- Leitat Technological Center, Terrassa, Spain
Bioelectrochemical power-to-gas represents a novel solution for electrical energy storage, currently under development. It allows storing renewable energy surplus in the form of methane (CH4), while treating wastewater, therefore bridging the electricity and natural gas (and wastewater) grids. The technology can be coupled with membrane contactors for carbon dioxide (CO2) capture, dissolving the CO2 in wastewater before feeding it to the bioelectrochemical system. This way, the integrated system can achieve simultaneous carbon capture and energy storage objectives, in the scenario of a wastewater treatment plant application. In this study, such technology was developed in a medium-scale prototype (32 L volume), which was operated for 400 days in different conditions of temperature, voltage and CO2 capture rate. The prototype achieved the highest CH4 production rate (147 ± 33 L m–3 d–1) at the lowest specific energy consumption (1.0 ± 0.3 kWh m–3 CH4) when operated at 25°C and applying a voltage of 0.7 V, while capturing and converting 22 L m–3 d–1 of CO2. The produced biogas was nearer to biomethane quality (CH4 > 90% v/v) when CO2 was not injected in the wastewater. Traces of hydrogen (H2) in the biogas, detectable during the periods of closed electrical circuit operation, indicated that hydrogenotrophic methanogenesis was taking place at the cathode. On the other hand, a relevant CH4 production during the periods of open electrical circuit operation confirmed the presence of acetoclastic methanogenic microorganisms in the microbial community, which was dominated by the archaeal genus Methanothrix (Euryarchaeota). Different operational taxonomic units belonging to the bacterial Synergistes phylum were found at the anode and the cathode, having a potential role in organic matter degradation and H2 production, respectively. In the panorama of methanation technologies currently available for power-to-gas, the performances of this bioelectrochemical prototype are not yet competitive, especially in terms of volumetric CH4 production rate and power density demand. However, the possibility to obtain a high-quality biogas (almost reaching biomethane quality standards) at a minimal energy consumption represents a potentially favorable business scenario for this technology.
Introduction
The European Commission fixed a target of 80% CO2 emissions reduction by 2050 (European Commission, 2011). The challenge of economy decarbonization requires the development and implementation of massive amounts of renewable energy sources (RES) and methods for carbon capture and use (CCU). However, a high penetration of renewable energy in the electricity market requires the simultaneous deployment of energy storage systems, to cope with both short-term and long-term fluctuations of the electricity grid (Denholm et al., 2010). Nowadays, electrical batteries can provide a solution for the short-term storage of RES peaks, while pumped hydro or compressed air storage can deal with the long-term storage, although with large investment costs and construction efforts. The development of alternative technologies is required for long-term, high capacity and potentially strategic energy storage (Blanco and Faaij, 2018). Power-to-X (PtX) technologies can convert surplus electrical energy into more easily storable gas or liquid fuels. The PtX technologies currently at higher TRL (technology readiness level) are the ones producing H2 or CH4 as gaseous energy storage vectors (Bailera et al., 2017). Especially the methanation technologies, converting CO2 to CH4, were spotted by the energy industry, as the ones allowing an immediate integration into the existing infrastructure and the interconnection between electrical and natural gas distribution grids, with several advantages in terms of energy cost, system resilience and security (Zeng et al., 2016). Furthermore, for each CH4 molecule produced, a CO2 molecule is consumed, reducing the climate impacts of burning natural gas. Methanation can be performed through thermal, chemical, electrochemical, biological or bioelectrochemical processes (Geppert et al., 2016). Moreover, it can be efficiently coupled with a biogas upgrading unit for cheap CO2 supply (Leonzio, 2019). This possibility opened the way to the idea of a distributed PtX application in wastewater treatment plants (WWTP), instead of centralized plants that would require huge piping of water and/or gaseous reactive streams (Inkeri et al., 2016).
In the recent years, bioelectrochemical systems (BES) emerged as a novel alternative for PtX plants (Geppert et al., 2016). BES use electro-active microorganisms as biocatalysts for wastewater treatment, with simultaneous recovery of energy and/or resources (Harnisch et al., 2011). The standard BES architecture is composed by an anode, where organic matter content of wastewater is oxidized, and a cathode, where a counter reaction occurs. In case of BES-based PtX, the desired energy vector is produced by the cathodic reaction. Cheng et al. (2009) were the first to propose cathodic reduction of CO2 (dissolved into an aqueous medium) into CH4 by an electromethanogenesis BES (hereafter, EMG-BES). The EMG process has been generally developed with double-chamber BES architectures, where anode and cathode are separated by an ionic exchange membrane, focusing on the optimization of the carbon conversion and Coulombic efficiency (Zhang et al., 2019) and aiming to a clear speciation of the microorganisms catalyzing the substrate oxidation at the anode, and the ones performing CO2 reduction at the cathode (Mayer et al., 2019). The single-chamber EMG-BES represents a different approach for PtX, in the panorama of BES-based technologies. It can be coupled directly with anaerobic digestion (Park et al., 2018) and it reduces maintenance, energy losses and complexity of the reactor. This has proven to be effective for biomethane generation, from laboratory to prototype scale (Muñoz-Aguilar et al., 2018; Ceballos-Escalera et al., 2020). In both cases of single and double-chamber EMG-BES, the methanation process is performed within a single step process, not requiring preliminary H2 production and occurring at mild temperature and pressure (25–35°C, 1–3 bar). Also, EMG-BES reactors treat wastewater at the same time than performing methanation, representing this an added value, compared with competitive PtX technologies. In this regard, EMG-BES can connect electrical, wastewater and natural gas grids, reducing overall energy cost and improving its resiliency. Previous studies summarized the factors affecting the performance of bioelectrochemical CH4 production in EMG-BES: microbial communities, cathode potential, electrode materials, among them (Zhen et al., 2018; Noori et al., 2019; Zakaria and Dhar, 2019).
The single-chamber EMG-BES process requires the presence of both organic matter and CO2 in wastewater, to sustain the complementary reactions of oxidation at the anode and reduction at the cathode. As organic matter oxidation generates CO2, the process can be run efficiently with a regular feeding of wastewater, or sludge (Moreno et al., 2016; Xu et al., 2020). On the other hand, CO2 injection can contribute to EMG (and generally to bioelectrochemical processes) by increasing the electrical conductivity of the medium, reducing cathode overpotentials and voltage requirements (Rodríguez-Alegre et al., 2019). Water scrubbing is the most used downstream technique for CO2 capture, adopted by 33.8% of the European biogas upgrading plants (Batlle-Vilanova et al., 2019). Being a pressure-driven technology, it can present operational issues including channeling and flooding. On the other hand, the use of membrane contactors avoids such problems, being a diffusion-driven process. Moreover, the contactors have a compact and scalable architecture, allowing to reach high gas-liquid contact areas (Nogalska et al., 2017). The integration of membrane contactors with a stack of laboratory-scale EMG-BES reactors was previously tested by the authors, achieving good results in terms of CO2 capture and CH4 production (Rodríguez-Alegre et al., 2019).
To the authors’ knowledge, no up-scaled studies have been reported previously about the integration of membrane contactors for CO2 capture in wastewater, and single-chamber EMG-BES converting the dissolved CO2 into biomethane. This study reports for the first time the long-term operation of a stack of EMG-BES cells fed with municipal wastewater, which was integrated with a stack of membrane contactors for CO2 capture in wastewater. The effect of different operational parameters was evaluated, among which temperature, applied voltage and CO2 capture (ON/OFF). A complete assessment in terms of wastewater treatment efficiency, biomethane production rate and quality, current and power consumption was performed. Coulombic efficiency of anode and cathode, and the conversion efficiency of CO2 to CH4 were estimated. Finally, the microbial community colonizing different areas of the EMG-BES cells was evaluated, to get a better understanding of the potential metabolic reactions taking place in the biological reactor.
Materials and Methods
The developed prototype was composed by a membrane-based CO2 capture system coupled to a stack of EMG-BES cells for biomethane production and energy storage (Figure 1). Three polypropylene membrane contactors (3M Liqui-CelTM EXF-2.5 × 8, United States) of 2.5 m2 each one were installed in parallel hydraulic configuration and used for CO2 capture and dissolution in wastewater.
A medium-scale EMG-BES prototype was built by stacking 45 cells together, grouped by 3 into 15 single-chamber, membrane-less reactor modules, as described by Ceballos-Escalera et al. (2020). The volume of each reactor module was 1.78 L (plus 5.2 L due to recirculation tank and piping volumes), reaching a total prototype volume of 32 L. Anode and cathode electrodes (170 cm2 projected surface, each one) were made of thermally treated carbon felt (SGL group, Germany). The total anode/cathode surface was 0.77 m2. The electrical connection to the external circuit was made by stainless steel current collectors. The stack was connected in parallel and powered at 0.7 V by an external power source (TENMA 72-2715, Farnell, Spain). An electrical monitoring system based on several shunt resistances, installed in series to each cell, and three 16-channels DAQ boards (PicoLog 1216, Farnell, Spain) allowed measuring the current consumed by each cell of the stack.
The modules were hydraulically connected in parallel, continuously recirculating the wastewater to a recirculation tank at a rate of 50 mL min–1 per module. A heating system (Huber thermostat CC-K6, Huber, Germany) was connected to the external jacket of the recirculation tank, allowing to control the operating temperature of the stack. Finally, the gas produced in the prototype was trapped by 3 external chambers (gas trap columns in Figure 1), each one connected to 5 reactor modules.
Wastewater Pre-treatment and CO2 Capture Protocol
The stack of EMG-BES reactors was successfully inoculated in 68 days, using anaerobic sludge collected from the anaerobic digester at the local WWTP. Then, the stack was continuously fed with municipal wastewater (primary settler effluent, collected at the local WWTP). Preliminary batch tests identified a hydraulic residence time (HRT) of 22 h as the one guaranteeing the best performances in terms of current density consumption and CH4 production rate (Ceballos-Escalera et al., 2020). However, due to practical constrains related to wastewater supply, adopted HRT was increased to 3.0 ± 0.5 days. The wastewater was initially pre-treated as detailed by Rodríguez-Alegre et al. (2019): wastewater was kept in a pre-treatment tank (60 L) at environmental temperature and basified to pH 13 by NaOH addition (35 mL of 3M NaOH solution per L of wastewater). After 24 h settling, the wastewater was poured to a homogenization tank (50 L) maintained at 4°C.
For a first experimental period (days 69–174), CO2 capture in wastewater was not performed. Therefore, its pH was neutralized by addition of 26 mmol CH3COOH per L, increasing the organic matter concentration to 1.3–1.4 g COD L–1 (where COD stands for chemical oxygen demand) and the conductivity to 10 mS cm–1. A variable quantity of H2SO4 was used to reach a uniform pH of 7.5 (41 ± 14 mmol H2SO4 L–1), before feeding the wastewater to the EMG-BES stack. For a second experimental period (days 175–400), the stack of membrane contactors was activated. Pure CO2 (Carburos Metálicos, Spain) was introduced in the contactors on their lumen-side, from the bottom to the top, while NaOH-basified wastewater was pumped in counter current configuration on the shell side. The wastewater was continuously recirculated to the homogenization tank at a flowrate of 2 L min–1. The pressure of influent CO2 was set at 1 bar (manometer pressure), while its flowrate was regulated at 2 L min–1. The pH of wastewater was continuously measured during the CO2 capture process (by HQ40 multimeter, Hach Lange, Spain). The process was stopped when the wastewater reached a pH of 10, which should have allowed a total carbon (TC) concentration near 1 g L–1, based on previous experience (Rodríguez-Alegre et al., 2019). Then, the wastewater pH was neutralized to 7.5 by addition of acetic acid (same amount as before) and H2SO4 (16 ± 5 mmol L–1), before feeding it to the EMG-BES stack. The entire sequence of operations performed is resumed in Figure 2.
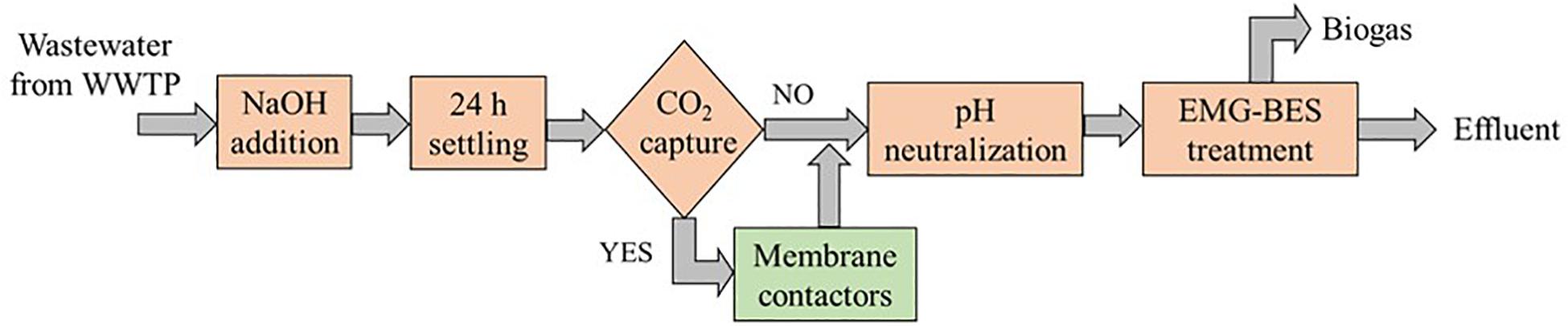
Figure 2. Flow-chart showing the sequence of operations performed, from wastewater pre-treatment to CO2 capture and EMG-BES stack operation.
Operational Conditions Tested
The EMG-BES prototype was tested under different operational conditions. While the influent wastewater was not saturated with CO2, two operation temperatures were tested. During days 69–132 the prototype was operated at 32°C, while for days 133–174 the temperature was decreased to 25°C. For both temperature conditions, both closed circuit (0.7 V) and open circuit voltage (OCV) tests were performed. For the rest of the experiment (days 175–400), the prototype was maintained in stable conditions (25°C, non-stop voltage application, CO2 capture ON) to evaluate long-term performance. The Table 1 resumes the tested operational conditions. Each condition is identified by a binary code, assigning letters A/B to the three independent parameters CO2 capture, temperature and voltage.
Liquid and Gas Phase Characterization
Influent wastewater was sampled after the pre-treatment protocol (either the CO2 capture was activated or not), to analyze its total and inorganic carbon concentration, TC and IC, respectively, (SHIMADZSU TOC-L CSH/CSN analyzer, Spain). The amount of absorbed CO2 (for the condition BAB) could be estimated as the difference between TC values measured before and after the CO2 saturation process. In addition, samples of effluent wastewater were collected twice a week. All the samples were characterized according to Standard Methods in terms of pH, conductivity (HQ40 multimeter, Hach Lange, Spain) and chemical oxygen demand (COD, by LCK 514 kits, Hach Lange, Spain) (APHA, 2005). For the last condition BAB, also the TC and IC concentrations of effluent wastewater were evaluated. Punctual sulfate (SO42–) analysis of the effluent were performed during conditions AAB and BAB, to investigate the dynamics of sulfur species in the prototype.
Gas samples were collected regularly from the gas trap columns by means of Tedlar bags. Their volumetric content in terms of CO2, CH4, O2, N2, and H2 was determined by a Micro-GC (Agilent 490, Spain) with dual channel cabinet and thermal conductivity detector. For the last condition BAB, also H2S content of gas samples was determined. On day 365, a 10 L sample of the produced biogas was collected in a Tedlar bag. The content of volatile organic compounds (VOCs) was captured from the sample by means of a Tenax-TA tube, then desorbed at 280°C and analyzed by gas chromatography (GC) coupled to mass spectrometry (MS) (Agilent 7890B, Agilent Technologies, Spain).
Analysis and Calculations
The organic matter removal efficiency (ηCOD) was determined by Eq. 1, where CODIN and CODOUT are the COD concentrations of influent and effluent wastewater, respectively.
The CH4 production rate was determined by multiplying the gas production rate (quantified daily through the measurement of the accumulated gas volume in the external columns) by its relative CH4 content. A normalized value of CH4 production could be achieved dividing it by the prototype volume.
Current density was calculated by dividing the electrical current by the cathode surface. Power demand of the stack was calculated by multiplying the consumed current by the applied voltage. The individual electrode potentials were measured periodically thanks to Ag/AgCl reference electrodes (+ 0.197 V vs. SHE, Xi’an Yima Opto-electrical Technology, China), installed inside each reactor module. The specific energy consumption of the EMG-BES reactor (in kWh m–3 CH4) was calculated dividing the consumed electrical power by the CH4 production rate. Anode and cathode Coulombic efficiencies were determined as explained by Ceballos-Escalera et al. (2020). All these variables (measured and/or calculated) were statistically treated in order to find the average and standard deviation values, for each operational condition tested.
Based on average current density data (dI), the maximum theoretical CH4 production rate achievable by EMG was estimated (for the operation conditions ABB, AAB and BAB), assuming a cathodic Coulombic efficiency (CEcat) of 100% (Eq. 2).
In Eq. 2, Vmol represents the molar volume of an ideal gas, calculated at the respective operation temperature (25 or 32°C), assuming a gas pressure of 1 atm. The term S refers to the total cathode surface (0.77 m2), C are the e– moles required to reduce 1 mol of CO2 to CH4 (8), F is the Faraday constant (96485 C mol–1) and V is the prototype volume (32 L).
Moreover, the carbon conversion efficiency (ηCONV) from dissolved CO2 to gaseous CH4 was estimated (Eq. 3), as explained by Rodríguez-Alegre et al. (2019).
In Eq. 3, Mm is the molar mass of C (12 g mol–1), ICIN is the inorganic carbon concentration of influent wastewater and Q (L d–1) is the measured feeding rate to the EMG-BES stack. The values of theoretical CH4 production rate achievable by EMG were used, instead than measured CH4 production values, as the excess amount would be due to acetoclastic methanogenesis, not contributing to CO2 conversion.
For these two last parameters (CH4 prod EMG and ηCONV), the standard deviation values were not calculated as their purpose was only orientative (not quantitative), as it will be explained in the section “Efficiency Parameters.”
Microbial Community Analysis
In order to obtain a better understanding of the microbial populations growing in the EMG-BES prototype, the bacterial and archaeal communities associated with (i) bulk medium, (ii) anode biofilm and (iii) cathode biofilm were determined by 16S rRNA gene sequence analysis. On day 211 the reactor module 15 was opened and biofilm samples were collected from all anode and cathode electrodes, together with two biomass samples from the bulk electrolyte.
The samples were centrifuged and stored at −20°C prior to community analysis. DNA was extracted from each sample using the Norgen Total Genomic DNA Purification kit (Norgen Biotek, Canada). PCR amplification of the bacterial (and archaeal) 16S rRNA gene V4 region was carried out with the barcoded primers 515F and 806R, using the DNA extracted from each sample (Caporaso et al., 2012). Amplicons were sequenced on an Illumina MiSeq. The amplicon sequencing data were processed with the software SEED v2.1 (Větrovský et al., 2018). Briefly, pair-end reads were merged using fast-join (Aronesty, 2013). Chimeric sequences were detected and deleted, and sequences were clustered using UPARSE implemented within Usearch, at a 97% similarity level (Edgar, 2013). The most abundant sequence was selected for each cluster, and the closest hits at a genus level were identified using BLAST against the GenBank database. From 16S rRNA in DNA, bacterial and archaeal genome count estimates were calculated based on the 16S copy numbers in the closest available sequenced genome as described previously (Větrovský and Baldrian, 2013). Relative abundance bar chart, heatmap and statistics comparing abundance between bulk medium, anode biofilm and cathode biofilm (multiple t tests) were performed using the software GraphPad v7.04. Significant differences between samples were confirmed by a probability value (p) minor than 0.05. Sequencing data are available in the SRA database with accession number PRJNA627951.
Results and Discussion
The prototype was long-term operated for 400 days. The effects of different operational parameters were evaluated, as presented in the following sections “Methane Production Rate and Electrical Current Consumption,” “Wastewater Treatment,” “Biogas Composition,” “Microbial Community Analysis,” and “Efficiency Parameters.”
Methane Production and Electrical Current Consumption
For the majority of the time, the EMG-BES stack was electrically connected in parallel at 0.7 V applied voltage. The potentials of anode and cathode electrodes, in all the reactor modules, were stable for the whole experiment duration (−0.4 V and −1.1 V vs. Ag/AgCl, respectively). Figure 3 presents the statistical distribution of the experimental values obtained for CH4 production rate (A) and current density (B), in the different experimental conditions tested. While the membrane contactors for CO2 capture were disconnected, two operational temperatures were tested (32 and 25°C), in both closed and open electrical circuit conditions. At 32°C the CH4 production reached 105 ± 11 L m–3 d–1, with a simultaneous current consumption of 0.56 ± 0.04 A m–2. However, the current density was not stable and slowly diminished over time, as shown in Supplementary Figure S1. That was due to the competitive growth of acetoclastic methanogens in the electrolyte medium, competing with electro-active bacteria (EAB) for acetate as unique substrate, as previously reported (Ceballos-Escalera et al., 2020). At 25°C both CH4 production rate and current consumption decreased (22 and 45% reduction, respectively), due to a slower microbial metabolism. At both temperatures, when excluding the EMG process, i.e., during the OCV tests (conditions ABA and AAA), the CH4 production rate slightly decreased compared with the periods applying voltage (conditions ABB and AAB), as shown in Figure 3A. This reduction was lower than the theoretical CH4 production achievable by EMG (see Supplementary Table S1), due to the microbial competition and dynamic equilibrium existing between electroactive and acetoclastic methanogenic populations. This behavior was previously reported when coupling anaerobic methanogenesis and EMG processes (Zhao et al., 2014). Even so, the surplus CH4 production obtained applying voltage (versus the base production in OCV) allowed the calculation of the energy storage efficiency of EMG-BES technology, which was estimated around 42–47% (for calculation details, see the work of Ceballos-Escalera et al., 2020). Strategies to reduce the competition of acetoclastic methanogenesis in the reactor, among other factors, must be developed to ensure an efficient conversion of electricity to CH4 by electro-active microorganisms (Flores-Rodriguez and Min, 2020).
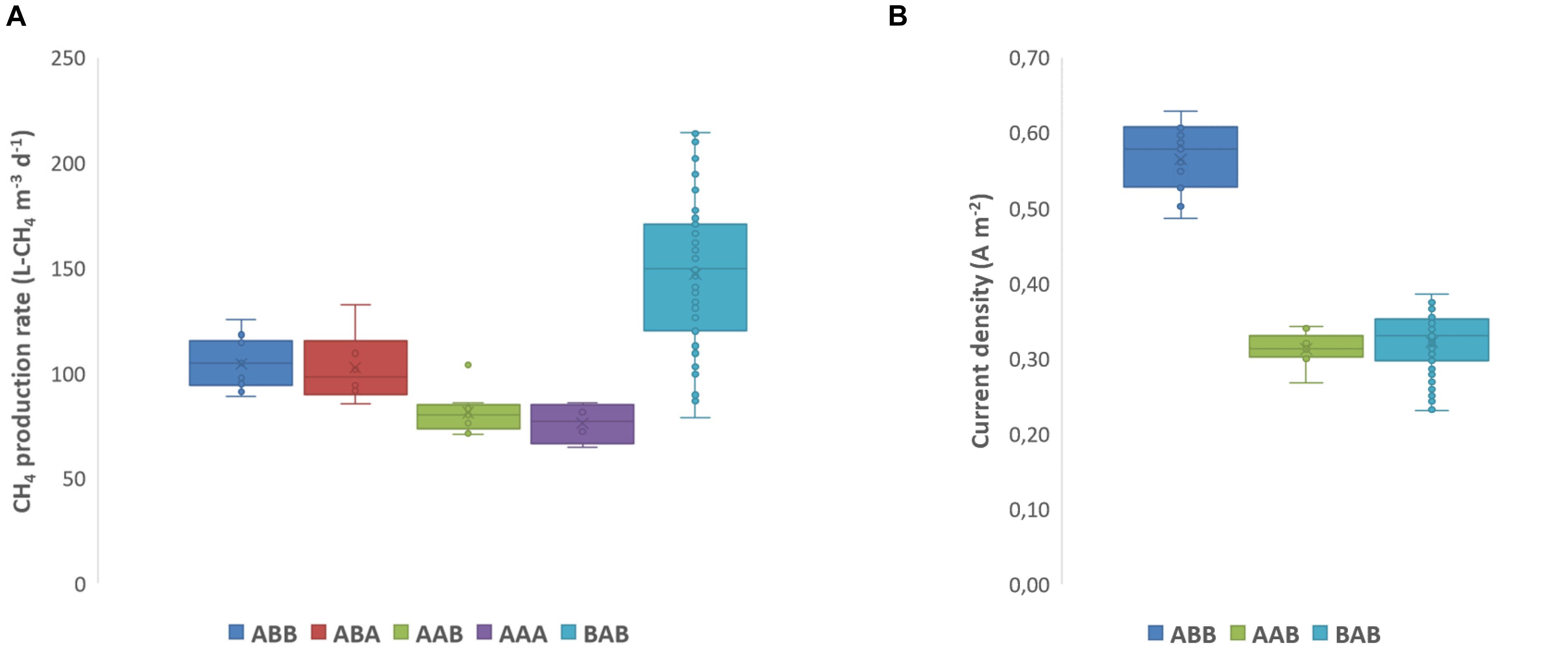
Figure 3. CH4 production rate (A) and current density (B) measured in tested operation conditions. ABB condition refers to days 90 to 118, when stationary phase was reached; current density was null in ABA and AAA conditions (OCV tests).
On the other hand, it is interesting to note the significant increase of CH4 production achieved in the last condition BAB, when the CO2 capture process was activated. The membrane contactors allowed to dissolve CO2 in the influent wastewater, increasing the inorganic carbon availability from 170 ± 17 to 509 ± 241 mg-IC L–1. The average CH4 production, which was equal to 81 ± 9 L m–3 d–1 during condition AAB, increased by 81% reaching an average value of 147 ± 33 L m–3 d–1. However, the wide whiskers in Figure 3A denote a fairly unstable production (see also Supplementary Figure S1). The increment of CH4 production was not matched by an equivalent increase of current density, as shown in Figure 3B. Therefore, it can be inferred that the surplus CH4 achieved by activating the CO2 capture process was not due to an increased activity of EAB at the cathode. Indeed, considering a hypothetical cathodic Coulombic efficiency of 100%, only 14% of the measured CH4 production could be ascribed to EMG in condition BAB (see the difference between CH4 prod. rate and CH4 prod. EMG for condition BAB, in Supplementary Table S1).
Wastewater Treatment
Figure 4A shows the evolution of sulfate concentration in the influent wastewater, for the tested operation conditions. Sulfates presence was mainly due to wastewater pre-treatment, where H2SO4 was adopted for pH neutralization (see Figure 2). This neutralization was needed to balance NaOH addition, which in turn was required to increase the pH and efficiently dissolve the CO2 in the wastewater (Rodríguez-Alegre et al., 2019). The same amount of NaOH was added for all the tested conditions, in order to replicate the same pre-treatment process, with and without CO2 capture. This turned into a significantly higher acid requirement during first conditions, resulting in an average SO42– concentration of 4.1 ± 0.9 g L–1 (condition AAB). Sulfates could act as alternative electron acceptors for the reduction process at the cathode, producing H2S and/or elemental sulfur (Coma et al., 2013) and resulting in a lower CH4 production rate and cathodic Coulombic efficiency (Batlle-Vilanova et al., 2015), when CO2 capture process was not active (see Supplementary Figure S2). Sulfate analysis at the effluent confirmed that 14% of it was removed, or accumulated inside the prototype, during condition AAB. The produced H2S could in turn inhibit the EMG process, affecting also the organic matter removal on the anode side (Supplementary Figure S3). Indeed, the average COD removal efficiency increased from 64 ± 5% to 91 ± 5% passing from condition AAB to BAB, when reducing H2SO4 addition to wastewater (Figure 4B). In this last condition (BAB), sulfates removal/conversion rate in the prototype was highly reduced, and the effluent revealed a slightly higher SO42– concentration than the influent wastewater (1.8 ± 0.7 vs. 1.6 ± 0.5 g L–1), likely due to the re-oxidation to sulfate of the elemental sulfur previously accumulated in the reactor.
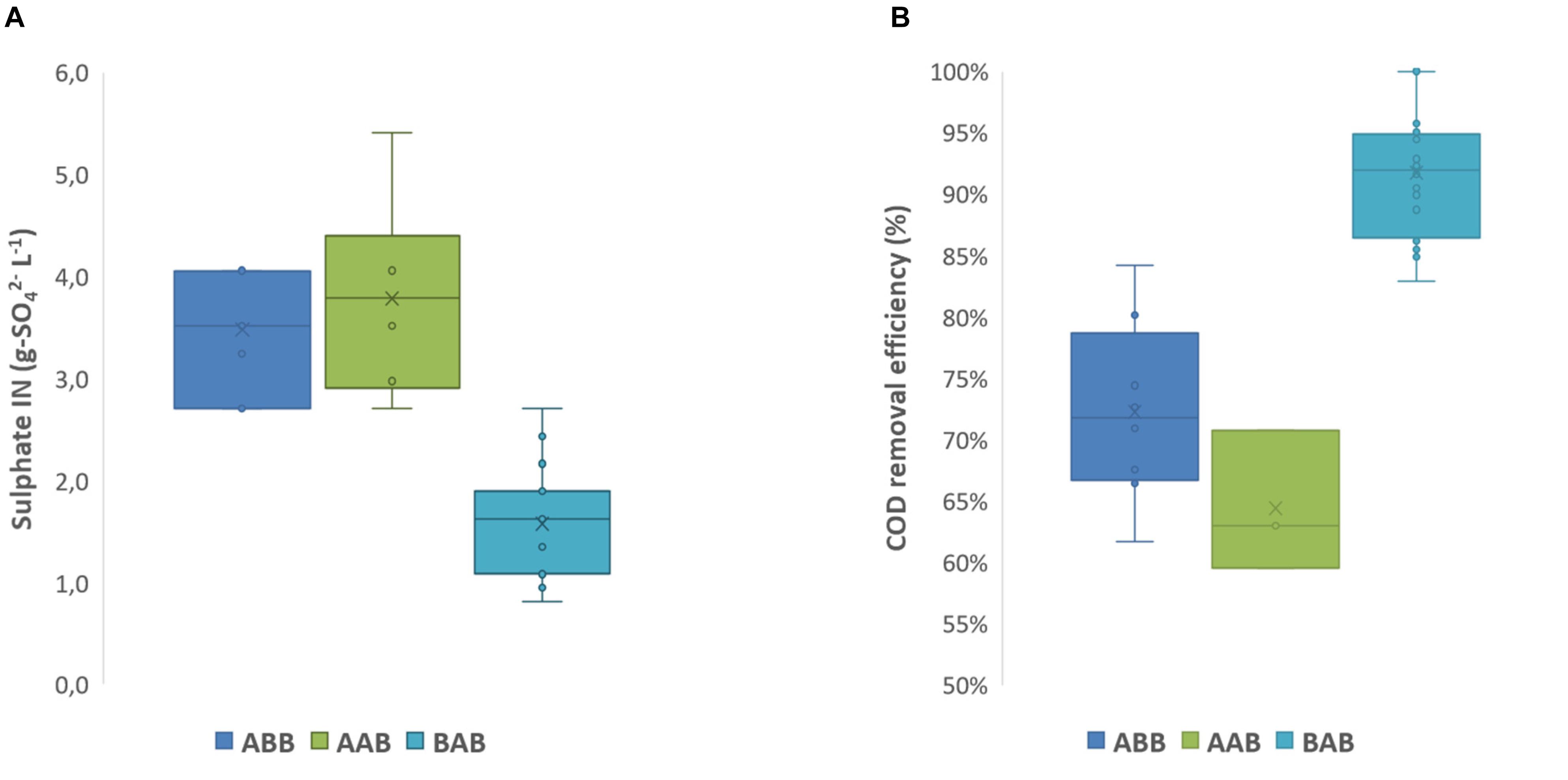
Figure 4. Sulfate concentration of influent wastewater (A) and COD removal efficiency (B) measured in tested operation conditions. ABB condition refers to days 90 to 118, when stationary phase was reached. ABA and AAA conditions are not reported here as not significative.
The ratio IC/TC of the wastewater varied depending on the activation of the CO2 capture process. The influent IC/TC ratio was approximately 26–35% without CO2 capture, and increased to 50% when membrane contactors were activated (Supplementary Table S1). Then, while organic matter was oxidized at the anode of EMG-BES cells, additional CO2 was released into the wastewater. The same CO2 was reduced at the cathode to CH4, resulting in the (partial) volatilization of the carbon content of wastewater. During the last condition BAB (the only one with available IC/TC data for influent and effluent), an average TC removal of 41% was estimated. The carbon remaining in the wastewater after the EMG-BES treatment (0.6 ± 0.2 g-TC L–1) was mostly inorganic (IC/TC ratio of 91%). Only 7% of the inorganic carbon load was valorized to CH4 (see Section “Efficiency Parameters”). Strategies to valorize the residual CO2 of the effluent wastewater must be developed, in order to avoid its later emission to the atmosphere.
The pH and conductivity trends were similar along all the experiment duration. While the pH increased around 1 point from the influent to effluent section of the prototype (due to H+ consumption during EMG process), the conductivity remained stable (Supplementary Table S1). No major variations were expected for these parameters, due to: (i) single-chamber EMG-BES architecture, (ii) natural buffering effect of used wastewater, and (iii) low current densities achieved.
Biogas Composition
The composition of the biogas produced by the prototype was routinely measured in terms of volumetric content of elemental gases. Figure 5 shows the average biogas composition for the different operation conditions. Generally, more than the 85% (v/v) of the biogas was composed by CH4, demonstrating that EMG-BES technology could store electricity in form of a valuable energy vector, near the quality standards of biomethane, i.e., O2 < 1%, H2 < 2%, O2 + CO2 + N2 < 5% (DIN EN 16723-1, 2017). The relevant presence of N2 in the biogas (6–11%) was due to air intrusion in the reactor, which could not be maintained completely airtight. On the other hand, the O2 content of biogas was always minor than 1%, as it was likely reduced to water at the cathode or consumed by heterotrophic bacteria, that were present due to the mixed microbial culture used as inoculum (Ceballos-Escalera et al., 2020). Th eventual inhibition effect of O2 over the electromethanogenic population is still unclear at this stage and will require further research to elucidate its impact on the overall process efficiency.
When the EMG-BES stack was electrically powered (and membrane contactors were not active), almost no CO2 could be found in the biogas effluent (0.5% in ABB, 0.04% in AAB condition). While a minor part of the inorganic carbon was valorized to CH4 (see section “Efficiency Parameters”), the most of it remained trapped in the effluent wastewater, in form of bicarbonate, due to the high pH (Supplementary Table S1). Interestingly, some CO2 was released when EMG was stopped, i.e., during the OCV tests (1.2% in ABA, 2.4% at AAA condition), as it could not be converted to CH4 and due to the slightly lower pH of the effluent. A few H2 was detected in the produced biogas, at a higher amount during electrical closed-circuit operation (0.01‰ in ABB, 0.004‰ in AAB condition) compared with the OCV tests. This suggests that H2 was produced at the cathode, operating indeed at a low potential (−1.1 V vs. Ag/AgCl) compared with the thermodynamic limit required for H2 evolution (−0.6 V vs. Ag/AgCl). The H2 was likely consumed at the same moment of its generation by hydrogenotrophic methanogens, this explaining the extremely low concentrations detected in the biogas (Villano et al., 2010).
When the CO2 capture process was active, CO2 was constantly detected in the biogas, sometimes at non-negligible concentration (1.8 ± 1.5%). Indeed, the CO2 was intentionally dissolved in excess in the wastewater, compared with cathodic reduction capacity, in order to achieve non-limiting EMG conditions (Rodríguez-Alegre et al., 2019). Interestingly, also H2 was detected at the relatively high volumetric concentration of 0.02‰. Hydrogen sulfide was likely always present in the biogas (0.4 ± 0.2‰), as the result of sulfates reduction at the cathode, but its determination could be implemented only for the last condition (BAB), and no values are available for the previous period.
On day 365, a significant volume of biogas was collected, in order to analyze its content of VOCs. Contaminants belonging to the family of alcohols, ketones, non-aromatic hydrocarbons, silicon and sulfur compounds, were found at concentrations higher than 100 μg m–3. Their presence was likely related to the adopted wastewater, which was collected at a municipal WWTP and surely contained some industrial discharges. All the contaminants were found at concentrations lower than threshold values for biomethane quality (Supplementary Table S2), confirming the good quality of the produced biogas.
Microbial Community Analysis
The bacterial and archaeal communities associated with bulk medium, anode and cathode biofilm were determined for the reactor module 15 (arbitrarily chosen). The Figure 6 reports the relative abundance of identified phyla. The biomass contained in the bulk medium was strongly dominated by the Archaea phylum Euryarchaeota (60.2 ± 1.8%). Members of this phylum are well known CH4 producers (Blasco-Gómez et al., 2017), while some of them are also recognized as sulfate reducing bacteria (SRB) (Muyzer and Stams, 2008). On the other hand, the abundance of the phylum Euryarchaeota (41.2 ± 8.2%) significantly decreased (p = 0.003) in the biofilm of the anodes, where the Synergistes (21.7 ± 1.6%) and Chloroflexi (25.2 ± 4.2%) phyla concurrently increased their presence (p = 0.003 and p = 0.013, respectively). Synergistes are significant contributors in the degradation of sludge for the production of biogas, in anaerobic digesters (Rivière et al., 2009). The Chloroflexi phylum is also common in anaerobic digesters (Flores-Rodriguez and Min, 2020). The presence of these two phyla on the anode (although treating wastewater) likely originated from the inoculum sludge and formed symbiotic relationships. On the cathode samples, Euryarchaeota (44.4 ± 11.6%) and Synergistes (12.3 ± 5.0%) were still the dominant phyla. As previously mentioned, Euryarchaeota were surely responsible of CH4 production by EMG, and were likely participating in sulfate reduction. Synergistes are known also to be responsible of H2 production in anaerobic digesters (Rivière et al., 2009), and could contribute in this case to H2 evolution at the cathode, confirming the indications coming from biogas composition analysis (see section “Biogas Composition”). However, in comparison with the wastewater filling the reactor and the anode, Actinobacteria became the dominant phylum in the cathode (23.7 ± 4.5%, p = 0.019 and p = 0.002, respectively). Actinobacteria was previously reported as dominant phylum on biocathodes performing EMG, co-responsible of H2 production hereby taking place (Fu et al., 2015).
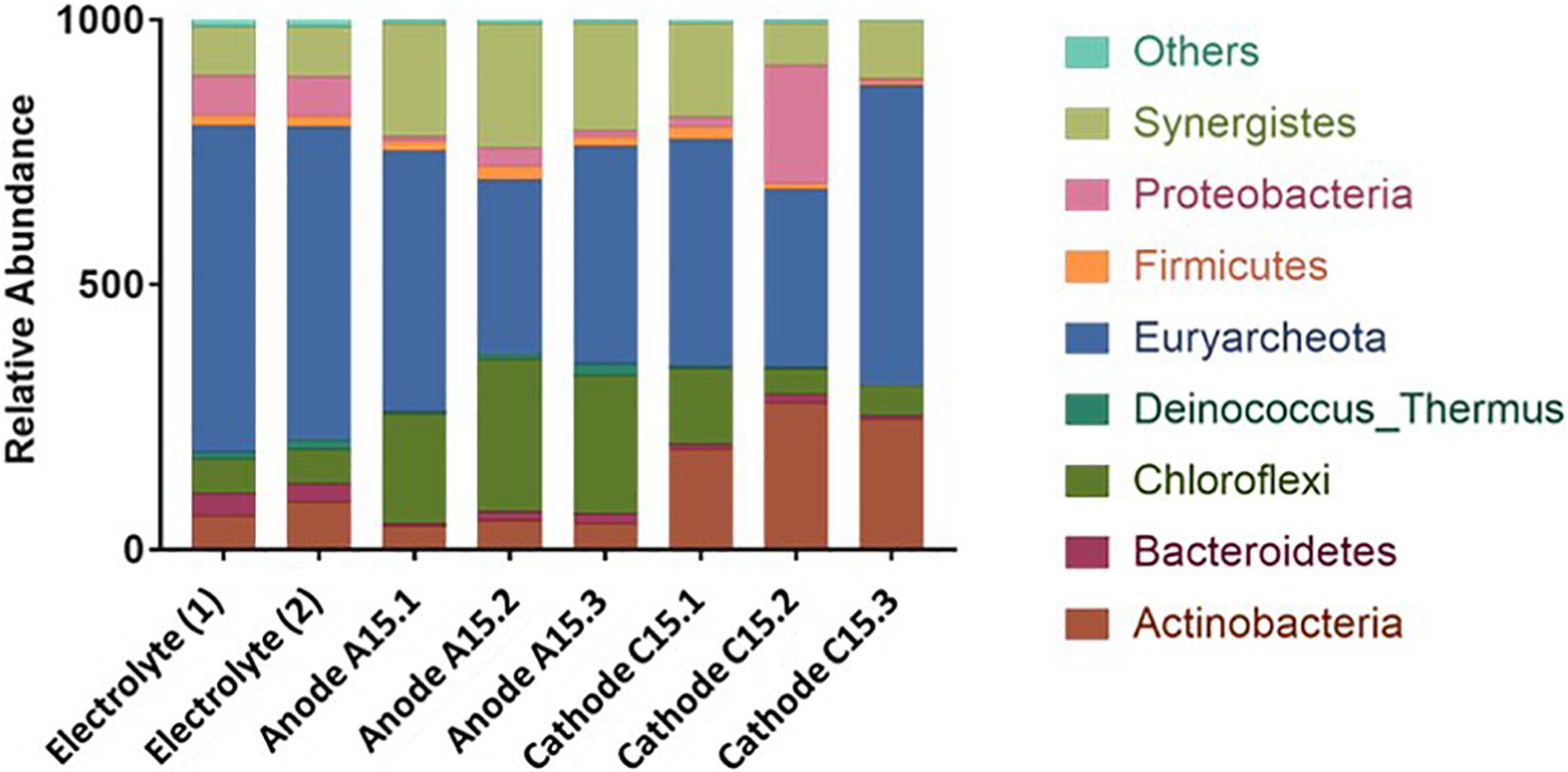
Figure 6. Relative abundance (‰) of the phyla identified in the bulk electrolyte, anode and cathode biofilms of module 15 (samples taken on day 211).
In order to obtain a deeper insight into the microbial diversity, Figure 7 shows the relative abundance of the 20 most abundant Operational Taxonomic Units (OTUs) found in the different samples. This way, it can be observed that OTU 1, belonging to the genus Methanothrix (Euryarchaeota) was the most abundant genus within all the samples, and especially in the bulk medium (58.1 ± 1.4%, p = 0.026 vs. anode, p = 0.044 vs. cathode). Methanothrix can use both acetate and CO2 for CH4 production (Enzmann et al., 2018; Liu et al., 2019), thereby confirming the coexistence of acetoclastic methanogenesis and EMG processes in the prototype. OTU 11 and OTU 7, belonging to the genera Thermovirga (Synergistes) and Levilinea (Chloroflexi), were more abundant in the anodes (7.9 ± 0.1% and 0.9 ± 0.1%, respectively, p = 0.005 and p = 0.011 vs. bulk medium, p = 0.053 and p = 0.016 vs. cathode). Thermovirga was previously reported to accelerate hydrolysis of long-chain fatty acids in anaerobic fermentation reactors, providing low-chain molecules to methanogens for CH4 production (Du et al., 2019) and possibly explaining the relevant presence of Methanothrix on the anode (36.4 ± 5.7%, not previously reported for similar systems). Equal considerations are valid for Levilinea (Zakaria and Dhar, 2019), which was previously reported in the anode bacterial community of microbial electrolysis cells treating waste activated sludge from municipal WWTPs (Zhao et al., 2016). Apart from Methanothrix (32.4 ± 8.4%), the cathode samples revealed the significant presence of OTU 14 and OTU 3, belonging to the genera Atopobium (Actinobacteria) and Methanobacterium (Euryarchaeota) (1.3 ± 0.01% and 0.1 ± 0.01%, respectively, p = 0.011 and p = 0.003 vs. bulk medium; p = 0.002 and p < 0.001 vs. anode). While Methanobacterium is a well-known H2-consuming methanogen and was often reported in the microbial community of EMG biocathodes (Flores-Rodriguez and Min, 2020; Xu et al., 2020; among others), Atopobium was not previously reported and its role is not clear at this stage. In conclusion, the description of the microbial community colonizing the EMG-BES prototype indicated the biological syntrophic relationships between its more relevant members and, as a consequence, the opportunities of such bioelectrochemical system for the simultaneous achievement of electricity storage, CO2 conversion to CH4 and wastewater treatment, due to the metabolic potential of the microbial diversity inhabiting this particular ecosystem. The presence of microorganisms linked to the sulfur cycle opens a potential new field of research, to elucidate the relationship between them and the electromethanogenic population.
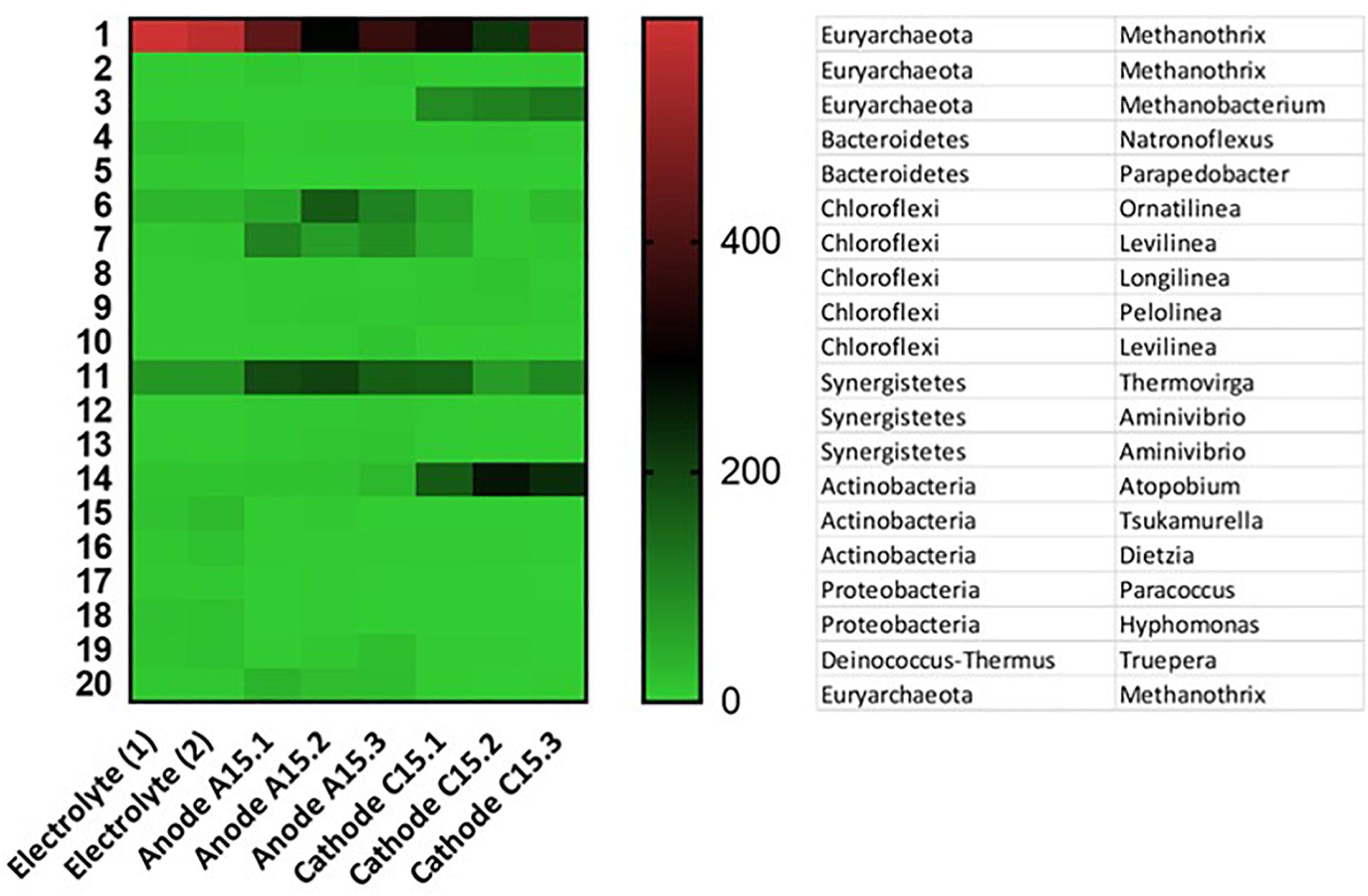
Figure 7. Relative abundance (‰) of the 20 most abundant microorganisms (OTUs) identified in the electrolyte (2 replicates), anode and cathode biofilms of module 15.
Efficiency Parameters
The Coulombic efficiency of anode and cathode was estimated for the different tested conditions. Once the current density stabilized, the anode Coulombic efficiency remained stable (16 ± 2% at condition AAB, 14 ± 4% at condition BAB), showing that CO2 capture activation did not offer a competitive advantage to oxidative EAB at the anode. On the other hand, the cathode Coulombic efficiency increased from 399 ± 49% (AAB) to 694 ± 226% (BAB). As explained by Ceballos-Escalera et al. (2020), cathode Coulombic efficiencies higher than 100% indicate situations where the measured CH4 production is higher than that achievable only by EMG, i.e., acetoclastic methanogenesis became with time the main pathway of acetate degradation in the prototype (Zhao et al., 2014). However, it is meaningful to observe that the specific energy consumption of EMG-BES cells reached its optimum during condition BAB, i.e., when CO2 was injected in the wastewater. A minimum energy input of 1.0 ± 0.3 kWh was necessary to produce 1 m–3 CH4 (compared with 1.6 ± 0.2 kWh m–3 CH4 for condition AAA). This confirmed the validity of the EMG-BES technology, already proofed at laboratory stage by Rodríguez-Alegre et al. (2019), merging the two complementary needs of carbon capture and electricity storage.
In this regard, the CCU potential of the integrated technology of membrane contactors and EMG-BES cells was estimated in terms of (maximum) carbon conversion efficiency from dissolved CO2 to gaseous CH4. The 30% of inorganic carbon was potentially converted to CH4 during condition ABB (see Eq. 3 for calculation details). Reducing the temperature from 32°C to 25°C, only 15% of the inorganic carbon load could be converted. Injecting additional CO2 to the wastewater (condition BAB), only 7% of the carbon was valorized, confirming the values obtained in the laboratory by Rodríguez-Alegre et al. (2019). It must be noted that the estimation of this parameter is not easy, as organic and inorganic carbon can undergo different metabolic processes at both anode and cathode of an EMG-BES reactor. Therefore, the values of carbon conversion efficiency hereby commented should not be taken as quantitative, but only orientative.
Conclusion
A bioelectrochemical power-to-gas prototype was long-term operated (400 days), integrating membrane contactors for CO2 capture in wastewater with a stack of BES cells performing electromethanogenesis. Different operational conditions were tested, acting on parameters like temperature, applied voltage and CO2 capture (ON/OFF). The prototype achieved the highest CH4 production rate (147 ± 33 L m–3 d–1), with a high gas quality (CH4 > 85% v/v) and the lowest energy consumption (1.0 ± 0.3 kWh m–3 CH4), when operated at 25°C and 0.7 V, while capturing and converting 22 L m–3 d–1 of CO2. Only 7% of the injected carbon load could be valorized to CH4, because CO2 was dissolved in excess in the wastewater, compared with cathodic reduction capacity, in order to achieve non-limiting EMG. Traces of H2 in the biogas, detectable during the periods of closed electric circuit, indicated that hydrogenotrophic methanogenesis was taking place at the cathode. On the other hand, a relevant CH4 production during the periods of open electric circuit confirmed the presence of acetoclastic methanogenic microorganisms in the microbial community. This was dominated by the archaea Methanothrix (Euryarchaeota). Different microorganisms belonging to the Synergistes phylum were found at the anode and the cathode, having a potential role in organic matter degradation and H2 production, respectively. In the panorama of methanation technologies currently available for PtX, the performances of this EMG-BES prototype are not yet competitive, especially because of a low CH4 production rate, a limited current/power density demand (<10 W m–3) and a low CO2 conversion efficiency (7–30%). On the other hand, the possibility to obtain a high-quality biogas (near biomethane quality) at a minimal energy consumption leads to a potentially favorable business scenario for this technology, compared with biological methanation or anaerobic digestion.
Data Availability Statement
The datasets presented in this study can be found in online repositories. The names of the repository/repositories and accession number(s) can be found below: https://www.ncbi.nlm.nih.gov/, PRJNA627951.
Author Contributions
DM, PB-J, EB, EL, and RR-A contributed to the prototype design and construction. AM-E and RR-A (among others) performed periodical CO2 saturation of wastewater and took care of the maintenance of the membrane contactors. DM performed experiments on the EMG-BES stack, collected and elaborated the experimental data. JG and SL performed the microbial community analysis. DM wrote the first draft of the manuscript, while all authors contributed to its revision, read and approved the submitted version. MD, PB-J, and EB managed funding acquisition and project direction. All authors contributed to the article and approved the submitted version.
Funding
This work has been financially supported by the Spanish Ministry of Economy and Competitiveness under the project Power2Biomethane (RTC-2016-5024-3, 2016).
Conflict of Interest
The authors declare that the research was conducted in the absence of any commercial or financial relationships that could be construed as a potential conflict of interest.
Acknowledgments
The authors wish to acknowledge David Gali, Olga Gomez Navarro, and the other Leitat collaborators who took part in the Power2Biomethane project.
Supplementary Material
The Supplementary Material for this article can be found online at: https://www.frontiersin.org/articles/10.3389/fenrg.2020.00174/full#supplementary-material
Abbreviations
ηCOD, organic matter removal efficiency; ηCONV, carbon conversion efficiency; BES, bioelectrochemical system; CCU, carbon capture and use; COD, chemical oxygen demand; EAB, electro-active bacteria; EMG, electromethanogenesis; HRT, hydraulic residence time; IC, inorganic carbon; OCV, open circuit voltage; OTU, operational taxonomic unit; PtX, power-to-X; RES, renewable energy source; SHE, standard hydrogen electrode; TC, total carbon; TRL, technology readiness level; VOC, volatile organic compound; WWTP, wastewater treatment plant.
References
APHA (2005). Standard Methods for the Examination of Water and Wastewater, 19th Edn. Washington, DC: American Public Healt Association.
Aronesty, E. (2013). Comparison of sequencing utility programs. TOBIOIJ 7, 1–8. doi: 10.2174/1875036201307010001
Bailera, M., Lisbona, P., Romeo, L. M., and Espatolero, S. (2017). Power to Gas projects review: lab, pilot and demo plants for storing renewable energy and CO2. Renew. Sust. Energ. Rev. 69, 292–312. doi: 10.1016/j.rser.2016.11.130
Batlle-Vilanova, P., Puig, S., Gonzalez-Olmos, R., Vilajeliu-Pons, A., Balaguer, M. D., and Colprim, J. (2015). Deciphering the electron transfer mechanisms for biogas upgrading to biomethane within a mixed culture biocathode. RSC Adv. 5, 52243–52251. doi: 10.1039/c5ra09039c
Batlle-Vilanova, P., Rovira-Alsina, L., Puig, S., Balaguer, M. D., Icaran, P., Monsalvo, V. M., et al. (2019). Biogas upgrading, CO2 valorization and economic revaluation of bioelectrochemical systems through anodic chlorine production in the framework of wastewater treatment plants. Sci. Total Environ. 690, 352–360. doi: 10.1016/j.scitotenv.2019.06.361
Blanco, H., and Faaij, A. (2018). A review at the role of storage in energy systems with a focus on Power to Gas and long-term storage. Renew. Sust. Energ. Rev. 81, 1049–1086. doi: 10.1016/j.rser.2017.07.062
Blasco-Gómez, R., Batlle-Vilanova, P., Villano, M., Balaguer, M., Colprim, J., and Puig, S. (2017). On the edge of research and technological application: a critical review of electromethanogenesis. Int. J. Mol. Sci. 18, 874–874.
Caporaso, J. G., Lauber, C. L., Walters, W. A., Berg-Lyons, D., Huntley, J., Fierer, N., et al. (2012). Ultra-high-throughput microbial community analysis on the Illumina HiSeq and MiSeq platforms. ISME J. 6, 1621–1624. doi: 10.1038/ismej.2012.8
Ceballos-Escalera, A., Molognoni, D., Bosch-Jimenez, P., Shahparasti, M., Bouchakour, S., Luna, A., et al. (2020). Bioelectrochemical systems for energy storage: a scaled-up power-to-gas approach. Appl. Energ. 260:114138. doi: 10.1016/j.apenergy.2019.114138
Cheng, S., Xing, D., Call, D. F., and Logan, B. E. (2009). Direct biological conversion of electrical current into methane by electromethanogenesis. Environ. Sci. Technol. 43, 3953–3958. doi: 10.1021/es803531g
Coma, M., Puig, S., Pous, N., Balaguer, M. D. D., and Colprim, J. (2013). Biocatalysed sulphate removal in a BES cathode. Bioresour. Technol. 130, 218–223. doi: 10.1016/j.biortech.2012.12.050
Denholm, P., Ela, E., Kirby, B., and Milligan, M. (2010). Role of Energy Storage with Renewable Electricity Generation. Golden, CO: National Renewable Energy Lab.
DIN EN 16723-1 (2017). Natural gas and Biomethane for use in Transport and Biomethane for Injection in the Natural Gas Network - Part 1: Specifications for Biomethane for Injection in the Natural Gas Network. Berlin: German Institute for Standardisation.
Du, S., Sun, C., Ding, A., Chen, W., Zhang, M., Cheng, R., et al. (2019). Microbial dynamics and performance in a microbial electrolysis cell-anaerobic membrane bioreactor. J. Zhejiang Univ. Sci. A 20, 533–545. doi: 10.1631/jzus.a1900009
Edgar, R. C. (2013). UPARSE: highly accurate OTU sequences from microbial amplicon reads. Nat. Methods 10, 996–998. doi: 10.1038/nmeth.2604
Enzmann, F., Mayer, F., Rother, M., and Holtmann, D. (2018). Methanogens: biochemical background and biotechnological applications. AMB Exp. 8:1.
European Commission (2011). European Energy Roadmap 2050. Available online at: https://ec.europa.eu/energy/sites/ener/files/documents/2012_energy_roadmap_2050_en_0.pdf (accessed June 5, 2019).
Flores-Rodriguez, C., and Min, B. (2020). Enrichment of specific microbial communities by optimum applied voltages for enhanced methane production by microbial electrosynthesis in anaerobic digestion. Bioresour. Technol. 300:22624.
Fu, Q., Kuramochi, Y., Fukushima, N., Maeda, H., Sato, K., and Kobayashi, H. (2015). Bioelectrochemical analyses of the development of a thermophilic biocathode catalyzing electromethanogenesis. Environ. Sci. Technol. 49, 1225–1232. doi: 10.1021/es5052233
Geppert, F., Liu, D., van Eerten-Jansen, M., Weidner, E., Buisman, C., and ter Heijne, A. (2016). Bioelectrochemical power-to-gas: state of the art and future perspectives. Trends Biotechnol. 34, 879–894. doi: 10.1016/j.tibtech.2016.08.010
Harnisch, F., Aulenta, F., and Schröder, U. (2011). “Microbial fuel cells and bioelectrochemical systems,” in Comprehensive Biotechnology (Amsterdam: Elsevier), 643–659. doi: 10.1016/b978-0-08-088504-9.00462-1
Inkeri, E., Sihvonen, T., Karjunen, H., Tynjälä, T., Tähtinen, M., and Weiss, R. (2016). “Integration of power-to-gas process to wastewater treatment plant with biogas production,” in Conference Proceedings of the 10th International Renewable Energy Storage Conference (IRES 2016), Düsseldorf, 11.
Leonzio, G. (2019). Power to gas systems integrated with anaerobic digesters and gasification systems. Waste Biomass Valor [Epub ahead of print].
Liu, C., Sun, D., Zhao, Z., Dang, Y., and Holmes, D. E. (2019). Methanothrix enhances biogas upgrading in microbial electrolysis cell via direct electron transfer. Bioresour. Technol. 291:121877. doi: 10.1016/j.biortech.2019.121877
Mayer, F., Enzmann, F., Lopez, A. M., and Holtmann, D. (2019). Performance of different methanogenic species for the microbial electrosynthesis of methane from carbon dioxide. Bioresour. Technol. 289:121706. doi: 10.1016/j.biortech.2019.121706
Moreno, R., San-Martín, M. I., Escapa, A., and Morán, A. (2016). Domestic wastewater treatment in parallel with methane production in a microbial electrolysis cell. Renew. Energy 93, 442–448. doi: 10.1016/j.renene.2016.02.083
Muñoz-Aguilar, R., Molognoni, D., Bosch-Jimenez, P., Borràs, E., Della Pirriera, M., and Luna, Á (2018). Design, operation, modeling and grid integration of power-to-gas bioelectrochemical systems. Energies 11, 1947–1947.
Muyzer, G., and Stams, A. J. M. (2008). The ecology and biotechnology of sulphate-reducing bacteria. Nat. Rev. Microbiol. 6, 441–454. doi: 10.1038/nrmicro1892
Nogalska, A., Trojanowska, A., and Garcia-Valls, R. (2017). Membrane contactors for CO2 capture processes – critical review. Phys. Sci. Rev. 2, [Epub ahead of print].
Noori, M. T., Vu, M. T., Ali, R. B., and Min, B. (2019). Recent advances in cathode materials and configurations for upgrading methane in bioelectrochemical systems integrated with anaerobic digestion. Chemi. Eng. J. 3292:123689. doi: 10.1016/j.cej.2019.123689
Park, J.-G., Lee, B., Park, H., and Jun, H. (2018). Long-term evaluation of methane production in a bio-electrochemical anaerobic digestion reactor according to the organic loading rate. Bioresour. Technol. 273, 478–486. doi: 10.1016/j.biortech.2018.11.021
Rivière, D., Desvignes, V., Pelletier, E., Chaussonnerie, S., Guermazi, S., Weissenbach, J., et al. (2009). Towards the definition of a core of microorganisms involved in anaerobic digestion of sludge. ISME J. 3, 700–714. doi: 10.1038/ismej.2009.2
Rodríguez-Alegre, R., Ceballos-Escalera, A., Molognoni, D., Bosch-Jimenez, P., Galí, D., Licon, E., et al. (2019). Integration of membrane contactors and bioelectrochemical systems for CO2 Conversion to CH4. Energies 12, 361–361.
Větrovský, T., and Baldrian, P. (2013). The variability of the 16S rRNA Gene in bacterial genomes and its consequences for bacterial community analyses. PLoS One 8:e57923. doi: 10.1371/journal.pone.0057923
Větrovský, T., Baldrian, P., and Morais, D. (2018). SEED 2: a user-friendly platform for amplicon high-throughput sequencing data analyses. Bioinformatics 34, 2292–2294. doi: 10.1093/bioinformatics/bty071
Villano, M., Aulenta, F., Ciucci, C., Ferri, T., Giuliano, A., and Majone, M. (2010). Bioelectrochemical reduction of CO2 to CH4 via direct and indirect extracellular electron transfer by a hydrogenophilic methanogenic culture. Bioresour. Technol. 101, 3085–3090. doi: 10.1016/j.biortech.2009.12.077
Xu, X.-J., Wang, W.-Q., Chen, C., Xie, P., Liu, W.-Z., Zhou, X., et al. (2020). Bioelectrochemical system for the enhancement of methane production by anaerobic digestion of alkaline pretreated sludge. Bioresour. Technol. 304:123000. doi: 10.1016/j.biortech.2020.123000
Zakaria, B. S., and Dhar, B. R. (2019). Progress towards catalyzing electro-methanogenesis in anaerobic digestion process: fundamentals, process optimization, design and scale-up considerations. Bioresour. Technol. 289:121738. doi: 10.1016/j.biortech.2019.121738
Zeng, Q., Fang, J., Li, J., and Chen, Z. (2016). Steady-state analysis of the integrated natural gas and electric power system with bi-directional energy conversion. Appl. Energ. 184, 1483–1492. doi: 10.1016/j.apenergy.2016.05.060
Zhang, Z., Song, Y., Zheng, S., Zhen, G., Lu, X., Takuro, K., et al. (2019). Electro-conversion of carbon dioxide (CO2) to low-carbon methane by bioelectromethanogenesis process in microbial electrolysis cells: the current status and future perspective. Bioresour. Technol. 279, 339–349. doi: 10.1016/j.biortech.2019.01.145
Zhao, Z., Zhang, Y., Chen, S., Quan, X., and Yu, Q. (2014). Bioelectrochemical enhancement of anaerobic methanogenesis for high organic load rate wastewater treatment in a up-flow anaerobic sludge blanket (UASB) reactor. Sci. Rep. 4, 6658–6658.
Zhao, Z., Zhang, Y., Yu, Q., Ma, W., Sun, J., and Quan, X. (2016). Enhanced decomposition of waste activated sludge via anodic oxidation for methane production and bioenergy recovery. Int. Biodeterior. Biodegradation 106, 161–169. doi: 10.1016/j.ibiod.2015.10.020
Zhen, G., Zheng, S., Lu, X., Zhu, X., Mei, J., Kobayashi, T., et al. (2018). A comprehensive comparison of five different carbon-based cathode materials in CO2 electromethanogenesis: long-term performance, cell-electrode contact behaviors and extracellular electron transfer pathways. Bioresour. Technol. 266, 382–388. doi: 10.1016/j.biortech.2018.06.101
Keywords: carbon capture, electromethanogenesis, energy storage, methanation, microbial community, renewable energy
Citation: Molognoni D, Bosch-Jimenez P, Rodríguez-Alegre R, Marí-Espinosa A, Licon E, Gallego J, Lladó S, Borràs E and Della Pirriera M (2020) How Operational Parameters Affect Electromethanogenesis in a Bioelectrochemical Power-to-Gas Prototype. Front. Energy Res. 8:174. doi: 10.3389/fenrg.2020.00174
Received: 12 May 2020; Accepted: 06 July 2020;
Published: 28 July 2020.
Edited by:
Luis M. Romeo, University of Zaragoza, SpainCopyright © 2020 Molognoni, Bosch-Jimenez, Rodríguez-Alegre, Marí-Espinosa, Licon, Gallego, Lladó, Borràs and Della Pirriera. This is an open-access article distributed under the terms of the Creative Commons Attribution License (CC BY). The use, distribution or reproduction in other forums is permitted, provided the original author(s) and the copyright owner(s) are credited and that the original publication in this journal is cited, in accordance with accepted academic practice. No use, distribution or reproduction is permitted which does not comply with these terms.
*Correspondence: Daniele Molognoni, ZG1vbG9nbm9uaUBsZWl0YXQub3Jn