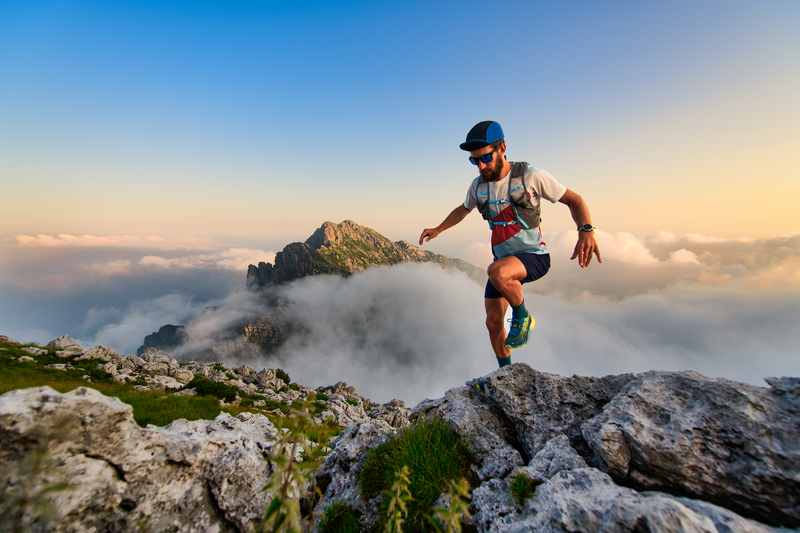
95% of researchers rate our articles as excellent or good
Learn more about the work of our research integrity team to safeguard the quality of each article we publish.
Find out more
PERSPECTIVE article
Front. Energy Res. , 10 July 2020
Sec. Process and Energy Systems Engineering
Volume 8 - 2020 | https://doi.org/10.3389/fenrg.2020.00153
This article is part of the Research Topic Advances in Power-to-X: Processes, Systems, and Deployment View all 10 articles
Power-to-X technologies provide avenues to help de-fossilize a range of large-scale technologies in the mobility, manufacturing, chemical, as well as energy delivery industries. This relates to energy carriers such as hydrogen and hydrocarbons as well as availability of feedstock materials, such as methanol. Technologies for solar and wind-generated electricity and subsequent electrochemical processing are available and are further developed for efficiency and yields. An alternative pathway can be pursued by fully integrating the electricity generation and chemical conversion into one device, thereby reducing technical complexity and removing inefficiencies due to multi-step cascading of processes. In this perspective article, we provide an overview on the recent developments and prospects of artificial photosynthesis, i.e., the chemical transformation of sunlight, water, and carbon dioxide into high-energy-rich fuels. Primary discussions will be focused on the recent development of scalable artificial photosynthesis technology using common semiconductors, e.g., silicon and gallium nitride, for solar fuel production. Technology advances for both hydrogen production from solar water splitting and liquid fuel generation from CO2 reduction will be discussed. The basic operating principles and potential integration with existing and emerging energy infrastructures will be analyzed. The challenges, future prospects of achieving sustainable, large scale applications will also be presented.
Power-to-X technologies provide avenues to help de-fossilize a range of large-scale technologies in the mobility, manufacturing, chemical, as well as energy delivery industries. This relates to energy carriers such as hydrogen and hydrocarbons as well as availability of feedstock materials, such as methanol and other chemicals (Energy Transitions Commission, 2018; International Energy Agency, 2018; National Academies of Sciences Engineering and Medicine, 2019).
Current global production and use quantities for hydrogen exceed 70 million metric tons per year with key applications in fertilizer production, petroleum reforming, and the chemical industry (International Energy Agency, 2019). This demand will further grow substantially as hydrogen is considered ever more strongly as an energy carrier for mobility needs and mid- and long-term energy storage for renewable energy systems. Energy transition scenarios, especially looking at so-called hard-to-abate sectors include the use of hydrogen also for steel making, replacing coal-based coke with hydrogen (Energy Transitions Commission, 2018). Projections are that global hydrogen markets could grow to $2.5T by 2050 (Alvéra, 2019).
In addition, rapidly growing opportunities to utilize CO2 as a feedstock for carbon-based chemicals, polymers, as well as fuels, will increase the demand for hydrogen. Today, the overwhelming amount of hydrogen is produced via steam methane reforming that converts methane and water into hydrogen and carbon dioxide. Direct production of hydrogen from water via electrolysis eliminates the formation and release of carbon dioxide. In particular, with increasing availability of carbon-free energy this becomes an avenue for decarbonizing hydrogen production. It is also an enabling pathway to buffer and store surplus wind-, solar- and hydro-electricity.
In a similar manner, carbon-free electricity can be used to produce hydrocarbons that currently are derived from petroleum or coal and are used as fuels or base chemicals to make a wide range of everyday products (Artz et al., 2018; Hoppe et al., 2018; Kätelhön et al., 2019; Michailos et al., 2019). Fischer-Tropsch-like synthesis that starts with syngas can be tailored to work with carbon monoxide that is produced from carbon dioxide (Styring et al., 2015).
In order to advance technologies for hydrogen and hydrocarbon production, exciting opportunities were created in recent years that integrate the production of electricity and the chemical conversion of water, or conversion of water and carbon dioxide. After a brief status review, prospects and examples are presented for semiconductor-based artificial photosynthesis technologies that could reduce complexity and increase efficiency of conversion processes.
Sunlight is the champion of energy sources: delivering more energy to the earth in an hour than world energy consumption is in a year and it may ultimately address the global energy problem: “The Terawatt Challenge” (Chu et al., 2017). The major obstacle, however, is how to develop an efficient, scalable, and cost-effective approach to store solar energy on demand on a global scale. Artificial photosynthesis, which mimics the natural photosynthesis and converts abundant solar energy into energy-rich chemicals and fuels, e.g., hydrogen (H2) and carbon compounds such as carbon monoxide (CO), methanol (CH3OH), formic acid (HCOOH), and methane (CH4), schematically shown in Figure 1, offers a promising approach to storing solar energy in the form of chemicals and fuels, which can replace conventional fossil fuels widely used in our society today (Bolton, 1996; Turner et al., 2004). Take solar H2 as an example: it can be utilized in fuel cells with water as the only emissions and can be readily employed as a feedstock for various large-scale industry processes, including methanol production via CO2 hydrogenation reaction and ammonia synthesis via Haber-Bosch reaction. For large scale production it is essential to develop efficient and cost-effective artificial photosynthesis system that can compete with traditional methods using fossil fuels.
Figure 1. Schematic illustration of the solar refinery system for converting CO2 and wastewater into chemicals and fuels, including syngas (1), formic acid (2), methanol (3), and methane (4). The selective and efficient reduction of CO2 to various chemicals and fuels can be potentially enabled by integrating suitable catalysts with semiconductor light absorbers.
There are three major paths for solar powered artificial photosynthesis, including photocatalytic, photoelectrochemical (PEC), and photovoltaic-electrolysis (PV-E) systems. The PV-E approach integrates PV cells with appropriate catalysts to drive the chemical reaction. This approach has led to solar-to-hydrogen conversion efficiencies over 10% (Cox et al., 2014; Luo et al., 2014; Nakamura et al., 2015). However, its high cost has been considered a major limiting factor for large scale adoption. In a photoelectrochemical system, the light harvesting and electrochemical reaction process are integrated into a single unit (Pinaud et al., 2013; McKone et al., 2014; Ager et al., 2015). Recent techno-economic analysis suggested that the cost of PEC systems can be significantly reduced compared to PV-E systems (Pinaud et al., 2013; Sathre et al., 2014; Shaner et al., 2016). With further improved efficiency and reliability, it can potentially become highly competitive compared with conventional fossil-fuels. Compared to PV-E and PEC methods, the photocatalytic artificial photosynthetic system is a completely wireless approach, i.e., without the use of any electrical connection or components, to produce solar fuels (Fabian et al., 2015). In addition, it has no stringent requirement on the electrolyte. Recent studies have shown that seawater and possibly wastewater can be utilized without degrading the system performance (Guan et al., 2018a). As such, the manufacturing and operating cost can be drastically reduced, compared to both PV-E and PEC systems.
Critical components for PEC and photocatalytic systems include semiconductor light absorber (photoelectrode) and catalysts. The light-harvesting semiconductor materials, an essential component of the artificial leaf, plays a critical role in determining the efficiency, cost, and stability of artificial photosynthesis systems. The semiconductor light absorber should have a relatively small energy bandgap to absorb a large portion of the solar spectrum while offering sufficient potentials to efficiently drive the artificial photosynthesis chemical reaction, including water oxidation, proton reduction, and/or CO2 reduction. In addition, the semiconductor light absorber should exhibit long-term stability in harsh chemical reactions, have low cost, and be non-toxic, and can be manufactured at large scale. In the past decades, numerous materials have been studied, but they often suffer from low efficiency and poor stability, and are not using industry-friendly materials and processing techniques (Fujishima and Honda, 1972; Hisatomi et al., 2014; Montoya et al., 2016).
Recently, significant advancements in efficient and artificial photosynthesis production of chemicals and fuels using industry-ready materials, e.g., gallium nitride (GaN) and silicon (Si) wafers, have been achieved (Kibria et al., 2015; Vanka et al., 2018; Vanka et al., 2019). The materials (GaN and Si) used to build such an artificial photosynthesis device have been widely used in industry: each year over 10,000 million square inches (or 6.5 million square meters) of Si wafers are produced, which serves as the backbone of the nearly $3-trillion consumer electronics market. GaN, the second most invested semiconductor material only next to Si, has been widely used industrially in solid-state lighting, blue/green laser diodes, and high-power electronic devices, whose market value will soon exceed $100 billion. Take GaN power transistor as an example, they can support current densities on the order of tens of kA/cm2 and hundreds of kilovolts, which are nearly five to six orders of magnitude larger than the photocurrent densities and voltage relevant for artificial photosynthesis devices. Therefore, this breakthrough research promises commercially viable large-scale production of clean chemicals and fuels from unassisted artificial photosynthesis.
Through nano-engineering, gallium nitride based semiconductors exhibit near-ideal electronic, optical, structural, and photocatalytic properties (Kibria and Mi, 2016) compared to other known semiconductor light absorbers for artificial photosynthesis (Kudo and Miseki, 2009). For III-nitride materials with thicknesses of 1 micrometer, nearly 100% of the incident photons above the energy bandgap can be absorbed. Moreover, the surfaces of GaN can be engineered to be N-rich, which can protect against photocorrosion and oxidation during harsh artificial photosynthesis chemical reaction (Kibria et al., 2016). Remarkable stability of up to 3,000 h continuous operation (i.e., over 500 days for usable sunlight ∼5.5 h per day) has been demonstrated without any performance degradation (Guan et al., 2018b; Vanka et al., 2019). In addition, InGaN and Si can be designed to have complementary bandgaps to construct a two-photon tandem system, which is similar to the Z-scheme in natural photosynthesis. A nearly ideal tandem configuration, consisting of InGaN and Si with energy bandgap of 1.75 eV/1.13 eV has been demonstrated recently (Fan et al., 2017). A maximum solar-to-fuel efficiency up to 30% has been predicted for such a Z-scheme artificial photosynthesis system (Hu et al., 2013).
In what follows, we provide a few examples to briefly illustrate the potential of such artificial photosynthesis technologies (Alotaibi et al., 2016; Chu et al., 2018; Shan et al., 2019; Zhou et al., 2019, 2020).
Syngas, a mixture of carbon monoxide and hydrogen, is a key chemical feedstock to upgrade into methanol and long-chain hydrocarbons including synthetic jet, kerosene and diesel fuels, via established industrial process (e.g., Fischer–Tropsch reaction). Global syngas and derivatives market size is estimated to reach 213,100 MW thermal (MWth) by 2020 and will likely continue to grow in the future. It is important to note that direct transportation of syngas, however, may not be trivial. Therefore, the future commercialization of such a system for syngas generation will be likely integrated with chemical synthesis industry to minimize the direct transportation of syngas.
Methanol, known as the simplest alcohol, is also one of the most important feed stocks in chemical industry with an annual production of about 65 million tons. It is a very important precursor for producing various high-value commodity chemicals including gasoline, olefins, propylene, methylamines, and methyl ethers, by well-established technologies. Additionally, methanol itself can be used as bulk chemical as solvent, energy carrier, and gasoline additive. To date, however, methanol is mainly derived from fossil fuels, leading to great emission of CO2 into the atmosphere. The direct hydrogenation of CO2 into methanol presents a viable path for consuming large amounts of captured CO2 due to the extensive market scale of methanol, especially using solar refinery.
Methane is the main component of natural gas and is widely used as fuel for domestic heating and electricity generation in gas turbines or steam generators. Moreover, methane is mainly used in Steam Methane Reforming (SMR) in industry for producing bulk hydrogen with a productivity of more than 50 million metric tons annually worldwide. However, the exploration of natural gas indicates great carbon emission. Recently, it has been demonstrated that methane can be produced with selectivity of up to 50% based on GaN-based materials from CO2 and water (Zhou et al., 2020). The technology not only helps reduce carbon emissions but also offers great economic benefits.
The mass and energy balance of the CO2 conversion system is analyzed based on the conversion of CO2 to syngas, shown in Eq. (1).
Assuming 2400 h operation per year (8 h a day and 300 days), for a conversion plant with a 10 MW capacity, 55 Kt of CO2 and 22.5 Kt of water will be consumed, and 35 Kt of CO, 2.5 Kt of H2 and 40 Kt of O2 will be produced. Similarly, 40 Kt of methanol or 20 Kt of methane can be produced from 55 Kt of CO2 to 45 Kt of H2O, illustrated in Figure 2. Here 10 MW energy is stored in the form of chemical bonds of syngas. Performance, cost, and direct integration with current energy and chemical industry infrastructures have been considered as some of the major challenges for this technology to be quickly adopted for commercial production of various chemicals and fuels, which can be mitigated with further research and development and through innovations in photocatalyst materials and system design and integration.
Figure 2. Illustration of a 10 MW artificial photosynthesis plant, which can be potentially tuned to produce approximately 40 Kt of methanol and 60 Kt of O2 per year.
Artificial photosynthesis production of chemicals and fuels from some of the most abundant and free resources available on earth, i.e., sunlight, water, and carbon dioxide, is highly relevant for the societal transition to a sustainable and clean energy resource future. The GaN-based artificial photosynthetic system offers distinct advantages: the use of sunlight as the energy source, low cost and ambient operation, and leveraging well established semiconductor manufacturing processes. As such, it is expected that the development of artificial photosynthesis technology may follow the trend of solar cells and can be potentially scaled up in the near future. The products generated from this technology are chemicals and fuels such as hydrogen, oxygen, syngas, methane and methanol, which can be well integrated with current energy infrastructure, but with minimized environmental impact. The artificial photosynthesis technology is carbon negative with potentially the smallest greenhouse gas footprint compared to other competing approaches while meeting the increasing global energy demand.
ZM and VS contributed to the conception and design of this perspective. ZM is mainly responsible for the artificial photosynthesis section. VS provided the framework. Both authors contributed to the article and approved the submitted version.
ZM is the co-founder of NS Nanotech Inc., and NX Fuels, Inc., which are in the businesses of developing and commercializing nanowire-based light emitting devices and artificial photosynthesis technology.
The remaining author declares that the research was conducted in the absence of any commercial or financial relationships that could be construed as a potential conflict of interest.
VS is grateful for financial support from the Global CO2 Initiative. VS and ZM appreciate support from the Blue Sky program at the College of Engineering at the University of Michigan.
ZM expresses grateful thanks to Dr. Sheng Chu, Dr. Baowen Zhou, Dr. Mingxin Liu, and Dr. Faqrul Chowdhury for valuable discussions and for their help with analysis and technical input.
Ager, J. W., Shaner, M. R., Walczak, K. A., Sharp, I. D., and Ardo, S. (2015). Experimental demonstrations of spontaneous, solar-driven photoelectrochemical water splitting. Energy Environ. Sci. 8, 2811–2824. doi: 10.1039/c5ee00457h
Alotaibi, B., Kong, X., Vanka, S., Woo, S. Y., Pofelski, A., Oudjedi, F., et al. (2016). Photochemical carbon dioxide reduction on Mg-doped Ga(In)N nanowire arrays under visible light irradiation. ACS Energy Lett. 1, 246–252. doi: 10.1021/acsenergylett.6b00119
Artz, J., Müller, T. E., Thenert, K., Kleinekorte, J., Meys, R., Sternberg, A., et al. (2018). Sustainable conversion of carbon dioxide: an integrated review of catalysis and life cycle assessment. Chem. Rev. 118, 434–504. doi: 10.1021/acs.chemrev.7b00435
Bolton, J. R. (1996). Solar photoproduction of hydrogen: a review. Solar Energy 57, 37–50. doi: 10.1016/0038-092x(96)00032-1
Chu, S., Li, W., Yan, Y., Hamann, T., Shih, I., Wang, D., et al. (2017). Roadmap on solar water splitting: current status and future prospects. Nano Futures 1:022001. doi: 10.1088/2399-1984/aa88a1
Chu, S., Ou, P. F., Ghamari, P., Vanka, S., Zhou, B. W., Shih, I., et al. (2018). Photoelectrochemical CO2 reduction into syngas with the metal/oxide interface. J. Am. Chem. Soc. 140, 7869–7877. doi: 10.1021/jacs.8b03067
Cox, C. R., Lee, J. Z., Nocera, D. G., and Buonassisi, T. (2014). Ten-percent solar-to-fuel conversion with nonprecious materials. Proc. Natl. Acad. Sci. U.S.A. 111, 14057–14061. doi: 10.1073/pnas.1414290111
Energy Transitions Commission (2018). Mission Possible: Reaching Net-Zero Carbon Emissions From Harder-To-Abate Sectors by Mid-Century. London: Energy Transitions Commission.
Fabian, D. M., Hu, S., Singh, N., Houle, F. A., Hisatomi, T., Domen, K., et al. (2015). Particle suspension reactors and materials for solar-driven water splitting. Energy Environ. Sci. 8, 2825–2850. doi: 10.1039/c5ee01434d
Fan, S., Shih, I., and Mi, Z. (2017). A monolithically integrated ingan nanowire/si tandem photoanode approaching the ideal bandgap configuration of 1.75/1.13 eV. Adv. Energy Mater. 7:1600952. doi: 10.1002/aenm.201600952
Fujishima, A., and Honda, K. (1972). Electrochemical photolysis of water at a semiconductor electrode. Nature 238:37. doi: 10.1038/238037a0
Guan, X., Chowdhury, F. A., Pant, N., Guo, L., Vayssieres, L., and Mi, Z. (2018a). Efficient unassisted overall photocatalytic seawater splitting on GaN-based nanowire arrays. J. Phys. Chem. C 122, 13797–13802. doi: 10.1021/acs.jpcc.8b00875
Guan, X., Chowdhury, F. A., Wang, Y., Pant, N., Vanka, S., Trudeau, M. L., et al. (2018b). Making of an industry-friendly artificial photosynthesis device. ACS Energy Lett. 3, 2230–2231. doi: 10.1021/acsenergylett.8b01377
Hisatomi, T., Kubota, J., and Domen, K. (2014). Recent advances in semiconductors for photocatalytic and photoelectrochemical water splitting. Chem. Soc. Rev. 43, 7520–7535. doi: 10.1039/c3cs60378d
Hoppe, W., Bringezu, S., and Wachter, N. (2018). Economic assessment of CO2-based methane, methanol and polyoxymethylene production. J. CO2 Utilizat. 27, 170–178. doi: 10.1016/j.jcou.2018.06.019
Hu, S., Xiang, C. X., Haussener, S., Berger, A. D., and Lewis, N. S. (2013). An analysis of the optimal band gaps of light absorbers in integrated tandem photoelectrochemical water-splitting systems. Energy Environ. Sci. 6, 2984–2993.
Kätelhön, A., Meys, R., Deutz, S., Suh, S., and Bardow, A. (2019). Climate change mitigation potential of carbon capture and utilization in the chemical industry. Proc. Natl. Acad. Sci. U.S.A. 116, 11187–11194. doi: 10.1073/pnas.1821029116
Kibria, M. G., Chowdhury, F. A., Zhao, S., Alotaibi, B., Trudeau, M. L., Guo, H., et al. (2015). Visible light-driven efficient overall water splitting using p-type metal-nitride nanowire arrays. Nat. Commun. 6:6797.
Kibria, M. G., and Mi, Z. (2016). Artificial photosynthesis using metal/nonmetal-nitride semiconductors: current status, prospects, and challenges. J. Mater. Chem. A 4, 2801–2820. doi: 10.1039/c5ta07364b
Kibria, M. G., Qiao, R., Yang, W., Boukahil, I., Kong, X., Chowdhury, F. A., et al. (2016). Atomic-scale origin of long-term stability and high performance of p-GaN nanowire arrays for photocatalytic overall pure water splitting. Adv. Mater. 28, 8388–8397. doi: 10.1002/adma.201602274
Kudo, A., and Miseki, Y. (2009). Heterogeneous photocatalyst materials for water splitting. Chem. Soc. Rev. 38, 253–278. doi: 10.1039/b800489g
Luo, J., Im, J.-H., Mayer, M. T., Schreier, M., Nazeeruddin, M. K., Park, N.-G., et al. (2014). Water photolysis at 12.3% efficiency via perovskite photovoltaics and Earth-abundant catalysts. Science 345, 1593–1596. doi: 10.1126/science.1258307
McKone, J. R., Lewis, N. S., and Gray, H. B. (2014). Will solar-driven water-splitting devices see the light of day? Chem. Mater. 26, 407–414. doi: 10.1021/cm4021518
Michailos, S., Mccord, S., Sick, V., Stokes, G., and Styring, P. (2019). Dimethyl ether synthesis via captured CO2 hydrogenation within the power to liquids concept: a techno-economic assessment. Energy Conv. Manag. 184, 262–276. doi: 10.1016/j.enconman.2019.01.046
Montoya, J. H., Seitz, L. C., Chakthranont, P., Vojvodic, A., Jaramillo, T. F., and Nørskov, J. K. (2016). Materials for solar fuels and chemicals. Nat. Mater. 16:70. doi: 10.1038/nmat4778
Nakamura, A., Ota, Y., Koike, K., Hidaka, Y., Nishioka, K., Sugiyama, M., et al. (2015). A 24.4% solar to hydrogen energy conversion efficiency by combining concentrator photovoltaic modules and electrochemical cells. Appl. Phys. Express 8:107101. doi: 10.7567/apex.8.107101
National Academies of Sciences Engineering and Medicine (2019). Deployment of Deep Decarbonization Technologies: Proceedings of a Workshop. Washington, DC: National Academies of Sciences Engineering and Medicine.
Pinaud, B. A., Benck, J. D., Seitz, L. C., Forman, A. J., Chen, Z. B., Deutsch, T. G., et al. (2013). Technical and economic feasibility of centralized facilities for solar hydrogen production via photocatalysis and photoelectrochemistry. Energy Environ. Sci. 6, 1983–2002.
Sathre, R., Scown, C. D., Morrow, W. R., Stevens, J. C., Sharp, I. D., Ager, J. W., et al. (2014). Life-cycle net energy assessment of large-scale hydrogen production via photoelectrochemical water splitting. Energy Environ. Sci. 7, 3264–3278. doi: 10.1039/c4ee01019a
Shan, B., Vanka, S., Li, T.-T., Troian-Gautier, L., Brennaman, M. K., Mi, Z., et al. (2019). Binary molecular-semiconductor p–n junctions for photoelectrocatalytic CO2 reduction. Nat. Energy 4, 290–299. doi: 10.1038/s41560-019-0345-y
Shaner, M. R., Atwater, H. A., Lewis, N. S., and Mcfarland, E. W. (2016). A comparative technoeconomic analysis of renewable hydrogen production using solar energy. Energy Environ. Sci. 9, 2354–2371. doi: 10.1039/c5ee02573g
Styring, P., Quadrelli, E. A., and Armstrong, K. (eds) (2015). Carbon Dioxide Utilisation: Closing the Carbon Cycle. Amsterdam: Elsevier.
Turner, J. A., Williams, M. C., and Rajeshwar, K. (2004). Hydrogen economy based on renewable energy sources. Electrochem. Soc. Interface 13:24.
Vanka, S., Arca, E., Cheng, S. B., Sun, K., Botton, G. A., Teeter, G., et al. (2018). High efficiency Si photocathode protected by multifunctional GaN nanostructures. Nano Lett. 18, 6530–6537. doi: 10.1021/acs.nanolett.8b03087
Vanka, S., Sun, K., Zeng, G., Pham, T. A., Toma, F. M., Ogitsu, T., et al. (2019). Long-term stability studies of a semiconductor photoelectrode in three-electrode configuration. J. Mater. Chem. A 7:27612. doi: 10.1039/c9ta09926c
Zhou, B., Kong, X., Vanka, S., Cheng, S., Pant, N., Chu, S., et al. (2019). A GaN:Sn nanoarchitecture integrated on a silicon platform for converting CO2 to HCOOH by photoelectrocatalysis. Energy Environ. Sci. 12, 2842–2848. doi: 10.1039/c9ee01339c
Keywords: power-to-X, artificial photosynthesis, green hydrogen, solar fuels, de-fossilization
Citation: Mi Z and Sick V (2020) Taking a Shortcut: Direct Power-to-X Conversion. Front. Energy Res. 8:153. doi: 10.3389/fenrg.2020.00153
Received: 03 March 2020; Accepted: 17 June 2020;
Published: 10 July 2020.
Edited by:
David Parra, Université de Genève, SwitzerlandReviewed by:
Qinghua He, Auburn University, United StatesCopyright © 2020 Mi and Sick. This is an open-access article distributed under the terms of the Creative Commons Attribution License (CC BY). The use, distribution or reproduction in other forums is permitted, provided the original author(s) and the copyright owner(s) are credited and that the original publication in this journal is cited, in accordance with accepted academic practice. No use, distribution or reproduction is permitted which does not comply with these terms.
*Correspondence: Volker Sick, dnNpY2tAdW1pY2guZWR1
Disclaimer: All claims expressed in this article are solely those of the authors and do not necessarily represent those of their affiliated organizations, or those of the publisher, the editors and the reviewers. Any product that may be evaluated in this article or claim that may be made by its manufacturer is not guaranteed or endorsed by the publisher.
Research integrity at Frontiers
Learn more about the work of our research integrity team to safeguard the quality of each article we publish.