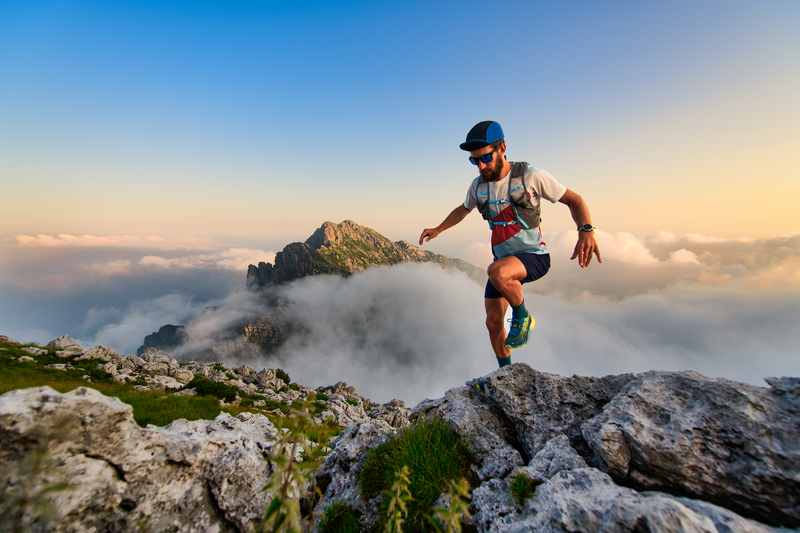
94% of researchers rate our articles as excellent or good
Learn more about the work of our research integrity team to safeguard the quality of each article we publish.
Find out more
REVIEW article
Front. Energy Res. , 03 September 2020
Sec. Electrochemical Energy Storage
Volume 8 - 2020 | https://doi.org/10.3389/fenrg.2020.00139
This article is part of the Research Topic Three-Dimensional Carbon Architectures for Energy Conversion and Storage View all 10 articles
This review focuses on describing the current state-of-the-art research in the synthesis of 3D architectures for electrochemical capacitor applications. The selection is based on both template and non-template strategies. Particular attention has been paid to carbon materials because of their structural interconnection, as they create not only the desired hierarchical porous channels but also ensure high conductivity and mechanical stability. A comprehensive overview of electrode materials is presented here with a detailed discussion of composite solutions, including their advantages and disadvantages. Numerous examples from the literature are presented for individual solutions. The future challenges posed for this type of material are finally summarized.
This review follows a slightly different manner than other papers that appear in the field. The great variety of the materials that might be successfully described and compared makes honest comparison and comprehensive description almost impossible in the frame of one paper. Undoubtedly, there are several reasons for such a situation, with a subjective point of view as the first one. The primary goal of this paper is instead to provide some insights and opinions on the methods and materials that have recently attracted scientific interest and might be considered as promising candidates for further development. Throughout the text, there are almost no values presented, compared, and discussed, essentially in the “better/worse” context; this kind of approach is followed for a specific purpose. Namely, a broad spectrum of the methods applied for the materials testing does not allow for reliable comparisons and evaluation, as the specific capacitance depends on many factors. Of course, taking into account the formula for energy stored in the system, one might expect some kind of contest in this field. But this way might quickly lead to … nowhere. For instance, such a competition in numbers generated the large amount of papers that have reported enormous capacitance values, reaching hundreds or thousands of Farads per gram. Still, they are (in a great majority of cases) battery-like materials, having nothing in common with capacitive or pseudocapacitive properties. Thus, the primary “take-home message” from this paper is that reasonable development does not mean that there should be a race or competition in specific capacitance values, cycle numbers, or Volts presented as operating voltage. Rational development and promising material must be sourced from multivariant analyses that also take into consideration the application of the device, user safety, and the broad spectrum of the final system demands. Without such a discussion and consideration, each new material will be just a small piece of a big cake, that might be (of course!) very tasty but must still contribute to the final effect. In other words, we do not pretend to be able to judge whether an apple pie is better than a brownie, but we instead describe and discuss how to prepare them if there is a wish to enjoy some treat.
The energy storage devices market has been growing over the last decades, and it is a big challenge to satisfy the requirements of modern society. Additionally, an increase in energy consumption and, consequently, fossil fuel depletion has led scientists from all over the world to try to use renewable energy sources and find new materials for advanced technologies (Wang X. et al., 2014; Yu et al., 2015c; Alola et al., 2019). Moreover, it is essential to store energy safely and use it efficiently when needed. Batteries and electrochemical capacitors (ECs) seem to be adequate devices for these purposes (Bernardo et al., 2011; Xu et al., 2013). A comparison between different devices based on specific power and energy values that can be reached by these systems is presented in the Ragone plot (Figure 1).
Figure 1. The Ragone plot presenting specific power and energy values reached by different energy storage devices. Based on data sourced from Simon and Gogotsi (2010), Béguin and Fra̧ckowiak (2013), Budde-Meiwes et al. (2013).
Lithium-ion batteries are one of the most popular devices, applied when required power is rather low, e.g., portable electronics such as cell phones and laptops, but also in electric vehicles. Batteries are also characterized by high energy density values – significantly higher if compared with ECs (Etacheri et al., 2011; Scrosati, 2011; Schipper et al., 2017). On the other hand, when moderate energy has to be delivered in a short time, ECs can be successfully used. ECs are used as a memory back-up in small electrical equipment, such as photo and video cameras or coffee machines. In the automotive industry, ECs can be coupled with batteries and used in hybrid cars, but also in city transportation where “stop-and-go” technology is implemented (Nomoto et al., 2001; Shukla et al., 2012; Miller et al., 2014). Hence, the utility of batteries and ECs strongly depends on the needed properties.
All the mentioned differences between batteries and ECs’ performance come from their different constructions and principles of operation, presented in Figure 2.
Figure 2. Electrochemical capacitor and lithium-ion cell construction. Adapted from Béguin and Fra̧ckowiak (2009) and Béguin and Fra̧ckowiak (2013) with permission from Wiley-VCH Verlag GmbH & Co. KGaA.
A Li-ion battery is composed of one or more cells where chemical reactions occur at a positive and a negative electrode during the charging and discharging processes. The positive electrode (cathode), as a source of lithium cations, can be made of lithium-metal oxides (e.g., LiMn2O4, LiCoO2), olivine LiFePO4, LiNiPO4 or LiCoPO4 and others containing nickel (LiMnNiCoO3, LiNi0.8Co0.15Al0.005O2). The negative electrode (anode) is usually a layered carbon material where lithium ions can be stored (during charge) and released from layers (during discharge). The most popular material is graphite because of its low cost, ready availability and relatively stable structure (Daniel, 2008; Nitta et al., 2015; Kim et al., 2019). There is also a separator between the electrodes that allows ions to flow from one electrode to another, but which prevents electrons from moving through it, to avoid a short circuit. In batteries, different types of electrolytes can be used. Still, liquid organic electrolytes with lithium salts like LiPF6 or LiBF4, or more complex compounds such as LiBC4O8 or Li[PF3(C2F5)3] in organic solvents (e.g., dimethyl carbonate DMC, ethyl methyl carbonate EMC, acetonitrile ACN, and propylene carbonate PC) are used (Younesi et al., 2015).
When the battery is being charged, electrons are moved from the positive electrode to the negative one through an external circuit (the positive electrode is being oxidized, the negative electrode is being reduced) and the positive electrode “releases” some of its lithium ions, which move via the electrolyte to the negative electrode. Energy is taken in and stored in the cell. During discharge, the opposite process takes place – the electrons move back to the positive electrode (reduction of electrode occurs) and lithium ions reach this electrode as well. Chemical energy is thus converted in this process into electricity, and the external device can be powered (Barai et al., 2015; Yu et al., 2019).
ECs’ construction is rather simple – two electrodes made of a highly porous carbon material (with a specific surface area around 2000 m2⋅g–1) are immersed in an electrolyte solution (which is a source of ions) and, like in batteries, separated by a separator (quite often made of glassy fiber) (Pandolfo and Hollenkamp, 2006; Béguin et al., 2014; Zhong et al., 2015; Fic et al., 2018). The principle of operation is based on electrostatic interaction between ions and an electrode surface. During this process, the so-called electrical double-layer is formed. Because of this phenomenon, ECs are also called electric double-layer capacitors (EDLCs). Ions’ attraction to the electrode surface is a very fast and reversible process. The specific power of these devices is higher if compared to batteries.
Electrolytes used for ECs can be aqueous (Khomenko et al., 2006; Ruiz et al., 2009; Fic et al., 2012a,b; Gao et al., 2012; Ratajczak et al., 2014), organic, or ionic liquids (Ruch et al., 2010; Krause and Balducci, 2011; Wang et al., 2012a; Weingarth et al., 2013; Mirzaeian et al., 2017; Hirota et al., 2018). Each of them have different advantages and disadvantages. An operating voltage strongly depends on the kind of medium used and, consequently, the specific energy depends on it as well (Frackowiak et al., 2005; Pandolfo and Hollenkamp, 2006; Béguin et al., 2014).
It seems to be evident that the same electrode materials cannot be used in both device types, because of asymmetric construction of batteries, symmetric construction of EDLCs, and different energy storage/conversion mechanisms. Moreover, the behavior of the electrodes during device operation is supposed to be different. Because of the intercalation process onto negative electrode occurring in Li-ion batteries, the graphite structure can be changed/destroyed when cycled, so it can (and does) expand. It is one of the factors that limits the battery lifetime. Theoretically, for EC’s electrodes, dimensional changes are not expected. However, investigations performed for organic electrolytes mostly show quite significant changes in electrode volume, especially when the electrode is polarized negatively (Hahn et al., 2006; Hantel et al., 2014; Prehal et al., 2015).
The capacity of batteries originates directly from redox reactions, hence its value is relatively high. Capacity is here understood as the amount of charge that can be stored in the cell. It is calculated for active material in the battery. This value is given in Ah and strongly depends on battery “state-of-health” – batteries lose their capacity over time. Moreover, the energy stored in batteries can reach much higher values than in the case of ECs.
To express ECs’ performance, a capacitance value should be calculated (Cdevice). The full device is considered as two capacitors connected in series and the capacitance can be calculated as follows:
In the case of the symmetric cell, both electrodes [Celectrode(+) and Celectrode(–)] have the same capacitance values, hence:
Because of different carbon materials utilization, various constructions, and electrode masses, one should recalculate capacitance given from Eq. 3 to gravimetric capacitance, based on the mass of active material (mact) (Eq. 5) or to normalized capacitance (based on the electrode area) (Eq. 5):
The specific energy value of ECs strongly depends on capacitance, and it can be calculated as follows:
Scientists all over the world have been working on ECs’ performance and energy improvement to reach values competitive to batteries. Hence, modifications of conventional EDLCs were invented (Figure 3).
Figure 3. Various charge storage mechanisms in ECs: electrical double-layer formation (A) and surface redox reactions (B) at the electrode/electrolyte interface; redox reactions from the redox-active electrolyte (C).
Except for typical electrical double-layer formations (Figure 3A), redox reactions can be implemented in ECs. These reactions may have a different origin. One possibility is a modification of the electrode material by introducing transition metal oxides (TMOs) (like manganese oxide) (Khomenko et al., 2006; Yang X.-H. et al., 2007; Malak et al., 2010), or intrinsically conducting polymers (ICPs) (Peng et al., 2008; Li et al., 2009; Fang et al., 2010) or heteroatoms-enriched carbons (Figueiredo et al., 1999; Frackowiak et al., 2006; Raymundo-Piñero et al., 2006; Lota et al., 2007; Figure 3B). This “additional capacitance” from redox reactions occurring at the electrode/electrolyte interface is called pseudocapacitance. Materials that could be used for this purpose are discussed in detail in the next section of this review. Redox-active electrolytes can also be used in ECs as a redox-active species source (Figure 3C). The most popular ones in the aqueous medium are halides (bromide and iodide anions) (Frackowiak et al., 2012; Chen et al., 2015; Menzel et al., 2015; Sathyamoorthi et al., 2016). However, recently, a different group has also been implemented as a redox-active electrolyte – pseudohalides (thiocyanates and selenocyanates) (Gorska et al., 2017; Bujewska et al., 2019).
The key components of the EC are the electrode materials presented in Figure 4, and the electrolyte (Frackowiak et al., 2013). The role of charging the ion-sensitive interface between electrode and electrolyte is here fundamental (Béguin et al., 2014). It seems that higher values of capacitance are obtained when the larger surface area of the electrode is used. However, it has been demonstrated that the pore volume corresponds more substantially with specific capacitance than the surface area does (Frackowiak et al., 2006). Advancement of the specific surface area typically makes the pore walls thinner and the pore diameter a bit wider, but so-called screening effects begin to influence the capacitance properties severely. Therefore, the electrode pore size arrangement cannot be neglected (Pandolfo and Hollenkamp, 2006). Recently, the production of electrode materials has been geared toward achieving sufficient pore size (in correlation with selected electrolyte) with effective pore distribution during long-term cycling for enhanced charge propagation and chemical stability (Alexandru and Andrea, 2017).
Activated carbon (AC) is the material most widely used as an electrode component. Activated carbon has attracted significant attention due to its large surface area, great electrochemical properties, and moderate cost (Pineiro-Prado et al., 2016). Activated carbons can be produced from various types of carbonaceous materials, which are well abundant, via either physical or chemical activation. Physical activation requires the treatment of carbon precursors in the presence of gases such as air, CO2, or steam at high temperatures from 700 to 1200°C. With regards to chemical activation, the activating agents such as sodium hydroxide, potassium hydroxide, zinc chloride, and phosphoric acid are used at lower temperatures from 400 to 700°C (Pandolfo and Hollenkamp, 2006). Activated carbons’ porous structure is obtained using activation processes that have a wide distribution of the pore size consisting of macro-, meso-, and micropores (<2 nm). Overactivation results in large volumes of pores, which in turn leads to drawbacks such as poor conductivity and material density, resulting in low energy density and power loss (Heimbckel et al., 2019). Development and further efforts in carbonization procedures seem to be necessary to consider them as industrially reasonable and feasible.
Activated carbons made of biomass have attracted the attention of scientists around the world as they use abundant and renewable resources. Besides that, they have intriguing properties that derive from the relatively high content of various elements which can provide higher electron density and pseudocapacitive contribution (Yang et al., 2019). An essential feature of the synthesis of ACs from biomass is the low production price. Activated carbons could be produced from different materials like shells, leaves, flowers, bamboo, banana peels, seaweeds, olive stones, and coffee grounds (Fic et al., 2018). Coconut shell is an abundant material with a proper compact structure that is most commonly used in research (Ghosh et al., 2019). Usually, biomass carbonization yield is highly dependent on the type of precursor, preliminary drying, and purification processes, and the yield has a wide range from 30 to 50%. Quite often, the authors are picking biomass without considering a yield of carbonization, elemental composition, quality, price, etc. (Herou et al., 2018). For example, the carbonization of certain flowers is highly doubtful and meaningless due to the scarcity and minor content of carbon, which affects the yield of carbonization (Zhao and Zheng, 2015).
Graphene has recently enjoyed tremendous attention (Marcano et al., 2010). One atom-thick 2D structure has emerged as a unique carbon material with potential for use in energy storage devices due to its outstanding characteristics of high electrical conductivity, chemical stability, and large surface area (Miller et al., 2018; Najib and Erdem, 2019). Recently, it has been suggested that graphene could be used in ECs because it does not rely on the distribution of solid-state pores compared to other carbon materials such as ACs or carbon nanotubes (CNTs; Kim et al., 2013). When the entire specific surface area is fully used, graphene could achieve a capacitance up to 550 F⋅g–1 (Qingqing and John, 2016). The leading quality is that both of the main graphene sheet surfaces could be exterior and easily accessible by an electrolyte. In the area of high-power applications, it is necessary to produce capacitors with high specific capacitance and quick charging times at high current density. It could be possible by synthesizing graphene from the modified Hummer’s method and tip sonication, for use in electric vehicles (Vivekchand et al., 2008). The major drawback of graphene is the process of agglomeration which can lead to restacking back to graphite. This downside could be reduced by thermal reduction of graphite oxide at high temperatures and later rapid cooling using liquid nitrogen.
Carbon nanotubes caught a lot of attention in electrochemistry because of their specific pore structure, good mechanical and thermal stability, and excellent electrical properties (Winkless, 2014). Carbon nanotubes are usually formed by catalytic decomposition of certain hydrocarbons. It is possible to create nanostructures in different frameworks by manipulating different parameters (Zhou Y. et al., 2013). The CNT, unlike other carbon-based electrodes, has mesopores that are intertwined, enabling a continuous charge delivery that uses almost all of the available surface area. Nanotubes are distinguished by their lower electrical resistance values due to the high content of mesopores, which promote the diffusion of ions (Jian et al., 2012). Carbon nanotubes can be used as high-power electrode materials because of their great conductivity and accessible surface area. CNTs could also be a support for other active materials because of their elasticity and mechanical resistance. Unfortunately, nanotubes have a small specific surface area (<500 m2⋅g–1) which in turn leads to a low energy density relative to ACs. However, it is possible to improve specific capacitance of CNTs using chemical activation with potassium hydroxide (Simon and Gogotsi, 2008).
Moreover, carbon materials can be easily modified to improve energy storage devices’ performance. In the case of ECs, surface modifications are the most important due to the surface-related mechanism of charge storage. However, most of the changes also concern the bulk of the material. A comprehensive discussion on this topic can be found in the literature (Liu et al., 2010; Wang G. et al., 2014; Wang et al., 2015; Slesinski et al., 2018; Yao et al., 2020). In many cases, this kind of modification allows an operating voltage to be increased, most likely by changing the pH at the electrode/electrolyte interface (in the case of aqueous electrolytes). It can be done through the self-adjusting mechanism of the pH gradient generation and by creating a protective layer on the electrodes responsible for higher solvent decomposition overpotentials. The introduction of functional groups on the electrode surface may also lead to the redox activity, which directly affects the obtained high capacity value of the device. Nevertheless, one should be aware that oxygen groups present at the surface can diminish the material conductivity; hence, the power and lifetime of the device operating with modified electrodes can be aggravated.
Intrinsically conducting polymers (ICPs) have obtained significant interest as electrode materials for ECs. That is because of their simple manufacturing process and low cost (Kang et al., 2016). Also, in comparison with carbon materials, conductive polymers have a relatively high conductivity and equivalent series resistance. ICPs use reduction-oxidation processes to store and release charge. During the oxidation process (doping), ions are transferred from solution to the polymer chain, but during the reduction process (de-doping) ions are released back to the solution (Thanh-Hai et al., 2017). The reduction-oxidation process creates mechanical stress which, in effect, restricts the stability of the electrode material through many cycles of charge-discharge (Mastragostino et al., 2002).
One of the most popular intrinsically conducting polymer is polyaniline (PANI) due to its high conductivity, simple method of synthesis, and low cost (Zhu H. et al., 2013). Nevertheless, PANI is susceptible to rapid deterioration of performance due to swelling and shrinking during repeated charge-discharge cycles. To avoid this restriction, the combination of PANI with carbon materials has been shown to improve PANI’s stability as well as increase the capacitance value (Chen and Elabd, 2017; Simotwo and Kalra, 2018). Other conductive polymers are polypyrrole (PPy) and poly(3, 4-ethylenedioxythiophene) (PEDOT). They have many advantages, including low cost, flexibility and good electrical conductivity and pseudocapacitance (Tong et al., 2015; Kashani et al., 2016). However, the major drawback remains the same as in the case of PANI: poor stability implying a noticeable decrease in efficiency after a certain number of charge-discharge cycles (Sen and De, 2010; Huang et al., 2016). Another factor that restrains the use of PEDOT and PPy in the ECs industry is limited capacitance. There are several solutions to solve these problems, including the application of conducting nanofillers to improve conductivity and the mixing or depositing of metal oxide to increase capacitance (Zhao et al., 2016).
Transition metal oxides can be listed as materials of interest for use in ECs applications, but caution should be taken when reporting their electrochemical metrics. Some of them provide enough high conductivity, high specific capacity, and low equivalent series resistance, thus making the construction of ECs with high energy and reasonable power appear to be easy (Ullah et al., 2015; Crosnier et al., 2018). RuO2 is among the most highly studied TMOs (Nguyen and Rochefort, 2014). RuO2 has been met with considerable interest due to its long cycle life, wide potential window and highly reversible reduction-oxidation reaction (Amir et al., 2016). However, RuO2 has lost the attention of the industry because of the high production price (Augustyn et al., 2014). Other TMOs commonly used in energy storage devices are NiO (in hybrid systems) and MnO2 (for pseudocapacitive storage) (Zhao et al., 2017; Sadayappan and Kwang-Sun, 2019). Their advantages include their low production cost and environmental friendliness. Nevertheless, they cause cracking of electrodes which results in short-term stability, as their pores cannot be designed or altered in any form (Julien and Mauger, 2017). This is a significant disadvantage that limits their use in electrochemistry.
Metal sulfides, metal selenides, or polyanionic compounds have also received considerable attention. Nickel cobalt sulfide electrodes in asymmetric EDLCs might achieve high values of capacitance and power density with a short charging time (Chen W. et al., 2014). Metal selenides, like cobalt selenides, also exhibit high specific capacitance values and they are distinguished by the great flexibility of final electrodes that might be interesting for use in personal electronic application (Zhang et al., 2019). Polyanionic molybdenophosphate anodes could largely extend the cathodic stability of water and allow the assembly of 2.7 V aqueous ECs (Song et al., 2019). However, all of these materials suffer from low conductivity, which restricts the fast electron transport required for high rate capability, which can even work as an insulator. This kind of problem has been overcome by incorporating highly conductive carbon materials in pseudocapacitive electrodes – it allows the hybrid system to be constructed (Theerthagiri et al., 2018).
Recently, transition metal dichalcogenides (TMDs) have enjoyed widespread interest as electrode materials for ECs. The TMDs are inorganic structured materials composed of transition metals (M) and chalcogens (X: S, Se, Te) which form with a chemical composition of MX2, that provides a rich range of physicochemical properties (Manish et al., 2013). Many two-dimensional (2D) TMDs are used in ECs such as: MoS2, MoSe2, WS2, WTe2, etc. The most commonly used and studied among these TMDs is MoS2. However, MoSe2 presents better electrochemical properties than MoS2, because transition metal selenides demonstrate higher electrical conductivity than their sulfide counterparts (Eftekhari, 2017). The existence of several oxidation states and the large surface area allows 2D TMDs to store charge by Faradic mechanism as well as electric double-layer (EDL) mechanism, ensuring a high specific capacitance and energy density (Nam et al., 2016; Choi et al., 2017). With some interesting features of 2D TMDs, their low inner electrical conductivity hampers their potential to be great electrode materials for ECs (Pumera et al., 2014; Yang et al., 2014).
MXenes are a group of two-dimensional materials that consist of very thin layers of transition metal carbides, nitrides, or carbonitrides (Lukatskaya et al., 2013; Dall’agnese et al., 2014; Ghidiu et al., 2014). The suffix “ene” was added to MAX phases to emphasize their similarity to graphene (Naguib and Gogotsi, 2015). The best known and first discovered MXene is Ti3C2. This material shows capacitance values up to 100 F⋅g–1 for insertion of Li+, Mg2+, or Al3+ in aqueous solutions (Dall’agnese et al., 2016). MXene efficiency can be dramatically improved by adjusting the specific surface groups, or by delaminating or incorporating carbon nanoparticles (Liu Y. et al., 2015; Zhao et al., 2015). However, MXene has been studied primarily in aqueous electrolytes, which have a limited potential window due to water electrolysis. Furthermore, oxidation of Ti3C2 in aqueous electrolytes under strong anodic potentials also restricts its use mostly to asymmetric systems. As both the energy and the power density increase with the square of the voltage, it is crucial to expand the potential window which will improve the performance of ECs.
Composite electrodes combine carbon-based materials with either metal oxide, intrinsically conducting polymer materials, or different carbon materials, which in turn provides both physical and chemical charging storage mechanisms in one single electrode (Vangari et al., 2013).
Carbon materials have a high specific surface area which leads to high capacitance values. By enriching with other carbonaceous material, it is possible to increase the active surface area and thus obtain carbon–carbon ECs with higher energy and power. This would also solve some of the material’s drawbacks. For example, the chemical reduction of graphene oxide may lead to its aggregation and restacking through van der Waals interactions back to graphite, thereby making less area for the electrolyte. However, the use of single-walled CNTs as their spacers is sufficient to prevent the restacking. Furthermore, by injecting CNTs, the intra-pores for the electrolyte are generated (Zhang et al., 2013b). However, from an industrial point of view, such a sophisticated approach might be too expensive.
Another way of obtaining a good composite is by adding a conducting polymer to the carbon material. It has been confirmed that mixing PANI with AC (Wang et al., 2019) or PEDOT and CNTs (Dettlaff et al., 2018) results in a higher specific capacitance of carbon-conductive polymer materials than individual components separately. Also, mixing metal oxides with carbonaceous materials develops a specific capacitance. Synthesis of CNTs and an MnO2 hybrid (Dan et al., 2020) or graphene and MnO2 (Cheng et al., 2011) results in improved conductivity, which affects the electrochemical properties of the capacitor. Creating hybrid materials has many advantages. However, they do not significantly improve the properties of electrode materials and, unfortunately, it increases production price (Borenstein et al., 2017).
Nanostructured materials can be divided into four general groups: (1) 0D zero-dimensional nanomaterials (nanoparticles, nanospheres); (2) 1D one-dimensional nanomaterials (nanorods, nanowires); (3) 2D two-dimensional nanomaterials (nanoplates); and (4) 3D three-dimensional nanomaterials. Nanosized particles that have their length and width within the nanometer range (<100 nm) belong to 0D (1). All dimensions (length, width, and height) are presented by one parameter, e.g., radius. In 1D nanomaterials (2), one dimension is outside the nanoscale, in 2D nanomaterials (3) two dimensions are outside the nanoscale. 3D nanomaterials (4) are materials that are not confined to the nanoscale in any dimension.
Currently, three-dimensional (3D) carbon materials are becoming a promising alternative to AC for ECs application. They can be used both as an active material and as supporting scaffolds for other pseudocapacitive materials with relatively limited electrical conductivity (Wang et al., 2013a; Cao et al., 2014a; Zhang et al., 2014). 3D materials, if used in ECs as electrodes, should have strictly designed porosity, surface, density, wettability, flexibility, etc. Such structures are intended to provide primarily high conductivity and to control the distribution of heteroatoms. The unique pore network (e.g., hierarchical porous structures) reduces dead volume (the volume of the electrode that the ions cannot access). Such solutions ensure the rapid diffusion of ions from the electrolyte bulk to the electrode surface and efficient charge accumulation (Suss et al., 2013; Song et al., 2018). Quite often, electrodes based on 3D materials do not require any additives or binders to prepare the electrodes, while maintaining high flexibility. However, one should be aware that these architectures are not suitable for every type of energy storage/conversion device.
It is accepted that 3D materials construction should perfectly match the device parameters and their work conditions (like the device dimensions, type of electrolyte, temperature, pressure, or susceptibility to disruptions) to ensure good performance. Some advantages and disadvantages of different 3D structures are presented in Scheme 1.
It has to be pointed out that there are some incorrect definitions of 3D materials in the literature. For instance, ACs should not be bracketed within 3D materials. Activated carbons have, indeed, a highly developed porosity and surface, however, their structure is disordered – there are no repetitive elements. Furthermore, ACs are not ideal for a new generation of ECs because of three major disadvantages:
(1) Quite often, during electrode preparation, electrically insulating binders need to be added; that increases the total weight of the device (so-called dead mass) and hinders the transport of ions.
(2) Micropores in ACs make up about 90% of its porosity (Simon and Gogotsi, 2013; Fic et al., 2018). Such a large number of micropores hinders ion diffusion in pores and limits the capacitance at ultra-fast charging conditions (especially for pores with a diameter smaller than ion size) (He et al., 2016).
(3) Disordered pore distribution leads to a tortuous and stochastic porous network, which also reduces the rate of ion diffusion (Zhu et al., 2016).
Synthesis of materials based on the template method appears to be the easiest way to create 3D architectures. The matrix can be a permanent scaffold for a 3D electrode, and it participates in the creation of the electrode. There are also methods where the matrix acts only in a transitional stage (schematic illustration presented in Figure 5).
Figure 5. Schematic illustration of template-based 3D materials. SEM pictures adapted from Ahn et al. (2019), Ussia et al. (2019), Zhou (2019), and Wang et al. (2020) under the Creative Commons CC BY license.
After applying the proper electrode material and its anastomosis with the active material, the matrix is removed, and the created 3D electrode material resembles its shape. Removing the matrix allows the highly developed surface area electrode to be formed. However, in many cases, the presence of the matrix ensures the entire structure stability. Examples of active materials include metal oxides and hydroxides, which provide high gravimetric/volumetric capacity in hybrid storage systems. The matrix method uses such processes as: (1) a chemical vapor deposition (CVD), (2) a hydrothermal reaction, or (3) electrodeposition. There are three main types of templates: (1) nickel foams, (2) silica matrixes, or (3) polystyrene (PS) spheres.
Nickel foam (NF) is made of a conductive, connected framework of pure nickel. The pore density of such a skeleton varies between 50 and 90%, and the conductivity ∼350 S⋅cm–1. Electrodes manufactured based on a continuous NF network usually adopt a monolithic structure. Popular active materials include: metal oxides (Yan D. et al., 2012; Chen et al., 2013; Zhou C. et al., 2013; Xu et al., 2014; Zhai et al., 2015), sulfurs (Pu et al., 2013; Wei et al., 2014), hydroxides (Guan et al., 2011; Yuan et al., 2014; Qu et al., 2015), and their composites (Guan et al., 2011; Tang et al., 2013; Cai D. et al., 2014). Incorporation of metal oxides to the nickel skeleton can be realized by electrochemical reduction and electrosorption (Zhang et al., 2014). Besides, the nickel susceptibility makes the improvement of nickel oxides or hydroxides deposition possible (Cai S. et al., 2014; Xiong et al., 2015). It is worth mentioning that intermediate elements are also used as the additive to the matrix and active compounds. The most common additives are graphene coatings applied to NF. In turn, the active material is applied to the graphene layer. Graphene growth is realized by chemical vapor deposition (CVD; Zongping et al., 2011; Ji et al., 2012; Chen M. et al., 2014; Liu et al., 2014; Patil et al., 2014; Zhang et al., 2014) or by an electrochemical method of dissolving and reducing graphene oxide (product – ERGO material). In the CVD technique, the graphene layer is deposited in the atmosphere CH4/H2/Ar (∼ 1000°C) (Zhang et al., 2014) on NF, and then impregnated by, for example, poly(methyl methacrylate) (PMMA). The use of PMMA protects the graphene layer from damage when the NF is removed. Poly(methyl methacrylate) dissolves, and poly(dimethylsiloxane) (PDSM) is introduced into the graphene framework. There is an increase in strength and flexibility of such a network (Zongping et al., 2011). Finally, the templates are selectively removed (e.g., by FeCl3-HCl or 6 mol⋅L–1 HCl) to create a 3D graphene architecture. There are also hybrid solutions using CNTs, which serve as a conductive carrier but also increase the surface area of the metal oxides/hydroxides used. The alternative is the hydrothermal reduction of graphene oxide suspension (Luan et al., 2013). This technique does not require the use of PMMA impregnation (Zhai et al., 2015). Nickel and cobalt-based particles are then deposited on graphene foam to catalyze CNT growth. Then a dense CNT layer is grown on both the outer and inner spaces of each empty graphene wall (Liu et al., 2014).
Silica templates have different morphologies: (1) nanoparticles (Pan et al., 2012), (2) nanosheets (Nakanishi et al., 2013), and (3) ordered mesoporous/macroporous structures (Huang et al., 2012). This method is usually accompanied by high-temperature treatment or a strongly acidic environment (Lei et al., 2011). The use of these templates is mainly limited to carbonization of organic precursors to produce porous carbon networks (e.g., ordered mesoporous carbon) or for the preparation of materials that are stable against strong alkalis (Lee et al., 2000; Ryoo et al., 2001; Lee et al., 2004). Silica particles, used as templates, are firstly dispersed in the reaction solution. Then the material growth process takes place on the dispersed silica template. The general preparation route may consist of three stages: (1) impregnation of the template with the active material, (2) use of pyrolysis/crystallization/sublimation to obtain the desired product with the desired crystallinity, and (3) removal of the silica matrix (HF etching or hot etching with, e.g., LiOH, KOH) (Lu et al., 2014a; Zhang et al., 2017). The material is activated by KOH treatment. The produced porous electrode material is applied onto the foil (e.g., Cu, Al) and used as a current collector. The separated nature of the material hinders the capacitive performance of the EC without the use of a foil. Despite the monodisperse features of silica particles, this technique is also known for obtaining a continuous 3D structure by combining silica particles into a colloidal 3D crystal (Kuai et al., 2003; Wong et al., 2003). This technique involves matrix colloid precipitation under external forces (gravity, capillary forces, electrostatic forces, and hydrophobic interactions on the oil–water interface) (Liu Y. et al., 2013). After creating the connections between the particles, a 3D network is obtained, and the active material can be placed in the porous space. However, the scale of grain connection is still smaller than in the case of NF, due to the small size of the silica grains. This method is still expensive; therefore it is used as an auxiliary tool for integrating mesopores into a macroporous backbone (e.g., graphene foam or aerogel) for the construction of bimodal or tri-modal porous 3D frames (Lu et al., 2011; Huang et al., 2012). Composite-based 3D electrode templates (e.g., a combination of GA graphene aerogel and a SiO2 mesoporous silica layer) are obtained with this approach. It is also possible to insert silica into a macroporous structure to increase its strength and to ensure the hierarchical structure. An example of such a material is highly conductive graphite. Between graphene layers, mesoporous carbon spheres are located, which are obtained as a result of the deposition of carbonaceous material in silica spheres. In the next stage, the carbon spheres are removed (leaving an ordered mesoporous carbon structure). In this case, graphene provides high conductivity, while incorporated carbon spheres play the role of conductive spacers (Lu et al., 2011).
3D structures obtained with polymer templates include two manufacturing strategies: (1) converting colloids into 3D colloidal crystals before forming a matrix (Zhou M. et al., 2013), and (2) self-assembly of such templates with other materials (e.g., graphene) to generate additional porosity (Liu et al., 2009). Transfer methods to a conductive substrate include:
(1) dropping polymer colloids on the lateral surface of the substrate along with further evaporation (Müller et al., 2000),
(2) deposition of the polymer on a vertically suspended colloidal polymer substrate by solvent evaporation (Bartlett et al., 2000; Karuturi et al., 2012),
(3) a dipping method in which the substrate is withdrawn from a colloidal polymer solution at a constant speed and angle (Xia et al., 2010; Elias et al., 2013; Hsia et al., 2014),
(4) electrophoretic deposition of polymer spheres on a conductive substrate (Yamaguchi et al., 2009).
Colloidal 3D crystals preparation usually takes more time compared to procedures that directly integrate polymer spheres into macroporous matrices. The most popular matrix is PS template, but polyurethane sponges (PU) might be considered as well.
Metal–organic frameworks (MOFs) or skeletons enable much greater flexibility in the design of 3D electrodes. In the case of the previously mentioned templates, a higher-order structure was used to produce a lower-order structure. On the contrary, the MOF structure is made up of microscopic particles with geometric symmetry and places that coordinate metal ions, thus making the 3D structure design more flexible (Li and Xu, 2013). Until now, their usage was limited to fulfilling the function of precursors/matrices for carbons (Salunkhe et al., 2015; Wang et al., 2016) or as composites with conductive additives such as graphene (Choi et al., 2014). Recent research results propose solutions for using MOF as pure active substances without the use of a binding agent (Sheberla et al., 2016). The ECs’ capacitance based on MOFs quite often exceeds the capacitance of devices operating with carbon electrodes. This might originate from the well-developed specific surface area; however, one should pay special attention to the absolute values reported from the nitrogen adsorption technique (Farha et al., 2012). The conductivity of such materials reaches similar values as graphene (5000 S⋅m–1), significantly exceeding the conductivity of AC and graphite (Yuxi et al., 2014). The conditions for producing MOFs are much milder than those used in the preparation of graphite carbons (John et al., 2015).
Other materials used as templates include macroscopic skeletons (Lang et al., 2011, 2012; Liu N. et al., 2013; He et al., 2014) and molecular templates (Meng et al., 2013) combined with self-organizing graphene. Among them, there are structures with an active layer embedded on them in graphene, which in turn is an electron-conducting path (Liu N. et al., 2013). Hybrid 3D electrodes are another type (Lang et al., 2011). Molecular templates (e.g., CaCO3) are used as supports in the self-assembly of graphene or as forms for the design of hollow structures (similar to silica and polymer templates) (Meng et al., 2013).
The unconventional methods for obtaining electrodes are an alternative to the template methods that allow many of the disadvantages (associated with the first production method) to be eliminated (Figure 6). Obtaining 3D architectures involves:
Figure 6. Schematic illustration of non-template-based 3D materials. SEM pictures adapted from Hayashi and Hakuta (2010), Deng et al. (2015), Tomczykowa and Plonska-Brzezinska (2019), and He et al. (2020) under the Creative Commons CC BY license.
(1) self-organization of low-dimensional monomers,
(2) multi-stage assembly of hierarchical structures, and
(3) processing of natural 3D precursors.
Thanks to their irreplaceable properties, carbon materials are commonly used for electrode preparation in ECs application. Thus, this type of material is also very often considered for 3D electrodes production.
Graphene has a unique lamellar structure. Graphene is a type of single-layer carbon with sp2 hybridization that allows the electron mobility to be high. Moreover, it is characterized by its high mechanical strength and large available surface area (2600 m2⋅g–1). Graphene is a component of graphite, where many units of graphene are arranged in parallel. Graphite was initially considered as a potential component of capacitor electrodes due to the distance between the graphene layers, which is 0.354 nm. Such a distance ensures the free movement of ions in the material. This also enables the formation of ionic or covalent intercalated graphite compounds (GICs; Liu J. et al., 2013; Abdelkader et al., 2015) as a result of the exfoliation process. The most critical stages of material exfoliation are GIC formation and volumetric expansion (Wang G. et al., 2011; Wang J. et al., 2011; Wei et al., 2012; Parvez et al., 2014). GIC formation can be performed with chemical exfoliation – by oxidation of natural graphite powder with strong oxidants or by electrochemical exfoliation. The most cost-effective technique of exfoliation is the second one, because of its low equipment requirements and the lack of strong acids and oxidants. Moreover, it has a limited impact on the environment that can be an additional advantage (Yenny et al., 2008; Abdelkader et al., 2015; Yang et al., 2016). However, the degree of exfoliation should be carefully monitored. Too high a degree breaks the graphite into small pieces of graphene, which reduces time efficiency and increases production costs but also worsens the internal continuity and conductivity of the 3D graphite network. To meet those requirements, different strategies for partial electrochemical exfoliation, without damaging the structural integrity of graphite, were developed (Song et al., 2014; Song et al., 2015b). Such exfoliation consists of intense gas evolution followed by ion intercalation. The upper graphite layers are exfoliated in aqueous solution by the evolution of gas. Then the ions (e.g., nitrate ions) are intercalated through the upper layers under positive voltage to further exfoliate the graphite layers underneath (Song et al., 2016). Bent graphene layers can also be obtained from graphene oxide (GO) dispersion by direct drying or spray drying (Wen et al., 2012; Yan J. et al., 2012; Yoon et al., 2013). Under these conditions, graphene sheets tend to create a strongly crumpled surface with a large pore volume, which prevents their agglomeration. Stabilization of GO sheets is carried out in an aqueous environment (hydrothermal method), which is associated with a balance of van der Waals forces between GO sheets and electrostatic repulsion of hydrophilic oxygen groups on the edges of these sheets. The self-organization of sheets is possible when the balance of forces is broken. Chemical doping with effective atoms is performed to improve charge density and electrical conductivity. Moreover, this solution introduces additional pseudocapacitance in the system. The most common additives are nitrogen (Wu et al., 2012), sulfur (Zhang et al., 2013a), phosphorus (Some et al., 2012; Zhang et al., 2013a), boron (Martins et al., 2007; Wu et al., 2012), double Ni-Co hydroxide (Song et al., 2015a), PANI (Geng et al., 2017; Ye et al., 2017), and tungsten trioxide (Yu et al., 2015b).
Filtration is another common method for constructing 3D graphene from GO dispersion. In this situation, graphene appears macroscopically as thin paper (Dmitriy et al., 2007; Chen et al., 2008; Yang et al., 2011). The main problem in these processes is easy aggregation and re-arranging of graphene sheets into a graphite structure. The reason for this phenomenon is the interaction between p–p stacks and van der Waals attraction between their underlying planes. Aggregation hinders the diffusion of ions into the space of densely packed graphene sheets. To solve this problem, spacers in the form of CNTs (Fan et al., 2010; Du et al., 2011; You et al., 2013), metal oxides (Shao et al., 2010; Xie et al., 2013; Cao et al., 2014b), polymers (Meng et al., 2013; Zhang et al., 2013d), and others (Gong et al., 2014) can be implemented. Another solution is the use of intercalation ions in the graphite (e.g., Li+). Graphene layers fold under the influence of these ions. After that, ions, e.g., ClO4,– are introduced. After immersion of such an electrode in water, hydroxylate and carbonyl groups are integrated on the corrugated surface of graphene.
Carbon cloth (CC; also called tissue or textile) can play a dual function. In some cases, it plays the role of a matrix material for other structures, but it can also exist as an individual electrode material. However, it requires previous processing due to its low capacitance (∼ 1 F⋅g–1) and surface area (∼ 5 m2⋅g–1) (Wang et al., 2015) at the initial production step. Activation involves chemical oxidation and a two-stage reduction with hydrazine and ammonia vapors (Liu T. et al., 2015). This leads to increased fiber roughness and reduced resistance. The capacitance of such a material increases significantly. Electrochemical peeling can also be used for CC activation (Song et al., 2018). Carbon cloth is treated with H2SO4-HNO3 mixture with a positive bias of 3.0 V (Liu T. et al., 2015). There is also an acid-free method that includes peeling, oxidation, and reduction steps (Song et al., 2017). In the first stage, the material is exfoliated by NO ions. Then CC is oxidized by NO3–GIC hydrolysis. Reductions of oxygen content are carried out in 0.1% aqueous hydrate solution. The material after this process has a higher conductivity. Such a structure can also buffer the volumetric deformation of the electrode during charge/discharge processes, thereby increasing the life of the device. The combination of matrix fabric with several compounds results in promising pseudocapacitive composite electrodes. The applied compounds include PPy (Huang et al., 2015), MnO2 (Feng et al., 2016), TiN (Lu et al., 2012; Lu et al., 2014a), VN (Lu et al., 2013), V6O13–x (Zhai et al., 2014), V6O13 (Wang et al., 2012b), and Fe2O3 (Liu T. et al., 2015).
The CNTs are porous tubular structures. There are mainly two strategies for producing 3D CNT based electrodes: using CNT as “wires” for weaving into 3D architecture (Niu et al., 2012), and direct deposition of active substances on the CNT surface (e.g., manganese oxide and PANI) (Kohlmeyer et al., 2011). Intrinsically existing CNT structures are not a promising material; however, their combination with pseudocapacitive materials allows the unique properties of the composite material to be obtained (Chen et al., 2009, 2011; Zhang et al., 2011; Chen et al., 2012). Twisted graphene sheets (in the form of a horn) can also be seen as structures belonging to the CNT group. These structures are also characterized by high self-assembly into three-dimensional balls with diameters below 100 nm (Yang C.-M. et al., 2007; Azami et al., 2008; Izadi-Najafabadi et al., 2011; Zhang et al., 2011; Deshmukh and Shelke, 2013; Jung et al., 2013). Modifications of these materials (in the form of open cones) demonstrate higher capacitance in the double-layer compared to classic CNTs (Yang C.-M. et al., 2007). Nanocorns, in combination with pseudocapacitive materials, also show high capacitance.
Hierarchical carbon materials are described as materials with well-developed micro-, meso-, and macro-porous structure. Micropores have a significant contribution to energy storage. Mesopores and macropores are channels that distribute ions to the micropores in the electrode. This group of materials includes porous graphite carbons (HPGC) (Wang et al., 2008) and carbon aerogels (CA; Miller and Dunn, 1999; Li et al., 2006a,b; Hwang and Hyun, 2007; An et al., 2010; Chien et al., 2012) obtained from the pyrolysis of polymeric materials. Also, in the case of these materials, there are examples of composites with pseudocapacitive materials, such as nitrogen atoms (Li et al., 2012; Dhawale et al., 2013; Hou et al., 2015; Wei et al., 2016), MnO2 (Li et al., 2006a; Chien et al., 2012), NiCo2O4 (Chien et al., 2012), SnO2 (Hwang and Hyun, 2007), and PPy (An et al., 2010). The synthesis process consists of three main stages (Zhang et al., 2016): (1) mixing the carbon precursor (e.g., chitosan) and the blowing agent (e.g., K2CO3), (2) gelation (e.g., with glutaraldehyde), (3) lyophilization, and (4) annealing (∼ 600°C). Processes (2) and (3) enhance carbon sheets into a porous structure. Step (4), in turn, generates a meso- and microporous structure.
Organic carbide (biocarbon) is also a key material for the production of 3D architecture (Kalpana et al., 2009; Lv et al., 2012; Biswal et al., 2013; He et al., 2013; Yun et al., 2013; Jin et al., 2014; Genovese et al., 2015). This type of material is usually obtained by pyrolysis. Activation is ensured by natural compounds found in the precursor itself (Biswal et al., 2013). The pyrolysis process and exfoliation through thermal rinsing allows the amorphous environment to be removed, and, as a result, the material takes the form of thin sheets of carbon. Exfoliated biocarbon is also functionalized by oxygen groups that enables a high capacitance. Generating 3D structures from natural materials and waste is one of the most desirable solutions.
Metal oxides such as MnO2 (Yu et al., 2013; Jin et al., 2014), NiO (Liang et al., 2012; Xia et al., 2012), Co3O4 (Zhang et al., 2013c; Guan et al., 2014; Xia et al., 2014a), VOx (Wang et al., 2012b; Zhai et al., 2014), and Fe2O3 (Lu et al., 2014b) are materials that might be claimed as promising for hybrid storage mechanisms. They provide higher specific capacity values than carbon materials, which further increases the EC energy density (Lu et al., 2014b). The reason for that is their ability to store energy via the electrical double-layer formation and charge accumulation, but also through reversible Faradaic reactions. The methods of constructing 3D metal oxide electrodes can be briefly divided into one-stage and multi-stage assembly.
One-stage assembly. The self-organization of three-dimensional metal oxides is based on crystal growth. Popular methods of synthesis of metal oxide crystals include:
(1) electrochemical deposition,
(2) the hydrothermal method, and
(3) the solvothermal method (Liang et al., 2012; Xie et al., 2013; Zhu J. et al., 2013).
The controlled production of 3D architectures is accomplished by selecting conditions during the growth of metal oxide crystals. The hydrothermal method allows for adjusting the synthesis of nanocrystalline oxides by choosing the reaction time, precursors, temperature, and additives.
Multi-stage assembly. The multi-stage assembly of 3D material production is based on the production of low-dimensional skeletal material, followed by the embedding of branched material. Often ZnO is used as the basis (Sun et al., 2013) because of the well-known synthesis of this material. In addition, there are also Co3O4 (Liu et al., 2011), SnO2 (Yan et al., 2011), CuO (Yu and Thomas, 2014), and MnMoO4 (Mai et al., 2011) skeletons. In the next stage, a thin layer of branched MnO2 (Liu et al., 2011; Sun et al., 2013), NiO (Xia et al., 2012), V2O5 (Yan et al., 2011), NiOH (Guan et al., 2011), Fe2O3 (Wu et al., 2013), and CoMnO4 (Mai et al., 2011) is deposited on the skeleton while maintaining skeletal morphology (Liu et al., 2012; Xia et al., 2012). Through the use of synergistic effects from capacitance, the 3D structure usually provides favorable electrochemical parameters.
Other materials. This group of materials also belongs to conductive polymers like PANI (Fan et al., 2007; Meng et al., 2014; Yu et al., 2014), PPy, PEDOT, and poly (styrene sulfonate) PEDOT-PSS (Cuentas Gallegos and Rincón, 2006). The 3D material is produced by the self-assembly of small-sized nanostructures (Cuentas Gallegos and Rincón, 2006; Yu et al., 2014). Typical preparation methods are mainly divided into electrochemical polymerization (Fan et al., 2007; Meng et al., 2014; Yu et al., 2014) and direct chemical polymerization (Cuentas Gallegos and Rincón, 2006; Antiohos et al., 2011). Polymer electrodes are characterized by their high extensibility and very low capacitance decrease in repeated bending, and there are also premises on the materials based on nitrides (Lu et al., 2012; Balogun et al., 2015; Yu et al., 2015a), sulfides (Xia et al., 2014b; Xiao et al., 2014), and metal selenides (Wang et al., 2013b).
This method allows the highest degree of control of the porous structure to be formed. 3D printing techniques, such as direct ink writing (DIW), are becoming powerful material engineering tools (Ambrosi and Pumera, 2016). In this case, the moving nozzle forming the material is regulated by computer software based on a previously planned computer-generated project (Lewis, 2006). The extruded material, in the form of a liquid or semi-liquid, creates 3D architecture layer by layer. Requirements for DIW inks are their continuity and resistance to collapse, expansion, and sagging. To meet these requirements, the printing materials must be shear thinned fluids: the viscosity of the fluid should drastically decrease under shear and recover quickly after removing the force. The surface area, electrical conductivity, and capacitance are controlled by the proportion of individual components of such an ink. An example of DIW is a material based on graphene aerogel (Zhu et al., 2016). The ink contains graphene oxide [a graphene aerogel precursor (Worsley et al., 2010)], graphene nanoplates (electrically conductive additive), resorcinol-formaldehyde resin (gelation catalyst), and fumed silica (they serve as both a porogen and a viscosity enhancer) (Figure 7). The print consists of alternately applied aerogel gird structures.
Thousands of solutions have still not allowed us to construct the perfect energy storage device that provides both high power and energy density. Looking ahead, there are still challenges to be solved. In particular, 3D carbons and their composites have great application potential. These structures can be successfully implemented in energy storage devices as a new generation of high-power electrodes at high mass loading, breaking the energy and power limits of recent systems. However, these technologies are not versatile, and, above all, they suffer from complicated manufacturing processes and quite often very high prices. Further work is needed to understand their chemistry and charge storage mechanisms.
It is also necessary to improve the electrical conductivity of these materials. Separating ion transport and accumulation from electrons in different phases of the composite could offer a unique interfacial storage mechanism, which would dominate the internal limitations of traditional electrode materials. For electrode materials – their flexibility plays a significant role. This property not only allows for using these materials in devices subjected to bending but also extends their lifetime. Repeated charge and discharge processes result in volumetric changes of the electrodes. In the case of traditional electrode materials, the performance of such a device decreases over time as a result of micro-cracks arising and an increase in the resistance to charge transfer at the electrode/electrolyte interface. 3D structures significantly limit this adverse effect. Porous 3D conductive scaffoldings offer interpenetrating electron and ion transport paths that ensure very efficient charge delivery to and from electrodes. These transport routes are necessary to translate the extraordinary performance achieved through nanoscale materials into macroscopic electrodes. The ability to increase the mass loading of active materials, without compromising charge (energy) storage efficiency, is required not only to capture the benefits of a new generation of high-performance electrode materials in practice but also contributes to crossing the border of traditional electrode materials by reducing the dead volume of the electrode or the number of passive materials. These improvements can increase the overall energy and power density.
Template methods allow us to control the morphology of the pores in the final structure because the electrodes inherit the macroscopic shape of the template. However, these techniques might be ineffective at generating large scale and well-ordered micro- and mesoporous structures that have a much more pronounced effect on capacitive performance than macropores. The solutions proposed to solve this problem relate to the combination of chemical activation on hard matrices. Micro- and meso-structures generated by activation, together with macropores formation by removing the matrix, create hierarchical porous structures that can increase the surface area available for ions, while facilitating fast ions diffusion. Another more straightforward solution is to use templates with pore sizes in the range of mesopores and micropores. Besides, the use of interactions (e.g., electrostatic attraction, complexation) between the matrix and the deposited material would be crucial in the implementation of a uniform meso- and microporous dispersion. However, the thickness of the carbon film on the matrix should be taken into account. If the coated carbon material is too thin, structural integrity may not be maintained during the matrix removal process.
Like the matrix technique, the DIW technique works in the engineering of size and distribution of macropores but shows little efficiency in the case of meso- and micropores. Improving print resolution through the joint efforts of industry and the academic community is desirable to transform DIW into a more efficient electrode production tool. Besides, the ink must be varied by incorporating more electrochemically active materials. For example, converting biomass precursors used in the template-free method into print inks could be a promising direction for future research.
In addition to the morphology itself, controlling the conditions of these materials’ production plays an important role. For example, the degree of exfoliation should be strictly controlled because it is an essential parameter for structural integrity as well as for electrochemical performance. Currently, the control of the degree of exfoliation is still insufficient. There is no effective approach to the controlled conversion of entire loose structures into porous, connected 3D networks. Potential in this area is derived from two-stage exfoliation, which is a combination of severe and then mild exfoliation. The first stage is designed to reveal the internal space through deep exfoliation and the second stage, in turn, can further fine-tune the degree of exfoliation without destroying the monolithic 3D network. However, this technology is not fully understood and requires further research. It has to be mentioned here that 3D architectures usually demonstrate high (or at least satisfactory) gravimetric capacitance values. However, their porosity makes the volumetric capacitance rather moderate. This might be another aspect that requires further development, as for portable electronics the volume required for an energy storage system is quite often very limited. It also raises the question of the right performance metrics to be reported that allows for fair comparison between materials (Balducci et al., 2017). In our opinion, both characteristics should be presented – or at least the data that allows to calculate it should be provided.
Moreover, very sophisticated 3D structures might be destroyed during electrode material preparation; hence, the future of this group is foreseen for self-assembled structures and techniques that produce the electrodes “ready-to-use,” similar to carbon monoliths or CCs. This seems to be the only way to save the 3D architecture in the final electrode material.
Nowadays, organic materials have become a fashionable trend. In addition to the limited or complete absence of negative effects on the environment, electrode materials based on them are characterized by a simple production scheme. However, the unpredictable behavior of structural evolution during pyrolysis under various conditions (time, temperature, heating speed, etc.) justifies the difficulty of controlling the size of the pores, their distribution, and capacitance. That is why it is so important to know the mechanistic evolution of the structure during pyrolysis under given annealing conditions, to improve the controllability of this method. An indispensable element is also the repeatability of obtaining 3D material. The composition of the biomass precursors of subsequent batches will always vary, which is related to their origin, time, and processing technique. It imposes the need to modify the conditions of biomass processing into the final electrode product.
To ensure the continuous development of electrode materials, it is also necessary to provide various research techniques, including primarily high-resolution TEM in situ, atomic force microscopy in situ, X-ray spectroscopy in situ, NMR in situ spectroscopy and cryo-electron and transmission microscopy. Consequently, it will make it possible to visualize electrochemical processes in real-time and will finally allow for the electrochemical mechanisms to be described in detail. This can help to formulate guiding principles for material development and electrode design.
Every year, energy-related devices are increasingly subjected to safety regulations. When the energy is released accidentally, in an uncontrolled and cascading way, it results in temperature rises and disastrous consequences. Interest should be focused on developing compatible electrolytes and separators with high thermal stability, fully compatible with the proposed structures and compounds, which can further prevent overheating, internal short circuits, fires or explosions. In the research world, the cell and case design are very often neglected. However, when one thinks about system commercialization, it is necessary to develop new solutions strictly designed for the “heart” of the device (electrodes, electrolyte). When designing commercial, poorly understood 3D electrode structures, all inconveniences should be taken into account from the very beginning, to prevent them from hampering cell construction and from being hazardous both to the user and the environment.
The theoretical considerations supported by molecular simulations are also an indispensable element. They play an irreplaceable role in understanding the mechanisms of charge storage and the synthesis-structure-property relationship associated with 3D electrodes. The theory can support experiments and create a basis for further studies. An optimally planned experimental route reduces both research time and costs.
In this article, recent progress in the use of 3D carbon nanostructures as electrode materials for advanced capacitor devices has been compiled. The review begins with a comprehensive discussion of common electrode materials used in energy storage devices and ends with an updated review and division of carbon-based 3D architectures. Particular attention was paid to clarifying the definition of 3D materials and errors in the use of this definition in the literature. The basic division of these structures has been done based on the technology of their manufacture. This review summarizes three basic groups: materials created based on matrices, matrix-less materials, and materials produced using 3D printing. Due to the structural interconnection of carbon-based 3D nanostructures, they not only create hierarchical porous channels but also have a higher electrical conductivity and mechanical stability than commonly used materials. A rational combination of connected meso- and micropores in electrode materials significantly improves electrochemical parameters. Significant progress has been made so far, but a full understanding of the relationship between 3D carbon nanostructures and improved electrochemical performance is still unclear. There is great potential in the design and synthesis of new 3D materials for advanced ECs with high energy and power density as well as a long life. The road for creating the ideal energy storage device is still very long, but the reasonable development of the proposed structures could bring the goal a bit closer.
All authors listed have made a substantial, direct and intellectual contribution to the work, and approved it for publication.
The European Commission, in the form of The European Research Council, is acknowledged for its financial support within the Starting Grant Project (GA 759603) under the European Union’s Horizon 2020 Research and Innovation Programme.
The authors declare that the research was conducted in the absence of any commercial or financial relationships that could be construed as a potential conflict of interest.
Abdelkader, A. M., Cooper, A. J., Dryfe, R. A. W., and Kinloch, I. A. (2015). How to get between the sheets: a review of recent works on the electrochemical exfoliation of graphene materials from bulk graphite. Nanoscale 7, 6944–6956. doi: 10.1039/C4NR06942K
Ahn, J., Ahn, C., Jeon, S., and Park, J. (2019). Atomic layer deposition of inorganic thin films on 3D polymer nanonetworks. Appl. Sci. 9:1990. doi: 10.3390/app9101990
Alexandru, V., and Andrea, B. (2017). Supercapacitors: porous materials get energized. Nat. Mater. 16:161. doi: 10.1038/nmat4851
Alola, A. A., Bekun, F. V., and Sarkodie, S. A. (2019). Dynamic impact of trade policy, economic growth, fertility rate, renewable and non-renewable energy consumption on ecological footprint in Europe. Sci. Total Environ. 685, 702–709. doi: 10.1016/j.scitotenv.2019.05.139
Ambrosi, A., and Pumera, M. (2016). 3D-printing technologies for electrochemical applications. Chem. Soc. Rev. 45, 2740–2755. doi: 10.1039/C5CS00714C
Amir, F. Z., Pham, V. H., Mullinax, D. W., and Dickerson, J. H. (2016). Enhanced performance of HRGO-RuO2 solid state flexible supercapacitors fabricated by electrophoretic deposition. Carbon 107, 338–343. doi: 10.1016/j.carbon.2016.06.013
An, H., Wang, Y., Wang, X., Zheng, L., Wang, X., Yi, L., et al. (2010). Polypyrrole/carbon aerogel composite materials for supercapacitor. J. Power Sources 195, 6964–6969. doi: 10.1016/j.jpowsour.2010.04.074
Antiohos, D., Folkes, G., Sherrell, P., Ashraf, S., Wallace, G. G., Aitchison, P., et al. (2011). Compositional effects of PEDOT-PSS/single walled carbon nanotube films on supercapacitor device performance. J. Mater. Chem. 21:15987. doi: 10.1039/c1jm12986d
Augustyn, V., Simon, P., and Dunn, B. (2014). Pseudocapacitive oxide materials for high-rate electrochemical energy storage. Energy Environ. Sci. 7, 1597–1614. doi: 10.1039/c3ee44164d
Azami, T., Kasuya, D., Yuge, R., Yudasaka, M., Iijima, S., Yoshitake, T., et al. (2008). Large-Scale Production of Single-Wall Carbon Nanohorns with High Purity. J. Phys. Chem. C 112, 1330–1334. doi: 10.1021/jp076365o
Balducci, A., Belanger, D., Brousse, T., Long, J. W., and Sugimoto, W. (2017). A Guideline for Reporting Performance Metrics with Electrochemical Capacitors: From Electrode Materials to Full Devices. J. Electrochem. Soc. 164, A1487–A1488. doi: 10.1149/2.0851707jes
Balogun, M.-S., Qiu, W., Wang, W., Fang, P., Lu, X., and Tong, Y. (2015). Recent advances in metal nitrides as high-performance electrode materials for energy storage devices. J. Mater. Chem. A 3, 1364–1387. doi: 10.1039/C4TA05565A
Barai, A., Chouchelamane, G. H., Guo, Y., Mcgordon, A., and Jennings, P. (2015). A study on the impact of lithium-ion cell relaxation on electrochemical impedance spectroscopy. J. Power Sources 280, 74–80. doi: 10.1016/j.jpowsour.2015.01.097
Bartlett, P. N., Birkin, P. R., and Ghanem, M. A. (2000). Electrochemical deposition of macroporous platinum, palladium and cobalt films using polystyrene latex sphere templates. Chem. Commun. 17, 1671–1672. doi: 10.1039/b004398m
Béguin, F., and Fra̧ckowiak, E. (2009). Carbons for Electrochemical Energy Storage and Conversion Systems. New York, NY: CRC Press. doi: 10.1201/9781420055405
Béguin, F., and Fra̧ckowiak, E. (2013). Supercapacitors. Weinheim: Wiley-VCH Verlag GmbH & Co. KGaA. doi: 10.1002/9783527646661
Béguin, F., Presser, V., Balducci, A., and Frackowiak, E. (2014). Carbons and electrolytes for advanced supercapacitors. Adv. Mater. 26, 2219–2251. doi: 10.1002/adma.201304137
Bernardo, P., Dentzer, J., Gadiou, R., Märkle, W., Goers, D., Novák, P., et al. (2011). Influence of graphite surface properties on the first electrochemical lithium intercalation. Carbon 49, 4867–4876. doi: 10.1016/j.carbon.2011.07.007
Biswal, M., Banerjee, A., Deo, M., and Ogale, S. (2013). From dead leaves to high energy density supercapacitors. Energy Environ. Sci. 6, 1249–1259. doi: 10.1039/c3ee22325f
Borenstein, A., Hanna, O., Attias, R., Luski, S., Brousse, T., and Aurbach, D. (2017). Carbon-based composite materials for supercapacitor electrodes: a review. J. Mater. Chem. A 5, 12653–12672. doi: 10.1039/C7TA00863E
Budde-Meiwes, H., Drillkens, J., Lunz, B., Muennix, J., Lehner, S., Kowal, J., et al. (2013). A review of current automotive battery technology and future prospects. Proc. Inst. Mech. Eng. Part D 227, 761–776. doi: 10.1177/0954407013485567
Bujewska, P., Gorska, B., and Fic, K. (2019). Redox activity of selenocyanate anion in electrochemical capacitor application. Synthetic Metals 253, 62–72. doi: 10.1016/j.synthmet.2019.04.024
Cai, D., Huang, H., Wang, D., Liu, B., Wang, L., Liu, Y., et al. (2014). High-Performance Supercapacitor Electrode Based on the Unique ZnO@Co 3 O 4 Core/Shell Heterostructures on Nickel Foam. ACS Appl. Mater. Interf. 6, 15905–15912. doi: 10.1021/am5035494
Cai, S., Zhang, D., Shi, L., Xu, J., Zhang, L., Huang, L., et al. (2014). Porous NiMn oxide nanosheets in situ formed on nickel foam as 3D hierarchical monolith de-NO x catalysts. Nanoscale 6, 7346–7353. doi: 10.1039/C4NR00475B
Cao, X., Yin, Z., and Zhang, H. (2014a). Three-dimensional graphene materials: preparation, structures and application in supercapacitors. Energy Environ. Sci. 7, 1850–1865. doi: 10.1039/C4EE00050A
Cao, X., Zheng, B., Rui, X., Shi, W., Yan, Q., and Zhang, H. (2014b). Metal Oxide-Coated Three-Dimensional Graphene Prepared by the Use of Metal–Organic Frameworks as Precursors. Angewandte Chem. Int. Edn. 53, 1404–1409. doi: 10.1002/anie.201308013
Chen, H., Müller, M. B., Gilmore, K. J., Wallace, G. G., and Li, D. (2008). Mechanically Strong, Electrically Conductive, and Biocompatible Graphene Paper. Adv. Mater. 20, 3557–3561. doi: 10.1002/adma.200800757
Chen, M., Liu, J., Chao, D., Wang, J., Yin, J., Lin, J., et al. (2014). Porous α-Fe2O3 nanorods supported on carbon nanotubes-graphene foam as superior anode for lithium ion batteries. Nano Energy 9, 364–372. doi: 10.1016/j.nanoen.2014.08.011
Chen, W., Xia, C., and Alshareef, H. N. (2014). One-step electrodeposited nickel cobalt sulfide nanosheet arrays for high-performance asymmetric supercapacitors. ACS Nano 8:9531. doi: 10.1021/nn503814y
Chen, S.-E., Evanko, B., Wang, X., Vonlanthen, D., Ji, X., Stucky, G. D., et al. (2015). Design of aqueous redox-enhanced electrochemical capacitors with high specific energies and slow self-discharge. Nat. Commun. 6, 1–10. doi: 10.1038/ncomms8818
Chen, T.-L., and Elabd, Y. A. (2017). Hybrid-Capacitors with Polyaniline/Carbon Electrodes Fabricated via Simultaneous Electrospinning/Electrospraying. Electrochim. Acta 229, 65–72. doi: 10.1016/j.electacta.2017.01.140
Chen, Y., Qu, B., Hu, L., Xu, Z., Li, Q., and Wang, T. (2013). High-performance supercapacitor and lithium-ion battery based on 3D hierarchical NH 4 F-induced nickel cobaltate nanosheetnanowire cluster arrays as self-supported electrodes. Nanoscale 5, 9812–9820. doi: 10.1039/c3nr02972g
Chen, Z., Augustyn, V., Jia, X., Xiao, Q., Dunn, B., and Lu, Y. (2012). High-performance sodium-ion pseudocapacitors based on hierarchically porous nanowire composites. ACS Nano 6, 4319–4327. doi: 10.1021/nn300920e
Chen, Z., Augustyn, V., Wen, J., Zhang, Y., Shen, M., Dunn, B., et al. (2011). High-Performance Supercapacitors Based on Intertwined CNT/V2O5 Nanowire Nanocomposites. Adv. Mater.Adv. Mater. 23, 791–795. doi: 10.1002/adma.201003658
Chen, Z., Qin, Y., Weng, D., Xiao, Q., Peng, Y., Wang, X., et al. (2009). Design and Synthesis of Hierarchical Nanowire Composites for Electrochemical Energy Storage. Adv. Funct. Mater. 19, 3420–3426. doi: 10.1002/adfm.200900971
Cheng, Q., Tang, J., Ma, J., Zhang, H., Shinya, N., and Qin, L.-C. (2011). Graphene and nanostructured MnO 2 composite electrodes for supercapacitors. Carbon 49, 2917–2925. doi: 10.1016/j.carbon.2011.02.068
Chien, H. C., Cheng, W. Y., Wang, Y. H., and Lu, S. Y. (2012). Ultrahigh Specific Capacitances for Supercapacitors Achieved by Nickel Cobaltite/Carbon Aerogel Composites. Adv. Funct. Mater. 22, 5038–5043. doi: 10.1002/adfm.201201176
Choi, K. M., Jeong, H. M., Park, J. H., Zhang, Y.-B., and Kang, J. K. (2014). Supercapacitors of Nanocrystalline Metal À Organic Frameworks. ACS Nano 8, 7451–7457. doi: 10.1021/nn5027092
Choi, W., Choudhary, N., Han, G. H., Park, J., Akinwande, D., and Lee, Y. H. (2017). Recent development of two-dimensional transition metal dichalcogenides and their applications. Mater. Today 20, 116–130. doi: 10.1016/j.mattod.2016.10.002
Crosnier, O., Goubard-Bretesché, N., Buvat, G., Athouël, L., Douard, C., Lannelongue, P., et al. (2018). Polycationic oxides as potential electrode materials for aqueous-based electrochemical capacitors. Curr. Opin. Electrochem. 9, 87–94. doi: 10.1016/j.coelec.2018.05.005
Cuentas Gallegos, A. K., and Rincón, M. E. (2006). Carbon nanofiber and PEDOT-PSS bilayer systems as electrodes for symmetric and asymmetric electrochemical capacitor cells. J. Power Sour. 162, 743–747. doi: 10.1016/j.jpowsour.2006.06.085
Dall’agnese, Y., Lukatskaya, M. R., Cook, K. M., Taberna, P.-L., Gogotsi, Y., and Simon, P. (2014). High capacitance of surface-modified 2D titanium carbide in acidic electrolyte. Electrochem. Commun. 48, 118–122. doi: 10.1016/j.elecom.2014.09.002
Dall’agnese, Y., Rozier, P., Taberna, P.-L., Gogotsi, Y., and Simon, P. (2016). Capacitance of two-dimensional titanium carbide (MXene) and MXene/carbon nanotube composites in organic electrolytes. J. Power Sour. 306, 510–515. doi: 10.1016/j.jpowsour.2015.12.036
Dan, W., Xiubo, X., Yuping, Z., Dongmei, Z., Wei, D., Xiaoyu, Z., et al. (2020). MnO2/carbon composites for supercapacitor: synthesis and electrochemical performance. Front. Mater. 7:2. doi: 10.3389/fmats.2020.00002
Daniel, C. (2008). Materials and processing for lithium-ion batteries. JOM 60, 43–48. doi: 10.1007/s11837-008-0116-x
Deng, D., Zhang, L., Niu, T., Liu, H., and Zhang, H. (2015). Microstructures and Wear Performance of PTAW Deposited Ni-Based Coatings with Spherical Tungsten Carbide. Metals 5, 1984–1996. doi: 10.3390/met5041984
Deshmukh, A. B., and Shelke, M. V. (2013). Synthesis and electrochemical performance of a single walled carbon nanohornFe 3 O 4 nanocomposite supercapacitor electrode. RSC Adv. 3, 21390–21393. doi: 10.1039/C3RA43079K
Dettlaff, A., Das, P. R., Komsiyska, L., Osters, O., Łuczak, J., and Wilamowska-Zawłocka, M. (2018). Electrode materials for electrochemical capacitors based on poly(3,4 ethylenedioxythiophene) and functionalized multi-walled carbon nanotubes characterized in aqueous and aprotic electrolytes. Synthetic Metals 244, 80–91. doi: 10.1016/j.synthmet.2018.07.006
Dhawale, D. S., Mane, G. P., Joseph, S., Anand, C., Ariga, K., and Vinu, A. (2013). Enhanced Supercapacitor Performance of N-Doped Mesoporous Carbons Prepared from a Gelatin Biomolecule. ChemPhysChem 14, 1563–1569. doi: 10.1002/cphc.201300132
Dmitriy, A. D., Sasha, S., Eric, J. Z., Richard, D. P., Geoffrey, H. B. D., Guennadi, E., et al. (2007). Preparation and characterization of graphene oxide paper. Nature 448:457. doi: 10.1038/nature06016
Du, F., Yu, D., Dai, L., Ganguli, S., Varshney, V., and Roy, A. K. (2011). Preparation of Tunable 3D Pillared Carbon Nanotube–Graphene Networks for High-Performance Capacitance. Chem. Mater. 23, 4810–4816. doi: 10.1021/cm2021214
Eftekhari, A. (2017). Molybdenum diselenide (MoSe 2) for energy storage, catalysis, and optoelectronics. Appl. Mater. Today 8, 1–17. doi: 10.1016/j.apmt.2017.01.006
Elias, J., Utke, I., Yoon, S., Bechelany, M., Weidenkaff, A., Michler, J., et al. (2013). Electrochemical growth of ZnO nanowires on atomic layer deposition coated polystyrene sphere templates. Electrochim. Acta 110, 387–392. doi: 10.1016/j.electacta.2013.04.168
Etacheri, V., Marom, R., Elazari, R., Salitra, G., and Aurbach, D. (2011). Challenges in the development of advanced Li-ion batteries: a review. Energy Environ. Sci. 4, 3243–3262. doi: 10.1039/c1ee01598b
Fan, L. Z., Hu, Y. S., Maier, J., Adelhelm, P., Smarsly, B., and Antonietti, M. (2007). High Electroactivity of Polyaniline in Supercapacitors by Using a Hierarchically Porous Carbon Monolith as a Support. Adv. Funct. Mater. 17, 3083–3087. doi: 10.1002/adfm.200700518
Fan, Z., Yan, J., Zhi, L., Zhang, Q., Wei, T., Feng, J., et al. (2010). A Three-Dimensional Carbon Nanotube/Graphene Sandwich and Its Application as Electrode in Supercapacitors. Adv. Mater. 22, 3723–3728. doi: 10.1002/adma.201001029
Fang, Y., Liu, J., Yu, D. J., Wicksted, J. P., Kalkan, K., Topal, C. O., et al. (2010). Self-supported supercapacitor membranes: Polypyrrole-coated carbon nanotube networks enabled by pulsed electrodeposition. J. Power Sour. 195, 674–679. doi: 10.1016/j.jpowsour.2009.07.033
Farha, O. K., Eryazici, I., Jeong, N. C., Hauser, B. G., Wilmer, C. E., Sarjeant, A. A., et al. (2012). Metal-organic framework materials with ultrahigh surface areas: is the sky the limit? J. Am. Chem. Soc. 134:15016. doi: 10.1021/ja3055639
Feng, D.-Y., Song, Y., Huang, Z.-H., Xu, X.-X., and Liu, X.-X. (2016). Rate capability improvement of polypyrrole via integration with functionalized commercial carbon cloth for pseudocapacitor. J. Power Sour. 324, 788–797. doi: 10.1016/j.jpowsour.2016.05.112
Fic, K., Frackowiak, E., and Béguin, F. (2012a). Unusual energy enhancement in carbon-based electrochemical capacitors. J. Mater. Chem. 22:24213. doi: 10.1039/c2jm35711a
Fic, K., Lota, G., Meller, M., and Frackowiak, E. (2012b). Novel insight into neutral medium as electrolyte for high-voltage supercapacitors. Energy Environ. Sci. 5, 5842–5850. doi: 10.1039/C1EE02262H
Fic, K., Platek, A., Piwek, J., and Frackowiak, E. (2018). Sustainable materials for electrochemical capacitors. Mater. Today 21, 437–454. doi: 10.1016/j.mattod.2018.03.005
Figueiredo, J. L., Pereira, M. F. R., Freitas, M. M. A., and Órfão, J. J. M. (1999). Modification of the surface chemistry of activated carbons. Carbon 37, 1379–1389. doi: 10.1016/S0008-6223(98)00333-9
Frackowiak, E., Abbas, Q., and Béguin, F. (2013). Carbon/carbon supercapacitors. J. Energy Chem. 22, 226–240. doi: 10.1016/S2095-4956(13)60028-5
Frackowiak, E., Fic, K., Meller, M., and Lota, G. (2012). Electrochemistry serving people and nature: high-energy ecocapacitors based on redox-active electrolytes. ChemSusChem 5, 1181–1185. doi: 10.1002/cssc.201200227
Frackowiak, E., Lota, G., Machnikowski, J., Vix-Guterl, C., and Beguin, F. (2006). Optimisation of supercapacitors using carbons with controlled nanotexture and nitrogen content. Electrochim. Acta 51, 2209–2214. doi: 10.1016/j.electacta.2005.04.080
Frackowiak, E., Lota, G., and Pernak, J. (2005). Room-temperature phosphonium ionic liquids for supercapacitor application. Appl. Phys. Lett. 86, 1–3. doi: 10.1063/1.1906320
Gao, Q., Demarconnay, L., Raymundo-Piero, E., and Bguin, F. (2012). Exploring the large voltage range of carbon/carbon supercapacitors in aqueous lithium sulfate electrolyte. Energy Environ. Sci. 5, 9611–9617. doi: 10.1039/c2ee22284a
Geng, J.-W., Ye, Y.-J., Guo, D., and Liu, X.-X. (2017). Concurrent electropolymerization of aniline and electrochemical deposition of tungsten oxide for supercapacitor. J. Power Sour. 342, 980–989. doi: 10.1016/j.jpowsour.2017.01.029
Genovese, M., Jiang, J., Lian, K., and Holm, N. (2015). High capacitive performance of exfoliated biochar nanosheets from biomass waste corn cob. J. Mater. Chem. A 3, 2903–2913. doi: 10.1039/C4TA06110A
Ghidiu, M., Lukatskaya, M. R., Zhao, M.-Q., Gogotsi, Y., and Barsoum, M. W. (2014). Conductive two-dimensional titanium carbide ‘clay’ with high volumetric capacitance. Nature 516:78. doi: 10.1038/nature13970
Ghosh, S., Santhosh, R., Jeniffer, S., Raghavan, V., Jacob, G., Nanaji, K., et al. (2019). Natural biomass derived hard carbon and activated carbons as electrochemical supercapacitor electrodes. Sci. Rep. 9:16315. doi: 10.1038/s41598-019-52006-x
Gong, Y., Yang, S., Zhan, L., Ma, L., Vajtai, R., and Ajayan, P. M. (2014). A Bottom-Up Approach to Build 3D Architectures from Nanosheets for Superior Lithium Storage. Adv. Funct. Mater. 24, 125–130. doi: 10.1002/adfm.201300844
Gorska, B., Bujewska, P., and Fic, K. (2017). Thiocyanates as attractive redox-active electrolytes for high-energy and environmentally-friendly electrochemical capacitors. Phys. Chem. Chem. Phys. 19, 7923–7935. doi: 10.1039/C7CP00722A
Guan, C., Liu, J., Cheng, C., Li, H., Li, X., Zhou, W., et al. (2011). Hybrid structure of cobalt monoxide nanowire @ nickel hydroxidenitrate nanoflake aligned on nickel foam for high-rate supercapacitor. Energy Environ. Sci. 4, 4496–4499. doi: 10.1039/c1ee01685g
Guan, C., Zeng, Z., Li, X., Cao, X., Fan, Y., Xia, X., et al. (2014). Atomic-Layer-Deposition-Assisted Formation of Carbon Nanoflakes on Metal Oxides and Energy Storage Application. Small 10, 300–307. doi: 10.1002/smll.201301009
Hahn, M., Barbieri, O., Gallay, R., and Kotz, R. (2006). A dilatometric study of the voltage limitation of carbonaceous electrodes in aprotic EDLC type electrolytes by charge-induced strain. Carbon 44, 2523–2533. doi: 10.1016/j.carbon.2006.05.002
Hantel, M. M., Weingarth, D., and Kotz, R. (2014). Parameters determining dimensional changes of porous carbons during capacitive charging. Carbon 69, 275–286. doi: 10.1016/j.carbon.2013.12.026
Hayashi, H., and Hakuta, Y. (2010). Hydrothermal Synthesis of Metal Oxide Nanoparticles in Supercritical Water. Materials 3, 3794–3817. doi: 10.3390/ma3073794
He, W., Jiang, C., Wang, J., and Lu, L. (2014). High-Rate Oxygen Electroreduction over Graphitic-N Species Exposed on 3D Hierarchically Porous Nitrogen-Doped Carbons. Angewandte Chem. Int. Edn. 53, 9503–9507. doi: 10.1002/anie.201404333
He, X., Ling, P., Yu, M., Wang, X., Zhang, X., and Zheng, M. (2013). Rice husk-derived porous carbons with high capacitance by ZnCl2 activation for supercapacitors. Electrochim. Acta 105, 635–641. doi: 10.1016/j.electacta.2013.05.050
He, X., Wang, X., Sun, B., Wan, J., Wang, Y., He, D., et al. (2020). Synthesis of three-dimensional hierarchical furball-like tungsten trioxide microspheres for high performance supercapacitor electrodes. RSC Adv. 10, 13437–13441. doi: 10.1039/C9RA10995A
He, Y., Qiao, R., Vatamanu, J., Borodin, O., Bedrov, D., Huang, J., et al. (2016). Importance of Ion Packing on the Dynamics of Ionic Liquids during Micropore Charging. J. Phys. Chem. Lett. 7:36. doi: 10.1021/acs.jpclett.5b02378
Heimbckel, R., Hoffmann, F., and Frba, M. (2019). Insights into the influence of the pore size and surface area of activated carbons on the energy storage of electric double layer capacitors with a new potentially universally applicable capacitor model. Phys. Chemi. Chem. Phys. 21, 3122–3133. doi: 10.1039/C8CP06443A
Herou, S., Schlee, P., Jorge, A. B., and Titirici, M. (2018). Biomass-derived electrodes for flexible supercapacitors. Curr. Opin. Green Sustain. Chem. 9, 18–24. doi: 10.1016/j.cogsc.2017.10.005
Hirota, N., Okuno, K., Majima, M., Hosoe, A., Uchida, S., and Ishikawa, M. (2018). High-performance lithium-ion capacitor composed of electrodes with porous three-dimensional current collector and bis(fluorosulfonyl)imide-based ionic liquid electrolyte. Electrochim. Acta 276, 125–133. doi: 10.1016/j.electacta.2018.04.148
Hou, J., Cao, C., Idrees, F., and Ma, X. (2015). Hierarchical porous nitrogen-doped carbon nanosheets derived from silk for ultrahigh-capacity battery anodes and supercapacitors. ACS Nano 9:2556. doi: 10.1021/nn506394r
Hsia, B., Kim, M. S., Luna, L. E., Mair, N. R., Kim, Y., Carraro, C., et al. (2014). Templated 3D Ultrathin CVD graphite networks with controllable geometry: synthesis and application as supercapacitor electrodes. ACS Appl. Mater. Interf. 6, 18413–18417. doi: 10.1021/am504695t
Huang, X., Qian, K., Yang, J., Zhang, J., Li, L., Yu, C., et al. (2012). Functional Nanoporous Graphene Foams with Controlled Pore Sizes. Adv. Mater. 24, 4419–4423. doi: 10.1002/adma.201201680
Huang, Y., Li, H., Wang, Z., Zhu, M., Pei, Z., Xue, Q., et al. (2016). Nanostructured Polypyrrole as a flexible electrode material of supercapacitor. Nano Energy 22, 422–438. doi: 10.1016/j.nanoen.2016.02.047
Huang, Z.-H., Song, Y., Xu, X.-X., and Liu, X.-X. (2015). Ordered Polypyrrole Nanowire Arrays Grown on a Carbon Cloth Substrate for a High-Performance Pseudocapacitor Electrode. ACS Appl. Mater. Interf. 7:25506. doi: 10.1021/acsami.5b08830
Hwang, S.-W., and Hyun, S.-H. (2007). Synthesis and characterization of tin oxide/carbon aerogel composite electrodes for electrochemical supercapacitors. J. Power Sour. 172, 451–459. doi: 10.1016/j.jpowsour.2007.07.061
Izadi-Najafabadi, A., Yamada, T., Futaba, D. N., Yudasaka, M., Takagi, H., Hatori, H., et al. (2011). High-power supercapacitor electrodes from single-walled carbon nanohorn/nanotube composite. ACS Nano 5:811. doi: 10.1021/nn1017457
Ji, H., Zhang, L., Pettes, M., Li, H., Chen, S. S., Shi, L., et al. (2012). Ultrathin graphite foam: a three-dimensional conductive network for battery electrodes. Nano Lett. 12, 2446–2451. doi: 10.1021/nl300528p
Jian, L., Xiaoqian, C., Alexey, S., and Michael, K. (2012). Review of Electrochemical Capacitors Based on Carbon Nanotubes and Graphene. Graphene 1, 1–13. doi: 10.4236/graphene.2012.11001
Jin, H., Wang, X., Shen, Y., and Gu, Z. (2014). A high-performance carbon derived from corn stover via microwave and slow pyrolysis for supercapacitors. J. Anal. Appl. Pyrol. 110, 18–23. doi: 10.1016/j.jaap.2014.07.010
John, W. F. T., Zheng, C., Hongbin, Y., Jiajun, H., Kwanpyo, K., Ho-Hsiu, C., et al. (2015). Ultrahigh Surface Area Three-Dimensional Porous Graphitic Carbon from Conjugated Polymeric Molecular Framework. ACS Central Sci. 1, 68–76. doi: 10.1021/acscentsci.5b00149
Julien, C. M., and Mauger, A. (2017). Nanostructured MnO 2 as Electrode Materials for Energy Storage. Nanomaterials 7:396. doi: 10.3390/nano7110396
Jung, H. J., Kim, Y.-J., Han, J. H., Yudasaka, M., Iijima, S., Kanoh, H., et al. (2013). Thermal-Treatment-Induced Enhancement in Effective Surface Area of Single-Walled Carbon Nanohorns for Supercapacitor Application. J. Phys. Chem. C 117, 25877–25883. doi: 10.1021/jp405839z
Kalpana, D., Cho, S. H., Lee, S. B., Lee, Y. S., Misra, R., and Renganathan, N. G. (2009). Recycled waste paper—A new source of raw material for electric double-layer capacitors. J. Power Sour. 190, 587–591. doi: 10.1016/j.jpowsour.2009.01.058
Kang, M., Lee, J. E., Shim, H. W., Jeong, M. S., Im, W. B., and Yoon, H. (2016). Correction: intrinsically conductive polymer binders for electrochemical capacitor application. RSC Adv. 6, 23658–23658. doi: 10.1039/C6RA90020H
Karuturi, S. K., Luo, J., Cheng, C., Liu, L., Su, L. T., Tok, A. I. Y., et al. (2012). A Novel Photoanode with Three-Dimensionally, Hierarchically Ordered Nanobushes for Highly Efficient Photoelectrochemical Cells. Adv. Mater. 24, 4157–4162. doi: 10.1002/adma.201104428
Kashani, H., Chen, L., Ito, Y., Han, J., Hirata, A., and Chen, M. (2016). Bicontinuous nanotubular graphene–polypyrrole hybrid for high performance flexible supercapacitors. Nano Energy 19, 391–400. doi: 10.1016/j.nanoen.2015.11.029
Khomenko, V., Raymundo-Piñero, E., Frackowiak, E., and Béguin, F. (2006). High-voltage asymmetric supercapacitors operating in aqueous electrolyte. Appl. Phys. A 82, 567–573. doi: 10.1007/s00339-005-3397-8
Kim, T., Jung, G., Yoo, S., Suh, K. S., and Ruoff, R. S. (2013). Activated graphene-based carbons as supercapacitor electrodes with macro- and mesopores. ACS Nano 7:6899. doi: 10.1021/nn402077v
Kim, T., Song, W., Son, D.-Y., Ono, L. K., and Qi, Y. (2019). Lithium-ion batteries: outlook on present, future, and hybridized technologies. J. Mater. Chem. A 7, 2942–2964. doi: 10.1039/C8TA10513H
Kohlmeyer, R. R., Lor, M., Deng, J., Liu, H., and Chen, J. (2011). Preparation of stable carbon nanotube aerogels with high electrical conductivity and porosity. Carbon 49, 2352–2361. doi: 10.1016/j.carbon.2011.02.001
Krause, A., and Balducci, A. (2011). High voltage electrochemical double layer capacitor containing mixtures of ionic liquids and organic carbonate as electrolytes. Electrochem. Commun. 13, 814–817. doi: 10.1016/j.elecom.2011.05.010
Kuai, S., Badilescu, S., Bader, G., Brüning, R., Hu, X., and Truong, V. V. (2003). Preparation of Large-Area 3D Ordered Macroporous Titania Films by Silica Colloidal Crystal Templating. Adv. Mater. 15, 73–75. doi: 10.1002/adma.200390015
Lang, X., Hirata, A., Fujita, T., and Chen, M. (2011). Nanoporous metal/oxide hybrid electrodes for electrochemical supercapacitors. Nat. Nanotechnol. 6, 232–236. doi: 10.1038/nnano.2011.13
Lang, X., Zhang, L., Fujita, T., Ding, Y., and Chen, M. (2012). Three-dimensional bicontinuous nanoporous Au/polyaniline hybrid films for high-performance electrochemical supercapacitors. J. Power Sour. 197, 325–329. doi: 10.1016/j.jpowsour.2011.09.006
Lee, J., Han, S., and Hyeon, T. (2004). Synthesis of new nanoporous carbon materials using nanostructured silica materials as templates. J. Mater. Chem. 14:478. doi: 10.1039/b311541k
Lee, J., Yoon, S., Oh, S. M., Shin, C. H., and Hyeon, T. (2000). Development of a New Mesoporous Carbon Using an HMS Aluminosilicate Template. Adv. Mater. 12, 359–362. doi: 10.1002/(SICI)1521-4095(200003)12:5<359::AID-ADMA359>3.0.CO;2-1
Lei, Z., Zhang, J., and Zhao, X. S. (2011). Ultrathin MnO2 nanofibers grown on graphitic carbon spheres as high-performance asymmetric supercapacitor electrodes. J. Mater. Chem. 22, 153. doi: 10.1039/C1JM13872C
Lewis, J. A. (2006). Direct Ink Writing of 3D Functional Materials. Adv. Funct. Mater. 16, 2193–2204. doi: 10.1002/adfm.200600434
Li, H., Wang, J., Chu, Q., Wang, Z., Zhang, F., and Wang, S. (2009). Theoretical and experimental specific capacitance of polyaniline in sulfuric acid. J. Power Sour. 190, 578–586. doi: 10.1016/j.jpowsour.2009.01.052
Li, J., Wang, X., Huang, Q., Gamboa, S., and Sebastian, P. J. (2006a). A new type of MnO.sub.2[middle dot]xH.sub.2O/CRF composite electrode for supercapacitors.(Report). J. Power Sour. 160:1501. doi: 10.1016/j.jpowsour.2006.02.045
Li, J., Wang, X., Huang, Q., Gamboa, S., and Sebastian, P. J. (2006b). Studies on preparation and performances of carbon aerogel electrodes for the application of supercapacitor. J. Power Sour. 158, 784–788. doi: 10.1016/j.jpowsour.2005.09.045
Li, S.-L., and Xu, Q. (2013). Metalorganic frameworks as platforms for clean energy. Energy Environ. Sci. 6, 1656–1683. doi: 10.1039/c3ee40507a
Li, Z., Zhang, L., Amirkhiz, B. S., Tan, X., Xu, Z., Wang, H., et al. (2012). Carbonized Chicken Eggshell Membranes with 3D Architectures as High-Performance Electrode Materials for Supercapacitors (Adv. Energy Mater. 4/2012). Adv. Energy Mater. 2, 430–430. doi: 10.1002/aenm.201290018
Liang, K., Tang, X., and Hu, W. (2012). High-performance three-dimensional nanoporous NiO film as a supercapacitor electrode. J. Mater. Chem. 22:11062. doi: 10.1039/c2jm31526b
Liu, C., Li, F., Ma, L. P., and Cheng, H. M. (2010). Advanced Materials for Energy Storage. Adv. Mater. 22:E28. doi: 10.1002/adma.200903328
Liu, H.-J., Cui, W.-J., Jin, L.-H., Wang, C.-X., and Xia, Y.-Y. (2009). Preparation of three-dimensional ordered mesoporous carbon sphere arrays by a two-step templating route and their application for supercapacitors. J. Mater. Chem. 19:3661. doi: 10.1039/b819820a
Liu, J., Jiang, J., Bosman, M., and Fan, H. J. (2012). Three-dimensional tubular arrays of MnO 2 –NiO nanoflakes with high areal pseudocapacitance. J. Mater. Chem. 22, 2419–2426. doi: 10.1039/C1JM14804D
Liu, J., Jiang, J., Cheng, C., Li, H., Zhang, J., Gong, H., et al. (2011). Energy Storage: Co3O4 Nanowire@MnO2 Ultrathin Nanosheet Core/Shell Arrays: a new class of high-performance pseudocapacitive materials (Adv. Mater. 18/2011. Adv. Mater. 23, 2075–2075. doi: 10.1002/adma.201190066
Liu, J., Poh, C. K., Zhan, D., Lai, L., Lim, S. H., Wang, L., et al. (2013). Improved synthesis of graphene flakes from the multiple electrochemical exfoliation of graphite rod. Nano Energy 2, 377–386. doi: 10.1016/j.nanoen.2012.11.003
Liu, N., Ma, W., Tao, J., Zhang, X., Su, J., Li, L., et al. (2013). Cable-Type Supercapacitors of Three-Dimensional Cotton Thread Based Multi-Grade Nanostructures for Wearable Energy Storage. Adv. Mater. 25, 4925–4931. doi: 10.1002/adma.201301311
Liu, Y., Goebl, J., and Yin, Y. (2013). Templated synthesis of nanostructured materials. Chem. Soc. Rev. 42, 2610–2653. doi: 10.1039/C2CS35369E
Liu, J., Zhang, L., Wu, H. B., Lin, J., Shen, Z., and Lou, X. W. (2014). High-performance flexible asymmetric supercapacitors based on a new graphene foam/carbon nanotube hybrid film. Energy Environ. Sci. 7, 3709–3719. doi: 10.1039/C4EE01475H
Liu, T., Ling, Y., Yang, Y., Finn, L., Collazo, E., Zhai, T., et al. (2015). Investigation of hematite nanorod-nanoflake morphological transformation and the application of ultrathin nanoflakes for electrochemical devices. Nano Energy 10:169. doi: 10.1016/j.nanoen.2014.12.023
Liu, Y., Wang, W., Ying, Y., Wang, Y., and Peng, X. (2015). Binder-free layered Ti 3 C 2/CNTs nanocomposite anodes with enhanced capacity and long-cycle life for lithium-ion batteries. Dalton Trans. 44, 7123–7126. doi: 10.1039/C4DT02058H
Lota, G., Lota, K., and Frackowiak, E. (2007). Nanotubes based composites rich in nitrogen for supercapacitor application. Electrochem. Commun. 9, 1828–1832. doi: 10.1016/j.elecom.2007.04.015
Lu, X., Liu, T., Zhai, T., Wang, G., Yu, M., Xie, S., et al. (2014a). Improving the cycling stability of metal–nitride supercapacitor electrodes with a thin carbon shell. Adv. Energy Mater. 4:1300994. doi: 10.1002/aenm.201300994
Lu, X., Zeng, Y., Yu, M., Zhai, T., Liang, C., Xie, S., et al. (2014b). Oxygen-Deficient Hematite Nanorods as High-Performance and Novel Negative Electrodes for Flexible Asymmetric Supercapacitors. Adv. Mater. 26, 3148–3155. doi: 10.1002/adma.201305851
Lu, X., Wang, G., Zhai, T., Yu, M., Xie, S., Ling, Y., et al. (2012). Stabilized TiN Nanowire Arrays for High-Performance and Flexible Supercapacitors. Nano Lett. 12, 5376–5381. doi: 10.1021/nl302761z
Lu, X., Yu, M., Zhai, T., Wang, G., Xie, S., Liu, T., et al. (2013). High energy density asymmetric quasi-solid-state supercapacitor based on porous vanadium nitride nanowire anode. Nano Lett. 13:2628. doi: 10.1021/nl400760a
Lu, X., Zheng, D., Zhai, T., Liu, Z., Huang, Y., Xie, S., et al. (2011). Facile synthesis of large-area manganese oxide nanorod arrays as a high-performance electrochemical supercapacitor. Energy Environ. Sci. 4, 2915–2921. doi: 10.1039/c1ee01338f
Luan, F., Wang, G., Ling, Y., Lu, X., Wang, H., Tong, Y., et al. (2013). High energy density asymmetric supercapacitors with a nickel oxide nanoflake cathode and a 3D reduced graphene oxide anode. Nanoscale 5, 7984–7990. doi: 10.1039/c3nr02710d
Lukatskaya, M. R., Mashtalir, O., Ren, C. E., Dall’agnese, Y., Rozier, P., Taberna, P. L., et al. (2013). Cation intercalation and high volumetric capacitance of two-dimensional titanium carbide.(REPORTS)(Author abstract). Science 341:1502. doi: 10.1126/science.1241488
Lv, Y., Gan, L., Liu, M., Xiong, W., Xu, Z., Zhu, D., et al. (2012). A self-template synthesis of hierarchical porous carbon foams based on banana peel for supercapacitor electrodes. J. Power Sour. 209, 152–157. doi: 10.1016/j.jpowsour.2012.02.089
Mai, L.-Q., Yang, F., Zhao, Y.-L., Xu, X., Xu, L., and Luo, Y.-Z. (2011). Hierarchical MnMoO4/CoMoO4 heterostructured nanowires with enhanced supercapacitor performance. Nat. Commun. 2:381. doi: 10.1038/ncomms1387
Malak, A., Fic, K., Lota, G., Vix-Guterl, C., and Frackowiak, E. (2010). Hybrid materials for supercapacitor application. J. Solid State Electrochem. 14, 811–816. doi: 10.1007/s10008-009-0856-8
Manish, C., Hyeon Suk, S., Goki, E., Lain-Jong, L., Kian Ping, L., and Hua, Z. (2013). The chemistry of two-dimensional layered transition metal dichalcogenide nanosheets. Nat. Chem. 5:263. doi: 10.1038/nchem.1589
Marcano, D. C., Kosynkin, D. V., Berlin, J. M., Sinitskii, A., Sun, Z., Slesarev, A., et al. (2010). Improved synthesis of graphene oxide. ACS Nano 4:4806. doi: 10.1021/nn1006368
Martins, T. B., Miwa, R. H., Da Silva, A. J. R., and Fazzio, A. (2007). Electronic and transport properties of boron-doped graphene nanoribbons. Phys. Rev. Lett. 98:196803. doi: 10.1103/PhysRevLett.98.196803
Mastragostino, M., Arbizzani, C., and Soavi, F. (2002). Conducting polymers as electrode materials in supercapacitors. Solid State Ionics 148, 493–498. doi: 10.1016/S0167-2738(02)00093-0
Meng, C., Maeng, J., John, S. W. M., and Irazoqui, P. P. (2014). Ultrasmall Integrated 3D micro-supercapacitors solve energy storage for miniature devices. Adv. Energy Mater. 4:1301269. doi: 10.1002/aenm.201301269
Meng, Y., Wang, K., Zhang, Y., and Wei, Z. (2013). Hierarchical Porous Graphene/Polyaniline Composite Film with Superior Rate Performance for Flexible Supercapacitors. Adv. Mater. 25, 6985–6990. doi: 10.1002/adma.201303529
Menzel, J., Fic, K., and Frackowiak, E. (2015). Hybrid aqueous capacitors with improved energy/power performance. Progr. Nat. Sci. 25, 642–649. doi: 10.1016/j.pnsc.2015.12.001
Miller, E. E., Hua, Y., and Tezel, F. H. (2018). Materials for energy storage: review of electrode materials and methods of increasing capacitance for supercapacitors. Jo. Energy Stor. 20, 30–40. doi: 10.1016/j.est.2018.08.009
Miller, J. M., and Dunn, B. (1999). Morphology and electrochemistry of ruthenium/carbon aerogel nanostructures. Langmuir 15, 799–806. doi: 10.1021/la980799g
Miller, J. M., Onar, O. C., White, C., Campbell, S., Coomer, C., Seiber, L., et al. (2014). Demonstrating dynamic wireless charging of an electric vehicle: the benefit of electrochemical capacitor smoothing. IEEE Power Electr. Magn. 1, 12–24. doi: 10.1109/MPEL.2014.2300978
Mirzaeian, M., Abbas, Q., Ogwu, A., Hall, P., Goldin, M., Mirzaeian, M., et al. (2017). Electrode and electrolyte materials for electrochemical capacitors. Int. J. Hydrog. Energy 42, 25565–25587. doi: 10.1016/j.ijhydene.2017.04.241
Müller, M., Zentel, R., Maka, T., Romanov, S. G., and Sotomayor Torres, C. M. (2000). Photonic Crystal Films with High Refractive Index Contrast. Adv. Mater. 12, 1499–1503. doi: 10.1002/1521-4095(200010)12:20<1499::AID-ADMA1499>3.0.CO;2-M
Naguib, M., and Gogotsi, Y. (2015). Synthesis of two-dimensional materials by selective extraction. Acc Chem. Res. 48, 128–135. doi: 10.1021/ar500346b
Najib, S., and Erdem, E. (2019). Current progress achieved in novel materials for supercapacitor electrodes: mini review. Nanoscale Adv. 1, 2817–2827. doi: 10.1039/C9NA00345B
Nakanishi, K., Tomita, M., and Kato, K. (2013). Improvement in the catalytic activity of cytochrome c by immobilisation on a novel mesoporous silica sheet. RSC Adv. 4, 4732–4735. doi: 10.1039/C3RA45861J
Nam, M. S., Patil, U., Park, B., Sim, H. B., and Jun, S. C. (2016). A binder free synthesis of 1D PANI and 2D MoS 2 nanostructured hybrid composite electrodes by the electrophoretic deposition (EPD) method for supercapacitor application. RSC Adv. 6, 101592–101601. doi: 10.1039/C6RA16078F
Nguyen, N. L., and Rochefort, D. (2014). Electrochemistry of ruthenium dioxide composite electrodes in diethylmethylammonium-triflate protic ionic liquid and its mixtures with acetonitrile. Electrochim. Acta 147, 96–103. doi: 10.1016/j.electacta.2014.08.143
Nitta, N., Wu, F., Lee, J. T., and Yushin, G. (2015). Li-ion battery materials: present and future. Mater. Today 18, 252–264. doi: 10.1016/j.mattod.2014.10.040
Niu, Z., Chen, J., Hng, H. H., Ma, J., and Chen, X. (2012). A Leavening Strategy to Prepare Reduced Graphene Oxide Foams. Adv. Mater. 24, 4144–4150. doi: 10.1002/adma.201200197
Nomoto, S., Nakata, H., Yoshioka, K., Yoshida, A., and Yoneda, H. (2001). Advanced capacitors and their application. J. Power Sour. 9, 807–811. doi: 10.1016/S0378-7753(01)00612-7
Pan, L., He, Q., Liu, J., Chen, Y., Ma, M., Zhang, L., et al. (2012). Nuclear-Targeted Drug Delivery of TAT Peptide-Conjugated Monodisperse Mesoporous Silica Nanoparticles. J. Am. Chem. Soc. 134, 5722–5725. doi: 10.1021/ja211035w
Pandolfo, A. G., and Hollenkamp, A. F. (2006). Carbon properties and their role in supercapacitors. J. Power Sour. 157, 11–27. doi: 10.1016/j.jpowsour.2006.02.065
Parvez, K., Wu, Z.-S., Li, R., Liu, X., Graf, R., Feng, X., et al. (2014). Exfoliation of graphite into graphene in aqueous solutions of inorganic salts. J. Am. Chem. Soc. 136:6083. doi: 10.1021/ja5017156
Patil, U. M., Sohn, J. S., Kulkarni, S. B., Lee, S. C., Park, H. G., Gurav, K. V., et al. (2014). Enhanced supercapacitive performance of chemically grown cobalt-nickel hydroxides on three-dimensional graphene foam electrodes. ACS Appl. Mater. Interf. 6:2450. doi: 10.1021/am404863z
Peng, C., Zhang, S., Jewell, D., and Chen, G. Z. (2008). Carbon nanotube and conducting polymer composites for supercapacitors. Progr. Nat. Sci. 18, 777–788. doi: 10.1016/j.pnsc.2008.03.002
Pineiro-Prado, I., Salinas-Torres, D., Ruiz-Rosas, R., Morallon, E., and Cazorla-Amoros, D. (2016). Design of Activated Carbon/Activated Carbon Asymmetric Capacitors. (Report)(Author abstract) 3:16. doi: 10.3389/fmats.2016.00016
Prehal, C., Weingarth, D., Perre, E., Lechner, R. T., Amenitsch, H., Paris, O., et al. (2015). Tracking the structural arrangement of ions in carbon supercapacitor nanopores using in situ small-angle X-ray scattering. Energy Environ. Sci. 8, 1725–1735. doi: 10.1039/C5EE00488H
Pu, J., Wang, Z., Wu, K., Yu, N., and Sheng, E. (2013). Co 9 S 8 nanotube arrays supported on nickel foam for high-performance supercapacitors. Phys. Chemi. Chem. Phys. 16, 785–791. doi: 10.1039/C3CP54192D
Pumera, M., Sofer, Z., and Ambrosi, A. (2014). Layered transition metal dichalcogenides for electrochemical energy generation and storage. J. Mater. Chem. A 2, 8981–8987. doi: 10.1039/C4TA00652F
Qingqing, K., and John, W. (2016). Graphene-based materials for supercapacitor electrodes – A review. J. Materiom. 2, 37–54. doi: 10.1016/j.jmat.2016.01.001
Qu, L., Zhao, Y., Khan, A. M., Han, C., Hercule, K. M., Yan, M., et al. (2015). Interwoven Three-Dimensional Architecture of Cobalt Oxide Nanobrush-Graphene@Ni x Co 2 x (OH) 6 x for High-Performance Supercapacitors. Nano Lett. 15, 2037–2044. doi: 10.1021/nl504901p
Ratajczak, P., Jurewicz, K., Skowron, P., Abbas, Q., and Béguin, F. (2014). Effect of accelerated ageing on the performance of high voltage carbon/carbon electrochemical capacitors in salt aqueous electrolyte. Electrochim. Acta 130, 344–350. doi: 10.1016/j.electacta.2014.02.140
Raymundo-Piñero, E., Leroux, F., and Béguin, F. (2006). A high-performance carbon for supercapacitors obtained by carbonization of a seaweed biopolymer. Adv. Mater. 18, 1877–1882. doi: 10.1002/adma.200501905
Ruch, P. W., Cericola, D., Foelske, A., Kötz, R., and Wokaun, A. (2010). A comparison of the aging of electrochemical double layer capacitors with acetonitrile and propylene carbonate-based electrolytes at elevated voltages. Electrochim. Acta 55, 2352–2357. doi: 10.1016/j.electacta.2009.11.098
Ruiz, V., Santamaria, R., Granda, M., and Blanco, C. (2009). Long-term cycling of carbon-based supercapacitors in aqueous media. Electrochim. Acta 54, 4481–4486. doi: 10.1016/j.electacta.2009.03.024
Ryoo, R., Joo, S. H., Kruk, M., and Jaroniec, M. (2001). Ordered Mesoporous Carbons. Adv. Mater. 13, 677–681. doi: 10.1002/1521-4095(200105)13:9<677::AID-ADMA677>3.0.CO;2-C
Sadayappan, N., and Kwang-Sun, R. (2019). Synthesis of Ag/NiO Honeycomb Structured Nanoarrays as the Electrode Material for High Performance Asymmetric Supercapacitor Devices. Sci. Rep. 9, 1–11. doi: 10.1038/s41598-019-41446-0
Salunkhe, R. R., Tang, J., Kamachi, Y., Nakato, T., Kim, J. H., and Yamauchi, Y. (2015). Asymmetric Supercapacitors Using 3D Nanoporous Carbon and Cobalt Oxide Electrodes Synthesized from a Single Metal-Organic Framework. ACS Nano 9:6288. doi: 10.1021/acsnano.5b01790
Sathyamoorthi, S., Kanagaraj, M., Kathiresan, M., Suryanarayanan, V., and Velayutham, D. (2016). Ethyl viologen dibromide as a novel dual redox shuttle for supercapacitors. J. Mater. Chem. A 4, 4562–4569. doi: 10.1039/C6TA00858E
Schipper, F., Erickson, E. M., Erk, C., Shin, J.-Y., Chesneau, F. F., and Aurbach, D. (2017). Review—Recent advances and remaining challenges for lithium ion battery cathodes: I. Nickel-Rich, LiNiCoMnO. J. Electrochem. Soc. 164, A6220–A6228. doi: 10.1149/2.0351701jes
Scrosati, B. (2011). History of lithium batteries. J. Solid State Electrochem. 15, 1623–1630. doi: 10.1007/s10008-011-1386-8
Sen, P., and De, A. (2010). Electrochemical performances of poly(3,4-ethylenedioxythiophene)–NiFe 2O 4 nanocomposite as electrode for supercapacitor. Electrochim. Acta 55, 4677–4684. doi: 10.1016/j.electacta.2010.03.077
Shao, P., Zhang, Q., Li, Y., and Wang, H. (2010). Aqueous synthesis of color-tunable and stable Mn2+-doped ZnSe quantum dots. J. Mater. Chem. 21:151. doi: 10.1039/C0JM01878C
Sheberla, D., Bachman, J. C., Elias, J. S., Sun, C.-J., Shao-Horn, Y., and Dincã, M. (2016). Conductive MOF electrodes for stable supercapacitors with high areal capacitance. Nat. Mater. 16, 220–224. doi: 10.1038/nmat4766
Shukla, A. K., Banerjee, A., Ravikumar, M. K., and Jalajakshi, A. (2012). Electrochemical capacitors: technical challenges and prognosis for future markets. Electrochim. Acta 84, 165–173. doi: 10.1016/j.electacta.2012.03.059
Simon, P., and Gogotsi, Y. (2008). Materials for electrochemical capacitors. Nat. Mater. 7, 845–854. doi: 10.1038/nmat2297
Simon, P., and Gogotsi, Y. (2010). Charge storage mechanism in nanoporous carbons and its consequence for electrical double layer capacitors. Philos. Trans. A Math. Phys. Eng. Sci. 368, 3457–3467. doi: 10.1098/rsta.2010.0109
Simon, P., and Gogotsi, Y. (2013). Capacitive Energy Storage in Nanostructured Carbon-Electrolyte Systems. ACC. Chem. Res. 46, 1094–1103. doi: 10.1021/ar200306b
Simotwo, S. K., and Kalra, V. (2018). Polyaniline-carbon based binder-free asymmetric supercapacitor in neutral aqueous electrolyte. Electrochim. Acta 268, 131–138. doi: 10.1016/j.electacta.2018.01.157
Slesinski, A., Matei-Ghimbeu, C., Fic, K., Béguin, F., and Frackowiak, E. (2018). Self-buffered pH at carbon surfaces in aqueous supercapacitors. Carbon 129, 758–765. doi: 10.1016/j.carbon.2017.12.101
Some, S., Kim, J., Lee, K., Kulkarni, A., Yoon, Y., Lee, S., et al. (2012). Highly Air-Stable Phosphorus-Doped n-Type Graphene Field-Effect Transistors. Adv. Mater. 24, 5481–5486. doi: 10.1002/adma.201202255
Song, Y., Cai, X., Xu, X., and Liu, X.-X. (2015a). Integration of nickelcobalt double hydroxide nanosheets and polypyrrole films with functionalized partially exfoliated graphite for asymmetric supercapacitors with improved rate capability. J. Mater. Chem. A 3, 14712–14720. doi: 10.1039/C5TA02810H
Song, Y., Feng, D.-Y., Liu, T.-Y., Li, Y., and Liu, X.-X. (2015b). Controlled partial-exfoliation of graphite foil and integration with MnO 2 nanosheets for electrochemical capacitors. Nanoscale 7, 3581–3587. doi: 10.1039/C4NR06559J
Song, Y., Deng, P., Qin, Z., Feng, D., Guo, D., Sun, X., et al. (2019). A polyanionic molybdenophosphate anode for a 2.7 V aqueous pseudocapacitor. Nano Energy 65:104010. doi: 10.1016/j.nanoen.2019.104010
Song, Y., Liu, T., Qian, F., Zhu, C., Yao, B., Duoss, E., et al. (2018). Three-dimensional carbon architectures for electrochemical capacitors. J. Colloid Interface Sci. 509, 529–545. doi: 10.1016/j.jcis.2017.07.081
Song, Y., Liu, T.-Y., Xu, G.-L., Feng, D.-Y., Yao, B., Kou, T.-Y., et al. (2016). Tri-layered graphite foil for electrochemical capacitors. J. Mater. Chem. A 4, 7683–7688. doi: 10.1039/C6TA02075E
Song, Y., Liu, T. Y., Yao, B., Kou, T. Y., Feng, D. Y., Liu, X. X., et al. (2017). Amorphous mixed-valence vanadium oxide/exfoliated carbon cloth structure shows a record high cycling stability. Small 13:1700067. doi: 10.1002/smll.201700067
Song, Y., Xu, J.-L., and Liu, X.-X. (2014). Electrochemical anchoring of dual doping polypyrrole on graphene sheets partially exfoliated from graphite foil for high-performance supercapacitor electrode.(Report). J. Power Sour. 249:48. doi: 10.1016/j.jpowsour.2013.10.102
Sun, X., Li, Q., Lü, Y., and Mao, Y. (2013). Three-dimensional ZnO@MnO2 core@shell nanostructures for electrochemical energy storage. Chem. Commun. 49:4456. doi: 10.1039/c3cc41048j
Suss, M. E., Baumann, T. F., Worsley, M. A., Rose, K. A., Jaramillo, T. F., Stadermann, M., et al. (2013). Impedance-based study of capacitive porous carbon electrodes with hierarchical and bimodal porosity. J. Power Sour. 241, 266–273. doi: 10.1016/j.jpowsour.2013.03.178
Tang, C.-H., Yin, X., and Gong, H. (2013). Superior Performance Asymmetric Supercapacitors Based on a Directly Grown Commercial Mass 3D Co 3 O 4 @Ni(OH) 2 Core–Shell Electrode. ACS Appl. Mater. Interf. 5, 10574–10582. doi: 10.1021/am402436q
Thanh-Hai, L., Yukyung, K., and Hyeonseok, Y. (2017). Electrical and Electrochemical Properties of Conducting Polymers. Polymers 9:150. doi: 10.3390/polym9040150
Theerthagiri, J., Karuppasamy, K., Durai, G., Rana, A. U. H. S., Arunachalam, P., Sangeetha, K., et al. (2018). Recent Advances in Metal Chalcogenides (MX; =) nanostructures for electrochemical supercapacitor applications: a brief review. Nanomaterials 8:256. doi: 10.3390/nano8040256
Tomczykowa, M., and Plonska-Brzezinska, M. E. (2019). Conducting polymers. hydrogels and their composites: preparation, properties and bioapplications. Polymers 11:350. doi: 10.3390/polym11020350
Tong, L., Skorenko, K. H., Faucett, A. C., Boyer, S. M., Liu, J., Mativetsky, J. M., et al. (2015). Vapor-phase polymerization of poly(3,4-ethylenedioxythiophene) (PEDOT) on commercial carbon coated aluminum foil as enhanced electrodes for supercapacitors. J. Power Sour. 297, 195–201. doi: 10.1016/j.jpowsour.2015.06.128
Ullah, N., Mcarthur, M. A., and Omanovic, S. (2015). Iridium-ruthenium-oxide coatings for supercapacitors. Can. J. Chem. Eng., 93, 1941–1948. doi: 10.1002/cjce.22318
Ussia, M., Urso, M., Miritello, M., Bruno, E., Curcuruto, G., Vitalini, D., et al. (2019). Hybrid nickel-free graphene/porphyrin rings for photodegradation of emerging pollutants in water. RSC Adv. 9, 30182–30194. doi: 10.1039/C9RA06328E
Vangari, M., Pryor, T., and Jiang, L. (2013). Supercapacitors: review of materials and fabrication methods. J. Energy Eng. 139, 72–79. doi: 10.1061/(ASCE)EY.1943-7897.0000102
Vivekchand, S., Rout, C., Subrahmanyam, K., Govindaraj, A., and Rao, C. (2008). Graphene-based electrochemical supercapacitors. J. Chem. Sci. 120, 9–13. doi: 10.1007/s12039-008-0002-7
Wang, D. W., Li, F., Liu, M., Lu, G. Q., and Cheng, H. M. (2008). 3D aperiodic hierarchical porous graphitic carbon material for high-rate electrochemical capacitive energy storage. Angewandte Chem. Int. Edn. 47, 373–376. doi: 10.1002/anie.200702721
Wang, G., Lei, Z., and Jiujun, Z. (2012a). A review of electrode materials for electrochemical supercapacitors. ChemsucChem 5:797. doi: 10.1039/C1CS15060J
Wang, G., Lu, X., Ling, Y., Zhai, T., Wang, H., Tong, Y., et al. (2012b). LiCl/PVA gel electrolyte stabilizes vanadium oxide nanowire electrodes for pseudocapacitors. ACS Nano 6:10296. doi: 10.1021/nn304178b
Wang, G., Ling, Y., Qian, F., Yang, X., Liu, X.-X., and Li, Y. (2011). Enhanced capacitance in partially exfoliated multi-walled carbon nanotubes. J. Power Sour. 196, 5209–5214. doi: 10.1016/j.jpowsour.2011.02.019
Wang, J., Manga, K. K., Bao, Q., and Loh, K. P. (2011). High-yield synthesis of few-layer graphene flakes through electrochemical expansion of graphite in propylene carbonate electrolyte. J. Am. Chem. Soc. 133, 8888. doi: 10.1021/ja203725d
Wang, G., Wang, H., Lu, X., Ling, Y., Yu, M., Zhai, T., et al. (2014). Solid-State Supercapacitor Based on Activated Carbon Cloths Exhibits Excellent Rate Capability. Adv. Mater. 26, 2676–2682. doi: 10.1002/adma.201304756
Wang, X., Lu, X., Liu, B., Chen, D., Tong, Y., and Shen, G. (2014). Flexible Energy-Storage Devices: Design Consideration and Recent Progress. Adv. Mater. 26, 4763–4782. doi: 10.1002/adma.201400910
Wang, L., Han, Y., Feng, X., Zhou, J., Qi, P., and Wang, B. (2016). Metal-organic frameworks for energy storage: batteries and supercapacitors. Coordinat. Chem. Rev. 307, 361–381. doi: 10.1016/j.ccr.2015.09.002
Wang, W., Guo, S., Penchev, M., Ruiz, I., Bozhilov, K. N., Yan, D., et al. (2013a). Three dimensional few layer graphene and carbon nanotube foam architectures for high fidelity supercapacitors. Nano Energy 2, 294–303. doi: 10.1016/j.nanoen.2012.10.001
Wang, X., Liu, B., Wang, Q., Song, W., Hou, X., Chen, D., et al. (2013b). Three-Dimensional Hierarchical GeSe 2 Nanostructures for High Performance Flexible All-Solid-State Supercapacitors. Adv. Mater. 25, 1479–1486. doi: 10.1002/adma.201370061
Wang, W., Liu, W., Zeng, Y., Han, Y., Yu, M., Lu, X., et al. (2015). A Novel Exfoliation Strategy to Significantly Boost the Energy Storage Capability of Commercial Carbon Cloth. Adv. Mater. 27, 3572–3578. doi: 10.1002/adma.201500707
Wang, X., Ding, X., and Zou, H. (2020). Mesoporous Silica Nanosheets with Tunable Pore Lengths Supporting Metal Nanoparticles for Enhanced Hydrogenation Reactions. Catalysts 10:12. doi: 10.3390/catal10010012
Wang, X., Wu, D., Song, X., Du, W., Zhao, X., and Zhang, D. (2019). Review on carbon/polyaniline hybrids: design and synthesis for supercapacitor. Molecules 24:2263. doi: 10.3390/molecules24122263
Wei, D., Grande, L., Chundi, V., White, R., Bower, C., Andrew, P., et al. (2012). Graphene from electrochemical exfoliation and its direct applications in enhanced energy storage devices. Chem. Commun. 48, 1239–1241. doi: 10.1039/C2CC16859F
Wei, W., Mi, L., Gao, Y., Zheng, Z., Chen, W., and Guan, X. (2014). Partial Ion-Exchange of Nickel-Sulfide-Derived Electrodes for High Performance Supercapacitors. Chem. Mater. 26, 3418–3426. doi: 10.1021/cm5006482
Wei, X., Jiang, X., Wei, J., and Gao, S. (2016). Functional Groups and Pore Size Distribution Do Matter to Hierarchically Porous Carbons as High-Rate-Performance Supercapacitors. Chem. Mate. 28, 445–458. doi: 10.1021/acs.chemmater.5b02336
Weingarth, D., Noh, H., Foelske-Schmitz, A., Wokaun, A., and Kotz, R. (2013). A reliable determination method of stability limits for electrochemical double layer capacitors. Electrochim. Acta 103, 119–124. doi: 10.1016/j.electacta.2013.04.057
Wen, Z., Wang, X., Mao, S., Bo, Z., Kim, H., Cui, S., et al. (2012). Crumpled Nitrogen-Doped Graphene Nanosheets with Ultrahigh Pore Volume for High-Performance Supercapacitor. Adv. Mater. 24, 5610–5616. doi: 10.1002/adma.201201920
Winkless, L. (2014). Carbon nanotube supercapacitors for portable electronics. Mater. Today 17, 266–266. doi: 10.1016/j.mattod.2014.06.013
Wong, S., Kitaev, V., and Ozin, G. A. (2003). Colloidal crystal films: advances in universality and perfection. J. Am. Chem. Soc. 125, 15589–15598. doi: 10.1021/ja0379969
Worsley, M. A., Pauzauskie, P. J., Olson, T. Y., Biener, J., Satcher, J. H., and Baumann, T. F. (2010). Synthesis of graphene aerogel with high electrical conductivity. J. Am. Chem. Soc. 132:14067. doi: 10.1021/ja1072299
Wu, H., Xu, M., Wang, Y., and Zheng, G. (2013). Branched Co 3 O 4/Fe 2 O 3 nanowires as high capacity lithium-ion battery anodes. Nano Res. 6, 167–173. doi: 10.1007/s12274-013-0292-z
Wu, Z. S., Winter, A., Chen, L., Sun, Y., Turchanin, A., Feng, X., et al. (2012). Three-dimensional nitrogen and boron co-doped graphene for high-performance all-solid-state supercapacitors. Adv. Mater. 24, 5130–5135. doi: 10.1002/adma.201201948
Xia, X., Chao, D., Fan, Z., Guan, C., Cao, X., Zhang, H., et al. (2014a). A new type of porous graphite foams and their integrated composites with oxide/polymer core/shell nanowires for supercapacitors: structural design, fabrication, and full supercapacitor demonstrations. Nano Lett. 14:1651. doi: 10.1021/nl5001778
Xia, X., Zhu, C., Luo, J., Zeng, Z., Guan, C., Ng, C. F., et al. (2014b). Synthesis of Free-Standing Metal Sulfide Nanoarrays via Anion Exchange Reaction and Their Electrochemical Energy Storage Application. Small 10, 766–773. doi: 10.1002/smll.201302224
Xia, X., Tu, J., Zhang, Y., Wang, X., Gu, C., Zhao, X.-B., et al. (2012). High-quality metal oxide core/shell nanowire arrays on conductive substrates for electrochemical energy storage. ACS Nano 6:5531. doi: 10.1021/nn301454q
Xia, X. H., Tu, J. P., Zhang, J., Xiang, J. Y., Wang, X. L., and Zhao, X. B. (2010). Cobalt Oxide Ordered Bowl-Like Array Films Prepared by Electrodeposition through Monolayer Polystyrene Sphere Template and Electrochromic Properties. ACS Appl. Mater. Interf. 2, 186–192. doi: 10.1021/am900636g
Xiao, J., Wan, L., Yang, S., Xiao, F., and Wang, S. (2014). Design Hierarchical Electrodes with Highly Conductive NiCo 2 S 4 Nanotube Arrays Grown on Carbon Fiber Paper for High-Performance Pseudocapacitors. Nano Lett. 14, 831–838. doi: 10.1021/nl404199v
Xie, X., Zhang, C., Wu, M.-B., Tao, Y., Lv, W., and Yang, Q.-H. (2013). Porous MnO 2 for use in a high performance supercapacitor: replication of a 3D graphene network as a reactive template. Chem. Commun. 49, 11092–11094. doi: 10.1039/c3cc46867d
Xiong, X., Ding, D., Chen, D., Waller, G., Bu, Y., Wang, Z., et al. (2015). Three-dimensional ultrathin Ni(OH)2 nanosheets grown on nickel foam for high-performance supercapacitors. Nano Energy 11, 154–161. doi: 10.1016/j.nanoen.2014.10.029
Xu, C., Xu, B., Gu, Y., Xiong, Z., Sun, J., and Zhao, X. S. (2013). Graphene-based electrodes for electrochemical energy storage. Energy Environ. Sci. 6, 1388–1414. doi: 10.1039/c3ee23870a
Xu, K., Li, W., Liu, Q., Li, B., Liu, X., An, L., et al. (2014). Hierarchical mesoporous NiCo 2 O 4 @MnO 2 coreshell nanowire arrays on nickel foam for aqueous asymmetric supercapacitors. J. Mater. Chem. A 2, 4795–4802. doi: 10.1039/c3ta14647b
Yamaguchi, I., Watanabe, M., Shinagawa, T., Chigane, M., Inaba, M., Tasaka, A., et al. (2009). Preparation of Core/Shell and Hollow Nanostructures of Cerium Oxide by Electrodeposition on a Polystyrene Sphere Template. ACS Appl. Mater. Interf. 1, 1070–1075. doi: 10.1021/am900040c
Yan, D., Guo, Z., Zhu, G., Yu, Z., Xu, H., and Yu, A. (2012). MnO.sub.2 film with three-dimensional structure prepared by hydrothermal process for supercapacitor.(Report). J. Power Sour. 199:409. doi: 10.1016/j.jpowsour.2011.10.051
Yan, J., Liu, J., Fan, Z., Wei, T., and Zhang, L. (2012). High-performance supercapacitor electrodes based on highly corrugated graphene sheets. Carbon 50, 2179–2188. doi: 10.1016/j.carbon.2012.01.028
Yan, J., Sumboja, A., Khoo, E., and Lee, P. S. (2011). V2O5 Loaded on SnO2 Nanowires for High-Rate Li Ion Batteries. Adv. Mater. 23, 746–750. doi: 10.1002/adma.201003805
Yang, C.-M., Kim, Y.-J., Endo, M., Kanoh, H., Yudasaka, M., Iijima, S., et al. (2007). Nanowindow-regulated specific capacitance of supercapacitor electrodes of single-wall carbon nanohorns. J. Am. Chem. Soc. 129:20. doi: 10.1021/ja065501k
Yang, X.-H., Wang, Y.-G., Xiong, H.-M., and Xia, Y.-Y. (2007). Interfacial synthesis of porous MnO 2 and its application in electrochemical capacitor. Electrochim. Acta 53, 752–757. doi: 10.1016/j.electacta.2007.07.043
Yang, H., Ye, S., Zhou, J., and Liang, T. (2019). Biomass-derived porous carbon materials for supercapacitor. Front. Chem. 7:274. doi: 10.3389/fchem.2019.00274
Yang, S., Lohe, M. R., Müllen, K., and Feng, X. (2016). New-generation graphene from electrochemical approaches: production and applications. Adv. Mater. 28, 6213–6221. doi: 10.1002/adma.201505326
Yang, X., Zhu, J., Qiu, L., and Li, D. (2011). Graphene assembly: bioinspired effective prevention of restacking in multilayered graphene films: towards the next generation of high-performance supercapacitors (Adv. Mater. 25/2011. Adv. Mater. 23, 2771–2771. doi: 10.1002/adma.201190093
Yang, Y., Fei, H., Ruan, G., Xiang, C., and Tour, J. M. (2014). Edge-Oriented MoS2 Nanoporous Films as Flexible Electrodes for Hydrogen Evolution Reactions and Supercapacitor Devices. Adv. Mater. 26, 8163–8168. doi: 10.1002/adma.201402847
Yao, B., Chandrasekaran, S., Zhang, H., Ma, A., Kang, J., Zhang, L., et al. (2020). 3D-Printed Structure Boosts the Kinetics and Intrinsic Capacitance of Pseudocapacitive Graphene Aerogels. Adv. Mater. 32:1906652. doi: 10.1002/adma.201906652
Ye, Y.-J., Huang, Z.-H., Song, Y., Geng, J.-W., Xu, X.-X., and Liu, X.-X. (2017). Electrochemical Growth of Polyaniline Nanowire Arrays on Graphene Sheets in Partially Exfoliated Graphite Foil for High-Performance Supercapacitive Materials. Electrochim. Acta 240, 72–79. doi: 10.1016/j.electacta.2017.04.025
Yenny, H., Valeria, N., Mustafa, L., Fiona, M. B., Zhenyu, S., Sukanta, D., et al. (2008). High- yield production of graphene by liquid- phase exfoliation of graphite. Nat. Nanotechnol. 3:563.
Yoon, Y., Lee, K., Baik, C., Yoo, H., Min, M., Park, Y., et al. (2013). Anti-Solvent Derived Non-Stacked Reduced Graphene Oxide for High Performance Supercapacitors. Adv. Mater. 25, 4437–4444. doi: 10.1002/adma.201301230
You, B., Wang, L., Yao, L., and Yang, J. (2013). Three dimensional N-doped grapheneCNT networks for supercapacitor. Chem. Commun. 49, 5016–5018. doi: 10.1039/c3cc41949e
Younesi, S. R., Veith, G. M., Johansson, P., Edström, K., and Vegge, T. (2015). Lithium salts for advanced lithium batteries: Li-metal. Li-O2, and Li-S. Energy Environ. Sci. 8, 1905–1922. doi: 10.1039/C5EE01215E
Yu, M., Han, Y., Cheng, X., Hu, L., Zeng, Y., Chen, M., et al. (2015a). Holey tungsten oxynitride nanowires: novel anodes efficiently integrate microbial chemical energy conversion and electrochemical energy storage. Adv. Mater. 27, 3085–3091. doi: 10.1002/adma.201500493
Yu, M., Huang, Y., Li, C., Zeng, Y., Wang, W., Li, Y., et al. (2015b). Building Three-Dimensional Graphene Frameworks for Energy Storage and Catalysis. Adv. Funct. Mater. 25, 324–330. doi: 10.1002/adfm.201402964
Yu, M., Qiu, W., Wang, F., Zhai, T., Fang, P., Lu, X., et al. (2015c). Three dimensional architectures: design, assembly and application in electrochemical capacitors. J. Mater. Chem. A 3, 15792–15823. doi: 10.1039/C5TA02743H
Yu, M., Patrick, H., Annette Von, J., and Alexandre, Y. (2019). Current Li-Ion Battery Technologies in Electric Vehicles and Opportunities for Advancements. Energies 12:1074. doi: 10.3390/en12061074
Yu, M., Zhai, T., Lu, X., Chen, X., Xie, S., Li, W., et al. (2013). Manganese dioxide nanorod arrays on carbon fabric for flexible solid-state supercapacitors. J. Power Sour. 239, 64–71. doi: 10.1016/j.jpowsour.2013.03.083
Yu, M., Zhang, Y., Zeng, Y., Balogun, M. S., Mai, K., Zhang, Z., et al. (2014). Water Surface Assisted Synthesis of Large-Scale Carbon Nanotube Film for High-Performance and Stretchable Supercapacitors. Adv. Mater. 26, 4724–4729. doi: 10.1002/adma.201401196
Yu, Z., and Thomas, J. (2014). Energy storage: energy storing electrical cables: integrating energy storage and electrical conduction (Adv. Mater. 25/2014. Adv. Mater. 26, 4400–4400. doi: 10.1002/adma.201470172
Yuan, P., Zhang, N., Zhang, D., Liu, T., Chen, L., Liu, X., et al. (2014). Fabrication of nickel-foam-supported layered zinccobalt hydroxide nanoflakes for high electrochemical performance in supercapacitors. Chem. Commun. 50, 11188–11191. doi: 10.1039/C4CC05057F
Yun, Y. S., Cho, S. Y., Shim, J., Kim, B. H., Chang, S. J., Baek, S. J., et al. (2013). Microporous Carbon Nanoplates from Regenerated Silk Proteins for Supercapacitors. Adv. Mater. 25, 1993–1998. doi: 10.1002/adma.201204692
Yuxi, X., Zhaoyang, L., Xing, Z., Xiaoqing, H., Nathan, O. W., Yu, H., et al. (2014). Holey graphene frameworks for highly efficient capacitive energy storage. Nat. Commun. 5:4554. doi: 10.1038/ncomms5554
Zhai, T., Lu, X., Ling, Y., Yu, M., Wang, G., Liu, T., et al. (2014). A New Benchmark Capacitance for Supercapacitor Anodes by Mixed-Valence Sulfur-Doped V 6 O 13- x. Adv. Mater. 26, 5869–5875. doi: 10.1002/adma.201402041
Zhai, T., Lu, X., Wang, H., Wang, G., Mathis, T., Liu, T., et al. (2015). An Electrochemical Capacitor with Applicable Energy Density of 7.4 Wh/kg at Average Power Density of 3000 W/kg. Nano Lett., 15, 3189–3194. doi: 10.1021/acs.nanolett.5b00321
Zhang, C., Mahmood, N., Yin, H., Liu, F., and Hou, Y. (2013a). Synthesis of Phosphorus-Doped Graphene and its Multifunctional Applications for Oxygen Reduction Reaction and Lithium Ion Batteries. Adv. Mater. 25, 4932–4937. doi: 10.1002/adma.201301870
Zhang, F., Tang, J., Shinya, N., and Qin, L.-C. (2013b). Hybrid graphene electrodes for supercapacitors of high energy density. Chem. Phys. Lett. 584, 124–129. doi: 10.1016/j.cplett.2013.08.021
Zhang, F., Yuan, C., Zhu, J., Wang, J., Zhang, X., and Lou, X. W. (2013c). Flexible Films Derived from Electrospun Carbon Nanofibers Incorporated with Co 3 O 4 Hollow Nanoparticles as Self-Supported Electrodes for Electrochemical Capacitors. Adv. Funct. Mater. 23, 3909–3915. doi: 10.1002/adfm.201203844
Zhang, L., Zhang, F., Yang, X., Long, G., Wu, Y., Zhang, T., et al. (2013d). Porous 3D graphene-based bulk materials with exceptional high surface area and excellent conductivity for supercapacitors. Sci. Rep. 3:1408. doi: 10.1038/srep01408
Zhang, F., Liu, T., Hou, G., Kou, T., Yue, L., Guan, R., et al. (2016). Hierarchically porous carbon foams for electric double layer capacitors. Nano Res. 9, 2875–2888. doi: 10.1007/s12274-016-1173-z
Zhang, F., Liu, T., Li, M., Yu, M., Luo, Y., Tong, Y., et al. (2017). Multiscale Pore Network Boosts Capacitance of Carbon Electrodes for Ultrafast Charging. Nano Lett. 17:3097. doi: 10.1021/acs.nanolett.7b00533
Zhang, X., Gong, J., Zhang, K., Zhu, W., Li, J.-C., and Ding, Q. (2019). All-solid-state asymmetric supercapacitor based on porous cobalt selenide thin films. J. Alloys Compounds 772, 25–32. doi: 10.1016/j.jallcom.2018.09.023
Zhang, X., Shi, W., Zhu, J., Kharistal, D. J., Zhao, W., Lalia, B. S., et al. (2011). High-power and high-energy-density flexible pseudocapacitor electrodes made from porous CuO nanobelts and single-walled carbon nanotubes. ACS Nano 5:2013. doi: 10.1021/nn2020453
Zhang, Z., Xiao, F., Qian, L., Xiao, J., Wang, S., and Liu, Y. (2014). Facile Synthesis of 3D MnO 2 -Graphene and Carbon Nanotube-Graphene Composite Networks for High-Performance, Flexible, All-Solid-State Asymmetric Supercapacitors. Adv. Energy Mater. 4:1400064. doi: 10.1002/aenm.201400064
Zhao, C., and Zheng, W. (2015). A Review for Aqueous Electrochemical Supercapacitors.(Report)(Author abstract). Front. Energy Res. 3:23. doi: 10.3389/fenrg.2015.00023
Zhao, M. Q., Ren, C. E., Ling, Z., Lukatskaya, M. R., Zhang, C., Van Aken, K. L., et al. (2015). Flexible MXene/Carbon Nanotube Composite Paper with High Volumetric Capacitance. Adv. Mater. 27, 339–345. doi: 10.1002/adma.201404140
Zhao, X., Hou, Y., Wang, Y., Yang, L., Zhu, L., Cao, R., et al. (2017). Prepared MnO 2 with different crystal forms as electrode materials for supercapacitors: experimental research from hydrothermal crystallization process to electrochemical performances. RSC Adv. 7, 40286–40294. doi: 10.1039/C7RA06369E
Zhao, Z., Richardson, G. F., Meng, Q., Zhu, S., Kuan, H.-C., and Ma, J. (2016). Pedot-based composites as electrode materials for supercapacitors. Nanotechnology 27:042001. doi: 10.1088/0957-4484/27/4/042001
Zhong, C., Deng, Y., Hu, W., Qiao, J., Zhang, L., and Zhang, J. (2015). A review of electrolyte materials and compositions for electrochemical supercapacitors. Chem. Soc. Rev. 44, 7484–7539. doi: 10.1039/C5CS00303B
Zhou, C., Zhang, Y., Li, Y., and Liu, J. (2013). Construction of High-Capacitance 3D CoO@Polypyrrole Nanowire Array Electrode for Aqueous Asymmetric Supercapacitor. Nano Lett. 13, 2078–2085. doi: 10.1021/nl400378j
Zhou, M., Wu, H. B., Bao, J., Liang, L., Lou, X. W., and Xie, Y. (2013). Ordered Macroporous BiVO 4 Architectures with Controllable Dual Porosity for Efficient Solar Water Splitting. Angewandte Chem. Int. Edn. 52, 8579–8583. doi: 10.1002/anie.201302680
Zhou, Y., Ghaffari, M., Lin, M., Parsons, E. M., Liu, Y., Wardle, B. L., et al. (2013). High volumetric electrochemical performance of ultra-high density aligned carbon nanotube supercapacitors with controlled nanomorphology. Electrochim. Acta 111:608. doi: 10.1016/j.electacta.2013.08.032
Zhu, C., Liu, T., Qian, F., Han, T. Y.-J., Duoss, E. B., Kuntz, J. D., et al. (2016). Supercapacitors Based on Three-Dimensional Hierarchical Graphene Aerogels with Periodic Macropores. Nano Lett. 16:3448. doi: 10.1021/acs.nanolett.5b04965
Zhu, H., Peng, S., and Jiang, W. (2013). Electrochemical Properties of PANI as Single Electrode of Electrochemical Capacitors in Acid Electrolytes. Sci. World J. 2013:940153. doi: 10.1155/2013/940153
Zhu, J., Cao, L., Wu, Y., Gong, Y., Liu, Z., Hoster, H. E., et al. (2013). Building 3D Structures of Vanadium Pentoxide Nanosheets and Application as Electrodes in Supercapacitors. Nano Lett. 13, 5408–5413. doi: 10.1021/nl402969r
Keywords: electrochemical energy storage, porous carbon materials, supercapacitors, supercapacitor (SC), activated-carbon, 3D carbon materials
Citation: Galek P, Mackowiak A, Bujewska P and Fic K (2020) Three-Dimensional Architectures in Electrochemical Capacitor Applications – Insights, Opinions, and Perspectives. Front. Energy Res. 8:139. doi: 10.3389/fenrg.2020.00139
Received: 01 April 2020; Accepted: 05 June 2020;
Published: 03 September 2020.
Edited by:
Tianyu Liu, Virginia Tech, United StatesReviewed by:
Yu Song, Northeastern University, ChinaCopyright © 2020 Galek, Mackowiak, Bujewska and Fic. This is an open-access article distributed under the terms of the Creative Commons Attribution License (CC BY). The use, distribution or reproduction in other forums is permitted, provided the original author(s) and the copyright owner(s) are credited and that the original publication in this journal is cited, in accordance with accepted academic practice. No use, distribution or reproduction is permitted which does not comply with these terms.
*Correspondence: Krzysztof Fic, a3J6eXN6dG9mLmZpY0BwdXQucG96bmFuLnBs
Disclaimer: All claims expressed in this article are solely those of the authors and do not necessarily represent those of their affiliated organizations, or those of the publisher, the editors and the reviewers. Any product that may be evaluated in this article or claim that may be made by its manufacturer is not guaranteed or endorsed by the publisher.
Research integrity at Frontiers
Learn more about the work of our research integrity team to safeguard the quality of each article we publish.