143. doi: 10.1016/j.electacta.2013.08.136
Lee, J.-W., Seo, S.-D., and Kim, D.-W. (2019). Comparative study on ternary spinel cathode Zn-Mn-O microspheres for aqueous rechargeable zinc-ion batteries. J. Alloys Compd. 800, 478–482. doi: 10.1016/j.jallcom.2019.06.051
Li, D.-S., Wu, S., Wang, Y.-F., Sun, M., Liu, W.-L., Ren, M.-M., et al. (2019). Manganese oxides/N-doped carbon particles with high capacity retention for aqueous rechargeable zinc battery. J. Nanopart. Res. 21:52. doi: 10.1007/s11051-019-4491-8
Li, J., Zhang, W., Zhang, X., Huo, L., Liang, J., Wu, L., et al. (2020). Copolymer derived micro/meso-porous carbon nanofibers with vacancy-type defects for high-performance supercapacitors. J. Mater. Chem. A 8, 2463–2471. doi: 10.1039/c9ta08850d
Li, Y., Xu, Y. X., Liu, Y., and Pang, H. (2019). Exposing {001} crystal plane on hexagonal Ni-MOF with surface-grown cross-linked mesh-structures for electrochemical energy storage. Small 15:1902463. doi: 10.1002/smll.201902463
Li, Y., Zhai, X., Liu, Y., Wei, H., Ma, J., Chen, M., et al. (2020). WO3-based materials as electrocatalysts for hydrogen evolution reaction. Front. Mater. 7:105. doi: 10.3389/fmats.2020.00105
Lian, S., Sun, C., Xu, W., Huo, W., Luo, Y., Zhao, K., et al. (2019). Built-in oriented electric field facilitating durable Zn-MnO2 battery. Nano Energy 62, 79–84. doi: 10.1016/j.nanoen.2019.04.038
Liu, F., Chen, Z., Fang, G., Wang, Z., Cai, Y., Tang, B., et al. (2019a). V2O5 nanospheres with mixed vanadium valences as high electrochemically active aqueous zinc-ion battery cathode. Nano Micro Lett. 11:25. doi: 10.1007/s40820-019-0256-2
Liu, G., Huang, H., Bi, R., Xiao, X., Ma, T., and Zhang, L. (2019b). K+ pre-intercalated manganese dioxide with enhanced Zn2+ diffusion for high rate and durable aqueous zinc-ion batteries. J. Mater. Chem. A 7, 20806–20812. doi: 10.1039/c9ta08049j
Liu, G. L., Cui, J., Luo, R. J., Liu, Y., Huang, X. X., Wu, N. T., et al. (2019c). 2D MoS2 grown on biomass-based hollow carbon fibers for energy storage. Appl. Surf. Sci. 469, 854–863. doi: 10.1016/j.apsusc.2018.11.067
Liu, X., Yuan, Y., Liu, J., Liu, B., Chen, X., Ding, J., et al. (2019d). Utilizing solar energy to improve the oxygen evolution reaction kinetics in zinc–air battery. Nat. Commun. 10:4767. doi: 10.1038/s41467-019-12627-2
Liu, Y., Wang, H. C., Yang, K. K., Yang, Y. N., Ma, J. Q., Pan, K. M., et al. (2019e). Enhanced electrochemical performance of Sb2O3 as an anode for lithium-ion batteries by a stable cross-linked binder. Appl. Sci. 9:2677. doi: 10.3390/app9132677
Liu, Y., Wang, Y., Wang, F., Lei, Z. X., Zhang, W. H., Pan, K. M., et al. (2019f). Facile synthesis of antimony tungstate nanosheets as anodes for lithium-ion batteries. Nanomaterials 9:1689. doi: 10.3390/nano9121689
Liu, G. L., Li, M. L., Wu, N. T., Cui, L., Huang, X. X., and Liu, X. M. (2018). Single-crystalline particles: an effective way to ameliorate the intragranular cracking, thermal stability, and capacity fading of the LiNi0.6Co0.2Mn0.2O2 electrodes. J. Electrochem. Soc. 165, A3040–A3047. doi: 10.1149/2.0491813jes
Liu, Z., Liu, C., Li, L. F., Qin, W., and Xu, A. R. (2016). CO2 separation by supported ionic liquid membranes and prediction of separation performance. Int. J. Greenhouse Gas Control 53, 79–84. doi: 10.1016/j.ijggc.2016.07.041
Ma, J. L., Ren, F. Z., Wang, G. X., Xiong, Y., Li, Y. Q., and Wen, J. B. (2017). Electrochemical performance of melt-spinning Al-Mg-Sn based anode alloys. Int. J. Hydrogen Energy 42, 11654–11661. doi: 10.1016/j.ijhydene.2017.02.185
Ma, J. L., Zhang, Y., Qin, C. H., Ren, F. Z., and Wang, G. X. (2020). Effects of polystyrene sulfonate/graphene and Mn3O4/graphene on property of aluminum(zinc)-air batteries. Int. J. Hydrogen Energy 45, 13025–13034. doi: 10.1016/j.ijhydene.2020.02.222
Ma, J. Q., Wei, H. J., Liu, Y., Ren, X. Y., Li, Y. X., Wang, F., et al. (2020). Application of Co3O4-based materials in electrocatalytic hydrogen evolution reaction: a review. Int. J. Hydrogen Energy 41, 21205–21220. doi: 10.1016/j.ijhydene.2020.05.280
Ma, J. Y., Guo, X. T., Xue, H. G., Pan, K. M., Liu, C. S., and Pang, H. (2020). Niobium/tantalum-based materials: synthesis and applications in electrochemical energy storage. Chem. Eng. J. 380:122428. doi: 10.1016/j.cej.2019.122428
Ma, X. D., Xiong, X. H., Zou, P. J., Liu, W. Z., Wang, F., Liang, L. W., et al. (2019). General and scalable fabrication of core-shell metal Sulfides@C anchored on 3D N-doped foam toward flexible sodium ion batteries. Small 15:1903259. doi: 10.1002/smll.201903259
Palaniyandy, N., Kebede, M. A., Raju, K., Ozoemena, K. I., le Roux, L., Mathe, M. K., et al. (2019). alpha-Mno(2) nanorod/onion-like carbon composite cathode material for aqueous zinc-ion battery. Mater. Chem. Phys. 230, 258–266. doi: 10.1016/j.matchemphys.2019.03.069
Pan, H., Shao, Y., Yan, P., Cheng, Y., Han, K. S., Nie, Z., et al. (2016). Reversible aqueous zinc/manganese oxide energy storage from conversion reactions. Nat. Energy 1:16039. doi: 10.1038/nenergy.2016.39
Pan, K., Wang, F., Wei, S., Siyal, S. H., Ren, Y., Xu, L., et al. (2020). Low-temperature solution synthesis and characterization of enhanced titanium dioxide photocatalyst on tailored mesoporous γ-Al2O3 support. Compos. Commun. 19, 82–89. doi: 10.1016/j.coco.2020.02.009
Poyraz, A. S., Laughlin, J., and Zec, Z. (2019). Improving the cycle life of cryptomelane type manganese dioxides in aqueous rechargeable zinc ion batteries: the effect of electrolyte concentration. Electrochim. Acta 305, 423–432. doi: 10.1016/j.electacta.2019.03.093
Qiu, N., Chen, H., Yang, Z., Sun, S., and Wang, Y. (2018a). Low-cost birnessite as a promising cathode for high-performance aqueous rechargeable batteries. Electrochim. Acta 272, 154–160. doi: 10.1016/j.electacta.2018.04.012
Qiu, N., Chen, H., Yang, Z., Sun, S., and Wang, Y. (2018b). Synthesis of manganese-based complex as cathode material for aqueous rechargeable batteries. RSC Adv. 8, 15703–15708. doi: 10.1039/c8ra01982g
Song, C. K., Feng, W. J., Wang, X., and Shi, Z. J. (2020). Enhanced electrochemical performance of Li1.2Mn0.54Ni0.13Co0.13O2 cathode material with bamboo essential oil. Ionics 26, 661–672. doi: 10.1007/s11581-019-03233-9
Song, M., Tan, H., Chao, D., and Fan, H. J. (2018). Recent advances in Zn-ion batteries. Adv. Funct. Mater. 28:1802564. doi: 10.1002/adfm.201802564
Sun, W., Wang, F., Hou, S., Yang, C., Fan, X., Ma, Z., et al. (2017). Zn/MnO2 battery chemistry with H+ and Zn2+ Coinsertion. J. Am. Chem. Soc. 139, 9775–9778. doi: 10.1021/jacs.7b04471
Tang, B., Shan, L., Liang, S., and Zhou, J. (2019). Issues and opportunities facing aqueous zinc-ion batteries. Energy Environ. Sci. 12, 3288–3304. doi: 10.1039/C9EE02526J
Tao, Y., Li, Z., Tang, L., Pu, X., Cao, T., Cheng, D., et al. (2020). Nickel and cobalt Co-substituted spinel ZnMn2O4@N-rGO for increased capacity and stability as a cathode material for rechargeable aqueous zinc-ion battery. Electrochim. Acta 331:135296. doi: 10.1016/j.electacta.2019.135296
Wan, F., and Niu, Z. Q. (2019). Design strategies for vanadium-based aqueous zinc-ion batteries. Angew. Chem. Int. Ed. 58, 16358–16367. doi: 10.1002/anie.201903941
Wang, C., Zeng, Y., Xiao, X., Wu, S., Zhong, G., Xu, K., et al. (2020). gamma-MnO2 nanorods/graphene composite as efficient cathode for advanced rechargeable aqueous zinc-ion battery. J. Energy Chem. 43, 182–187. doi: 10.1016/j.jechem.2019.08.011
Wang, F., Liu, Y., Wei, H., Wang, G., Ren, F., Liu, X., et al. (2020). Graphene induced growth of Sb2WO6 nanosheets for high-performance pseudocapacitive lithium-ion storage. J. Alloys Compd. 839:155614. doi: 10.1016/j.jallcom.2020.155614
Wang, F., Liu, Y., Zhao, Y. F., Wang, Y., Wang, Z. J., Zhang, W. H., et al. (2018). Facile synthesis of two-dimensional porous MgCo2O4 nanosheets as anode for lithium-ion batteries. Appl. Sci. 8:22. doi: 10.3390/app8010022
Wang, G., Chen, C., Chen, Y. H., Kang, X. W., Yang, C. H., Wang, F., et al. (2020). Self-stabilized and strongly adhesive supramolecular polymer protective layer enables ultrahigh-rate and large-capacity lithium-metal anode. Angew. Chem. Int. Ed. 59, 2055–2060. doi: 10.1002/anie.201913351
Wang, J., Sun, X., Zhao, H., Xu, L., Xia, J., Luo, M., et al. (2019a). Superior-performance aqueous zinc ion battery based on structural transformation of MnO2 by rare earth doping. J. Phys. Chem. C 123, 22735–22741. doi: 10.1021/acs.jpcc.9b05535
Wang, J., Wang, J.-G., Liu, H., Wei, C., and Kang, F. (2019b). Zinc ion stabilized MnO2 nanospheres for high capacity and long lifespan aqueous zinc-ion batteries. J. Mater. Chem. A 7, 13727–13735. doi: 10.1039/c9ta03541a
Wang, J. D., Xiao, X., Liu, Y., Pan, K. M., Pang, H., and Wei, S. Z. (2019c). The application of CeO2-based materials in electrocatalysis. J. Mater. Chem. A 7, 17675–17702. doi: 10.1039/c9ta04804a
Wang, R., Cao, X., Zhao, D., Zhu, L., Xie, L., Liu, J., et al. (2020). Wet-chemistry synthesis of Li4Ti5O12 as anode materials rendering high-rate Li-ion storage. Int. J. Energy Res. 44, 4211–4223. doi: 10.1002/er.5020
Wang, W., Sun, K., and Liu, H. (2020). Effects of different aluminum sources on morphologies and properties of ceramic floor tiles from red mud. Constr. Build. Mater. 241:118119. doi: 10.1016/j.conbuildmat.2020.118119
Wang, X. W., Yang, C. H., Xiong, X. H., Chen, G. L., Huang, M. Z., Wang, J. H., et al. (2019d). A robust sulfur host with dual lithium polysulfide immobilization mechanism for long cycle life and high capacity Li-S batteries. Energy Storage Mater. 16, 344–353. doi: 10.1016/j.ensm.2018.06.015
Wu, B., Zhang, G., Yan, M., Xiong, T., He, P., He, L., et al. (2018). Graphene scroll-coated alpha-MnO2 nanowires as high-performance cathode materials for aqueous Zn-ion battery. Small 14:1703850. doi: 10.1002/smll.201703850
Wu, N., Shen, J., Sun, L., Yuan, M., Shao, Y., Ma, J., et al. (2019). Hierarchical N-doped graphene coated 1D cobalt oxide microrods for robust and fast lithium storage at elevated temperature. Electrochim. Acta 310, 70–77. doi: 10.1016/j.electacta.2019.04.115
Wu, X., Li, Y., Li, C., He, Z., Xiang, Y., Xiong, L., et al. (2015). The electrochemical performance improvement of LiMn2O4/Zn based on zinc foil as the current collector and thiourea as an electrolyte additive. J. Power Sources 300, 453–459. doi: 10.1016/j.jpowsour.2015.09.096
Wu, X., Xiang, Y., Peng, Q., Wu, X., Li, Y., Tang, F., et al. (2017). Green-low-cost rechargeable aqueous zinc-ion batteries using hollow porous spinel ZnMn2O4 as the cathode material. J. Mater. Chem. A 5, 17990–17997. doi: 10.1039/c7ta00100b
Xiao, X., Zhang, G. X., Xu, Y. X., Zhang, H. L., Guo, X. T., Liu, Y., et al. (2019). A new strategy for the controllable growth of MOF@PBA architectures. J. Mater. Chem. A 7, 17266–17271. doi: 10.1039/c9ta05409j
Xu, C., Li, B., Du, H., and Kang, F. (2012). Energetic zinc ion chemistry: the rechargeable zinc ion battery. Angew. Chem. Int. Ed. 51, 933–935. doi: 10.1002/anie.201106307
Xu, C. J., Du, H. D., Li, B. H., Kang, F. Y., and Zeng, Y. Q. (2009). Reversible insertion properties of zinc ion into manganese dioxide and its application for energy storage. Electrochem. Solid State Lett. 12, A61–A65. doi: 10.1149/1.3065967
Xu, D., Li, B., Wei, C., He, Y.-B., Du, H., Chu, X., et al. (2014). Preparation and characterization of MnO2/acid-treated CNT nanocomposites for energy storage with zinc ions. Electrochim. Acta 133, 254–261. doi: 10.1016/j.electacta.2014.04.001
Yang, S., Zhang, M., Wu, X., Wu, X., Zeng, F., Li, Y., et al. (2019). The excellent electrochemical performances of ZnMn2O4/Mn2O3: the composite cathode material for potential aqueous zinc ion batteries. J. Electroanal. Chem. 832, 69–74. doi: 10.1016/j.jelechem.2018.10.051
Yixuan, L., Xiaoliang, Z., Yong, L., Huijie, W., Junqing, M., Min, C., et al. (2020). WO3-based materials as electrocatalysts for hydrogen evolution reaction. Front. Mater. 7:105. doi: 10.3389/fmats.2020.00105
Yu, M., Yin, Z., Yan, G., Wang, Z., Guo, H., Li, G., et al. (2020). Synergy of interlayer expansion and capacitive contribution promoting sodium ion storage in S, N-Doped mesoporous carbon nanofiber. J. Power Sources 449:227514. doi: 10.1016/j.jpowsour.2019.227514
Yuan, C. L., Zhang, Y., Pan, Y., Liu, X. W., Wang, G. L., and Cao, D. X. (2014). Investigation of the intercalation of polyvalent cations (Mg2+, Zn2+) into lambda-MnO2 for rechargeable aqueous battery. Electrochim. Acta 116, 404–412. doi: 10.1016/j.electacta.2013.11.090
Yuan, G., Geng, M., Zhang, P., and Li, B. (2020). Hybrids of LiMn2O4 nanoparticles anchored on carbon nanotubes/graphene sheets as long-cycle-life cathode material for rechargeable hybrid aqueous batteries. J. Solid State Electrochem. 24, 601–607. doi: 10.1007/s10008-020-04504-6
Yuan, M. J., Guo, X. T., Liu, Y., and Pang, H. (2019). Si-based materials derived from biomass: synthesis and applications in electrochemical energy storage. J. Mater. Chem. A 7, 22123–22147. doi: 10.1039/c9ta06934h
Zhang, J., Dong, Y. N., Liu, Q. X., Zhou, M., Mi, G., and Du, X. G. (2019). Hierarchically alloyed Pd-Cu microarchitecture with tunable shapes: morphological engineering, and catalysis for hydrogen evolution reaction of ammonia borane. Int. J. Hydrogen Energy 44, 30226–30236. doi: 10.1016/j.ijhydene.2019.09.213
Zhang, N., Cheng, F., Liu, J., Wang, L., Long, X., Liu, X., et al. (2017). Rechargeable aqueous zinc-manganese dioxide batteries with high energy and power densities. Nat. Commun. 8:405. doi: 10.1038/s41467-017-00467-x
Zhang, N., Cheng, F., Liu, Y., Zhao, Q., Lei, K., Chen, C., et al. (2016). Cation-deficient spinel ZnMn2O4 cathode in Zn(CF3SO3)2 electrolyte for rechargeable aqueous Zn-ion battery. J. Am. Chem. Soc. 138, 12894–12901. doi: 10.1021/jacs.6b05958
Zhang, Y., Chen, L., Hao, C., Zheng, X., Guo, Y., Chen, L., et al. (2020). Potassium pre-inserted K1.04Mn8O16 as cathode materials for aqueous Li-ion and Na-ion hybrid capacitors. J. Energy Chem. 46, 53–61. doi: 10.1016/j.jechem.2019.10.015
Zhao, K., Wang, C., Yu, Y., Yan, M., Wei, Q., He, P., et al. (2018). Ultrathin surface coating enables stabilized zinc metal anode. Adv. Mater. Interfaces 5:1800848. doi: 10.1002/admi.201800848
Zhao, L., Dong, L., Liu, W., and Xu, C. (2018). Binary and ternary manganese dioxide composites cathode for aqueous zinc-ion battery. Chemistryselect 3, 12661–12665. doi: 10.1002/slct.201802954
Zhao, Q., Hao, X. G., Su, S. M., Ma, J. B., Hu, Y., Liu, Y., et al. (2019). Expanded-graphite embedded in lithium metal as dendrite-free anode of lithium metal batteries. J. Mater. Chem. A 7, 15871–15879. doi: 10.1039/c9ta04240g
Zhao, X., Chen, H. L., Kong, F. G., Zhang, Y. J., Wang, S. J., Liu, S. X., et al. (2019). Fabrication, characteristics and applications of carbon materials with different morphologies and porous structures produced from wood liquefaction: a review. Chem. Eng. J. 364, 226–243. doi: 10.1016/j.cej.2019.01.159
Zhu, C., Fang, G., Liang, S., Chen, Z., Wang, Z., Ma, J., et al. (2020). Electrochemically induced cationic defect in MnO intercalation cathode for aqueous zinc-ion battery. Energy Storage Mater. 24, 394–401. doi: 10.1016/j.ensm.2019.07.030
Zhu, C., Fang, G., Zhou, J., Guo, J., Wang, Z., Wang, C., et al. (2018). Binder-free stainless steel@Mn3O4 nanoflower composite: a high-activity aqueous zinc-ion battery cathode with high-capacity and long-cycle-life. J. Mater. Chem. A 6, 9677–9683. doi: 10.1039/c8ta01198b
Keywords: manganese oxide, manganate, cathode materials, zinc-ion batteries, aqueous electrolyte
Citation: Zhang W, Zhai X, Zhang Y, Wei H, Ma J, Wang J, Liang L, Liu Y, Wang G, Ren F and Wei S (2020) Application of Manganese-Based Materials in Aqueous Rechargeable Zinc-Ion Batteries. Front. Energy Res. 8:195. doi: 10.3389/fenrg.2020.00195
Received: 31 May 2020; Accepted: 24 July 2020;
Published: 02 September 2020.
Edited by:
Cheng Zhong, Tianjin University, ChinaCopyright © 2020 Zhang, Zhai, Zhang, Wei, Ma, Wang, Liang, Liu, Wang, Ren and Wei. This is an open-access article distributed under the terms of the Creative Commons Attribution License (CC BY). The use, distribution or reproduction in other forums is permitted, provided the original author(s) and the copyright owner(s) are credited and that the original publication in this journal is cited, in accordance with accepted academic practice. No use, distribution or reproduction is permitted which does not comply with these terms.
*Correspondence: Wanhong Zhang, emhhbmd3aEBoYXVzdC5lZHUuY24=; Yong Liu, bGl1eW9uZzIwOUBoYXVzdC5lZHUuY24=; Shizhong Wei, d3N6QGhhdXN0LmVkdS5jbg==
†These authors have contributed equally to this work