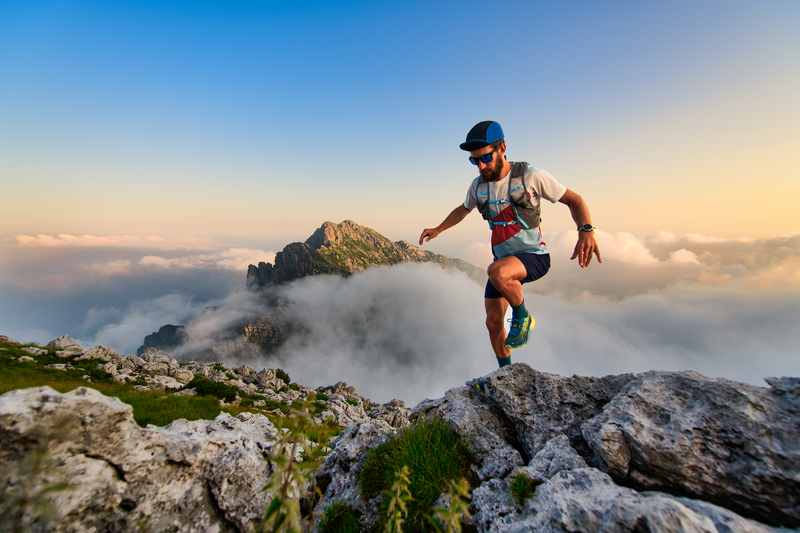
95% of researchers rate our articles as excellent or good
Learn more about the work of our research integrity team to safeguard the quality of each article we publish.
Find out more
REVIEW article
Front. Energy Res. , 07 July 2020
Sec. Carbon Capture, Utilization and Storage
Volume 8 - 2020 | https://doi.org/10.3389/fenrg.2020.00111
This article is part of the Research Topic Beyond Current Research Trends in CO2 Utilization View all 11 articles
There is increasing interest in plasma technology for CO2 conversion because it can operate at mild conditions and it can store fluctuating renewable electricity into value-added compounds and renewable fuels. This perspective paper aims to provide a view on the future for non-specialists who want to understand the role of plasma technology in the new scenario for sustainable and low-carbon energy and chemistry. Thus, it is prepared to give a personal view on future opportunities and challenges. First, we introduce the current state-of-the-art and the potential of plasma-based CO2 conversion. Subsequently, we discuss the challenges to overcome the current limitations and to apply plasma technology on a large scale. The final section discusses the general context and the potential benefits of plasma-based CO2 conversion for our life and the impact on climate change. It also includes a brief analysis on the future scenario for energy and chemical production, and how plasma technology may realize new paths for CO2 utilization.
Plasma, also called the “fourth state of matter,” is an ionized gas consisting of electrons, various types of ions, radicals, excited atoms, and molecules, besides neutral ground state molecules. Thus, it is a very reactive environment, making it of interest for many applications (Bogaerts et al., 2002). Plasma is created by applying electricity to a gas. The light electrons are heated by the applied electric field, and they activate the gas molecules by excitation, ionization, and dissociation, creating the above-mentioned reactive species and allowing chemical reactions to occur at mild conditions (ambient temperature and pressure). This makes plasma of particular interest for CO2 conversion because the electrons can activate the CO2 molecules, giving rise to new products without the need to heat the entire gas (Fridman, 2008; Snoeckx and Bogaerts, 2017). Thus, plasma technology can provide an energy-efficient method for CO2 conversion, as discussed in section on Potential of plasma technology to reduce CO2 emissions, although more research is needed to overcome current limitations, as will be discussed in section What Is Needed to Overcome Current Limitations of Plasma-Based CO2 Conversion?
This perspective paper, like the others in this special issue, is not written as a classical review for specialists, but rather to provide to non-specialists an understanding of the background for this technology, the prospects and gaps, and the role of plasma technology in the new scenario for sustainable and low-carbon energy and chemistry. Therefore, it does not enter too much into mechanistic and other specialized aspects, but rather aims to give a bird's-eye overview of the field and the problems to be overcome in order to pass from lab-scale to industrial application. In fact, although plasma technologies are already applied commercially for a few sectors like waste incineration, their use in CO2 conversion to fuels and chemicals, and in general for the conversion of small molecules such as CO2, CH4, H2O, and N2, is still mainly at the laboratory scale. Nevertheless, this is a crucial aspect for the future of sustainable energy and chemistry, from a circular economy to energy/chemistry based on perennial (renewable) sources rather than on fossil fuels (Lanzafame et al., 2017a; Centi et al., 2019; Centi and Perathoner, 2020). As a consequence, it is necessary that non-specialists understand the possible contribution of plasma technologies to address this challenge.
Although this paper provides a status on the use of non-thermal plasma for CO2 conversion, we do not aim to give a systematic review of the state-of-the-art, and we refer to recent reviews on the topic (Snoeckx and Bogaerts, 2017; Chen et al., 2018; Puliyalil et al., 2018; Qin et al., 2018; Debek et al., 2019). Rather, we aim to give a glimpse about possibilities and issues in this area from our personal perspective. For this reason, the paper is largely based on our own results, put in a general perspective, and also provides some background information on the technology, as plasma technology is not yet well-known by the scientific community.
The general question is why plasma technology is relevant for CO2 conversion in view of the future of energy and chemistry from a sustainable, low-carbon perspective. These aspects will be discussed in section Benefits for Our Life and Impact on Climate Change, but are briefly introduced here in relation to competitive technologies. There are two overarching topics when discussing the future of energy and chemistry, particularly in regions such as Europe, where there are political commitments about the reduction of CO2 and other greenhouse gas (GHG) emissions (now with a target of 50% reduction for the year 2030), and where large initiatives such as the European Green Deal planning, a global investment of over 1 trillion Euro, are put into action with goals such as: (i) closing the carbon cycle (as part of circular economy) of CO2 emissions and putting them back in use as carbon sources, and (ii) using renewable (perennial) energy sources (RES) rather than deriving from fossil fuels.
Being low but not lowest in thermodynamic energy content, CO2 should react with other compounds to form chemicals with even lower thermodynamic energy level (inorganic carbonates) or react with higher energy level compounds to form chemicals/fuels with higher thermodynamic energy. The second route can involve either chemicals produced according to current routes (for example, reaction of CO2 with epoxides to form cyclic carbonates) or produced through the use of RES, such as H2, by electrolysis using electrical energy produced from wind or photovoltaic panels. The second option is preferable because it introduces RES in the energy/chemistry value chain, and thus reduces the dependence on fossil fuels and CO2 emissions (Centi and Perathoner, 2010; Perathoner and Centi, 2014; Ampelli et al., 2015).
There are two main options for converting CO2 to chemicals/fuels by using RES. The first, already developed at demonstration plants, is the production of H2 by electrolyzers followed by the catalytic conversion of CO2 to different products, from methane (power-to-X, Centi and Perathoner, 2020) to methanol or hydrocarbons. The second is the direct CO2 conversion in electrocatalytic reactors (with the electrical energy produced from a separate RES unit) or in photoelectrocatalytic (PEC) reactors, where the solar cell is integrated into the electrocatalytic cell (García de Arquer et al., 2020). Although the latter is still the more challenging, it provides two main advantages: (i) it greatly reduces several of the energy losses due to coupling different components, and (ii) it avoids energy losses related to the overpotential in forming H2 (from H+/e− which are the effective primary products in water electrolysis) and in using H2. In the current approach, electrolysis followed by thermal catalytic conversion, the overall efficiency is limited by the different steps operating at different conditions and having different dynamics. For example, in coupling H2 (produced in electrolyzers) to a second stage of catalytic conversion of CO2 to methane or methanol (the two most common examples of power-to-X technologies), there is the need to compress and heat H2, and there are severe issues in handling the different dynamics of the process and heat integration, and costs are relatively high.
This is thus considered the first generation, today at the stage of pilot/demo scale, and can achieve application depending on cost of renewable electrical energy, carbon taxes (or subsidies), and other aspects. However, moving to the second and third generation processes, which are those where the generation of H2 equivalent (H+/e−) and CO2 conversion are integrated in a single electrocatalytic device or when the photogeneration stage of the current/potential for water oxidation (i.e., H2O to O2 + H+/e−) is integrated in a single PEC device, can increase process efficiency and lower the costs around 15–20% and up to 25–30%, respectively (Perathoner and Centi, 2019).
In addition to these possibilities, direct photocatalytic conversion of CO2 to chemicals/fuels is possible. Although attractive, the main issues are the low productivity and the combined (in a single compartment) formation of products of reduction (methane or methanol from CO2) and oxidation (O2 derived from water), creating issues of safety (explosivity) and costs of separation. Although many researchers have worked on this approach, the exploitability of the results still appears rather distant. A different alternative is the use of solar radiation, not to generate charge separation and thus photoinduced electrical currents or reactions, but simply to provide the heat for running thermal reactions. This is the concept of concentrated solar power (CSP) plants. For example, by thermal heating at high temperature (above about 1,200°C), metal oxides may be reduced, which react then at lower temperatures (800–900°C) with CO2 or H2O to generate CO and H2, respectively. Notwithstanding intense research, the many issues on productivity, stability, and costs (Centi and Perathoner, 2010) have strongly hindered development beyond pilot units, and current interest on this route is decreasing.
The different possibilities of using RES in CO2 conversion technologies have been discussed in detail by Navarrete et al. (2017). In summary, electrocatalytic and (in a longer-term perspective) PEC approaches appear as the more promising routes in competition with plasma. Currently the major issue with the electrocatalytic route is to realizing high current densities together with high Faradaic efficiencies (FE) to the target compound. As a rough indication, current densities should be above 100 mA/cm2 and FE > 90%. This is currently feasible only with formic acid, which is a 2e− reduction of CO2. More challenging reactions such as methanol formation, which is a 6e- reduction, have still significantly lower performances. Some results have been also reported with higher current densities. For example, García de Arquer et al. (2020) reported current densities up to 1 A in the reduction of CO2 to ethylene, but at low FE (45%) and using as electrolyte 7 M KOH (pH ≈ 15), which creates severe problems with materials stability. Zheng et al. (2019) reported high current densities (around 80 mA/cm2) and high FE (above 90%) to CO in a 10 × 10 cm electrode cell, but with performances lowering in 6 h tests, thus indicating stability issues. In addition, CO must be further converted by catalytic routes in the presence of H2 (from renewable sources), so the effective advantage with respect to direct catalytic conversion of CO2 is not very evident.
It is out of scope here to discuss in more detail other approaches to convert CO2 by using RES, but these examples indicate how there is continuous progress in these alternative routes, though the results are still far from the possibility of application, at least in the short run. Therefore, when comparing plasma technology with these alternative possibilities, we need to keep in mind that it is an area still under fast development and thus not well-consolidated to allow a reliable comparison and indication of which route is the most promising, even if attempts have been made (Liu M. et al., 2019).
Plasma, as will be discussed in more detail in the following sections, has some advantages: (i) plasma is generated by electricity and can simply be switched on/off, allowing storage of fluctuating energy; (ii) the reaction occurs virtually in the entire volume of the reactor (although mainly confined at the discharges), as in thermal catalysis (i.e., the reactions occur on the catalyst, but it occupies a significant fraction of the volume of the reactor), while for electro- and photo-catalytic processes the reaction is on the surface of the electrode (thus, to simplify, a 3D vs. 2D approach); (iii) it is a relatively low cost technology in comparison with photo- and electro-catalytic routes requiring more sophisticated and costly devices and materials. On the other hand, as will be clarified later, the complexity of the phenomena in plasma chemistry and physics, and even more when coupled with a solid catalyst, require further progress in understanding to achieve high productivity, energy efficiency, and energy selectivity. Scalability of the technology is another challenge in exploiting the potential of plasma technology for industrial applications.
Different types of plasmas are being investigated for CO2 conversion, including dielectric barrier discharges (DBDs), microwave (MW) plasmas, gliding arc (GA) plasmas, atmospheric pressure glow discharges (APGDs), nanosecond-pulsed discharges, and corona and spark discharges. They differ in the way the electricity is applied and the actual value of the applied electric field, reactor configuration, pressure, power, etc. These differences manifest themselves in their performance toward CO2 conversion, as discussed in detail by Snoeckx and Bogaerts (2017).
The three most common plasma types for CO2 conversion are DBD, MW, and GA plasma, and they are depicted in Figure 1 (Bogaerts and Neyts, 2018). A DBD (Figure 1A) is created by applying an electric field between two electrodes, of which at least one is covered by a dielectric barrier. The two electrodes can be parallel plates, but for CO2 conversion, a cylindrical configuration in which the two electrodes are concentric cylinders (see Figure 1A), is more appropriate because it minimizes the amount of gas bypassing the plasma region. A DBD operates at atmospheric pressure and (near) room temperature. It has a simple design, making it easy for upscaling and commercial applications, as demonstrated a few decades ago for ozone synthesis (Kogelschatz, 2003). Moreover, the simple design allows also easy implementation of catalyst materials inside the plasma in order to better control the selective production of value-added compounds. Indeed, as mentioned in the introduction, plasma is very reactive, producing a plethora of different species, but the latter can react toward many different compounds. Therefore, if the selective production of specific molecules is targeted, plasma should be combined with catalysts, yielding so-called plasma catalysis (Neyts et al., 2015). Most studies on plasma catalysis have indeed been reported with DBD plasma reactors in a packed-bed configuration, where packing beads (of a few mm diameter) are typically coated by catalyst materials. A DBD reactor provides reasonable conversion but is not energy efficient compared to other plasma types, as shown in Figures 2, 3 below (Snoeckx and Bogaerts, 2017). The reason will be explained in next section.
Figure 1. Schematic picture of the three most common plasma reactors for CO2 conversion, i.e., dielectric barrier discharge (DBD) (A), microwave (MW) plasma (B), and gliding arc (GA) discharge, in classical configuration (C), and cylindrical geometry, called gliding arc plasmatron (GAP) (D). The plasma is each time presented in purple. Adopted from Bogaerts and Neyts (2018) with permission.
Figure 2. Comparison of all data from literature for CO2 splitting in different plasma reactors, illustrating the energy efficiency as a function of conversion. The 60% efficiency target is also indicated. Adopted from Snoeckx and Bogaerts (2017) with permission.
Figure 3. Comparison of all data from literature for DRM in different plasma reactors, illustrating the energy cost as a function of conversion. The target energy cost of 4.27 eV/molec for syngas production (corresponding to 60% efficiency target) is also indicated. The y-axis is reversed to allow easier comparison with Figure 2. Adopted from Snoeckx and Bogaerts (2017) with permission.
A MW plasma arises when applying MW power to a quartz tube filled with gas (Figure 1B). It can operate in a pressure range from a few mbar up to 1 bar, but typically the CO2 conversion and corresponding energy efficiency are better at reduced pressure (ca. 100–200 mbar) than at atmospheric pressure, for the reason explained in next section. Indeed, energy efficiencies up to 80% and even 90% (for CO2 conversions around 20 or 10%, respectively) have been reported for MW plasmas at 100–200 mbar under subsonic and supersonic flow conditions, respectively (Legasov et al., 1978; Asisov et al., 1983; Fridman, 2008), although it must be realized that these record values have not been reproduced since then. The gas temperature can easily rise to above 3,000 K at (sub)atmospheric pressure. For this reason, catalyst materials cannot be easily implemented inside a MW plasma, but they can be placed after the plasma reactor (so-called “post-plasma catalysis”), although not many studies have reported on MW-plasma catalysis yet.
In a classical GA plasma, also called “2D GA plasma,” an electric potential difference is applied between two flat diverging electrodes (see Figure 1C). An arc is created at the shortest interelectrode distance, and as a result of the gas flow it glides along the surface of the electrodes toward larger interelectrode distances until it extinguishes, and a new arc is created again at the shortest distance. A GA plasma typically operates at atmospheric pressure, and exhibits quite good energy efficiency (see Figures 2, 3); the reason will be explained in next section. However, the conversion is limited (typically about 10%), because of the limited residence time of the gas inside the arc plasma. Indeed, the gas flow rate must be high enough for the arc to glide along the electrodes.
To overcome the limitation of short residence time, several 3D GA plasma configurations have been developed, such as the rotating GA and the GA-Plasmatron (GAP), in which the reactor is cylindrical and the gas typically enters the reactor tangentially, leading to a vortex gas flow (see Figure 1D) (Nunnally et al., 2011). When the outlet diameter is considerably smaller than the reactor diameter, the gas first flows upwards in an outer vortex, creating an isolating effect from the hot plasma arc in the center (see below) toward the walls. Once the gas reaches the end of the reactor it has lost some speed due to friction and inertia, and moves downwards in a smaller inner (reverse) vortex, before it leaves the reactor (see Figure 1D). The inner vortex flow causes stabilization of the arc in the center of the reactor, and in the ideal case, all the gas within the inner vortex passes through the arc for optimal conversion. Indeed, the arc itself is hot (around 3,000 K), causing nearly complete conversion, mainly due to thermal reactions (see next section). In reality, however, only a limited fraction of the gas actually passes through the arc, thus limiting the overall conversion to about 10%. Thus, reactor design improvements are needed to further enhance the performance, as will be discussed in section What Is Needed to Overcome Current Limitations of Plasma-Based CO2 Conversion? Like in the MW plasma, catalysts cannot easily be implemented inside the plasma reactor due to the high temperature, but placing a catalyst bed after a GA plasma reactor has provided spectacular results in terms of enhanced conversion and energy efficiency (Li et al., 2016).
Figure 2 presents an overview of the energy efficiency vs. CO2 conversion, based on all data collected from literature, for different plasma reactor types (Snoeckx and Bogaerts, 2017). Note that the energy efficiency is calculated as the product of conversion and reaction enthalpy, divided by the specific energy input (SEI), which is equal to plasma power over gas flow rate. As mentioned above, MW plasmas [as well as some radiofrequency (RF) plasmas] yield the best energy efficiency, even up to 80 and 90% (for a conversion of 10%), but these record values were all obtained at reduced pressure (100–200 mbar) and in special configurations (e.g., supersonic expansion) (Legasov et al., 1978; Asisov et al., 1983; Fridman, 2008), and have not been reproduced since then. Likewise, Silva et al. reported conversions of 50–80%, but at corresponding energy efficiencies of 10% (Silva et al., 2014), and again at reduced pressure (1–10 mbar). At (sub)atmospheric pressure, yielding temperatures of 3,000 K and above, the best energy efficiencies achieved were around 40–50 %, for a conversion around 10–20% at 100–200 mbar (den Harder et al., 2017), and up to 600 mbar (Bongers et al., 2017). Vice versa, conversion values up to 80% have also been reported for energy efficiencies of 20%, in quenching experiments at 200 mbar (Bongers et al., 2017). GA plasmas at atmospheric pressure have typical energy efficiencies around 20–40%, but for limited conversions up to 10% (Nunnally et al., 2011; Ramakers et al., 2017), although some configurations have resulted in energy efficiencies above 60% (Snoeckx and Bogaerts, 2017).
Note that an efficiency target of 60% (cf. green dashed line in Figure 2) was defined by Snoeckx and Bogaerts (2017) based on two criteria: (i) the comparison with electrochemical water splitting, being the main competitor of plasma conversion for storing renewable energy, and reaching commercial energy efficiencies of 65–75%; and (ii) the comparison with other novel technologies for CO2 conversion, which directly use solar energy (e.g., solar thermochemical conversion), and for which a solar-to-fuel conversion efficiency of 20% was stated to be industrially competitive. Based on a solar panel efficiency of 25%, plasma-based CO2 conversion at an energy efficiency of 60–80% would thus give rise to a competitive solar-to-fuel efficiency of 15–20%.
It is clear from Figure 2 that several of the (reduced pressure) MW (& RF) plasma results and a few of the (atmospheric pressure) GA plasma results obtained in literature are already above this efficiency target. The DBD results, on the other hand, are clearly below the efficiency target, with energy efficiencies up to maximum ca. 10%, albeit at reasonable conversion values up to 40%.
A similar collection of data is presented in Figure 3 for dry reforming of methane (DRM) (Snoeckx and Bogaerts, 2017). The combined conversion of CH4 and CO2 allows the production of other compounds besides CO and O2, such as syngas (CO/H2), higher hydrocarbons, and oxygenates. Note that the y-axis in this figure does not present the energy efficiency, but the energy cost, showing the highest values at the bottom and the lowest (i.e., best) values at the top of the axis, to allow easy comparison with Figure 2. Indeed, the energy efficiency could not be determined from all data points in literature because it is only possible when reporting all products formed, which was often not the case. The energy cost is determined as the ratio of SEI over conversion, so it can be defined even if not all products are identified. Note that an efficiency target of 4.27 eV/molec was determined by Snoeckx and Bogaerts (2017) (cf. green dashed line in Figure 3) based on the formation of syngas (corresponding to the 60% energy efficiency target).
The best results are now obtained in GA plasmas, with several data points already above the efficiency target, showing energy costs down to almost 1 eV/molec for conversions up to 40% (Li et al., 2016; Cleiren et al., 2017; Slaets et al., 2020). MW plasmas, on the other hand, have not been extensively studied yet for DRM, and the few results found in literature exhibit a very high energy cost. This is quite surprising in view of the very promising results for CO2 splitting (cf. Figure 2), and it indicates the need for more research to exploit the potential of MW plasmas for DRM as well. Most results have been obtained in DBD reactors, often in combination with catalysis (packed bed DBDs), but they are again characterized by high energy costs, making them not competitive with other technologies. However, it should be noted that the efficiency target is based on the formation of syngas. If more valuable products, such as olefins or oxygenates, could selectively be formed, the efficiency target would not be so strict to be competitive with other technologies, where these products would be formed in a two-step process. This means that DBD plasmas, in spite of their low energy efficiency, could be still of interest for their easy implementation of catalysts, but only if suitable catalysts can be designed that selectively produce these value-added compounds in a one-step process in the DBD plasma. This will be further discussed in section What Is Needed to Overcome Current Limitations of Plasma-Based CO2 Conversion? Finally, Figure 3 also shows the results obtained in some other plasma types, such as corona, ns-pulsed, and spark discharges, as well as APGDs. APGDs especially provided very promising results, with energy costs down to 1 eV/molec at conversions up to 80–90%. However, the data points are limited, and clearly more research is needed to verify these results and to further improve the capabilities of the APGD and other plasma types for DRM.
Besides CH4, H2O or H2 can also be added as H-sources to produce value-added compounds from CO2. However, only very limited data are reported in literature for the combined CO2/H2O or CO2/H2 conversion (see Figure 4). Note that in this case, no efficiency target could be defined. Indeed, the latter requires knowledge on all products formed, and they are generally not all reported in literature for CO2/H2O or CO2/H2 mixtures. It is clear from this figure that the values reported for energy cost and conversion in these gas mixtures are not yet competitive with those obtained already for DRM (cf. Figure 3). The reason for this is the (virtual) absence of CH3 and CH2 radicals in these gas mixtures, which would contribute to the CO2 conversion, as predicted by numerical simulations (De Bie et al., 2016). Moreover, the fact that CHO is formed (H + CO + M → CHO + M), which can form CO2 again (CHO + O → CO2 + H), as well as the recombination of CO with OH radicals, again forming CO2, both limit the CO2 conversion, as will be discussed in section What Is Needed to Overcome Current Limitations of Plasma-Based CO2 Conversion? Nevertheless, the combined conversion of CO2 and H2O would be of great interest, as H2O is the cheapest H-source. Therefore, more research should be performed to explore the possibilities and overcome the current limitations of the combined plasma-based CO2/H2O conversion.
Figure 4. Comparison of all data from literature for the combined CO2 + H2O (*) and the combined CO2 + H2 (•) conversion in different plasma reactors, illustrating the energy cost per converted CO2 molecule as a function of conversion. Some data are recalculated from the original references, to account for (among others) dilution effects.
It is clear from Figures 2, 3 that MW plasmas (especially at reduced pressure, and in case of CO2 conversion) and GA plasmas exhibit much better energy efficiency than DBD reactors. In earlier work (e.g., Fridman, 2008) this was attributed to the role of vibrational excitation in MW and GA plasmas, although more recent insights point toward thermal conversion in these plasmas at practical operating conditions (see below).
In the past decade, many papers, both experimental (e.g., Silva et al., 2014, 2017; Klarenaar et al., 2017, 2018, 2019; Stewig et al., 2020; Terraz et al., 2020; van den Bekerom et al., 2020) and computational (e.g., Kozák and Bogaerts, 2014, 2015; Pietanza et al., 2015, 2017a,b, 2018a,b, 2020; Berthelot and Bogaerts, 2016, 2018; Capitelli et al., 2017, Heijkers and Bogaerts, 2017; Grofulović et al., 2018; Silva et al., 2018; Vermeiren and Bogaerts, 2018, 2019; Kotov and Koelman, 2019; Terraz et al., 2020), focused on the role of the vibrational kinetics in plasmas, but mainly at low pressure conditions (few mbar up to a few hundred mbar). Most of these studies revealed the importance of the vibrational kinetics at specific conditions (low gas temperature, low pressure) and their contribution to a high energy efficiency.
It is generally assumed that the electric field applied in MW and GA plasmas is lower than in DBD plasmas, resulting in somewhat lower electron energy (or temperature) that is more suitable for electron-impact vibrational excitation of the CO2 molecules compared to electronic excitation, ionization, or dissociation. This is illustrated in Figure 5, which plots the fraction of electron energy transferred to different CO2 vibrational levels and the sum of all these levels, as well as the fraction of electron energy transferred to electronic excitation, ionization, and dissociation as a function of the reduced electric field (E/n) (Snoeckx and Bogaerts, 2017). The reduced electric field (E/n) is the ratio of electric field (E) over gas number density (n), and is usually expressed in Townsend (1 Td = 10−21 V m2). It is an important measure to distinguish the operating conditions of different plasma types. A DBD exhibits a reduced electric field around 200 Td and above, whereas MW and GA plasmas (and some other plasma types, such as APGD) typically operate around 50–100 Td (Bongers et al., 2017; Pietanza et al., 2020; van den Bekerom et al., 2020), although a recent model for determining the reduced electric field in CO2 MW plasma derived by the principle of impedance matching (Groen et al., 2019) predicted somewhat higher E/n values, i.e., 10–60 Td for the so-called contracted regime (higher pressure and power), but 80–180 Td for the so-called diffuse regime (lower pressure and power). As depicted in Figure 5, for reduced electric fields around 200 Td and above, the largest part of the electron energy (70–80%) goes to electronic excitation, while limited amounts are spent for dissociation and ionization (ca. 5%; rising with E/n), and vibrational excitation (10% of less). On the other hand, around 50–100 Td, the electron energy is too low for electron impact ionization and dissociation because their cross sections have higher thresholds, and to some extent it is also too low for electronic excitation. Hence, only 10% of the electron energy is spent for electronic excitation, while up to 90% goes into vibrational excitation, which has lower energy thresholds. If MW and GA plasmas can operate at E/n values around 50 Td, vibrational excitation is indeed the dominant electron energy loss process. Pietanza et al. (2020) predicted values of 25–42 Td for a MW plasma in the contracted regime, and 48–50 Td in the diffuse regime. Bongers et al. (2017) reported somewhat higher values in practice (70–80 Td), attributed to the high gas temperature causing a lower gas density. These values are already a bit too high for the most efficient electron energy transfer to the vibrational levels (van den Bekerom et al., 2020), but as seen in Figure 5, electron impact vibrational excitation is still the most important electron energy loss process up to E/n of 100 Td.
Figure 5. Fraction of electron energy used for different electron impact reactions with CO2, i.e., vibrational and electronic excitation, ionization and dissociation, as a function of the reduced electric field (E/n), calculated from the respective cross sections. The E/n regions characteristic for MW and GA plasma and for DBD plasma are indicated. Adopted from Snoeckx and Bogaerts (2017) with permission.
Furthermore, it is generally accepted that vibrational excitation yields energy-efficient CO2 conversion because it provides the most efficient CO2 dissociation path. This is illustrated in Figure 6, which schematically illustrates some CO2 electronic and vibrational levels (Bogaerts et al., 2015). Direct electron impact dissociation requires electron energies of at least 7 eV, as it proceeds through a dissociative electronically excited state. Knowing that theoretically, only 5.5 eV is required for C=O bond-breaking, it means that the extra energy is simply wasted. This process occurs in DBD plasmas (cf. Figure 5), explaining their lower energy efficiency (see previous section). In contrast, vibrational excitation requires significantly less energy, and once the lowest vibrational levels are populated, they can gradually populate the higher levels by vibrational-vibrational (VV) collisions, which gives rise to dissociation (cf. Figure 6). This process is called “ladder-climbing,” and requires just the minimum amount of 5.5 eV for bond-breaking. It thus provides a more efficient dissociation pathway. If this process can be exploit ed, for instance in MW and GA plasmas at specific conditions (e.g., reduced pressure or power pulsing, cf. next section), this can explain the higher energy efficiency in these plasma types.
Figure 6. Schematic picture of some CO2 electronic and vibrational levels, showing that direct electronic excitation–dissociation (as occurs in DBD plasmas) requires much more energy than stepwise vibrational excitation, or vibrational ladder climbing, which is stated to be important in MW and GA plasmas at specific conditions, and which may explain their higher energy efficiency.
However, the vibrationally excited molecules can also lose their energy upon collision with ground state molecules, called vibrational-translational (VT) relaxation. This process depopulates the vibrational levels, and is thus detrimental for energy-efficient CO2 conversion by the vibrational ladder climbing pathway. In addition, this process also heats up the gas molecules, and the rate coefficients for VT relaxation rise with higher gas temperature, further speeding up this vibrational depopulation process.
In practice, VT relaxation appears quite important in both MW plasmas at (sub)atmospheric pressure and GA plasmas, explaining their high gas temperature (3,000 K or higher; cf. section Different Plasma Types—Different Performance). Therefore, this type of plasmas is called “warm plasmas,” as their temperature is in between those of non-thermal plasmas such as DBD, which operate at (or slightly above) room temperature, and thermal plasmas, where the gas temperature reaches 10,000 K, comparable to the electron temperature. In MW and GA plasmas, the electron temperature is still higher than the gas temperature (hence, they are not really thermal plasmas), but the vibrational and gas temperature are often close to each other (i.e., VT equilibrium), so they can also be considered as quasi-thermal plasmas. For this reason, the CO2 conversion proceeds mainly by thermal reactions in MW and GA plasmas at practical operating conditions (van Rooij et al., 2015; Berthelot and Bogaerts, 2017; Bongers et al., 2017; den Harder et al., 2017; Heijkers and Bogaerts, 2017; Kotov and Koelman, 2019; van den Bekerom et al., 2019; Wolf et al., 2019, 2020).
Although the results obtained for both CO2 splitting and DRM are already very promising (see Figures 2, 3), and the performance of various plasma types is competitive with other emerging technologies (Snoeckx and Bogaerts, 2017), plasma-based CO2 conversion is up to now only performed on lab-scale and is not yet implemented in industrial processes. We believe that further improvements are needed in terms of (i) energy efficiency, (ii) conversion, and/or (iii) selectivity toward value-added compounds, before plasma reactors should be scaled up and implemented commercially.
As mentioned in the previous section, CO2 conversion by vibrational excitation, followed by VV relaxation to the highest levels, is in theory the most energy-efficient CO2 dissociation process. Therefore, a lot of experimental and modeling papers mainly focused on the vibrational pathway as the most energy-efficient CO2 dissociation process (see references in previous section). This vibrational pathway requires strong VT non-equilibrium, i.e., much higher vibrational levels than gas (translational) temperature, or in other words, a strong overpopulation of the higher vibrational levels, compared to a thermal vibrational distribution function (VDF). Such behavior can be exploited under specific conditions, e.g., MW plasmas at low pressure and supersonic expansion (Asisov et al., 1983) or pulsed MW plasmas at low pressure, depending on the pulse on-off times, as demonstrated both computationally and experimentally (Vermeiren and Bogaerts, 2019; van den Bekerom et al., 2020). Generally, modeling has revealed that this VT non-equilibrium can be realized in plasmas at low pressure, high power density, and low gas temperature (Berthelot and Bogaerts, 2017); see below.
It should be noted, however, that although the high energy efficiencies reported for low pressure MW plasmas in the 1980s (i.e., up to 80% for subsonic flow, and even up to 90% for supersonic flow conditions; e.g., Legasov et al., 1978; Asisov et al., 1983) were theoretically explained by vibrational excitation followed by vibrational ladder climbing, as depicted in Figure 6, none of the experimental or computational studies mentioned in previous section could reproduce or mimic these high energy efficiencies up to now.
Berthelot and Bogaerts (2018) modeled the different energy transfers in a low pressure (100 mbar) CO2 plasma for various conditions of reduced electric field, gas temperature, and ionization degree. At the most optimal conditions for energy-efficient CO2 conversion, a low reduced electric field (10 Td) and a low gas temperature (300 K), the model predicted a maximum conversion and energy efficiency of 32 and 47%, respectively. This is clearly lower than the record values of 80%, reported in the 1980s for low pressure subsonic MW plasmas (Legasov et al., 1978). Likewise, Vermeiren and Bogaerts (2019) were not able to predict the record energy efficiency of 90% for a MW plasma at 100 mbar and supersonic flow conditions (Asisov et al., 1983). The major limiting factor in the energy efficiency was pinpointed as the high activation energy of the reaction CO2 + O → CO + O2 (Berthelot and Bogaerts, 2018). Assuming that this activation energy can be entirely overcome, by either sufficient CO2 vibrational energy or kinetic energy of the collision partners, the model could predict an energy efficiency of up to 86% in the ideal scenario (Berthelot and Bogaerts, 2018). Note that the reason why it was not 100% is due to other losses in the kinetics of CO2 conversion, i.e., the fact that not all electron energy will go to vibrational excitation of CO2, but some fraction is also spent to the dissociation products (CO and O2). However, as mentioned, this maximum energy efficiency could not be realized in the model at practical operating conditions, where the vibrational overpopulation was not strong enough, even at ideal conditions.
On the other hand, more recent experiments reveal energy efficiencies close to 50% for (sub)atmospheric MW plasmas (van Rooij et al., 2015; Bongers et al., 2017; den Harder et al., 2017), around 30% for atmospheric pressure GA plasmas for CO2 conversion (Nunnally et al., 2011; Ramakers et al., 2017), and even up to 70–80% for DRM (i.e., energy cost of a few eV/molec) (Li et al., 2016; Cleiren et al., 2017; Slaets et al., 2020). These efficiencies, however, are all correlated to the high temperatures in the MW and GA plasmas, pointing toward the dominance of thermal conversion.
It was indeed demonstrated, both experimentally (e.g., van Rooij et al., 2015; Bongers et al., 2017; den Harder et al., 2017; van den Bekerom et al., 2019; Wolf et al., 2019, 2020) and computationally (e.g., Berthelot and Bogaerts, 2017; Heijkers and Bogaerts, 2017; Kotov and Koelman, 2019), that in practice, the vibrational non-equilibrium is not the leading mechanism in (sub)atmospheric MW or GA CO2 plasmas, and that the CO2 conversion is thermal, although Pietanza et al. (2020) suggested to act with caution on this assumption, as their model still showed clear deviations from equilibrium, even at temperatures of 3,500–5,500 K.
In previous modeling (e.g., Berthelot and Bogaerts, 2017; Heijkers and Bogaerts, 2017; Sun et al., 2017; Kotov and Koelman, 2019) and experiments (e.g., Bongers et al., 2017), suggestions were provided on how the vibration-induced pathway could be exploited to optimize the energy efficiency at practical MW and GA conditions. For instance, Bongers et al. (2017) studied CO2 dissociation in a vortex-stabilized MW plasma reactor in a wide range of conditions regarding MW field, residence time, quenching, and vortex configuration. They reported that the gas temperature was above 4,000 K, confirming dominant thermal CO2 dissociation, and they suggested strategies to enhance the conversion and energy efficiency. At that time, the general aim was still to exploit the VT non-equilibrium for maximum energy efficiency. The authors claimed that the temperature during the plasma must be as low as possible to reduce vibrational energy losses via VT relaxation, and they suggested that future experiments should focus on reducing the reduced electric field values to maximize the vibrational excitation, e.g., by increasing the MW cavity dimensions, and on reducing the gas temperature by lowering the temperature of the injected gas.
However, in recent years, there is growing insight that optimizing the vibration-induced dissociation pathway may not be the most realistic strategy. In fact, experiments indicate that thermal CO2 conversion gives rise to quite high energy efficiencies (up to 40–50%) (van Rooij et al., 2015; Bongers et al., 2017; den Harder et al., 2017; van den Bekerom et al., 2019; Wolf et al., 2019, 2020). Indeed, the rate coefficients of thermal reactions increase with gas temperature, thus enhancing the conversion. It was claimed (Wolf, 2020) that temperatures around 3,000–4,000 K are optimal for thermal conversion, to realize significant CO2 conversion at limited thermal losses. Thus, it may be wiser to focus on how to improve the energy efficiency beyond the thermal equilibrium limit instead of trying to exploit the VT non-equilibrium (van den Bekerom et al., 2019).
In the following, we first give some suggestions on how VT non-equilibrium could be exploited in theory, as this paper is meant as a perspective to give indications how to “fly toward the future” (i.e., imagine what we do not have today), but as these suggestions might not be easy to realize in practical plasma reactors, we also discuss how the energy efficiency of thermal CO2 conversion could be further improved.
Computer modeling has demonstrated that a strong VT non-equilibrium can be realized under the conditions of (i) reduced electric field values (E/n) around 50 Td (or lower), providing the most suitable electron energy for vibrational excitation; (ii) sufficient power density to create enough electrons available for vibrational excitation; (iii) low gas pressure; and (iv) low gas temperature, to limit the process of VT relaxation (Berthelot and Bogaerts, 2017). We will now discuss these different conditions one by one.
First, as shown in Figure 5 above, a reduced electric field around 50 Td is ideal for vibration-induced dissociation. While this value (or slightly higher) is reported for MW and GA plasmas, which may explain their higher energy efficiency (see section Different Dissociation Mechanisms in Different Plasma Types), DBD plasmas operate at higher E/n values. Thus, it would be of great interest if the reduced electric field in DBD plasmas could be lowered to values below 100 Td.
In theory, this can be realized in two ways: by lowering the applied electric field (E) or increasing the gas number density (n). The applied electric field is determined by the power supply, so applying lower power to a DBD may be beneficial, although the power must still be high enough to create a stable plasma. In addition, a lower power does not necessarily create a lower electric field, as a DBD plasma (operating in reactive gases, such as CO2, CH4, etc.) is typically filamentary. Hence, a lower power may also manifest itself in less filaments instead of a lower electric field in these filaments (Peeters et al., 2015; Ozkan et al., 2016). Thus, it would not be straightforward to control the electric field values in a DBD plasma.
In addition, CO2 conversion is most often performed in packed bed DBDs, in the context of plasma catalysis. Modeling has revealed that the electric field is typically enhanced at the contact points between the packing beads, resulting in a higher electron energy (Van Laer and Bogaerts, 2016). This can give rise to more electron impact electronic excitation, ionization, and dissociation for the same applied power as in a non-packed DBD reactor, explaining the higher energy efficiency of CO2 conversion in a packed bed DBD than in a non-packed reactor, at least when the effect is not compensated by the shorter residence time of the gas in the reactor due to the presence of the packing (Mei et al., 2015; Van Laer and Bogaerts, 2015; Zeng et al., 2015; Michielsen et al., 2017; Uytdenhouwen et al., 2018). However, the enhanced electron energy will give rise to less vibrational excitation, hence this effect is the opposite of exploiting conditions for enhancing the vibration-induced dissociation pathway. In other words, it is not clear whether a lower electric field, which is more suitable for vibrational excitation, can effectively be realized in a DBD.
Recent modeling, albeit in N2 plasma, has however indicated that vibrational excitation may be important in DBD, mainly in between the microdischarge filaments (so-called afterglows, characterized by lower E/n), and even inside the filaments, depending on how the power is distributed among the filaments and the afterglows (van ‘t Veer et al., 2020). Because it would be highly beneficial to realize VT non-equilibrium in DBD plasmas operating in CO2 as well, the effect of power introduction (and distribution) in DBD plasmas should be thoroughly investigated and verified experimentally, with the aim to optimize the E/n and thus the role of vibration-induced dissociation.
On the other hand, as mentioned above, the reduced electric field can also be lowered by a higher gas number density, and this should be easier to realize, i.e., by applying a higher gas pressure (above 1 bar). This was for instance demonstrated by Belov et al. (2017), resulting in a higher CO2 conversion at 1.5 bar compared to 1 bar (30% vs. 25%). However, a further increase in pressure did not result in a higher CO2 conversion because other effects play a role as well, as the higher pressure also resulted in more intense but fewer microdischarge filaments. In general, however, it is not the number of filaments that defines the efficiency, but their characteristics (in terms of average electric field and thus average electron energy). Thus, more intense filaments, with higher electric field, would give rise to less vibrational excitation. In addition, it should be realized that a higher pressure (or gas number density) would increase VT relaxation, but we expect this to be less critical as the gas temperature in DBD plasmas is typically not much above room temperature, and the rate coefficients of VT relaxation rise with temperature, so this process is probably not critical at typical DBD temperatures. A more obvious problem would be that a higher gas number density would also increase the required electric field strength to ignite and sustain the discharge, and thus the net E/n may be the same, as the higher electric field (E) would compensate for the higher gas number density (n).
Second, another way to enhance vibration-induced dissociation could be to apply a higher power density in MW or GA plasmas, as this will produce more electrons, which can give rise to more electron impact vibrational excitation, leading to a more pronounced overpopulation of the higher vibrational levels as predicted by modeling (Berthelot and Bogaerts, 2017). As illustrated in Figure 7, at low gas temperature (300 K), the VDF is clearly non-thermal, with strong overpopulation of the higher vibrational levels, especially at high power density. At 2,000 K, the effect of power density is less pronounced, but still the VDF is higher at higher power density. However, it must be mentioned that a higher power density will not only enhance the vibrational excitation, but also electronic excitation, ionization, and dissociation, which are not beneficial for energy-efficient CO2 conversion. Even more, a higher power density will enhance the electron temperature, thereby promoting electronic excitation, ionization, and dissociation, above vibrational excitation. In addition, a higher power density will enhance the gas temperature, leading to more VT relaxation, which is detrimental for vibration-induced dissociation. Thus, in the ideal case of strong VT non-equilibrium, a high power density must be accompanied by a controlled (low) gas temperature (see also below). This is, however, very difficult to realize in practice. Note that the VDFs plotted in Figure 7 were obtained from non-self-consistent model calculations to evaluate the effect of individual parameters. The gas temperature was fixed, and was thus not rising with power, as would be expected from self-consistent calculations.
Figure 7. Calculated vibrational distribution functions (VDFs) in a MW plasma, for a gas temperature of 300 K (A) and 2,000 K (B) and a pressure of 100 mbar, at two different power densities. Adopted from Berthelot and Bogaerts (2017) with permission.
Third, a lower gas pressure will give rise to less VT relaxation due to a lower number density of gas molecules, and thus it will also result in a more pronounced overpopulation of the higher vibrational levels, as again predicted by modeling (see Figure 8; Berthelot and Bogaerts, 2017). This will yield a larger contribution of the vibrational dissociation pathway, and thus more energy-efficient CO2 conversion, as claimed already back in the 1980s, for low pressure MW plasmas (Legasov et al., 1978; Asisov et al., 1983; Fridman, 2008). This also explains why most studies on the vibrational kinetics in CO2 plasmas (both experimental and computational) in the last decade were performed at reduced pressure (see references in section Different Dissociation Mechanisms in Different Plasma Types). However, a low pressure is less valuable for industrial processes, and the additional cost of pumping should also be accounted for in determining the overall energy cost or energy efficiency of the process.
Figure 8. Calculated vibrational distribution functions (VDFs) in a MW plasma, for a gas temperature of 300 K (A) and 2,000 K (B), at two different pressures and power densities. Adopted from Berthelot and Bogaerts (2017) with permission.
Fourth, a lower gas temperature will also limit VT relaxation, and thus promote the VT non-equilibrium, as mentioned above. However, it should be realized that the gas temperature in the plasma typically follows from the applied power and the various chemical reactions, and cannot easily be controlled. A reduced pressure typically gives rise to a lower gas temperature due to reduced VT relaxation (see above), but operation at atmospheric pressure is preferable from industrial perspective, as discussed above. Gas cooling could be applied, but it would also increase the overall energy cost.
A promising way to control the gas temperature is by applying pulsed power with a suitable duty cycle. Model calculations have revealed that the ideal pulse-on time is around 40–100 μs, with a pulse-off time of 100 ms and above, at a reduced pressure of 100 mbar (see Figure 9; Vermeiren and Bogaerts, 2019). Indeed, the pulse-on time should be long enough to reach a high vibrational temperature (i.e., allowing enough time for the VDF to build up to the highest levels), but not too long to avoid having the gas temperature rise as well. The characteristic timescale for VV relaxation is indeed much shorter than for VT relaxation, at least at temperatures below 2,500 K, and thus the vibrational temperature rises faster than the gas temperature (Vermeiren and Bogaerts, 2019). In addition, the pulse-off time should be long enough so that the gas temperature can cool down again before the next pulse starts, although it should be realized that for too long pulse-off time, the CO2 gas would not experience enough pulses within its residence time in the plasma for sufficient dissociation.
Figure 9. Calculated energy efficiency and conversion in a pulsed MW plasma, as a function of interpulse time (toff), for five different pulse-on times (ton), at an SEI of 1 eV/molec and a pressure of 100 mbar. The horizontal line represents the conversion and energy efficiency of a continuous plasma at the same SEI value. Adopted from Vermeiren and Bogaerts (2019) with permission.
van den Bekerom et al. (2019) performed very interesting experiments on a 100 μs pulsed MW plasma in CO2 at 25 mbar, 2.45 GHz, and up to 600 W peak power. The duty cycle was kept below 1% to ensure a low gas temperature at the discharge onset. Raman and Rayleigh scattering yielded space- and time-resolved vibrational, rotational, and gas temperatures. During the first 40 μs, the authors found a significant inter-vibrational non-equilibrium, with the temperature of the symmetric stretch and bending modes around 750 K and the temperature of the asymmetric stretch mode (generally known to be important for vibration-induced dissociation of CO2) around 1,150 K. In addition, they observed a maximum rotational-vibrational non-equilibrium after 60 μs, in which the rotational temperature was about half the vibrational temperature of the asymmetric stretch mode (1,150 K). The rotational and translational temperatures were found to be in equilibrium at all times. Thus, in agreement with the model predictions of Vermeiren and Bogaerts (2019), the authors reported a VT non-equilibrium for the entire pulse duration (100 μs) in this 25 mbar pulsed MW plasma with small duty cycle (below 1%), albeit less predominant than often assumed.
Finally, another way to limit the gas heating, which causes at the same time a pressure drop, can be realized by using a Laval nozzle, yielding supersonic expansion of the gas. In this way, the inlet and outlet pressure can still be atmospheric (or above), being compatible with industrial operations. Modeling has indeed predicted that supersonic expansion leads to a pronounced non-equilibrium VDF (see Figure 10, Vermeiren and Bogaerts, 2018), hence promoting vibration-induced dissociation, but the gas pressure and temperature rise again after the shockwave and the VDF becomes thermal (cf. Figure 10), thus limiting the overall enhancement in energy efficiency. However, if the supersonic expansion region could be extended over a longer distance, the vibrational dissociation pathway could be further enhanced, thus improving the energy efficiency. As mentioned in section Different Plasma Types—Different Performance, the best energy efficiency ever reported for plasma-based CO2 conversion, 90%, was for a MW plasma with supersonic expansion back in 1983 (Asisov et al., 1983), but these results have never been reproduced since then, as they are probably critically dependent on the exact operating conditions. Therefore, more experiments should be performed to optimize the conditions and plasma reactor/Laval nozzle design in order to maximize the supersonic region, and to reach the highest possible CO2 conversion and energy efficiency.
Figure 10. Calculated evolution of the VDF in a MW plasma with Laval nozzle for supersonic expansion, for different axial positions, as indicated in the lower panel, showing the pressure evolution as a function of position in the supersonic expansion region and shock wave, for an SEI of 0.2 eV/molec, an inlet and outlet pressure of 2 and 1 bar, respectively. Adopted from Vermeiren and Bogaerts (2018) with permission.
The above suggestions to improve the energy efficiency by promoting the vibrational dissociation pathway are not straightforward to realize in practice. Indeed, recent insights based on both modeling and experiments have revealed that the CO2 conversion in (sub)atmospheric pressure MW and GA plasmas proceeds by thermal reactions (van Rooij et al., 2015; Berthelot and Bogaerts, 2017; Bongers et al., 2017; den Harder et al., 2017; Heijkers and Bogaerts, 2017; Kotov and Koelman, 2019; van den Bekerom et al., 2019; Wolf et al., 2019, 2020), and this can also give rise to quite high energy efficiencies (up to 40–50%). As explained above, the rate coefficients of the thermal reactions between various plasma species rise upon higher gas temperature, thus enhancing the conversion. Temperatures around 3,000–4,000 K should be optimal for thermal conversion to realize significant CO2 conversion and still limit thermal losses (Wolf et al., 2020). In this section, we provide some suggestions how to further improve the energy efficiency of thermal CO2 conversion beyond the thermal equilibrium limits of 45–50%.
A limitation for energy-efficient CO2 conversion at thermal conditions is the backward (recombination) reaction of CO with O atoms or O2 molecules forming CO2 again, as these recombination reactions also become faster at higher temperature. Fast quenching (cooling) of the gas after passing through the plasma reactor can limit these backward reactions, and should therefore be extensively investigated. Yang et al. (2018) demonstrated the effects of supersonic quenching for a thermal CO2 plasma. The supporting computational fluid dynamics calculations revealed a fast quenching rate of 107 K/s, causing the gas temperature to drop from above 3,000 K to 1,000 K. The quenching method was based on (i) adiabatic expansion of the gas in a converging nozzle, yielding acceleration to sonic speed, or conversion of thermal energy into convective kinetic energy, followed by (ii) a cooling tube in which strong eddies are formed, greatly enhancing the heat transfer between the inverse-flowing fluid and the cooling tube. This quenching prevents the reverse reactions, enhancing the CO2 conversion and energy efficiency (so-called ideal quenching).
In addition, a beneficial side-effect of the quenching would be if the heat removed from the outflowing gas mixture due to the quenching/cooling process can be recovered to preheat the incoming gas so that the plasma power will not be used for initial heating the gas, and can be directly used for the conversion. We believe this could also improve the overall efficiency.
Another possibility to avoid the backward (recombination) reactions, in order to enhance the conversion and energy efficiency, may be the fast removal of the O atoms from the mixture. This was demonstrated by modeling in a classical GA plasma (Sun et al., 2017), but also experimentally for a thermal arc plasma (Liu P. et al., 2019), using a carbon bed after the plasma reactor, reporting a CO2 conversion of 67–94% with corresponding energy efficiency of about 70%, much higher than ever observed in other (non-thermal or warm) plasmas. Likewise, Mori et al. integrated a solid oxide electrolysis cell (SOEC) into a plasma reactor to remove the O atoms, and thus avoid the recombination reaction. They demonstrated this concept for a DBD and showed significant enhancement of the CO2 conversion, even up to 100% at 700°C (Mori et al., 2016).
Vice versa, yet another interesting idea to enhance the conversion and energy efficiency above the thermal equilibrium limit exploits the O atoms, formed by CO2 splitting, to react with another CO2 molecule (CO2 + O → CO + O2) instead of recombining with CO. This is called “super-ideal quenching,” and makes full use of the dissociation products. As mentioned above, however, this reaction has a significant energy barrier, which was pinpointed as the major limiting factor for energy-efficient CO2 conversion, not only in thermal conversion, but also in case of vibration-induced dissociation; cf. above (Berthelot and Bogaerts, 2018). Thus, conditions should be explored to enhance this reaction, e.g., by vibrational excitation of CO2, because the latter could reduce the energy barrier of this reaction.
As discussed before, the theoretically highest energy efficiency, induced by the vibrational pathway at VT non-equilibrium conditions, may not (easily) be realized in (sub)atmospheric MW and GA plasmas. However, in case of thermal CO2 conversion, we can focus on the cooler regions outside the hot plasma zone in order to exploit the vibrationally excited CO2 molecules to overcome the high energy barrier of the recombination with O atoms (super-ideal quenching), thus enhancing the CO2 conversion above the thermal limit.
van den Bekerom et al. (2019) studied in detail the gas heating dynamics and their relation to the CO2 dissociation efficiency in a pulsed CO2 MW plasma at a pressure range of 30–160 mbar, and duty cycle of 20–100%. They reported rotational (or gas) temperatures up to 3,000 K in the core, with a cool edge temperature around 500 K. After the pulse, the gas cooled down convectively, but it was found to remain overall too hot for strong overpopulation of the vibrational modes (still 2,200 K in the core). Because the CO2 dissociation was observed to scale with the observed peak rotational temperature, the authors concluded that thermal processes dominate.
They explained the separation between hot core and cool edge by the tangential gas injection yielding a swirl flow, causing recirculation zones with closed flow lines where the gas can only enter or exit by diffusion. This transport barrier is thought to be responsible for the steep temperature gradient between hot plasma core and cool edge, with only the hot core being heated by the MW power. This core temperature is stated to rise rapidly until equilibrium is reached between input MW power and diffusion. Note that other papers by different researchers also reported similar behavior. Typically, three different operating regimes could be distinguished for (sub)atmospheric MW plasmas (i.e., diffuse and two different constricted modes) depending on pressure (e.g., den Harder et al., 2017) and the constricted regimes were studied in detail by Wolf et al. (2019, 2020), who also confirmed the dominance of thermal dissociation.
van den Bekerom et al. (2019) discussed in detail how to increase the energy efficiency beyond the thermal equilibrium limit, focusing on the role of the edge region around the hot plasma core. Indeed, the gas that diffuses out of the hot core arrives in the stream of cold gas in the edge region, where it is subject to fast quenching. This means that the backward (recombination) reactions of CO and O to form CO2 again are strongly diminished by the drop in temperature, and the high temperature CO2 conversion remains frozen (ideal quenching).
In addition, although at thermal conditions there is no overpopulation of the CO2 vibrational levels (i.e., the VDF follows a Boltzmann distribution), a significant amount of energy is still stored in the vibrational levels because of the high temperature in the core. When the hot CO2 molecules diffuse out of the core, a VT non-equilibrium can be established because VT relaxation is much slower in the cooler edge.
If the quenching is fast enough, the O atoms do not recombine into CO or O2, but survive and can react in the cold gas stream with the vibrationally excited CO2 molecules to produce additional CO. The recycling of O atoms through reaction with vibrationally excited CO2 molecules was also suggested by Silva et al. (2017) in a MW post-discharge. As mentioned above, this reaction has a high activation energy, but it may indeed be overcome by either hot O atoms or the vibrationally excited CO2 molecules (Berthelot and Bogaerts, 2018). This super-ideal quenching may explain the energy efficiencies above the thermal equilibrium limits reported in the 1980s (Legasov et al., 1978; Asisov et al., 1983).
van den Bekerom et al. (2019) concluded that the research focus should shift from the core—which is in thermal equilibrium at the high temperature—to the cooler edge region, to investigate how effective is the latter in quenching products from the hot core to further enhance the energy efficiency above the thermal equilibrium limit. Although this study was applied to reduced pressure MW plasmas, the same concept may be valid to GA plasmas, where the hot arc region (ca. 3,000 K; Trenchev et al., 2017) is surrounded by cooler gas. Thus, the above suggestions should also be explored in detail for GA plasmas.
Likewise, besides unreacted CO2 coming from the hot plasma, adding extra CO2 in the post-conversion cooling zone could also be beneficial because (i) the cold CO2 gas can enhance the cooling rate and suppress the back-reaction by dilution, and (ii) it can enhance CO formation upon reaction with O atoms.
In general, we may conclude that the conditions outside the hot plasma zone or the post-discharge recombination kinetics, rather than the plasma condition itself, are the key for optimization of energy-efficient CO2 conversion. Maybe these phenomena will be able to explain the record energy efficiencies observed in the 1980s (Legasov et al., 1978; Asisov et al., 1983).
The above suggestions to improve the energy efficiency will at the same time enhance the conversion as well. In addition, we believe the conversion can be further improved by smart plasma reactor design, i.e., by increasing the number of molecules passing through the active plasma region. Modeling has indeed identified this fraction as the limiting factor for the conversion in GA plasmas. This is the case both in classical GA reactors (Sun et al., 2017), but also in the GAP, because the arc plasma is not filling the entire plasma reactor but is only located in the center, thus not all of the gas will pass through the plasma (Ramakers et al., 2017; Trenchev et al., 2017). Furthermore, it can also be a limiting factor in MW plasmas at atmospheric pressure or in APGDs due to plasma contraction in the center (Trenchev et al., 2019; van den Bekerom et al., 2019; Wolf et al., 2019, 2020). Thus, even if the conversion is close to 100% inside the plasma, if only a certain fraction of the gas passes through the plasma, the latter determines and limits the overall conversion in the reactor, unless significant thermal conversion still occurs in the region around the plasma if the latter is still at high enough temperature, as demonstrated for DRM (Cleiren et al., 2017; Slaets et al., 2020), or the edge region contributes to further conversion by the reaction of O atoms with unreacted CO2, as discussed in the previous section (van den Bekerom et al., 2019).
Major efforts are needed to enhance the processed gas fraction by smart reactor design, including gas inlet/outlet design. An example is shown in Figure 11, for CO2 conversion in the GAP with different gas outlet diameters (Ramakers et al., 2017). As explained in section Different Plasma Types—Different Performance above, the gas enters the reactor tangentially, and when the reactor diameter is larger than the outlet diameter, the gas flows initially in an outer vortex toward the upper end of the reactor, after which it follows a reverse vortex with smaller diameter toward the outlet (see Figure 1D above). In the ideal scenario, this reverse vortex coincides with the arc in the middle of the reactor to maximize the conversion. Figure 11 indeed illustrates that the reactor with the smallest outlet diameter yields the best conversion and energy efficiency, much greater than when the outlet diameter is the same as the reactor diameter, as the latter does not allow a reverse vortex gas flow to take place. Nevertheless, even for the smallest outlet diameter, the gas fraction that passes through the arc is still quite limited, and this explains the rather low overall CO2 conversion in this reactor. In addition, the gas temperature is quite high (~3,000 K; Trenchev et al., 2017) due to a hot cathode spot, which also causes severe electrode degradation. This clearly stresses the need for further reactor design improvements.
Figure 11. Measured conversion and energy efficiency as a function of SEI, in a gliding arc plasmatron (GAP) at atmospheric pressure, for three different reactor outlet diameters, as indicated by the legend. Adopted from Ramakers et al. (2017) with permission.
Figure 12A presents an innovative electrode design to improve the conversion capability of the GAP reactor by elongating the arc in two directions to increase the residence time of the gas inside the arc and to actively cool the cathode spot by rotation of the arc and gas convection. This novel reactor, called dual-vortex (GA) plasmatron (DVP), yields strong rotational gas flow up to the electrode outlets, as demonstrated from fluid dynamics simulations (Trenchev and Bogaerts, 2020). This allows rotating the cathode (or anode) hot spots, reducing electrode damage. The CO2 conversion was found to be around 9%, with a corresponding energy efficiency up to 41%, for a gas flow rate of 10 L/min and a plasma power of 446 W, yielding a specific energy input of 2.7 kJ/L (Figure 12B). It should be noted that the conversion obtained in this study was still limited, attributed to the power supply, which did not yield a stable plasma at gas flow rates above 12.5 L/min. In addition, no stable plasma could be obtained for SEI values between 2.2 and 2.7 kJ/L (cf. Figure 12B). Model calculations predicted that a higher flow rate of 20 L/min would greatly reduce the gas temperature, due to strong turbulent heat transfer. While the conversion is thermal in this GA plasma, a lower temperature might shift it to the non-equilibrium regime, where the vibrational pathway of CO2 dissociation could be promoted (see discussion in section Improving the Energy Efficiency). However, it is also very likely that the temperature would still be high enough for thermal conversion to dominate. On the other hand, a lower temperature could further cool the cathode spot, and thus reduce electrode degradation, which would be beneficial. Future work will thus require building a novel power supply to produce higher power values and to allow stable plasma operation at higher flow rates in order to evaluate whether this can further enhance the performance of the DVP.
Figure 12. Schematic illustration of the dual-vortex plasmatron (DVP) design (A), and conversion and energy efficiency obtained in this setup, at atmospheric pressure, as a function of the SEI (B). Adopted from Trenchev and Bogaerts (2020) with permission.
Another example of plasma reactor design improvement to enhance the CO2 conversion based on fluid dynamics simulations is given in Figure 13 for an APGD reactor (Trenchev et al., 2019). The basic APGD reactor has a pin-to-plate design with simple gas inlet, as illustrated in Figure 13A. It exhibits an overall CO2 conversion of only 3–4.5% (see Figure 13D), which could be explained by modeling, as the plasma was confined to the center of the reactor. The conversion inside the plasma was calculated to be 75%, but the overall conversion was much lower due to the limited fraction of gas passing through the plasma. The energy efficiency is around 30% (see Figure 13D), and modeling indicated that the conversion is thermal, and the VDF is Boltzmann-distributed.
Figure 13. Schematic illustration of the basic (A), vortex (B), and confined (C) APGD designs, as well as (D) conversion and energy efficiency obtained in these setups, at atmospheric pressure, at three different currents. The plasma power and corresponding SEI are also illustrated in (D). Adopted from Trenchev et al. (2019) with permission.
Figure 13B illustrates calculated gas flow pathlines from fluid dynamics simulations in a modified setup by employing a vortex flow generator. This vortex flow reduces the cathode temperature due to turbulence, and thus enables operation at higher power with longer interelectrode distance (22 mm instead of 18 mm in the basic design) without melting. In addition, the turbulence allows somewhat more gas to pass through the plasma. Both effects lead to a longer residence time of the gas in the plasma and to a higher conversion of about 8% at the longer interelectrode distance of 22 mm (Figure 13D). The high gas temperature again yields a thermal VDF, with an energy efficiency around 30%.
To further enhance the gas fraction passing through the plasma, a “confined” configuration was designed, based on a ceramic tube with inner radius of 2.5 mm (i.e., equal to the plasma radius, predicted by the model) that fits precisely with the cathode pin (see Figure 13C). By means of a spiral groove in the cathode pin, the gas could be guided into the tube. In addition, the groove yielded cooling of the cathode pin, preventing it from melting, so that again a higher power could be used. This confined design makes sure that the plasma fills the entire reactor, and thus all the gas molecules pass through the plasma, resulting in a higher conversion of up to 12.5%. The downside is that the plasma is now in direct contact with the walls, resulting in heat losses and loss of plasma species toward the walls. Therefore, the energy efficiency is slightly lower than in the basic and vortex flow APGD, ca. 26%. However, the conversion enhancement is much more prominent, being three times higher than in the basic APGD and one and a half to two times higher than in the vortex flow design. Further improvements should be possible by keeping the concept of the confined configuration in combination with turbulence and reducing the heat losses toward the walls. Fluid dynamics simulations will be very useful to further guide the experimental reactor design improvements.
Finally, besides improving the conversion and energy efficiency, there is significant room for improvement in the field of plasma catalysis for the selective production of value-added compounds. Indeed, as mentioned in section Different Plasma Types—Different Performance, plasma is very reactive, but therefore it is not selective in the production of targeted compounds. If high value products, such as olefins and oxygenates, could be selectively formed in a one-step process by plasma catalysis, the high energy cost of current DBD reactors would be less of a limitation because in classical DRM, syngas also needs to be further converted into higher value products, by Fischer-Tropsch or methanol synthesis, which also adds to the overall energy cost of production. In the past, plasma catalysis experiments mainly used thermal catalysts, which are not necessarily most suitable for combination with plasma. Indeed, plasma is characterized by many reactive species, such as vibrationally and electronically excited molecules, radicals, ions, and electrons, which will affect the catalytic reactions. Therefore, special catalysts must be designed and tailored to the plasma environment to fully exploit the potential of plasma catalysis.
While in conventional heterogeneous catalysis, reactants chemisorb and follow some surface-mediated reaction path that ultimately determines the products formed and their formation rates; plasma causes external activation of the reactants, providing extra possibilities. Thus, other research questions should be answered, e.g., how can the catalyst interact with these highly reactive/energetic plasma species without simply quenching them, and how can it provide a selective path of transformation? A further complication is that the plasma may also transform the catalyst, both structurally and compositionally, and it may modify intrinsic surface reaction rates and/or pathways through for instance charging or electric field effects.
For plasma-catalytic NH3 synthesis, microkinetic modeling demonstrated the effect of plasma-induced (vibrational) excitation of N2 molecules, for enhanced NH3 production at conditions that are thermally inaccessible, and also predicted that the optimal catalytic material (the “peak” in the so-called volcano plot) was shifted to materials with lower N binding energy, consistent with experimental observations (Mehta et al., 2018). This indicates that plasma-activation of N2, e.g., by vibrational excitation, can overcome typical classical NH3 synthesis scaling relations. In other words, the plasma can overcome the usual constraints of catalysts to have a low activation energy for N2 dissociation and at the same time a weak interaction with surface intermediates, needed for easy desorption. Hence, this illustrates the great potential of plasma-catalytic NH3 synthesis. Similar effects were recently predicted by microkinetic modeling for non-oxidative methane coupling by plasma catalysis, showing the role of both vibrational excitation and plasma radicals in enhancing plasma-catalytic reactions (Engelmann et al., 2020), and we may expect similar behavior for plasma-catalytic CO2 conversion with a H-source (e.g., CH4, H2O, or H2) as well. Mehta et al. recently reviewed the impact of plasma-catalytic DRM, highlighting the potential of plasma catalysis to allow chemical conversion at lower temperatures where thermally no conversion is expected (Mehta et al., 2019).
Besides the beneficial effects of plasma activation for enhanced catalytic reactions, catalysts may in turn also help to overcome current limitations in plasma-based CO2 conversion. This can be illustrated for the combined CO2/H2O conversion, where 1–2% of H2O addition already result in much lower CO2 conversion, corresponding to a much lower energy efficiency, than for pure CO2 conversion. In addition, H2 and CO were detected as the main products, and virtually no oxygenates were formed (Snoeckx et al., 2017). Indeed, modeling revealed that too many steps would be needed in plasma-based conversion without catalysts, all these steps require H atoms, and the latter react predominantly with OH into H2O or with O2 into HO2, which again produces H2O, as demonstrated in Figure 14. This also explains the low amount of H2O conversion in plasma. In addition, the OH radicals exhibit a fast reaction with CO into CO2, as predicted in the model, and this explains why the CO2 conversion is significantly lower upon H2O addition. Thus, it is clear that catalysts are needed to enable that CO and H2 formed in the plasma react into oxygenates. More specifically, the binding of the reactants at the catalyst surface must proceed faster than the recombination of OH with H atoms into H2O, as well as of OH with CO into CO2.
Figure 14. Main reaction pathways for CO2 and H2O conversion in a DBD plasma. The arrow lines denote the formation rates of the species, with full green lines being formation rates over 1017 cm−3 · s−1, orange dashed lines between 1017 and 1016 cm−3 · s−1 and red dotted lines between 1016 and 1015 cm−3 · s−1. Adopted from Snoeckx et al. (2017) with permission.
By combining a CO2/H2 DBD with CuO/ZnO/Al2O3 catalyst, Eliasson et al. (1998) obtained a methanol yield and selectivity more than ten times higher than without catalyst. We may expect similar effects for a CO2/H2O DBD because it also forms a CO/CO2/H2 mixture. Furthermore, a CO2/H2O MW plasma with NiO/TiO2 catalysts resulted in a rise in CO2 conversion (up to 48%), with energy cost of 14.5 eV/molec (Chen et al., 2017) (cf. best data point in Figure 4 above). This was explained by the fact that CO2 molecules adsorb at oxygen vacancies in the catalyst surface, which lowers the threshold for dissociative electron attachment of CO2 into adsorbed O atoms at the vacancies and CO molecules. Subsequently, the O atoms can recombine with gas phase O atoms or OH radicals into O2 (and H atoms). Thus, the catalyst lets the O atoms or OH radicals from the plasma react into O2, with help of surface-adsorbed O atoms, before they would react into CO2 and H2O, which is stated to be the major problem in CO2/H2O plasmas without catalysts. Nevertheless, the catalyst in this work could only improve the CO2 conversion and energy cost, but did not allow the selective production of oxygenates.
Clearly, major research efforts are needed, including in situ and operando surface characterization and carefully designed experiments to isolate specific contributions from plasma and catalyst, as well as microkinetic modeling, to improve our insights in the mutual interactions between plasma and catalysts, for rational design of specific catalysts. Achieving control over the selectivity is clearly frontier research. It requires to go beyond the current approach in considering how to realize the synergy between plasma and catalysis. This is the objective of the ERC Synergy grant SCOPE (810182).
After having introduced the general aspects and current prospects and gaps in plasma technology for CO2 conversion, and having shortly compared plasma technology with the alternative technologies for CO2 conversion (introduction, further aspects in the other contributions to this special issue), the aim of this section is to put plasma technology in the general context of the changing scenario of energy and chemistry transition. This is a complementary section to the other sections that will only in part discuss the specific case of plasma, because it is aimed to give a window on the future to understand better the directions, aims, and challenges of plasma technology beyond the technical aspects.
In general, the chemical and the process industries are crucial components of most of the value chains that allow a considerable improvement in the quality of life realized in the last century by delivering the goods and services needed to our society. The materials produced were enabling factors in many relevant societal aspects, including housing, mobility, communication, health, and food. Last but not least, these industries are crucial to create prosperity. The process industry sector in Europe, for example, creates many jobs (currently 6.3 million directly and 19 million indirectly) and contributes to the GDP (€565 billion/year); it drives innovation, and develops solutions for societal problems (European Commission, 2018). On the other hand, there is a loss of competitiveness in some geographical areas, such as Europe, in favor of emerging areas, such as Asia, and at the same time an increasing social pressure to reduce the environmental impact of this sector. In a globalized world, some of the old economic instruments such as depreciation are no longer valid, and thus there is increasing awareness that different approaches have to be pursued.
The European Green Deal, launched in December 2019 as a large initiative aimed to mobilize over one trillion Euro resources in the next decade, aims to transform the European Union into a modern, resource-efficient, and competitive economy. Thus, the aim is to use the environmental challenge (no net GHG emissions by 2050) to create innovation and industrial competitiveness, and thus combine the three faces of sustainability (economy, social growth, and environmental preservation). This is possible by a revolutionary rather than evolutionary approach, having science and technology at the core and as drivers for development. With the new targets in GHG emissions indicated at political level, disruptive technologies are needed, rather than a continuation of ongoing initiatives. Plasma technology for CO2 utilization has many of the characteristics required, even if significant improvements are still necessary, as discussed in the previous sections, before it can pass from lab to industrial scale, or from idea to innovation. Although this is currently valid for Europe, it will likely be a drag effect on other parts of the world, with sustainability, rather than globalization, being put at the core of the strategic agenda.
The chemical and process industries always operated at the intersection of energy and resources, with the nexus between energy and chemical production being a relevant factor for the common development (Abate et al., 2015a). The ongoing energy transition (Gielen et al., 2019) has major implications on the structure of the chemical production and process industry. Together with the increasing evidence that renewable energy sources (RES) are now already the cheaper and more sustainable energy source (International Renewable Energy Agency - IRENA, 2019), and will become even more so in the near future, this allows us to predict a radical change in the actual production of chemicals and energy production, with RES becoming the backbone of our society in substitution of fossil fuels (Lanzafame et al., 2017a,b; Perathoner and Centi, 2019). This also has major implications in terms of technological perspectives (Centi and Čejka, 2019; Centi et al., 2019), because it requires developing new disruptive technologies that allow the direct use of RES for chemical production and chemical energy storage, and to close the carbon cycle. This is the reason for interest in plasma chemistry. Although it is an area known for a long time already, with many sub-sectors of application (from preparation of materials to surface treatments), the application in sectors such as chemical synthesis and energy vectors production has been limited. For a long time, it remained a niche sector, while in more recent years, an expansion of research beyond the more traditional applications can be observed. As a simple example, journals such as Green Chemistry, which were in the past reluctant to consider plasma chemistry as part of the portfolio of relevant technologies for green chemistry, are now open to plasma chemistry (Slaets et al., 2020). The changing scenario in chemistry asks for new technologies, and plasma technology for CO2 conversion is one of the areas with great potential to contribute to this challenge.
Value chains will thus evolve to meet new societal demands such as climate change mitigation and prosperity, and to achieve these ambitious targets, an unprecedented wave of transformative innovation is needed. It was commented above how the “Green Deal” program in the EU is expected to enable innovation and competitiveness at the same time. Substituting current technologies based on fossil fuels with those based on RES and alternative carbon raw materials, including waste CO2 (preferably from biogenic resources, such as fermentation and biogas processes), is a key element in this direction. This is the reason for interest in plasma technology. Even if still many challenges exist, in terms of combining an improved energy efficiency and conversion with high selectivity, and the use of more realistic operating conditions (high pressure, the presence of complex mixtures and water vapor, among other aspects), plasma technology offers also many elements of interest over alternative solutions, as commented on in the introduction.
Plasma technology, together with other technologies allowing the direct use of RES in chemical transformations (primarily electro- and photo-catalysis; Perathoner and Centi, 2019), is thus part of this technological wave of change, and in general part of the crucial technologies to convert waste CO2 into fuels and chemicals as a more efficient and impactful way (up to an order of magnitude more effective) to store CO2 in a more or less permanent way. Therefore, plasma technology is one of the technologies under development to contribute to substitution of the use of fossil fuels (Schlögl et al., 2018).
Next to biomass and waste, CO2 is an alternative valuable source of carbon available in Europe and worldwide. It may be argued that the use of CO2 would extend the use of fossil fuels, but this is true only in the outdated version of carbon capture and storage (CCS) where captured CO2 is used for underground storage, for example in enhanced oil recovery (EOR) methods to stimulate further the extraction of oil in exhausted fields. In this case, CCS is even negative to meet societal demand of a more sustainable and low-carbon future. However, CO2 utilization from biogenic sources together with RES has instead not only a larger impact (up to one order to magnitude) in terms of effective reduction of CO2 equivalent emissions, but it is also a relevant way to boost the use of biomass by increasing economics and reducing the carbon footprint (Abate et al., 2015b). Thus, technologies for biogenic CO2 conversion play a relevant role in the development of the new concept of e-refinery, and the new model of distributed (small-scale) production of energy and chemical products for a novel sustainable model of development.
Biogenic CO2 resources also are present in emissions from municipal or agricultural/forestry waste combustion. To valorize these CO2 resources is not only necessary to close the carbon-cycle and push a circular economy, but it can become necessary if the carbon taxes on CO2 emissions will increase in the future, a realistic possibility considering political commitments to GHG reduction at least in some areas, such as Europe. In all of these cases, it is necessary to have technologies that are efficient at relatively small-scale with low cost of investment. Thus, new solutions with these characteristics that can take advantage of coupling with local RES availability are searched. This is another general motivation for plasma technology for CO2 conversion.
In case of fermentation and biogas production, highly concentrated CO2 streams are produced, which need only minor purification, and thus these streams can be directly transformed. A different case is, for example, the conversion of CO2 present in flue gas of biomass waste combustion (e.g., from forest or agriculture production). In this case, recovery of CO2 from the fuel gas is needed, but this is still a costly process (also from an energetic perspective). While improved technologies to recover CO2 from flue gas are under development (Song et al., 2018), CO2 capture will still remain an energy- and cost-intensive process. Thus, the possibility to directly use diluted concentrations of CO2 (in the 10–30% range) would be of interest. In plasma-based CO2 conversion, the concentration of CO2 may not be a critical issue, but the presence of other components, like H2O, which negatively affects the performance as commented above, is a critical issue. On the other hand, other admixtures, such as N2, are not detrimental for CO2 conversion, and can even enhance the conversion by chemical reactions with N2 electronically or vibrationally excited levels (Snoeckx et al., 2016; Ramakers et al., 2019). Nevertheless, in general, from an application point of view, the development of plasma-based CO2 conversion able to operate directly on fuel gas could be an important objective, although challenging.
This is just one example, but others can be indicated (in terms of reactor characteristics, issues related to downstream processing, safety, reliability of operations, possibility of management of fluctuations in space-velocity, temperature, and compositions, etc.) that have been considered only to a limited extent up to now, with research focused mainly on scientific rather than at technological aspects, with a view on the constraints and requirements from the application side. Putting plasma technology in this general context, as made here, also aims to focus attention on these aspects.
A general observation from this general perspective, which emerges from literature, as evidenced in the previous sections, is that there is a very large variability of the results obtained, which greatly depend on reactor typology, experimental conditions, fluid dynamics, etc. The variability in results is largely beyond that present in many other fields, for example, thermal catalysis. There are scientific motivations, as partly discussed in previous sections, but in general this variability indicates the complexity of the various factors, which can only partly be controlled. This has also major consequences for the scaling up of the results and the industrial exploitability. Today, for example, in terms of modeling a thermal catalytic fixed-bed reactor, a very simple model based on a plug-flow reactor gives a precision in terms of scalability higher than 15–20%, which is acceptable in most cases. When better precision is necessary, there are well-established reactor models which consider the presence of the catalyst, mass/heat transfer limitations, etc. Virtually, it is possible to scale-up a fixed-bed catalytic reactor from lab-scale to industrial-scale when enough precise data on the kinetics are available. Only for more complicated situations, such as for fluidized or circulating catalytic beds, or in the presence of multiphase operations, would modeling require intermediate steps of development. This is not valid for plasma technology, where upscaling is still largely empirical, like it was in the field of heterogeneous catalysis up to two to three decades ago for industrial reactor development.
Scale-up of plasma-based CO2 conversion is thus a major issue to realize its potential social impact. The most straightforward way is by placing a large number of plasma reactors in parallel, as demonstrated already for ozone synthesis in DBD plasma reactors (Kogelschatz, 2003). However, before numbering up, current lab-scale plasma reactors may also be scaled up in size to some extent, to allow higher flow rates and power. Nevertheless, if the plasma is still confined in the center (see discussion in section Improving the Conversion), a larger reactor might lead to a larger number of molecules that may not pass through the active plasma region, therefore still limiting the conversion. As discussed above, the reactor configuration has an important effect on the performance and energy efficiency already on a lab-scale. Upscaling may thus be very difficult due to the complexity of having reliable and scalable models of plasma reactors. Although advanced modeling of the process is significantly contributing to a better understanding and control of the phenomena, the industrial realization of plasma technology for CO2 remains a great challenge, requiring large effort.
Transferability of the results is another challenge, which requires attention to push plasma technology for CO2 conversion (and in general for chemical synthesis) out of the specific field of interest and become one of the backbone technologies for energy/chemistry transition. Results on the comparison between CO2 splitting and DRM reactions (Figures 2, 3) are instructive and reveal again the great dependence on feed composition, besides other aspects (type of reaction, other experimental conditions, typology of experiments). While in heterogeneous catalysis some general criteria are available and allow to restrict at least the field of investigation, the complexity of plasma, and even more if combined with a catalyst, makes it not easy to predict what could be expected. Some of the background chemical and physical motivations have been discussed in the previous sections, and significant improvements have been made in the last decade. However, it still remains a challenge to predict the results for new types of applications, among which nitrogen fixation has to be mentioned for its large impact in terms of developing novel solutions for sustainable ammonia and fertilizers production (Gorbanev et al., 2020). This is an example of an area in which the developments in plasma technology are currently ahead of those obtained by, for example, electrocatalysis (Chen et al., 2020).
In conclusion, plasma-based CO2 conversion may have a large and positive impact on the societal objective of a sustainable and low carbon future, but its industrial implementation still requires it to solve various aspects, for which only a knowledge-based approach can be effective to solve the complex phenomena discussed in the previous sections.
The purpose of this perspective paper is to explain why plasma may be promising for CO2 conversion into value-added compounds, as well as to present the current gaps and perspectives to solve them. We briefly presented the most common plasma types and their performance in terms of conversion and energy efficiency, and we explained the underlying reasons for their different performance. Subsequently, we discussed the major limitations and steps to be taken for further improvement. Finally, we gave a brief general presentation of the general context and relevance of this technology to achieve societal goals, particularly for sustainability and climate change mitigation. The objective was to indicate, starting from these windows into the future, some of the existing challenges that need attention from an application perspective, and explain why plasma technology is relevant for our future.
In general, plasma technology is quite promising for CO2 conversion because of (i) its high process versatility, allowing different kinds of reactions to be carried out (e.g., pure CO2 splitting, as well as CO2 conversion in the presence of a H-source, such as CH4, H2 or H2O); (ii) its low investment and operating costs; (iii) the fact that it does not require the use of rare earth metals; (iv) that it can be applied in a very modular setting, as plasma reactors scale up linearly with the plant output, allowing on-demand production; and (v) that it can be very easily combined with (various kinds of) renewable electricity. Moreover, as plasma can easily be switched on/off, it is highly suitable for the conversion of intermittent renewable energy into base chemicals or fuels, for peak shaving and grid stabilization.
However, more research is needed to further improve the capabilities of plasma-based CO2 conversion in terms of (i) energy efficiency, (ii) conversion, and (iii) product selectivity. The energy efficiency largely depends on the type of plasma reactor and operating conditions. The highest theoretical energy efficiency can be reached when the reduced electric field is around 50 Td or below, combined with a high plasma power to maximize vibrational excitation (as this provides theoretically the most energy-efficient dissociation pathway) with a low gas temperature (to minimize vibrational losses upon collision with other gas molecules, i.e., VT relaxation), or in other words, a strong VT non-equilibrium. The latter can also be achieved at low gas pressure, but that is less compatible with industrial operations. On the other hand, both experiments and modeling in recent years have revealed that in GA and MW plasmas at (sub)atmospheric pressure, the CO2 conversion proceeds mainly by thermal reactions, and the vibrational distribution is in equilibrium with the gas temperature (VT equilibrium) due to the high gas temperature (around 3,000 K or more). Nevertheless, these conditions still give rise to high energy efficiency if the backward (recombination) reactions of CO with O or O2 can be avoided by rapid quenching of the gas after the plasma reactor. In addition, if the O atoms can react with another CO2 molecule, instead of recombining with CO or O, the conversion and thus energy efficiency can be further enhanced beyond the thermal equilibrium limit (super-ideal quenching).
Furthermore, the conversion should be enhanced by increasing the fraction of gas treated by the plasma, i.e., by smart reactor design (including gas inlet/outlet), based on fluid dynamics simulations. Finally, there is still much room for improvement in terms of product selectivity because plasma is very reactive, but not selective for the production of targeted compounds, which may require post-reaction separation steps, adding to the overall energy cost of the process. Therefore, when targeting specific compounds, plasma needs to be combined with catalysts. However, more insight in the plasma-catalyst interactions is crucial for designing catalysts most suited to the plasma environment.
From technological and application point of view, important issues may be the scalability and the use of complex gas mixtures, rather than pure feed of CO2 or CO2/CH4. For example, the role of H2O in reducing the efficiency in CO2 transformation has been highlighted. But this also opens a window of opportunity because, for example, light-induced transformation of H2O over semiconductors may well be integrated with current plasma-based CO2 conversion technology. This is an area starting to be investigated, but it is still largely unexplored, in terms of realizing an effective synergy of operation. In general terms, the combination of catalysis with CO2 plasma has to pass from a mainly phenomenological approach to a knowledge-based and innovative approach, based on exploiting all possible synergies. This is the objective of the ERC Synergy grant SCOPE (810182). While the science of catalysis can be considered well-established (Čejka et al., 2018) and there are good bases for applications to new fields as well, such as the use of renewable resources (Centi and Perathoner, 2009; Čejka et al., 2018; Centi and Čejka, 2019), plasma technology is still not at the same level, and still has to be promoted to be considered as an integral and relevant part of the roadmaps on the future challenges in this area (Lanzafame et al., 2017b).
In conclusion, plasma technology for CO2 conversion has a large potential impact, but the complex phenomena present indicate the need of more disruptive approaches, based on integrated and multidisciplinary collaborations, to explore new ways of combining the potential of plasma with other methodologies, such as the mentioned combination with light-induced transformations on solid catalysts, using a knowledge-based approach. Modeling integrated with advanced design of plasma reactors and of nanostructured catalysts, as highlighted in the previous sections, are the keys to realize these challenges.
Potential of plasma technology to reduce CO2 emission, What is needed to overcome current limitations of plasma-based CO2 conversion?, and Conclusions are written by AB, while section Benefits for our life and impact on climate change is written by GC, and both wrote section Introduction. Both authors approved the other sections.
We acknowledge financial support from the European Research Council (ERC) under the European Union's Horizon 2020 research and innovation program (grant agreement no. 810182 – SCOPE ERC Synergy project).
The authors declare that the research was conducted in the absence of any commercial or financial relationships that could be construed as a potential conflict of interest.
We thank A. Berthelot, M. Ramakers, R. Snoeckx, G. Trenchev, and V. Vermeiren for providing the figures used in this article.
APGD, atmospheric pressure glow discharge; CCS, carbon capture and storage; DBD, dielectric barrier discharge; DRM, dry reforming of methane; DVP, dual-vortex plasmatron; EOR, enhanced oil recovery; ERC, European Research Council; GA, gliding arc; GAP, GA plasmatron; GDP, gross domestic product; molec, molecule; MW, microwave; PEC, photoelectro-chemical or -catalytic; RES, renewable energy sources; RF, radio-frequency; SEI, specific energy input; VDF, vibrational distribution function; VT, vibrational-translational; VV, vibrational-vibrational.
Abate, S., Centi, G., Lanzafame, P., and Perathoner, S. (2015a). The energy-chemistry nexus: a vision of the future from sustainability perspective. J. Energy Chem. 24, 535–547. doi: 10.1016/j.jechem.2015.08.005
Abate, S., Lanzafame, P., Perathoner, S., and Centi, G. (2015b). New sustainable model of biorefineries: biofactories and challenges of integrating bio-and solar refineries. ChemSusChem 8, 2854–2866. doi: 10.1002/cssc.201500277
Ampelli, C., Perathoner, S., and Centi, G. (2015). CO2 utilization: an enabling element to move to a resource- and energy-efficient chemical and fuel production. Philos. Trans. R Soc. A Math. Phys. Eng. Sci. 373:20140177. doi: 10.1098/rsta.2014.0177
Asisov, R. I., Vakar, A. K., Jivotov, V. K., Krotov, M. F., Zinoviev, O. A., Potapkin, B. V., et al. (1983). Non-equilibrium plasma-chemical process of CO2 decomposition in a supersonic microwave discharge. Proc. USSR Acad. Sci. 271, 94–97.
Belov, I., Paulussen, S., and Bogaerts, A. (2017). Pressure as an additional control handle for non-thermal atmospheric plasma processes. Plasma Proc. Polym. 14:e1700046. doi: 10.1002/ppap.201700046
Berthelot, A., and Bogaerts, A. (2016). Modeling of plasma-based CO2 conversion: lumping of the vibrational levels. Plasma Sources Sci. Technol. 25:045022. doi: 10.1088/0963-0252/25/4/045022
Berthelot, A., and Bogaerts, A. (2017). Modeling of CO2 splitting in a microwave plasma: how to improve the conversion and energy efficiency? J. Phys. Chem. C 121, 8236–8251. doi: 10.1021/acs.jpcc.6b12840
Berthelot, A., and Bogaerts, A. (2018). Pinpointing energy losses in plasma-based CO2 conversion. J. CO2 Util. 24, 479–499. doi: 10.1016/j.jcou.2018.02.011
Bogaerts, A., Kozák, T., Van Laer, K., and Snoeckx, R. (2015). Plasma-based conversion of CO2: current status and future challenges. Faraday Discuss. 183, 217–232. doi: 10.1039/C5FD00053J
Bogaerts, A., and Neyts, E. (2018). Plasma technology: an emerging technology for energy storage. ACS Energy Lett. 3, 1013–1027. doi: 10.1021/acsenergylett.8b00184
Bogaerts, A., Neyts, E., Gijbels, R., and van der Mullen, J. J. A. M. (2002). Gas discharge plasmas and their applications. Spectrochim. Acta B 57, 609–658. doi: 10.1016/S0584-8547(01)00406-2
Bongers, W., Bouwmeester, H., Wolf, B., Peeters, F., Welzel, S., van den Bekerom, D., et al. (2017). Plasma-driven dissociation of CO2 for fuel synthesis. Plasma Processes Polym. 14:1600126. doi: 10.1002/ppap.201600126
Capitelli, M., Colonna, G., D'Ammando, G., and Pietanza, L. D. (2017). Self-consistent time dependent vibrational and free electron kinetics for CO2 dissociation and ionization in cold plasmas. Plasma Sources Sci. Technol. 26:055009. doi: 10.1088/1361-6595/aa6427
Čejka, J., Nachtigall, P., and Centi, G. (2018). New catalytic materials for energy and chemistry in transition. Chem. Soc. Rev. 47, 8066–8071. doi: 10.1039/C8CS90119H
Centi, G., and Čejka, J. (2019). Needs and gaps for catalysis in addressing transitions in chemistry and energy from a sustainability perspective. ChemSusChem 12, 621–632. doi: 10.1002/cssc.201802637
Centi, G., Iaquaniello, G., and Perathoner, S. (2019). Chemical engineering role in the use of renewable energy and alternative carbon sources in chemical production. BMC Chem. Eng. 1:5. doi: 10.1186/s42480-019-0006-8
Centi, G., and Perathoner, S. (2009). Catalysis: role and challenges for a sustainable energy. Top. Catal. 52, 948–961. doi: 10.1007/s11244-009-9245-x
Centi, G., and Perathoner, S. (2010). Towards solar fuels from water and CO2. ChemSusChem 3, 195–208. doi: 10.1002/cssc.200900289
Centi, G., and Perathoner, S. (2020). Chemistry and energy beyond fossil fuels. A perspective view on the role of syngas from waste sources. Catal. Today. 342, 4–12. doi: 10.1016/j.cattod.2019.04.003
Chen, G., Britun, N., Godfroid, T., Georgieva, V., Snyders, R., and Delplancke-Ogletree, M.-P. (2017). An overview of CO2 conversion in a microwave discharge: the role of plasma-catalysis. J. Phys. D Appl. Phys. 50:084001. doi: 10.1088/1361-6463/aa5616
Chen, G., Wang, L., Godfroid, T., and Snyders, R. (2018). “Progress in plasma-assisted catalysis for carbon dioxide reduction,” in Plasma Chemistry and Gas Conversion, ed N. Britun (London: IntechOpen). doi: 10.5772/intechopen.80798
Chen, S., Perathoner, S., Ampelli, C., Wei, H., Abate, S., Zhang, B., et al. (2020). Enhanced performance in the direct electrocatalytic synthesis of ammonia from N2 and H2O by an in-situ electrochemical activation of CNT-supported iron oxide nanoparticles. J. Energy Chem. 49, 22–32. doi: 10.1016/j.jechem.2020.01.011
Cleiren, E., Heijkers, S., Ramakers, M., and Bogaerts, A. (2017). Dry reforming of methane in a gliding arc plasmatron: towards a better understanding of the plasma chemistry. ChemSusChem 10, 4025–4036. doi: 10.1002/cssc.201701274
De Bie, C., van Dijk, J., and Bogaerts, A. (2016). CO2 hydrogenation in a dielectric barrier discharge plasma revealed. J. Phys. Chem. C 120, 25210–25224. doi: 10.1021/acs.jpcc.6b07639
Debek, R., Azzolina-Jury, F., Travert, A., and Mauge, F. (2019). A review on plasma-?catalytic methanation of carbon dioxide - looking for an efficient catalyst. Renew. Sust. Energy Rev. 116:109427. doi: 10.1016/j.rser.2019.109427
den Harder, N., van den Bekerom, D. C. M., Al, R. S., Graswinckel, M. F., Palomares, J. M., Peeters, F. J. J., et al. (2017). Homogeneous CO2 conversion by microwave plasma: wave propagation and diagnostics. Plasma Processes Polym. 14:1600120. doi: 10.1002/ppap.201600120
Eliasson, B., Kogelschatz, U., Xue, B., and Zhou, L.-M. (1998). Hydrogenation of carbon dioxide to methanol with a discharge-activated catalyst. Ind. Eng. Chem. Res. 37, 3350–3357. doi: 10.1021/ie9709401
Engelmann, Y., Mehta, P., Neyts, E. C., Schneider, W. F., and Bogaerts, A. (2020). Predicted influence of plasma activation on non-oxidative coupling of methane on transition metal catalysts. ACS Sustain. Chem. Eng. 8, 6043–6054. doi: 10.1021/acssuschemeng.0c00906
European Commission (2018). 2050 Long-Term Strategy “A Clean Planet for All. A European Strategic Long-Term Vision for a Prosperous, Modern, Competitive and Climate Neutral Economy.
García de Arquer, F. P., Dinh, C.-T., Ozden, A., Wicks, J., Mccallum, C., Kirmani, A. R., et al. (2020). CO2 electrolysis to multicarbon products at activities greater than 1 A Cm−2. Science 367, 661–666. doi: 10.1126/science.aay4217
Gielen, D., Boshell, F., Saygin, D., Bazilian, M. D., Wagner, N., and Gorini, R. (2019). The role of renewable energy in the global energy transformation. Energy Strat. Rev. 24, 38–50. doi: 10.1016/j.esr.2019.01.006
Gorbanev, Y., Vervloessem, E., Nikiforov, A., and Bogaerts, A. (2020). Nitrogen fixation with water vapor by nonequilibrium plasma: toward sustainable ammonia production. ACS Sust. Chem. Eng. 8, 2996–3004. doi: 10.1021/acssuschemeng.9b07849
Groen, P. W. C., Wolf, A. W., Righart, T. W. H., van de Sanden, M. C. M., Peeters, F. J. J., and Bongers, W. A. (2019). Numerical model for the determination of the reduced electric field in a CO2 microwave plasma derived by the principle of impedance matching. Plasma Sources Sci. Technol. 28:075016. doi: 10.1088/1361-6595/ab1ca1
Grofulović, M., Silva, T., Klarenaar, B. L. M., Morillo-Candas, A. S., Guaitella, O., Engeln, R., et al. (2018). Kinetic study of CO2 plasmas under nonequilibrium conditions. II. Input of vibrational energy. Plasma Sources Sci. Technol. 27:115009. doi: 10.1088/1361-6595/aadb60
Heijkers, S., and Bogaerts, A. (2017). CO2 conversion in a gliding arc plasmatron: elucidating the chemistry through kinetic modelling. J. Phys. Chem. C 121, 22644–22655. doi: 10.1021/acs.jpcc.7b06524
International Renewable Energy Agency - IRENA (2019). Renewable Energy Statistics 2019. Abu Dhabi: The IRENA. ISBN: 978-92-9260-137-9
Klarenaar, B. L. M., Engeln, R., van den Bekerom, D. C. M., van de Sanden, M. C. M., Morillo-Candas, A. S., and Guaitella, O. (2017). Time evolution of vibrational temperatures in a CO2 glow discharge measured with infrared absorption spectroscopy. Plasma Sources Sci. Technol. 26:115008. doi: 10.1088/1361-6595/aa902e
Klarenaar, B. L. M., Grofulovi,ć, M., Morillo-Candas, A. S., van den Bekerom, D. C. M., Damen, M. A., van de Sanden, M. C. M., et al. (2018). A rotational raman study under non-thermal conditions in a pulsed CO2 glow discharge. Plasma Sources Sci. Technol. 27:045009. doi: 10.1088/1361-6595/aabab6
Klarenaar, B. L. M., Morillo-Candas, A. S., Grofulovi,ć, M., van de Sanden, M. C. M., Engeln, R., and Guaitella, O. (2019). Excitation and relaxation of the asymmetric stretch mode of CO2 in a pulsed glow discharge. Plasma Sources Sci. Technol. 28:035011. doi: 10.1088/1361-6595/aada5e
Kogelschatz, U. (2003). Dielectric-barrier discharges: their history, discharge physics, and industrial applications. Plasma Chem. Plasma Process. 23, 1–46. doi: 10.1023/A:1022470901385
Kotov, V., and Koelman, P. M. J. (2019). Plug flow reactor model of the plasma chemical conversion of CO2. Plasma Sources Sci. Technol. 28:095002. doi: 10.1088/1361-6595/ab3774
Kozák, T., and Bogaerts, A. (2014). Splitting of CO2 by vibrational excitation in non-equilibrium plasmas: a reaction kinetics model. Plasma Sources Sci. Technol. 23:045004. doi: 10.1088/0963-0252/23/4/045004
Kozák, T., and Bogaerts, A. (2015). Evaluation of the energy efficiency of CO2 conversion in microwave discharges using a reaction kinetics model. Plasma Sources Sci. Technol. 24, 015024. doi: 10.1088/0963-0252/24/1/015024
Lanzafame, P., Abate, S., Ampelli, C., Genovese, C. Passalacqua, R., Centi, G., et al. (2017a). Beyond solar fuels: renewable energy-driven chemistry. ChemSusChem 10, 4409–4419. doi: 10.1002/cssc.201701507
Lanzafame, P., Perathoner, S., Centi, G., Gross, S., and Hensen, E. J. M. (2017b). Grand challenges for catalysis in the science and technology roadmap on catalysis for Europe: moving ahead for a sustainable future. Catal. Sci. Technol. 7, 5182–5194. doi: 10.1039/C7CY01067B
Legasov, V. A., Zhivotov, V. K., Krasheninnikov, E. G., Krotov, M. F., Patrushev, L., Rusanov, V. D., et al. (1978). A nonequilibrium plasma-chemical process of CO2 dissociation in high-frequency and ultra-high-frequency discharges. Soviet Phys. Doklady. 23, 44–46.
Li, K., Liu, J. L., Li, X. S., Zhu, X., and Zhu, A. M. (2016). Warm plasma catalytic reforming of biogas in a heat-insulated reactor: dramatic energy efficiency and catalyst auto-reduction. Chem. Eng. J. 288, 671–679. doi: 10.1016/j.cej.2015.12.036
Liu, M., Yi, Y., Wang, L., Guo, H., and Bogaerts, A. (2019). Hydrogenation of carbon dioxide to value-added chemicals by heterogeneous catalysis and plasma catalysis. Catalysts 9:275. doi: 10.3390/catal9030275
Liu, P., Liu, X., Shen, J., Yin, Y., Yang, T., Huang, Q., et al. (2019). CO2 conversion by thermal plasma with carbon as reducing agent: high CO yield and energy efficiency. Plasma Sci. Technol. 21:012001. doi: 10.1088/2058-6272/aadf30
Mehta, P., Barboun, P., Go, D. B., Hicks, J. C., and Schneider, W. F. (2019). Catalysis enabled by plasma activation of strong chemical bonds: a review. ACS Energy Lett. 4, 1115–1133. doi: 10.1021/acsenergylett.9b00263
Mehta, P., Barboun, P., Herrera, F. A., Kim, J., Rumbach, P., Go, D. B., et al. (2018). Overcoming ammonia synthesis scaling relations with plasma-enabled catalysis. Nat. Catal. 1, 269–275. doi: 10.1038/s41929-018-0045-1
Mei, D., Zhu, X., He, Y., Yan, J. D., and Tu, X. (2015). Plasma-assisted conversion of CO2 in a dielectric barrier discharge reactor: understanding the effect of packing materials. Plasma Sources Sci. Technol. 24:015011. doi: 10.1088/0963-0252/24/1/015011
Michielsen, I., Uytdenhouwen, Y., Pype, J., Michielsen, B., Mertens, J., Reniers, F., et al. (2017). CO2 dissociation in a packed bed DBD reactor: first steps towards a better understanding of plasma catalysis. Chem. Eng. J. 326, 477–488. doi: 10.1016/j.cej.2017.05.177
Mori, S., Matsuura, N., Tun, L. L., and Suzuki, M. (2016). Direct synthesis of carbon nanotubes from only CO2 by a hybrid reactor of dielectric barrier discharge and solid oxide electrolyser cell. Plasma Chem. Plasma Process. 36, 231–239. doi: 10.1007/s11090-015-9681-2
Navarrete, A., Centi, G., Bogaerts, A., Martín, Á., York, A., and Stefanidis, G. (2017). Harvesting renewable energy for CO2 catalysis. Energy Technol. 5, 796–811. doi: 10.1002/ente.201600609
Neyts, E. C., Ostrikov, K., Sunkara, M. K., and Bogaerts, A. (2015). Plasma catalysis: synergistic effects at the nanoscale. Chem. Rev. 115, 13408–13446. doi: 10.1021/acs.chemrev.5b00362
Nunnally, T., Gutsol, K., Rabinovich, A., Fridman, A., Gutsol, A., and Kemoun, A. (2011). Dissociation of CO2 in a low current gliding arc plasmatron. J. Phys. D Appl. Phys. 44:274009. doi: 10.1088/0022-3727/44/27/274009
Ozkan, A., Dufour, T., Silva, T., Snyders, R., Bogaerts, A., and Reniers, F. (2016). The influence of power and frequency on the filamentary behavior of a flowing DBD – application to the splitting of CO2. Plasma Sources Sci. Technol. 25:025013. doi: 10.1088/0963-0252/25/2/025013
Peeters, F. J. J., Yang, R., and van de Sanden, M. C. M. (2015). The relation between the production efficiency of nitrogen atoms and the electrical characteristics of a dielectric barrier discharge. Plasma Sources Sci. Technol. 24:045006. doi: 10.1088/0963-0252/24/4/045006
Perathoner, S., and Centi, G. (2014). CO2 Recycling: a key strategy to introduce green energy in the chemical production chain. ChemSusChem 7, 1274–1282. doi: 10.1002/cssc.201300926
Perathoner, S., and Centi, G. (2019). Catalysis for solar-driven chemistry: the role of electrocatalysis. Catal. Today 330, 157–170. doi: 10.1016/j.cattod.2018.03.005
Pietanza, L. D., Colonna, G., and Capitelli, M. (2017b). Nonequilibrium plasma kinetics of reacting CO: an improved state to state approach. Plasma Sources Sci. Technol. 26:125007. doi: 10.1088/1361-6595/aa93bd
Pietanza, L. D., Colonna, G., and Capitelli, M. (2018a). Nonequilibrium electron and vibrational distributions under nanosecond repetitively pulsed CO discharges and afterglows: I. Optically thick plasmas. Plasma Sources Sci. Technol. 27:095004. doi: 10.1088/1361-6595/aad7ef
Pietanza, L. D., Colonna, G., and Capitelli, M. (2018b). Nonequilibrium electron and vibrational distributions under nanosecond repetitively pulsed CO discharges and afterglows: II. The role of radiative and quenching processes. Plasma Sources Sci. Technol. 27:095003. doi: 10.1088/1361-6595/aad7f2
Pietanza, L. D., Colonna, G., and Capitelli, M. (2020). Kinetics versus thermodynamics on CO2 dissociation in high temperature microwave discharges. Plasma Sources Sci. Technol. 29:035022. doi: 10.1088/1361-6595/ab6e5a
Pietanza, L. D., Colonna, G., D'Ammando, G., and Capitelli, M. (2017a). Time-dependent coupling of electron energy distribution function, vibrational kinetics of the asymmetric mode of CO2 and dissociation, ionization and electronic excitation kinetics under discharge and post-discharge conditions. Plasma Phys. Control. Fusion 59:014035. doi: 10.1088/0741-3335/59/1/014035
Pietanza, L. D., Colonna, G., D'Ammando, G., Laricchiuta, A., and Capitelli, M. (2015). Vibrational excitation and dissociation mechanisms of CO2 under non-equilibrium discharge and post-discharge conditions. Plasma Sources Sci. Technol. 24:042002. doi: 10.1088/0963-0252/24/4/042002
Puliyalil, H., Lasic Jurkovic, D., Dasireddy, V. D. B. C., and Likozar, B. (2018). A review of plasma-assisted catalytic conversion of gaseous carbon dioxide and methane into value-added platform chemicals and fuels. RSC Adv. 8, 27481–27508. doi: 10.1039/C8RA03146K
Qin, Y., Niu, G., Wang, X., Luo, D., and Duan, Y. (2018). Status of CO2 conversion using microwave plasma. J. CO2 Util. 28, 283–291. doi: 10.1016/j.jcou.2018.10.003
Ramakers, M., Heijkers, S., Tytgat, T., Lenaerts, S., and Bogaerts, A. (2019). Combining CO2 conversion and N2 fixation in a gliding arc plasmatron. J. CO2 Util. 33, 121–130. doi: 10.1016/j.jcou.2019.05.015
Ramakers, M., Trenchev, G., Heijkers, S., Wang, W., and Bogaerts, A. (2017). Gliding arc plasmatron: providing a novel method for CO2 conversion. ChemSusChem 10, 2642–2652. doi: 10.1002/cssc.201700589
Schlögl, R., Abanades, C., Aresta, M., Azapagic, A., Blekkan, E. A., Cantat, T., et al. (2018). Novel Carbon Capture and Utilisation Technologies: Research And Climate Aspects, SAPEA, Evidence Review Report No. 2. Berlin: SAPEA. ISBN 978-3-9819415-5-5.
Silva, T., Britun, N., Godfroid, T., and Snyders, R. (2014). Optical characterization of a microwave pulsed discharge used for dissociation of CO2. Plasma Sources Sci. Technol. 23:025009. doi: 10.1088/0963-0252/23/2/025009
Silva, T., Britun, N., Godfroid, T.h., and Snyders, R. (2017). Understanding CO2 decomposition in microwave plasma by means of optical diagnostics. Plasma Process Polym. 14:e1600103. doi: 10.1002/ppap.201600103
Silva, T., Grofulović, M., Klarenaar, B. L. M., Morillo-Candas, A. S., Guaitella, O., Engeln, R., et al. (2018). Kinetic study of low-temperature CO2 plasmas under non-equilibrium conditions. I. Relaxation of vibrational energy. Plasma Sources Sci. Technol. 27:015019. doi: 10.1088/1361-6595/aaa56a
Slaets, J., Ceulemans, S., Van Alphen, S., Aghaei, M., and Bogaerts, A. (2020). CO2 and CH4 conversion in “real” gas mixtures in a gliding arc plasmatron: how do N2 and O2 affect the performance? Green Chem. 22, 1366–1377. doi: 10.1039/C9GC03743H
Snoeckx, R., and Bogaerts, A. (2017). Plasma technology – a novel solution for CO2 conversion? Chem. Soc. Rev. 46, 5805–5863. doi: 10.1039/C6CS00066E
Snoeckx, R., Heijkers, S., Van Wesenbeeck, K., Lenaerts, S., and Bogaerts, A. (2016). CO2 conversion in a dielectric barrier discharge plasma: N2 in the mix as helping hand of problematic impurity? Energy Environm. Sci. 9, 999–1011. doi: 10.1039/C5EE03304G
Snoeckx, R., Ozkan, A., Reniers, F., and Bogaerts, A. (2017). The quest for value-added products from carbon dioxide and water in a dielectric barrier discharge plasma: a chemical kinetics study. ChemSusChem 10, 409–424. doi: 10.1002/cssc.201601234
Song, C., Liu, Q., Ji, N., Deng, S., Zhao, J., Li, Y., et al. (2018). Alternative pathways for efficient CO2 capture by hybrid processes- a review. Renew. Sust. Energy Rev. 82, 215–231. doi: 10.1016/j.rser.2017.09.040
Stewig, C., Schüttler, S., Urbanietz, T., Böke, M., and von Keudell, A. (2020). Excitation and dissociation of CO2 heavily diluted in noble gas atmospheric pressure plasma. J. Phys. D Appl. Phys. 53:125205. doi: 10.1088/1361-6463/ab634f
Sun, S. R., Wang, H. X., Mei, D. H., Tu, X., and Bogaerts, A. (2017). CO2 conversion in a gliding arc plasma: performance improvement based on chemical reaction modeling. J. CO2 Util. 17, 220–234. doi: 10.1016/j.jcou.2016.12.009
Terraz, L., Silva, T., Morillo-Candas, A., Guaitella, O., Tejero-del-Caz, A., Alves, L. L., et al. (2020). Influence of N2 on the CO2 vibrational distribution function and dissociation yield in non-equilibrium plasmas. J. Phys. D Appl. Phys. 53:094002. doi: 10.1088/1361-6463/ab55fb
Trenchev, G., and Bogaerts, A. (2020). Dual-vortex plasmatron – a novel plasma source for CO2 conversion. J. CO2 Utiliz. 39:101152. doi: 10.1016/j.jcou.2020.03.002
Trenchev, G., Kolev, S. T., Wang, W., Ramakers, M., and Bogaerts, A. (2017). CO2 conversion in a gliding arc plasmatron: multi-dimensional modeling for improved efficiency. J. Phys. Chem. C 121, 24470–24479. doi: 10.1021/acs.jpcc.7b08511
Trenchev, G., Nikiforov, A., Wang, W., Kolev, S.t., and Bogaerts, A. (2019). Atmospheric pressure glow discharge for CO2 conversion: model-based exploration of the optimum reactor configuration. Chem. Eng. J. 362, 830–841. doi: 10.1016/j.cej.2019.01.091
Uytdenhouwen, Y., Van Alphen, S., Michielsen, I., Meynen, V., Cool, P., and Bogaerts, A. (2018). A packed-bed DBD micro plasma reactor for CO2 dissociation: does size matter? Chem. Eng. J. 348, 557–568. doi: 10.1016/j.cej.2018.04.210
van ‘t Veer, K., Reniers, F., and Bogaerts, A. (2020). Zero-dimensional modelling of unpacked and packed bed dielectric barrier discharges: the role of vibrational kinetics in ammonia synthesis. Plasma Sources Sci. Technol. 29:045020. doi: 10.1088/1361-6595/ab7a8a
van den Bekerom, D. C. M., Palomares Linares, J. M., Verreycken, T., van Veldhuizen, E. M., Nijdam, S., Berden, G., et al. (2019). The importance of thermal dissociation in CO2 microwave discharges investigated by power pulsing and rotational raman scattering. Plasma Sources Sci. Technol. 28:055015. doi: 10.1088/1361-6595/aaf519
van den Bekerom, D. C. M., van de Steeg, A., van de Sanden, M. C. M., and van Rooij, G. J. (2020). Mode resolved heating dynamics in pulsed microwave CO2 plasma from laser Raman scattering. J. Phys. D Appl. Phys. 53:054002. doi: 10.1088/1361-6463/ab5311
Van Laer, K., and Bogaerts, A. (2015). Improving the conversion and energy efficiency of carbon dioxide splitting in a zirconia-packed dielectric barrier discharge reactor. Energy Technol. 3, 1038–1044. doi: 10.1002/ente.201500127
Van Laer, K., and Bogaerts, A. (2016). Fluid modeling of a packed bed dielectric barrier discharge plasma reactor. Plasma Sources Sci. Technol. 25:015002. doi: 10.1088/0963-0252/25/1/015002
van Rooij, G. J., Van Den Bekerom, D. C. M., Den Harder, N., Minea, T., Berden, G., Bongers, W. A., et al. (2015). Taming microwave plasma to beat thermodynamics in CO2 dissociation. Faraday Discuss. 183, 233–248. doi: 10.1039/C5FD00045A
Vermeiren, V., and Bogaerts, A. (2018). Supersonic microwave plasma: potential and limitations for energy-efficient CO2 conversion. J. Phys. Chem. C 122, 25869–25881. doi: 10.1021/acs.jpcc.8b08498
Vermeiren, V., and Bogaerts, A. (2019). Improving the energy efficiency of CO2 conversion in nonequilibrium plasmas through pulsing. J. Phys. Chem. C 123, 17650–17665. doi: 10.1021/acs.jpcc.9b02362
Wolf, A. (2020). Thermal aspects of CO2 conversion in the vortex-stabilized microwave plasma (Ph.D. dissertation), Eindhoven University of Technology, Eindhoven, Netherlands.
Wolf, A. J., Righart, T. W. H., Peeters, F. J. J., Bongers, W. A., and van de Sanden, M. C. M. (2020). Implications of thermo-chemical instability on the contracted modes in CO2 microwave plasmas. Plasma Sources Sci. Technol. 29:025005. doi: 10.1088/1361-6595/ab5eca
Wolf, A. J., Righart, T. W. H., Peeters, F. J. J., Groen, P. W. C., van de Sanden, M. C. M., and Bongers, W. A. (2019). Characterization of CO2 microwave plasma based on the phenomenon of skin-depth limited contraction. Plasma Sources Sci. Technol. 28:115022. doi: 10.1088/1361-6595/ab4e61
Yang, T., Shen, J., Ran, T., Li, J., Chen, P., and Yin, Y. (2018). Understanding CO2 decomposition by thermal plasma with supersonic expansion quench. Plasma Sci. Technol. 20:065502. doi: 10.1088/2058-6272/aaa969
Zeng, Y., Zhu, X., Mei, D., Ashford, B., and Tu, X. (2015). Plasma-catalytic dry reforming of methane over Al2O3 supported metal catalysts. Catal. Today. 256, 80–87. doi: 10.1016/j.cattod.2015.02.007
Keywords: plasma, plasma catalysis, dielectric barrier discharge, microwave plasma, gliding arc, CO2 conversion, dry reforming, energy efficiency
Citation: Bogaerts A and Centi G (2020) Plasma Technology for CO2 Conversion: A Personal Perspective on Prospects and Gaps. Front. Energy Res. 8:111. doi: 10.3389/fenrg.2020.00111
Received: 10 February 2020; Accepted: 11 May 2020;
Published: 07 July 2020.
Edited by:
Michele Aresta, IC2R srl, ItalyReviewed by:
Richard Van De Sanden, Dutch Institute for Fundamental Energy Research, NetherlandsCopyright © 2020 Bogaerts and Centi. This is an open-access article distributed under the terms of the Creative Commons Attribution License (CC BY). The use, distribution or reproduction in other forums is permitted, provided the original author(s) and the copyright owner(s) are credited and that the original publication in this journal is cited, in accordance with accepted academic practice. No use, distribution or reproduction is permitted which does not comply with these terms.
*Correspondence: Annemie Bogaerts, YW5uZW1pZS5ib2dhZXJ0c0B1YW50d2VycGVuLmJl
Disclaimer: All claims expressed in this article are solely those of the authors and do not necessarily represent those of their affiliated organizations, or those of the publisher, the editors and the reviewers. Any product that may be evaluated in this article or claim that may be made by its manufacturer is not guaranteed or endorsed by the publisher.
Research integrity at Frontiers
Learn more about the work of our research integrity team to safeguard the quality of each article we publish.