- 1Hefei National Laboratory for Physical Sciences at the Microscale, Department of Chemistry, University of Science & Technology of China, Hefei, China
- 2School of Environment and Energy, South China University of Technology, Guangzhou, China
- 3School of Materials Science and Engineering, Nanjing University of Science and Technology, Nanjing, China
Recently, Li-O2 batteries have been considered to be promising next-generation energy storage devices owing to their high theoretical specific energy. However, due to the sluggish reaction kinetics of oxygen conversion, practical applications cannot achieve the desired results. By introducing vacancies in the MoS2 basal plane and using an in-situ synthesis method, we demonstrated the excellent catalysis of MoS2−x for oxygen redox kinetics, which can improve Li-O2 battery performance. The prepared MoS2−x displays little polarization, with a potential gap of 0.59 V and a high discharge capacity of 8,851 mA h g−1 at a current density of 500 mA g−1. The improved performance is mainly attributable to abundant S defects and plentiful diffusion channels in the MoS2−x/carbon 3D structural cathodes, which enable the adsorption of gaseous oxygen, reaction intermediates, and discharge products. To the best of our knowledge, these structures fabricated through 3D network design and surface modulation are among the best oxygen conversion catalysts developed so far, offering a new vista for the design of Li-O2 catalysts and beyond.
Introduction
Rechargeable non-aqueous Li-O2 batteries, which have an ultra-high theoretical energy density (>3,450 Wh Kg−1), have been widely studied as a promising alternative energy storage system for the next generation of long-range electric vehicles and other high-energy devices (Cheng and Chen, 2012; Zhang P. et al., 2018; Zhang X. et al., 2018). Although the operation of Li-O2 batteries is based on a simple electrochemical reaction (2Li+ + O2 + 2e− > Li2O2, E0 = 2.96 V), on the cathode, where there is a triple-phase contact interface, much more complicated in-situ reactions occur consisting of an oxygen reduction reaction (ORR) and an oxygen evolution reaction (OER) (Xu et al., 2016; Lyu et al., 2017). During discharge, ORR has been generally accepted to occur in two major steps. Firstly, O2 is reduced to , followed by combination with Li+ to form LiO2. Then, these superoxide intermediates undergo further electrochemical reduction or chemical disproportionation to form Li2O2, which is deposited on the cathode (Aurbach et al., 2016; Dong et al., 2018; Chaozhu et al., 2019). The charge process (OER) is far more complicated since the mechanism is indistinct and there are multiple charging intermediates, such as Li2−xO2, LiO2, and (Li and Chen, 2017; Lim et al., 2017). Many researchers have demonstrated that the bonding ability of the reaction intermediates to the solid (Li2O2)-solid (catalyst) interface is a key factor governing OER activity in the presence of a catalyst (Lu et al., 2013; Zhu et al., 2015; Lai et al., 2018). In general, a series of reactions starting from the adsorption of gaseous oxygen place great demands on the active sites on the cathode surface, while simultaneously, the deposition of a large amount of insulating Li2O2 also requires that the cathode structure provide sufficient accommodation space (Liu et al., 2015; Feng et al., 2016). These sluggish and unsustainable reactions on the triple-phase contact interface of the cathode always result in poor round-trip efficiency, limited rate capacity, and weak durability, which are critical defects for Li-O2 batteries, severely hindering their practical application. Thus, designing catalysts with high activity and a porous structure to promote kinetics reaction is a prerequisite for further advancement of Li-O2 technology (Chang et al., 2017; Hu X. et al., 2017; Wu et al., 2017).
Until now, numerous studies have been devoted to exploring alternative electrocatalysts and designing a good cathode architecture as the main strategies for improving the kinetics of oxygen conversion. Among them, molybdenum disulfide (MoS2) has outstanding performance and low cost as a star catalyst (Asadi et al., 2016, 2018; Behranginia et al., 2016). However, as a typical layered transition metal dichalcogenide, its reactivity is mainly attributable to the low-coordinated unsaturated fringe zone of the layered structure, and yet the predominantly highly exposed basal plane atoms have not yet exerted their unique structural advantages (Jaramillo et al., 2007). In the field of electrochemical catalysis and Li-S batteries, a lot of research has focused on increasing the number of edge active sites and modifying the basal plane to further optimize its activity (Li et al., 2011; Kibsgaard et al., 2012; Xie et al., 2013; Yin et al., 2016; Hu J. et al., 2017). For instance, through introducing edge defect sites and doping oxygen to increase chaos, Xie et al. promoted the transfer of plane electrons to the edge to enhance their electrochemical reactivity (Xie et al., 2013; Xie J. et al., 2013). Lin et al. found that MoS2−x/rGO with defective sites can effectively catalyze the conversion of lithium polysulfide and accelerate its oxidation-reduction reaction kinetics. Defects such as MoS2 edge sites and terrace surfaces have exhibited great electrochemical activity for the deposition of discharge products (Lin et al., 2017). Thus, surface regulation of MoS2 to improve intrinsic conductivity and the number of active sites exposed on the basal plane could greatly improve catalysis activity, especially in Li-O2 systems that have high requirements on the adsorption of active sites.
To essentially manipulate the intrinsic properties of MoS2 to further enhance its application value for cathode catalysis, as well as to study the effect of S defects on MoS2 activity in Li-O2 batteries, we prepared sulfur-deficient MoS2 nanoflakes (MoS2−x) growing in-situ on macroporous carbon paper as the binder-free Li-O2 battery cathode. The 3D hierarchical porous cathode structure not only provides excellent electrical conductivity but also facilitates efficient gas transportation and lithium-ion diffusion and accommodates a large number of discharge products to increase battery capacity. Impressively, in the presence of the S defects in MoS2−x on porous carbon paper, the cathode exhibits excellent oxygen redox catalytic performance, with an overall potential gap of 0.59 V and a discharge capacity of 8,851 mA h g−1. Scanning electron microscopy (SEM) images obtained after discharge also show the different Li2O2 morphologies formed on MoS2 and MoS2−x, which relate to the adsorption of intermediates onto their surface. To the best of knowledge, this performance makes MoS2−x as one of the best oxygen conversion catalysts, and these methods of surface modulation could offer a new vista for the design of Li-O2 catalysts and beyond.
Experimental Details
Material Synthesis
In a typical procedure, 0.196 g ammonium molybdate [(NH4)6Mo7O24·4H2O] and 3.92 g sodium thiosulfate (Na2S2O3) were dissolved in 18 mL deionized water with ultrasonic assistance. Then, the solution was transferred into a 20-mL Teflon-lined stainless-steel autoclave with punched carbon paper with a diameter of 11 mm as the growth substrate. After keeping the hydrothermal reaction temperature at 200°C for 20 h, the carbon paper was taken out, cleaned with deionized water and ethanol, and dried in a vacuum oven at 60°C for 10 h. MoS2 growing on carbon paper was obtained at a loading of 0.8–1.0 mg. The MoS2−x sample was formed by heating the prepared MoS2 in a 10% H2/Ar atmosphere at 500°C for 3 h.
Structural Characterization
The morphologies were characterized by scanning electron microscopy (SEM, JEOL-JSM-6700F), transmission electron microscopy (TEM, Hitachi H7650), and high-angle annular dark-field scanning TEM (HAADF-STEM, JEOL JEM-ARF200F TEM/STEM). X-ray diffraction (XRD) measurement was conducted on a Philips X'pert Super diffractometer with Cu Kα, λ=1.54178 Å, and Raman spectra were recorded on a Renishaw RM 3000 Micro-Raman system. X-ray photoelectron spectroscopy (XPS, ESCALAB 250 spectrometer, Perkin-Elmer) was collected at the BL10B of the National Synchrotron Radiation Laboratory (NSRL). The surface area and pore distribution plots were collected by Brunauer–Emmett–Teller (BET, Micromeritics ASAP 2020).
Electrochemical Measurements
The Li-O2 electrochemical performance was measured in 2,032 cells with 19 pores on the steel cathode shells. All of the cells are assembled in an Ar-filled glove box (O2, H2O < 1 ppm) by using a lithium metal foil as the anode, a glass fiber separator as the separator, the prepared electrode as the gas cathode, and 75 vol% dimethyl sulfoxide (DMSO) and 25 vol% 1-ethyl-3-methylimidazolium tetrafluoroborate (EMIM-BF4) with 0.1 M lithium bis(trifluoromethanesulfonyl)imide (LiTFSI) (>99.0%) as the electrolyte. The cathode prepared by the coating method was made from a powder slurry of the corresponding material. The powdered samples were mixed with acetylene black and poly (vinylidene difluoride) (PVDF) in an 8:1:1 ratio, and commercial CNT samples were mixed with PVDF at 9:1 in N-methyl-2-pyrrolidone (NMP). The coating slurry was then applied to the carbon paper with a loading of 0.7 mg. The electrochemical performances of the cathodes were tested using a specific capacity-controlled mode at various current densities in pure O2. Electrochemical impedance spectroscopy (EIS) were performed under open-circuit voltage with the frequency ranging from 1 Hz to 100 kHz.
Results and Discussion
Material and Structural Characterization
As shown schematically in Figure 1a, molybdenum source and sulfur source will nucleate and grow into flake-like MoS2 crystals through hydrothermal reaction under high temperature and high pressure. When treated in an H2/Ar atmosphere at high temperature, part of the sulfur will be taken away by the reducing atmosphere to form H2S, leaving a lot of S defects on the MoS2 basal plane. MoS2−x with S defects shows more layers than do pristine MoS2 nanoflakes and has larger surface area, as the SEM images in Figure S1 show. It can also be seen from the TEM images in Figure S2 that MoS2−x has thinner nanoflakes at the edges, which may attribute to non-stoichiometric MoS2−x with increased disorder of the atomic arrangement. It is these disordered S defects that endow the inert basal plane with a large amount of active sites at which to adsorb oxygen and discharge/charge intermediates and discretely induce the deposition of discharge products, promoting a series of oxygen redox catalytic processes. The SEM image in Figure 1b shows MoS2−x nanoflakes uniformly and densely growing on carbon paper; this is enlarged in Figure S3. The TEM image and its enlarged view in Figure 1c and Figure S4, respectively, display the super-thin layer-like structure of MoS2−x nanoflakes, which are about several nanometers thick. The high-resolution (HRTEM) image in Figure 1d reveals well-resolved lattice fringes with an interplanar spacing of 0.62 nm, which are assigned to the (002) plane of hexagonal MoS2. We also performed HRTEM to characterize the defects of the MoS2−x basal plane, as shown in Figure S5. The red regular hexagon indicates that the shooting angle is focused on the basal surface of hexagonal MoS2−x. Many defects can be seen on the basal plane, as marked by the brown circle.
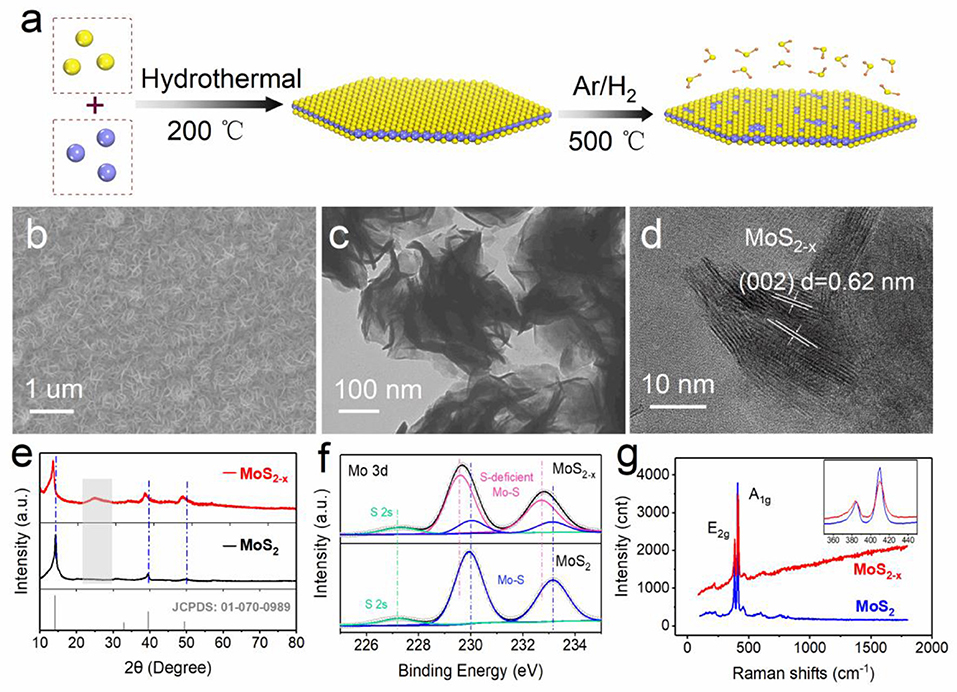
Figure 1. Material synthesis and characterization. (a) Schematic of the synthesis. (b) SEM image of MoS2−x on carbon paper. (c) TEM image of MoS2−x. (d) High-resolution TEM of MoS2−x. (e) XRD patterns of MoS2−x and MoS2. (f) XPS core-level Mo 3d spectra from MoS2−x and MoS2. (g) Raman spectra of MoS2−x and MoS2.
The effects of S defects on the structure of MoS2−x were analyzed by XRD, and the results are seen in Figure 1e. The diffraction peaks of the MoS2 nanoflakes can be well converted to No. 01-070-0989. For MoS2−x, there was a slight shift to a lower degree, indicating an increase in the lattice spacing, caused most likely by the removal of sulfur atoms. The resultant reduction of Mo, moving it to a lower oxidation state with a larger atomic radius, led to the increase in the lattice spacing. In addition, newly emerging diffraction peaks between 20 and 30 degrees represent the increase in the degree of disorder of the MoS2 lattice. XPS analysis was conducted to obtain insights into the chemical composition and electronic states of MoS2−x and MoS2. Figure 1f displays the deconvoluted XPS Mo 3d spectra, in which MoS2−x exhibits the characteristic peaks of both stoichiometric MoS2 (blue) and sulfur-deficient MoS2 (red). Specifically, the sulfur-deficient MoS2 possesses lower binding energy than stoichiometric MoS2 in Mo 3d5/2 (229.6 eV compared with 230.02 eV) and 3d3/2 (232.71 eV compared with 233.11 eV) doublets, illustrating the decrease in the valence state of Mo. Besides, the peak at 227.3 eV is attributed to S 2s, and the XPS total survey spectra and S 2p spectra are also displayed in Figure S6. In addition, the structural differences can also be detected by Raman spectroscopy. In Figure 1g, the fingerprint bands of MoS2 are located at 376 and 402 cm−1, caused by the in-plane (E2g) and out-plane (A1g) Mo-S phonon mode vibration of MoS2, respectively. Obviously, the weakening of Raman peak intensity can be explained by the decrease in the content of vibration groups. The Raman peak is a statistical result, and the same peak broadening is due to lower structural uniformity of the vibrating molecules, which is caused by inducing S defects.
Considering the huge influence of the electrode structure on the performance of the battery, the loading methods of the catalyst MoS2−X on porous carbon paper are also studied here. We compare a cathode of catalyst grown in-situ on carbon paper with a cathode coated with catalyst powder and find that the coated slurry easily blocked the original pores of the carbon paper and blocked the gas channel, as shown in Figures 2a,b; this can be compared with the pure carbon paper framework in Figure S7. Besides, the slurry used in the coating method will destroy the uniform arrangement of the catalyst on the conductive carbon paper, and the micron-level insulating binder used therein will also greatly affect the conductivity of the electrode, as shown in Figures 2c,d. N2 adsorption–desorption techniques were employed to characterize the surface structural features. MoS2−x (growing on carbon paper) exhibits a surface area of 64.587 m2 g−1, which is superior to the MoS2−x coating on carbon paper (24.378 m2 g−1) and pure carbon paper (9.093 m2 g−1), as shown in Figures 2e,f and Figure S8. The isotherm profile in Figure 2c is categorized as type IV with a hysteresis loop (H3), and the pore-size distribution determined by the BJH shows pore diameters centered at a range of 4–30 nm (mainly mesopores). This meaningful 3D hierarchical porous structure not only acts as ion and gas transmission channels but also contributes to increasing the specific surface area to deliver more active sites and sufficient room to accommodate solid discharge products to achieve satisfying specific capacity performance.
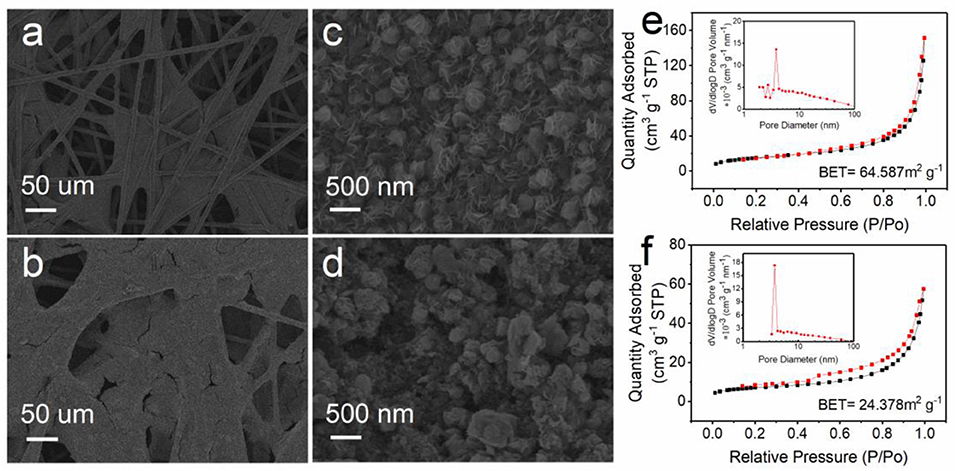
Figure 2. Structural characterization of MoS2−x growing on or coating carbon paper. (a,b) SEM images. (c,d) Enlarged SEM view of (a,b). (e,f) Nitrogen adsorption and desorption isotherms and pore-size distribution.
Electrochemical Performance of Li-O2 Batteries
To examine the electrochemical properties of the prepared MoS2−x and MoS2 cathodes, Kejen Black (denoted as KB) was employed as a comparison, and Li-O2 2032 coin cells were assembled. At a current of 500 mA g−1 and a cutoff capacity of 1,000 mA h g−1, the discharge and charge potential gap of MoS2−x is 0.59 V, much lower than that of MoS2 (0.87 V) and Kejen Black (1.03 V) at half capacity, as shown in Figure 3a. During the charge process, the charge potential platform of MoS2−x is 3.55 V and the overpotential is 0.57 V, which is significantly lower than for MoS2 (3.79, 0.81 V) and Kejen Black (3.96, 0.98 V), indicating excellent OER catalytic activity. For the discharge (ORR) process, as is enlarged in Figure 3b, MoS2−x displayed a discharge platform at 2.75 V, higher than MoS2 (2.71 V) and Kejen Black (2.68 V). Compared with the cathode constructed by the coating method, both MoS2−x and MoS2 have far superior performance in reducing overpotential, demonstrating the structural advantages of a self-growing cathode for Li-O2 batteries, as shown in Figure S9. The corresponding round-trip efficiency based on calculus is 85.56% for MoS2−x, much higher than the values of 76.46, 76.61, 74.85, and 71.9% obtained for MoS2 growing on carbon paper, MoS2−x, MoS2, and Kejen Black coating on carbon paper, respectively, as shown in Figure S10. Furthermore, to gain an insight into the full specific capacity of the MoS2−x cathode, the MoS2−x and MoS2-based Li-O2 batteries were tested under deep discharge/charge and limited capacity conditions. The MoS2 cathode could deliver a high discharge capacity of about 6,312 mA h g−1 during the discharge process, while the charge process showed quite serious polarization and poor reversibility (Figure 3c). On the contrary, the MoS2−x cathode could not only obtain a discharge capacity as high as 8,851 mA h g−1 but also exhibited better reversibility with lower charging energy. This improved performance can be attributed to it being a monolithic cathode with a hierarchical porous structure and extensive active deposition sites provided by S defects, which is vital for an O2-based electrode in an energy storage device. We also performed extended galvanostatic discharge and charge tests with the current density varying from 200 to 2,000 mA g−1 to further assess the rate performance. The results are shown in Figure S11. The MoS2−x cathode exhibits a small polarization with clear voltage plateaus and superior reversibility, demonstrating resistance to large currents and accelerated electrochemical reactions.
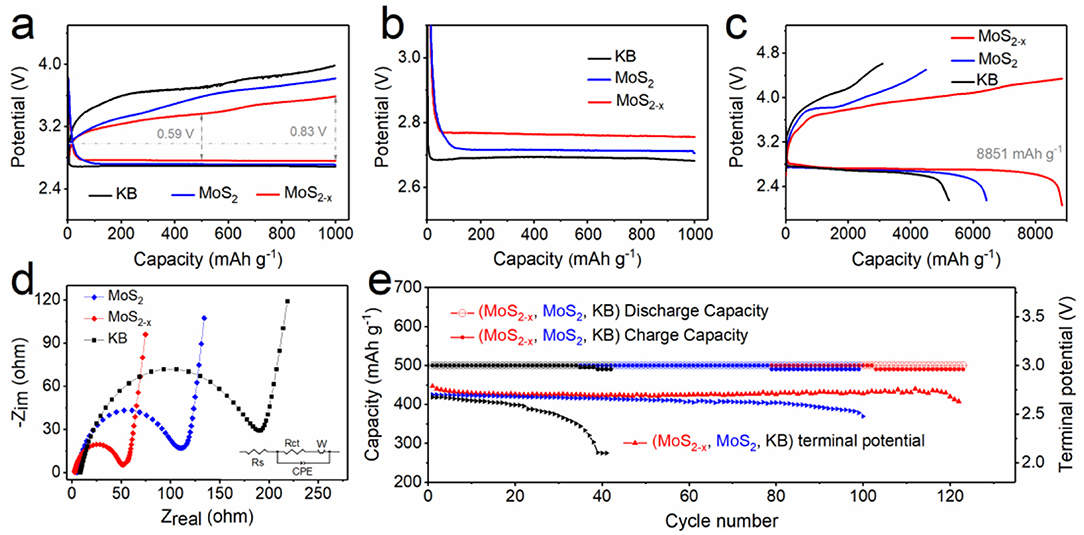
Figure 3. Electrochemical properties. (a) First cycling galvanostatic discharge and recharge profiles of MoS2−x, MoS2, and Kejen Black. (b) Enlarged image of the discharge curves in (a). (c) Full discharge and recharge curves of Li–O2 batteries with MoS2−x and MoS2 cathodes. (d) EIS spectra of MoS2−x, MoS2, and Kejen Black at open-circuit voltages. The inset is the equivalent circuit. (e) Cycling stability and the terminal discharge potential of MoS2−x and MoS2 cathodes.
In addition, we conducted an EIS test at open-circuit voltage to study the interfacial kinetics in Li-O2 batteries (Figure 3d), with the Nyquist plots fitted by an equivalent electrical circuit (Figure 3d, inset). MoS2−x exhibits a lower charge-transfer resistance (Rct) of 50.8 Ω compared with MoS2 (108.4 Ω) and Kejen Black (188.94 Ω), indicating that it has ultrafast charge transfer behavior. MoS2−x achieves a lower energy barrier to trigger and support robust oxygen conversion and charge transfer, demonstrating a significant promotion of ORR and OER catalytic activity in Li-O2 batteries. As shown in Figure 3e, the long cycling stability was tested at 500 mA g−1 with a cutoff capacity of 1,000 mA h g−1. The terminal discharge potential of the MoS2 cathode obviously decreases to 2.5 V after 99 cycles, while the battery with a MoS2−x cathode can achieve more than 123 cycles before the terminal discharge potential reaches 2.6 V, displaying that it has superior reversibility and cycling stability. Based on the above results, we can conclude that the introduction of S defects greatly improves the cathode catalytic activity and capacity for Li-O2 batteries.
Morphological Evolution of the Discharge Products
The morphologies of discharge products are accepted to be significant factors affecting the subsequent electrochemical reaction. Thus, SEM investigations were carried out to characterize the evolution of discharge products. After discharging to 500 mA g−1 for 1 h, the pores of the cathode were partially covered with film-like discharge products, as shown in Figures 4a,b. After continued discharge to 1,000 mA h g−1, thicker aggregates were present on the MoS2−x cathode (Figure 4c), indicating the continuous reduction of oxygen into discharge products and deposition on the cathode. For direct comparison, the typical morphologies of the MoS2 cathode after discharging were also tested, as shown in Figures 4d–f. After discharging to 1,000 mA h g−1, the cathode can be seen to be uniformly covered with patelloid products in Figure 4c and Figure S12, suggesting that the MoS2 cathode behaves quite differences from the MoS2−x cathode. During the discharging process, the exertion of a strong adsorption force on the intermediate discharge products always led the superoxide intermediate products to experience further electrochemical reduction, thus forming a film-like discharge product, while weak adsorption often led to large-sized crystal discharge products, which need higher energy for oxidative decomposition. On the surface of the MoS2−x cathode, strong adsorption to discharge intermediates can be attributed to S defects, which enhance the adsorption activity and the number of adsorption sites and provide an excellent prerequisite for the subsequent charging process.
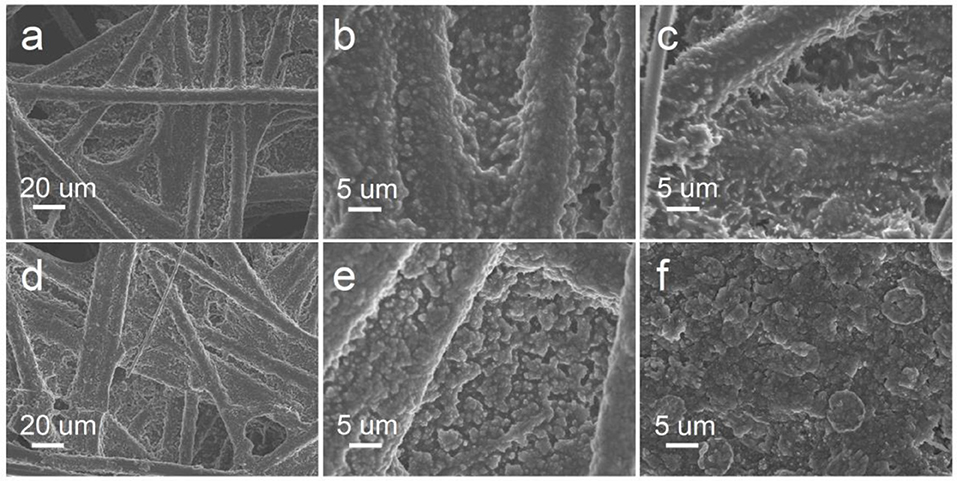
Figure 4. Morphological evolution of the discharge products. (a) SEM image of MoS2−x after discharge to 500 mA h g−1. (b) Enlarged view of (a). (C) SEM image of MoS2−x after discharge to 1,000 mA h g−1. (d) SEM image of MoS2 after discharge to 500 mA h g−1. (e) Enlarged view of (d). (f) SEM image of MoS2 after discharge to 1,000 mA h g−1. Galvanostatic discharge with current at 500 mA g−1.
Conclusions
In summary, we have demonstrated that MoS2 with S defects can intrinsically promote the corresponding thermodynamic and kinetic processes in Li-O2 batteries. XRD and XPS are used to characterize the defects of the MoS2 materials, which can act as active centers for the adsorption and conversion of superoxide intermediate products. SEM and BET tests demonstrate that in-situ growth on carbon paper can be used to successfully fabricate a multilayer hierarchical 3D structure that can provide abundant channels for ion and gas molecule diffusion. Due to these advantages, a MoS2−x electrode exhibits an overpotential of 0.59 V and a discharge capacity of 8,851 mA h g−1 at a current density of 500 mA g−1, showing the best performance. Moreover, the large number and film-like distribution of discharge products on the MoS2−x surface means a stronger adsorption effect on intermediate products. Therefore, this study, in designing the 3D host materials and tuning the electronic structure of the MoS2 surface, paves a new way to the high-energy application of Li-O2 batteries.
Data Availability Statement
The original contributions presented in the study are included in the article/Supplementary Material, further inquiries can be directed to the corresponding author/s.
Author Contributions
GW and TZ designed and supervised the project. GW, ZP, and YL conducted the project and wrote and revised the manuscript. YZ conducted XPS measurements. XL helped to disassemble the batteries. ZL and SN performed SEM characterization and provided valuable help in the data analysis. All of the authors discussed the results and commented on the manuscript.
Funding
This work was supported by the National Key Research and Development Program of China (2017YFA0206703), the Natural Science Fund of China (Nos. 21771169, 11722543, and 11505187), the Fundamental Research Funds for the Central Universities (WK2060190074, WK2060190081, and WK2310000066), USTC start-up funding, and the Recruitment Program of Global Experts.
Conflict of Interest
The authors declare that the research was conducted in the absence of any commercial or financial relationships that could be construed as a potential conflict of interest.
Acknowledgments
We thank the Photoemission End stations at BL10B, the National Synchrotron Radiation Laboratory, for XPS characterizations.
Supplementary Material
The Supplementary Material for this article can be found online at: https://www.frontiersin.org/articles/10.3389/fenrg.2020.00109/full#supplementary-material
References
Asadi, M., Kumar, B., Liu, C., Phillips, P., Yasaei, P., Behranginia, A., et al. (2016). Cathode based on molybdenum disulfide nanoflakes for lithium-oxygen batteries. ACS Nano 10, 2167–2175. doi: 10.1021/acsnano.5b06672
Asadi, M., Sayahpour, B., Abbasi, P., Ngo, A. T., Karis, K., Jokisaari, J. R., et al. (2018). A lithium-oxygen battery with a long cycle life in an air-like atmosphere. Nature 555, 502–507. doi: 10.1038/nature25984
Aurbach, D., McCloskey, B. D., Nazar, L. F., and Bruce, P. G. (2016). Advances in understanding mechanisms underpinning lithium-air batteries. Nat. Energy 1, 1–11. doi: 10.1038/nenergy.2016.128
Behranginia, A., Asadi, M., Liu, C., Yasaei, P., Kumar, B., Phillips, P., et al. (2016). Highly efficient hydrogen evolution reaction using crystalline layered three-dimensional molybdenum disulfides grown on graphene film. Chem. Mater. 28, 549–555. doi: 10.1021/acs.chemmater.5b03997
Chang, Z., Xu, J., and Zhang, X. (2017). Recent progress in electrocatalyst for Li-O2 batteries. Adv. Energy Mater. 7:1700875. doi: 10.1002/aenm.201700875
Chaozhu, S., Jiazhao, W., Jianping, L., Hua-Kun, L., and Shi-Xue, D. (2019). Understanding the reaction chemistry during charging in aprotic lithium–oxygen batteries: existing problems and solutions. Adv. Mater. 31:1804587. doi: 10.1002/adma.201804587
Cheng, F., and Chen, J. (2012). Metal-air batteries: from oxygen reduction electrochemistry to cathode catalysts. Chem. Soc. Rev. 41, 2172–2192. doi: 10.1039/c1cs15228a
Dong, J.-C., Zhang, X.-G., Briega-Martos, V., Jin, X., Yang, J., Chen, S., et al. (2018). In situ Raman spectroscopic evidence for oxygen reduction reaction intermediates at platinum single-crystal surfaces. Nat. Energy 4, 60–67. doi: 10.1038/s41560-018-0292-z
Feng, N., He, P., and Zhou, H. (2016). Critical challenges in rechargeable aprotic Li-O2 batteries. Adv. Energy Mater. 6:1502303. doi: 10.1002/aenm.201502303
Hu, J., Zhang, C., Jiang, L., Lin, H., An, Y., Zhou, D., et al. (2017). Nanohybridization of MoS2 with layered double hydroxides efficiently synergizes the hydrogen evolution in alkaline media. Joule 1, 383–393. doi: 10.1016/j.joule.2017.07.011
Hu, X., Wang, J., Li, Z., Wang, J., Gregory, D. H., and Chen, J. (2017). MCNTs@MnO2 nanocomposite cathode integrated with soluble O2-carrier co-salen in electrolyte for high-performance Li-air batteries. Nano Lett. 17, 2073–2078. doi: 10.1021/acs.nanolett.7b00203
Jaramillo, T. F., Jorgensen, K. P., Bonde, J., Nielsen, J. H., Horch, S., and Chorkendorff, I. (2007). Identification of active edge sites for electrochemical H2 evolution from MoS2 nanocatalysts. Science 317, 100–102. doi: 10.1126/science.1141483
Kibsgaard, J., Chen, Z., Reinecke, B. N., and Jaramillo, T. F. (2012). Engineering the surface structure of MoS2 to preferentially expose active edge sites for electrocatalysis. Nat. Mater. 11, 963–969. doi: 10.1038/nmat3439
Lai, N. C., Cong, G., Liang, Z., and Lu, Y. C. (2018). A highly active oxygen evolution catalyst for lithium-oxygen batteries enabled by high-surface-energy facets. Joule 2, 1511–1521. doi: 10.1016/j.joule.2018.04.009
Li, F., and Chen, J. (2017). Mechanistic evolution of aprotic lithium-oxygen batteries. Adv. Energy Mater. 7:1602934. doi: 10.1002/aenm.201602934
Li, Y., Wang, H., Xie, L., Liang, Y., Hong, G., and Dai, H. (2011). MoS2 nanoparticles grown on graphene: an advanced catalyst for the hydrogen evolution reaction. J. Am. Chem. Soc. 133, 7296–7299. doi: 10.1021/ja201269b
Lim, H. D., Lee, B., Bae, Y., Park, H., Ko, Y., Kim, H., et al. (2017). Reaction chemistry in rechargeable Li-O2 batteries. Chem. Soc. Rev. 46, 2873–2888. doi: 10.1039/c6cs00929h
Lin, H., Yang, L., Jiang, X., Li, G., Zhang, T., Yao, Q., et al. (2017). Electrocatalysis of polysulfide conversion by sulfur-deficient MoS2 nanoflakes for lithium–sulfur batteries. Energy Environ. Sci. 10, 1476–1486. doi: 10.1039/c7ee01047h
Liu, Q. C., Xu, J. J., Xu, D., and Zhang, X. B. (2015). Flexible lithium-oxygen battery based on a recoverable cathode. Nat. Commun. 6, 1–8. doi: 10.1038/ncomms8892
Lu, Y.-C., Gallant, B. M., Kwabi, D. G., Harding, J. R., Mitchell, R. R., Whittingham, M. S., et al. (2013). Lithium–oxygen batteries: bridging mechanistic understanding and battery performance. Energy Environ. Sci. 6, 750. doi: 10.1039/c3ee23966g
Lyu, Z., Zhou, Y., Dai, W., Cui, X., Lai, M., Wang, L., et al. (2017). Recent advances in understanding of the mechanism and control of Li2O2 formation in aprotic Li-O2 batteries. Chem. Soc. Rev. 46, 6046–6072. doi: 10.1039/c7cs00255f
Wu, S., Qiao, Y., Yang, S., Ishida, M., He, P., and Zhou, H. (2017). Organic hydrogen peroxide-driven low charge potentials for high-performance lithium-oxygen batteries with carbon cathodes. Nat. Commun. 8, 1–9. doi: 10.1038/ncomms15607
Xie, J., Zhang, H., Li, S., Wang, R., Sun, X., Zhou, M., et al. (2013). Defect-rich MoS2 ultrathin nanosheets with additional active edge sites for enhanced electrocatalytic hydrogen evolution. Adv. Mater. 25, 5807–5813. doi: 10.1002/adma.201302685
Xie, J. F., Zhang, J. J., Li, S., Grote, F., Zhang, X. D., Zhang, H., et al. (2013). Controllable disorder engineering in oxygen-incorporated MoS2 ultrathin nanosheets for efficient hydrogen evolution. J. Am. Chem. Soc. 135, 17881–17888. doi: 10.1021/ja408329q
Xu, J. J., Chang, Z. W., Wang, Y., Liu, D. P., Zhang, Y., and Zhang, X. B. (2016). Cathode surface-induced, solvation-mediated, micrometer-sized Li2 O2 cycling for Li-O2 batteries. Adv. Mater. 28, 9620–9628. doi: 10.1002/adma.201603454
Yin, Y., Han, J., Zhang, Y., Zhang, X., Xu, P., Yuan, Q., et al. (2016). Contributions of phase, sulfur vacancies, and edges to the hydrogen evolution reaction catalytic activity of porous molybdenum disulfide nanosheets. J. Am. Chem. Soc. 138, 7965–7972. doi: 10.1021/jacs.6b03714
Zhang, P., Zhao, Y., and Zhang, X. (2018). Functional and stability orientation synthesis of materials and structures in aprotic Li-O2 batteries. Chem. Soc. Rev. 47, 2921–3004. doi: 10.1039/c8cs00009c
Zhang, X., Mu, X., Yang, S., Wang, P., Guo, S., Han, M., et al. (2018). Research progress for the development of Li-air batteries: addressing parasitic reactions arising from air composition. Energy Environ. Mater. 1, 61–74. doi: 10.1002/eem2.12008
Keywords: MoS2-x, defects, catalytic sites, cathode, Li-O2 battery
Citation: Liu Y, Zang Y, Liu X, Cai J, Lu Z, Niu S, Pei Z, Zhai T and Wang G (2020) Three-Dimensional Carbon-Supported MoS2 With Sulfur Defects as Oxygen Electrodes for Li-O2 Batteries. Front. Energy Res. 8:109. doi: 10.3389/fenrg.2020.00109
Received: 21 April 2020; Accepted: 11 May 2020;
Published: 24 June 2020.
Edited by:
Jun Yan, Harbin Engineering University, ChinaReviewed by:
Liang Huang, Huazhong University of Science and Technology, ChinaXu Xiao, Drexel University, United States
Copyright © 2020 Liu, Zang, Liu, Cai, Lu, Niu, Pei, Zhai and Wang. This is an open-access article distributed under the terms of the Creative Commons Attribution License (CC BY). The use, distribution or reproduction in other forums is permitted, provided the original author(s) and the copyright owner(s) are credited and that the original publication in this journal is cited, in accordance with accepted academic practice. No use, distribution or reproduction is permitted which does not comply with these terms.
*Correspondence: Zhibin Pei, cGVpemImI3gwMDA0MDtzY3V0LmVkdS5jbg==; Gongming Wang, d2FuZ2dtJiN4MDAwNDA7dXN0Yy5lZHUuY24=