- 1Key Laboratory for UV Light-Emitting Materials and Technology of Ministry of Education, Northeast Normal University, Changchun, China
- 2National & Local United Engineering Lab for Power Battery, Faculty of Chemistry, Northeast Normal University, Changchun, China
The fluorophosphate NaVPO4F (NVPF) is a good candidate of cathode material for sodium-ion batteries (SIBs) due to its high theoretical specific capacity, high working voltage and stable structure. However, due to the low electronic conductivity of NVPF, its electrochemical properties are difficult to demonstrate. In order to address the insufficient and then enhance its electrochemical performance, a 3D carbon networks constructed NaVPO4F/C/rGO (NVPF/C/rGO) nanocomposite is prepared by freeze-drying assisted high-temperature solid-state method. When used as a cathode material for SIBs, the prepared NVPF/C/rGO can deliver a capacity of about 108.7 mA h g−1 at 0.05 C. Moreover, NVPF/C/rGO nanocomposite also exhibits the excellent electrochemical performance, including superior rate capacities (about 65.8 mA h g−1 specific capacity at 10 C) and outstanding cycling performance (~95.1% capacity retention after 200 cycles at 0.05 C), which can be attribute to the 3D carbon networks and the nanoparticles in NVPF/C/rGO nanocomposite. The preliminary results illustrate that the 3D carbon networks constructed NVPF/C/rGO could be a promising cathode material for SIBs.
Introduction
Nowadays, with the massive use of fossil energy, carbon dioxide emissions are increasing, thus aggravating the global warming (Dunn et al., 2011; Barpanda et al., 2014; Che et al., 2017). In response to this situation, it has become urgent to develop new renewable energy sources to replace the fossil energy (Kim et al., 2016; Lao et al., 2017; Yan et al., 2017). However, the renewable energy, such as wind, solar and tidal energy, is inherently intermittent and inconsecutive, and has strong regional characteristics. Therefore, advanced energy storage technology is more important for the development and application of renewable energy. Among them, electrochemical rechargeable batteries have the advantages of high energy density, long lifespans, simpler maintenance, etc., which have been paid more attention and studied by researchers (Keller et al., 2016; Kim et al., 2016). Given the fact that the lithium has lower redox potential (-3.04 V vs. SHE) and smaller atomic radius (0.76 Å), the lithium-ion batteries (LIBs) tend to be more widely studied. Nevertheless, the cost of LIBs is rise because of the limited and unevenly distributed lithium source in the Earth's crust, which leads to a boom in the study of sodium-ion batteries (SIBs) (Hwang et al., 2016; Nayak et al., 2018).
SIBs and LIBs have similar intercalation mechanism, making it possible to use the LIBs matured materials in SIBs. In addition, sodium is abundant in nature and easy to extract, so SIBs are the more cost-effective energy storage technology. Despite of numerous advantages, the development of SIBs still faces many problems, such as sodium has higher redox potential (−2.71 V vs. SHE) and larger atomic radius (1.02 Å), leading to the low energy density of SIBs (Guo et al., 2017; Pang et al., 2017). However, with the development of sustainable energy alternatives, the scientists are working to find and optimize electrode materials to create commercially viable SIBs. So far, the various types of cathodes are being reported, which includes layered oxides, polyanionic compound and so on (Barpanda et al., 2012; Jian et al., 2013; Guo S. et al., 2015). Due to the high structural stability, long-term cycling life and high ion mobility of polyanion-based cathode materials, they are even more impressive in SIBs (Barpanda et al., 2012; Fang et al., 2017). At the meantime, the fluorophosphate is even more prominent in this class, mainly because that fluorophosphate compounds have strong P-O covalent bond, which leads to greatly structural stability; furthermore, as cathode material of SIBs, the fluorophosphate materials always have higher working voltage due to strong electronegativity of F− (Park et al., 2013; Li et al., 2018).
Among them, NaVPO4F (NVPF) is a good cathode material candidate for SIBs, which possesses high theoretical capacity (143 mAh g−1), high working voltage and stable structure (Law and Balaya, 2018; Ge et al., 2019). NVPF was originally proposed by Barker et al. (2003), it has a symmetrical tetragonal structure similar to that of Na3Al2(PO4)2F3, as a cathode material, NVPF only has a discharge capacity of 82 mAh g−1 in SIBs, and it decays to half after just 30 cycles. To improve electrochemical performance of NVPF, many strategies have been tried, including doping other metal ions, coating with some carbon materials and so on (Liu et al., 2008), which can improve the electrochemical properties of NaVPO4F to a certain extent, but their capacity is still far below its theoretical capacity, and can't meet the requirements of application (Ling et al., 2018). For the same purpose, we adopt freeze-drying assisted high-temperature solid-state method to prepare NVPF. As far as we know, for synthesis, freeze-drying is a low-cost and simple method, so that it could be a promising method for the future application (Rui et al., 2015). Meanwhile, in view of the poor electronic conductivity of NVPF, so improving electronic conductivity is the key to design high performance NVPF materials. Currently, combining with various types of carbon substrates is a universal and efficient method to enhance the conductivity of the electrode materials (Guo et al., 2015; Liu et al., 2015; Fan et al., 2017; Yin et al., 2017; Yang et al., 2020). However, a single carbon material has limited effect on the electrochemical performance of electrode materials. The conductivity of a single particle can be improved by using the carbon layer, but the effect on electron transport between particles is not significant. Moreover, the reduced graphene oxide (rGO) is regarded as one of the valued materials for improving the conductivity of materials, and has been widely used in many fields (Share et al., 2016; Liu et al., 2017).
Hence, in this work, we prepare a 3D carbon networks constructed NaVPO4F/C/rGO (abbr. NVPF/C/rGO, the C means the amorphous carbon derived from the organics) nanocomposite by freeze-drying assisted high-temperature solid-state method. In the nanocomposite, the amorphous carbon is coated on the surface of NVPF particles, and rGO layers cover on the surface of the NVPF/C particles and connect these to form a 3D conductive network. Such a modified NVPF/C/rGO nanocomposite used as a cathode material for SIBs exhibits remarkably improved electrochemical performance, especially high discharge capacity (about 108.7 mA h g−1 specific capacity at 0.05 C), superior rate capacities (about 65.8 mA h g−1 specific capacity at 10 C) and the long cycling performance (more than 95% of the initial capacity after 200 cycles at 0.05 C).
Experimental Section
Preparation of NVPF/C/rGO Nanocomposite
GO was firstly prepared by oxidation of graphite using the improved Hummers method (Hummers and Offeman, 1958; Lim et al., 2013). NVPF/C/rGO nanocomposite was prepared by a sample freeze-drying method. In a typical preparation, with the continuous stirring, the NaF, NH4H2PO4 and NH4VO3 with the molar ratio of 1:1:1 were dissolved in the distilled water, then added the polyvinyl pyrrolidone (PVP) and citric acid, which can be used not only as the complexing agent but also as the carbon source, furthermore, the PVP also prevents the aggregation of colloidal particles and makes them more stable. Stirring the mixture at 80°C until the powder samples were completely dissolved to form a light orange-yellow aqueous solution. The above solution and the GO aqueous solution was added to a small beaker, then the sample was evenly dispersed by ultrasonic bath for 2 h. Then, the sample was rapidly frozen with liquid nitrogen, and it was dried in a freeze dryer. Finally, the sample was calcined at 750°C under the Ar atmosphere for 5 h, and the final material NVPF/C/rGO nanocomposite was formed after grinding. In this process, the GO can be reduced to the rGO at high temperature, thus playing the role of building the 3D conductive network.
Material Characterization
The X-ray powder diffraction (XRD) on the D8 Bruker diffractometer with Cu Kα radiation (λ = 1.5406 Å) was used to detect the structure of the NVPF/C/rGO in scan range (2θ) of 10–60°. X-ray photoelectron spectroscopy (XPS) study was tested on X-ray photoelectron spectrophotometer (ESCALABMKLL) from VG. The morphology and microstructure of NVPF/C/rGO sample was characterized using the scanning electron microscope (SEM, HITACHI-SU8010, 10 kV) and transmission electron microscope (TEM, JEOL-2100 F, 200 kV).
Electrochemical Measurement
The electrochemical properties were measured in the CR2032 coin cells. The working electrodes were made by casting a slurry of active material, acetylene black, and polyvinyl difluoride (PVDF) with a mass ratio of 8: 1: 1 in N-methylpyrrolidone (NMP) on aluminum foil and dried in vacuum. The loading mass of the active material was about 1.2–1.5 mg cm−2. Pure sodium foil was used as both counter and reference electrodes, and the separator was glass microfiber filter (Whatman). Electrolyte was 1 mol L−1 NaClO4 dissolved in ethylene carbonate (EC) and propylene carbonate (PC) (vEC:vPC=1:1) with 2 vol% fluoroethylene carbonate (FEC) as the additive. The constant current charge-discharge tests were implemented at battery testing system (LAND CT2001A) at 2.5~4.1 V. Cyclic voltammetry (CV) curves were tested using a Princeton Applied Research (VERSASTAT 3) at 0.1 mV s−1. For galvanostatic intermittent titration (GITT) analyses, the cells were cycled in same potential range at 0.05 C.
Results and Discussion
The NVPF/C/rGO nanocomposite was prepared by freeze-drying assisted high-temperature solid-state method. As shown in Figure 1, the structure of NVPF/C/rGO nanocomposite was characterized according to XRD test. Consistent with the reports in the literatures (Law and Balaya, 2018; Ge et al., 2019), the diffraction pattern reveals that the peaks of NVPF/C/rGO nanocomposite are well indexed to the monoclinic crystal structure (space group C2/c) according to the standard card (PDF33-0804) (Liu et al., 2008). There are no obvious impurity peaks in XRD pattern, confirming that the NVPF was successfully prepared by solid-state method.
XPS test was implemented to study the elements composition and bonding configurations of NVPF/C/rGO, and Figure 2 shows the XPS spectrum of NVPF/C/rGO nanocomposite. As shown in Figure 2A, there are six elements of Na, V, P, O, F, and C in the XPS full spectra of NVPF/C/rGO. From the high-resolution XPS spectra of V 2p (Figure 2B), the peaks at about 516.8 and 523.7 eV represent the electrons in V 2p3/2 and V 2p1/2, respectively, which are characteristic of V3+ species and consistent well with the previous report (Zhang et al., 2018; Chen et al., 2019). Figures 2C,D are the high-resolution XPS spectrum of Na 1s and P 2p, indicating that exists Na and P elements in material. The high-resolution XPS spectra of O 1s, as shown in Figure 2E, the peaks at about 533.26 and 531.86 eV are assigned to C-O and P-O bonds (Gu et al., 2020). Moreover, as shown in Figure 2F, the C 1s peak of NVPF/C/rGO can be fitted into four peaks at 288.86, 286.21, 285.06, and 284.56 eV, corresponding to O-C=O, C-O, C-C, and C=C bonds (Zhang et al., 2018).
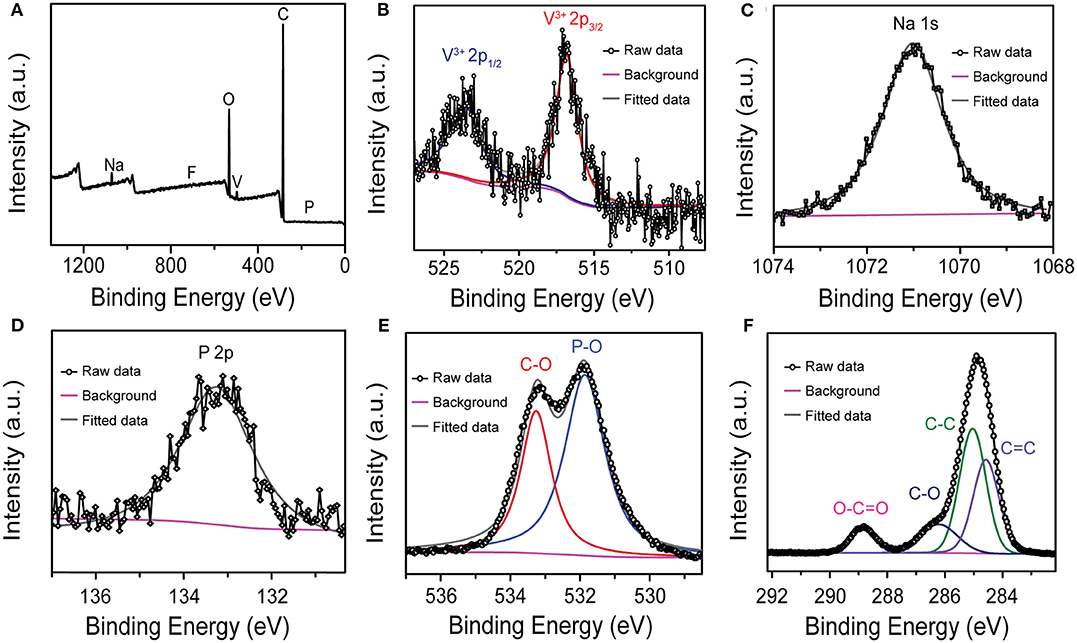
Figure 2. (A) Full XPS spectra of the NVPF/C/rGO; high-resolution (B) V 2p, (C) Na 1s, (D) P 2p, (E) O 1s, and (F) C 1s spectrum of the NVPF/C/rGO.
The morphology of the NVPF/C/rGO nanocomposite was firstly characterized by SEM. In Figures 3a,b, the NVPF/C/rGO is mainly composed of NVPF nanoparticles and the rGO sheets, and the particle size of NVPF material is about 200-500 nm, which is benefit for the electrochemical performance. Figure 3a shows the rGO layers cover on the surface of the NVPF nanocomposite and connect these to form a 3D conductive network, improving the electronic conductivity of the material. And the morphology of the NVPF/C/rGO nanocomposite can be represented by schematic illustration of Figure 3c. Moreover, the element energy dispersive spectrometer (EDS) was implemented to explain the elements distribution of NVPF/C/rGO material, indicating the Na, V, P, O, F elements are uniformly distributed in the NVPF/C/rGO (Figure 3d).
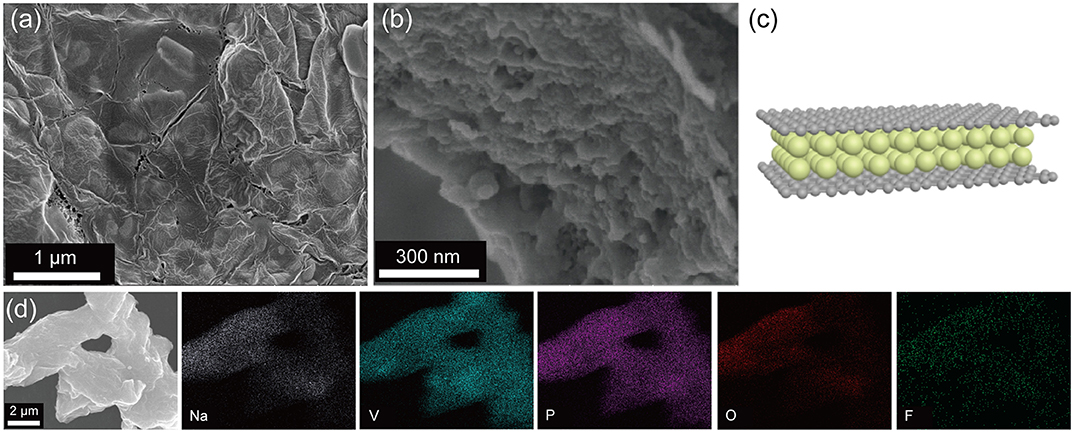
Figure 3. (a,b) SEM images of NVPF/C/rGO under different magnifications; (c) the schematic illustration of NVPF/C/rGO; (d) the EDS mapping of NVPF/C/rGO.
The TEM was implemented to further investigate the morphology of NVPF/C/rGO, as shown in Figure 4a, the prepared material is granular and the particle size is nano-scale, which is consistent well with the results of the SEM. In addition, TEM image of NVPF/C/rGO demonstrates that all the NVPF particles are coated evenly by the amorphous carbon, and the rGO layers cover on the surface of particles and connect these to form a 3D conductive network. In Figure 4b, the high-resolution TEM (HRTEM) image shows the NVPF particle and the carbon layer. Obviously, the NVPF has high crystallinity and the lattice distance is 0.38 nm, corresponding to the (211) plane of monoclinic NaVPO4F.
Used metallic Na plates as both counter and reference electrodes in the half-cells, the CV and galvanostatic charge-discharge (GCD) tests were implemented to investigate the electrochemical performance of the 3D carbon networks constructed NVPF/C/rGO. Figure 5A shows the CV curves, a couple of well-defined anodic/cathodic peaks at about 3.59/3.20 V vs. Na+/Na, which corresponds to reversible two-phase transformation between NaVPO4F and VPO4F, that is, the redox reaction of V4+/V3+ couple. However, at the sodiation process, this single peak splits as two chiseled peaks, which may be attributed to the FEC additive (Guo et al., 2015). The GCD profiles at 0.05 C in Figure 5B exhibit a pair of charge/discharge plateaus at about 3.42/3.34 V vs. Na+/Na, which is agree with CV test results. The charge and discharge capacities at first cycle of NVPF/C/rGO are 122.1 and 108.7 mA h g−1, respectively, and the corresponding Coulombic efficiency is only 89%, which may be caused by the side effects at first cycle due to the large specific surface area of NVPF/C/rGO nanocomposite. Moreover, the capacity of NVPF/C/rGO remains unchanged as the cycle progress, indicating the charge/discharge processes are highly reversible.
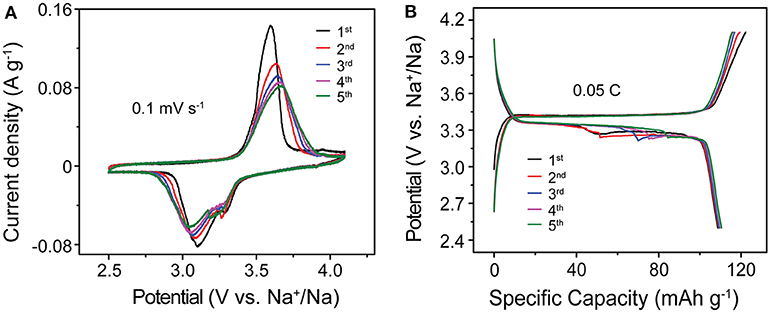
Figure 5. (A) CV curves at the scan rate of 0.1 mV s−1 and (B) galvanostatic charge–discharge profiles at 0.5C of the NVPF/C/rGO.
To further evaluate the electrochemical properties of the 3D carbon networks constructed NVPF/C/rGO, the rate and cycle performance of the cells were studied (Figure 6). Figure 6A exhibits the rate capabilities of NVPF/C/rGO at 0.05-10 C. At low rate of 0.05C, the NVPF/C/rGO nanocomposite delivers a capacity of 106.3 mA h g−1, and at a very high rate of 10 C, it still has specific capacity of about 65.8 mA h g−1, indicating the superior rate capabilities of NVPF/C/rGO nanocomposite. Moreover, when the rate returns to 0.05 C, the specific capacity of NVPF/C/rGO nanocomposite can still restore to 105.8 mA h g−1 after the rate tests of 55 cycles. The corresponding GCD curves are further shown in Figure 6B, and the charge and discharge plateaus are still obvious at 10 C. Furthermore, the NVPF/C/rGO nanocomposite also demonstrates the excellent cycling performance, and shown in Figure 6C. After 200 cycles at 0.05 C, the NVPF/C/rGO nanocomposite still has a capacity of 104.8 mA h g−1 with the capacity retention of above 95 %.
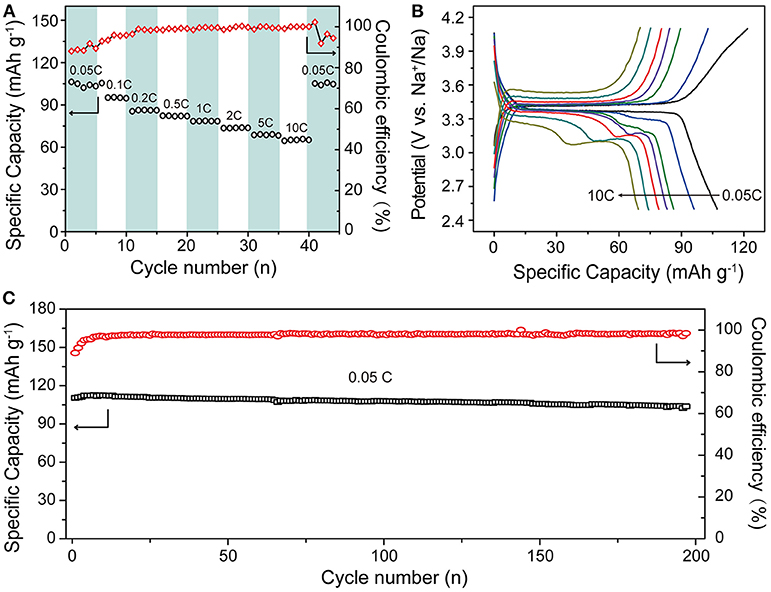
Figure 6. Electrochemical performance of NVPF/C/rGO: (A) rate capability at 0.05–10 C; (B) the corresponding charge–discharge profiles at different rate; and (C) cycling performance.
Combining the results of the above research to further study about the NVPF/C/rGO nanocomposite's kinetics characteristics, in Figure 7, GITT test was performed at a current density of 0.05 C within the potential range of 2.5–4.1 V. By applying a short enough current during the electrode is in a stable state, the voltage response curve of the system could be measured by GITT method to study the kinetics properties of the electrode material. Assuming the electrode system at steady state, Es is potential and τ is duration at the moment. The total change of the cell voltage is Er. MB is the electrode materials' relative molecular mass, and mB is the electrode materials' actual mass. Combining the above specific values and according to the formula (1), the apparent Na diffusion coefficient (Dapp) can be calculated (Guo et al., 2014):
Calculated from the GITT curves, the Dapp values of the cell are in the 10−12~10−8 cm2 s−1 order of magnitude, which is higher than those of NVPF (10−14~10−10 cm2 s−1) and other polyanion-type phosphate cathodes for SIBs (Liu Q. et al., 2015; Wang et al., 2015). 3D carbon networks can effectively improve the kinetics properties of NVPF material. Furthermore, the values at the plateau region are evidently low in both the charge and discharge processes, indicating that the Na intercalation/extraction process of NVPF/C/rGO nanocomposite requires more energy. The results indicate that the NVPF/C/rGO has excellent kinetics properties.
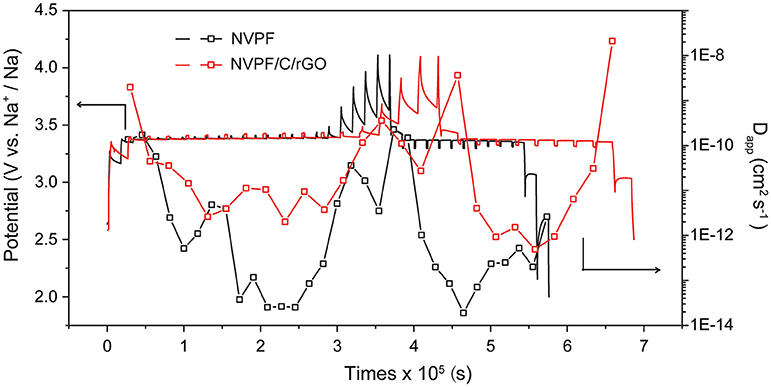
Figure 7. GITT test results containing Dapp and variation curve along with the GCD processes of NVPF/C/rGO and NVPF.
Conclusion
In summary, a 3D carbon networks constructed NVPF/C/rGO nanocomposite is prepared by freeze-drying assisted high-temperature solid-state method. In NVPF/C/rGO, the amorphous carbon is coated on the surface of NVPF particles, and the rGO layers cover on the surface of the NVPF/C particles and connect these to form a 3D conductive network, effectively improving the electronic conductivity of NVPF. The NVPF/C/rGO cathode material for SIBs exhibits remarkably improved electrochemical performance, in the aspect of significantly enhanced specific capacity, great rate capability and outstanding cycling stability. Moreover, the GITT test was implemented to analyse the kinetic properties of NVPF/C/rGO electrode. Therefore, this work provides a facile synthesis method for constructing 3D conductive network modified electrode materials, promoting the development of high-performance SIBs.
Data Availability Statement
All datasets generated for this study are included in the article/supplementary material.
Author Contributions
All authors listed have made a substantial, direct and intellectual contribution to the work, and approved it for publication.
Funding
The authors gratefully acknowledge the financial support from the National Natural Science Foundation of China (91963118), the Fundamental Research Funds for the Central Universities (2412019ZD010), the Project Funded by China Postdoctoral Science Foundation (2019M661187), and National Postdoctoral Program for Innovative Talents (BX20190064).
Conflict of Interest
The authors declare that the research was conducted in the absence of any commercial or financial relationships that could be construed as a potential conflict of interest.
References
Barker, J., Saidi, M. Y., and Swoyer, J. L. (2003). A sodium-ion cell based on the fluorophosphate compound NaVPO4F. Electrochem. Solid State Lett. 6:A1. doi: 10.1149/1.1523691
Barpanda, P., Nishimura, S. I., and Yamada, A. (2012). High-voltage pyrophosphate cathodes. Adv. Energy Mater. 2, 841–859. doi: 10.1002/aenm.201100772
Barpanda, P., Oyama, G., Nishimura, S. I., Chung, S. C., and Yamada, A. (2014). A 3.8-V earth-abundant sodium battery electrode. Nat. Commun. 5:4358. doi: 10.1038/ncomms5358
Che, H., Chen, S., Xie, Y., Wang, H., Amine, K., Liao, X. Z., et al. (2017). Electrolyte design strategies and research progress for room-temperature sodium-ion batteries. Energy Environ. Sci. 10, 1075–1101. doi: 10.1039/C7EE00524E
Chen, C., Li, T., Tian, H., Zou, Y., and Sun, J. (2019). Building highly stable and industrial NaVPO4F/C as bipolar electrodes for high-rate symmetric rechargeable sodium-ion full batteries. J. Mater. Chem. A 7, 18451–18457. doi: 10.1039/C9TA05396D
Dunn, B., Harish, K., and Tarascon, J. M. (2011). Electrical energy storage for the grid: a battery of choices. Science 334, 928–935. doi: 10.1126/science.1212741
Fan, H.-H., Li, H.-H., Huang, K.-C., Fan, C.-Y., Zhang, X.-Y., Wu, X.-L., et al. (2017). Metastable Marcasite-FeS2 as a new anode material for lithium ion batteries: CNFs-improved lithiation/delithiation reversibility and Li-storage properties. ACS Appl. Mater. Interfaces 9, 10708–10716. doi: 10.1021/acsami.7b00578
Fang, Y., Zhang, J., Xiao, L., Ai, X., Cao, Y., and Yang, H. (2017). Phosphate framework electrode materials for sodium ion batteries. Adv. Sci. 4:1600392. doi: 10.1002/advs.201600392
Ge, X., Li, X., Wang, Z., Guo, H., Yan, G., Wu, X., et al. (2019). Facile synthesis of NaVPO4F/C cathode with enhanced interfacial conductivity towards long-cycle and high-rate sodium-ion batteries. Chem. Eng. J. 357, 458–462. doi: 10.1016/j.cej.2018.09.099
Gu, Z.-Y., Guo, J.-Z., Sun, Z.-H., Zhao, X.-X., Li, W.-H., Yang, X., et al. (2020). Carbon-coating-increased working voltage and energy density towards an advanced Na3V2(PO4)2F3@C cathode in sodium-ion batteries. Sci. Bull. 65, 702–710. doi: 10.1016/j.scib.2020.01.018
Guo, J.-Z., Wang, P.-F., Wu, X.-L., Zhang, X.-H., Yan, Q., Chen, H., et al. (2017). High-energy/power and low-temperature cathode for sodium-ion batteries: in situ XRD study and superior full-cell performance. Adv. Mater. 29:1701968. doi: 10.1002/adma.201701968
Guo, J.-Z., Wu, X.-L., Wan, F., Wang, J., Zhang, X.-H., and Wang, S. R.- (2015). A Superior Na3V2(PO4)3-Based nanocornposite enhanced by both N-doped coating carbon and graphene as the cathode for sodium-ion batteries. Chem. Eur. J. 21, 17371–17378. doi: 10.1002/chem.201502583
Guo, S., Liu, P., Yu, H., Zhu, Y., Chen, M., Ishida, M., et al. (2015). A layered P2- and O3-type composite as a high-energy cathode for rechargeable sodium-ion batteries. Angew. Chem. Int. Ed. 54, 5894–5899. doi: 10.1002/anie.201411788
Guo, S., Yu, H., Jian, Z., Liu, P., Zhu, Y., Guo, X., et al. (2014). A high-capacity, low-cost layered sodium manganese oxide material as cathode for sodium-ion batteries. ChemSusChem 7, 2115–2119. doi: 10.1002/cssc.201402138
Hummers, W. S., and Offeman, R. E. (1958). Preparation of Graphitic Oxide. J. Am. Chem. Soc. 80, 1339. doi: 10.1021/ja01539a017
Hwang, J.-Y., S.-,Myung, T., Yoon, C. S., Kim, S.-S., Aurbach, D., and Sun, Y.-K. (2016). Novel cathode materials for Na-Ion batteries composed of spoke-like nanorods of Na[Ni0.61Co0.12Mn0.27]O2 assembled in spherical secondary particles. Adv. Funct. Mater. 26, 8083–8093. doi: 10.1002/adfm.201603439
Jian, Z., Han, W., Lu, X., Yang, H., Hu, Y.-S., Zhou, J., et al. (2013). Superior electrochemical performance and storage mechanism of Na3V2(PO4)3 cathode for room-temperature sodium-ion batteries. Adv. Energy Mater. 3, 156–160. doi: 10.1002/aenm.201200558
Keller, M., Buchholz, D., and Passerini, S. (2016). Layered Na-Ion cathodes with outstanding performance resulting from the synergetic effect of mixed P- and O-type phases. Adv. Energy Mater. 6:1501555. doi: 10.1002/aenm.201501555
Kim, J., Park, I., Kim, H., Park, K.-Y., Park, Y.-U., and Kang, K. (2016). Tailoring a new 4V-class cathode material for Na-Ion batteries. Adv. Energy Mater. 6:1502147. doi: 10.1002/aenm.201502147
Lao, M., Zhang, Y., Luo, W., Yan, Q., Sun, W., Dou, S., et al. (2017). Alloy-based anode materials toward advanced sodium-ion batteries. Adv. Mater. 29:1700622. doi: 10.1002/adma.201700622
Law, M., and Balaya, P. (2018). NaVPO4F with high cycling stability as a promising cathode for sodium-ion battery. Energy Storage Mater. 10, 102–113. doi: 10.1016/j.ensm.2017.08.007
Li, C., Shen, M., Hu, B., Lou, X., Zhang, X., Tong, W., et al. (2018). High-energy nanostructured Na3V2(PO4)2O1.6F1.4 cathodes for sodium-ion batteries and a new insight into their redox chemistry. J. Mater. Chem. A 6, 8340–8348. doi: 10.1039/C8TA00568K
Lim, C. H., Kannan, A. G, Lee, H.-W., and Kim, D. K. (2013). A high power density electrode with ultralow carbon via direct growth of particles on graphene sheets. J. Mater. Chem. A 1, 6183–6190. doi: 10.1039/c3ta10254h
Ling, M., Li, F., Yi, H., Li, X., Hou, G., Zheng, Q., et al. (2018). Superior Na-storage performance of molten-state-blending-synthesized monoclinic NaVPO4F nanoplates for Na-ion batteries. J. Mater. Chem. A 6, 24201–24209. doi: 10.1039/C8TA08842J
Liu, D.-H., Lü, H.-Y., Wu, X.-L., Hou, B.-H., Wan, F., Bao, S.-D., et al. (2015). Constructing the optimal conductive network in MnO-based nanohybrids as high-rate and long-life anode materials for lithium-ion batteries. J. Mater. Chem. A 3, 19738–19746. doi: 10.1039/C5TA03556B
Liu, Q., Wang, D., Yang, X., Chen, N., Wang, C., Bie, X., et al. (2015). Carbon-coated Na3V2(PO4)2F3 nanoparticles embedded in a mesoporous carbon matrix as a potential cathode material for sodium-ion batteries with superior rate capability and long-term cycle life. J. Mater. Chem. A. 3, 21478–85. doi: 10.1039/C5TA05939A
Liu, X., Chao, D., Su, D., Liu, S., Chen, L., Chi, C., et al. (2017). Graphene nanowires anchored to 3D graphene foam via self-assembly for high performance Li and Na ion storage. Nano Energy 37, 108–117. doi: 10.1016/j.nanoen.2017.04.051
Liu, Z.-M., Wang, X.-Y., Wang, Y., Tang, A.-P., Yang, S.-Y., and He, L.-F. (2008). Preparation of NaV1−xAlxPO4F cathode materials for application of sodium-ion battery. Trans. Nonferrous Metals Soc. 18, 346–350. doi: 10.1016/S1003-6326(08)60060-6
Nayak, P. K., Yang, L., Brehm, W., and Adelhelm, P. (2018). From Lithium-ion to sodium-ion batteries: advantages, challenges, and surprises. Angew. Chem. Int. Ed. 57, 102–120. doi: 10.1002/anie.201703772
Pang, W.-L., Zhang, X.-H., Guo, J.-Z., Li, J.-Y., Yan, X., Hou, B. H., et al. (2017). P2-type Na2/3Mn1−xAlxO2 cathode material for sodium-ion batteries: Al-doped enhanced electrochemical properties and studies on the electrode kinetics. J. Power Sources 356, 80–88. doi: 10.1016/j.jpowsour.2017.04.076
Park, Y.-U., Seo, D.-H., Kwon, H. S., Kim, B., Kim, J., Kim, H., et al. (2013). A new high-energy cathode for a na-ion battery with ultrahigh stability. J. Am. Chem. Soc. 135, 13870–13878. doi: 10.1021/ja406016j
Rui, X., Sun, W., Wu, C., Yu, Y., and Yan, Q. (2015). An advanced sodium-ion battery composed of carbon coated Na3V2(PO4)3 in a porous graphene network. Adv. Mater. 27, 6670–6676. doi: 10.1002/adma.201502864
Share, K., Cohn, A. P., Carter, R., Rogers, B., and Pint, C. L. (2016). Role of nitrogen-doped graphene for improved high-capacity potassium ion battery anodes. ACS Nano 10, 9738–9744. doi: 10.1021/acsnano.6b05998
Wang, Q., Zhao, B., Zhang, S., Gao, X., and Deng, C. (2015). Superior sodium intercalation of honeycomb-structured hierarchical porous Na3V2(PO4)3/C microballs prepared by a facile one-pot synthesis. J. Mater. Chem. A 3, 7732–7740. doi: 10.1039/C5TA00765H
Yan, X., Ye, H., Wu, X.-L., Zheng, Y.-P., Wan, F., Liu, M., et al. (2017). Three-dimensional carbon nanotube networks enhanced sodium trimesic: a new anode material for sodium ion batteries and Na-storage mechanism revealed by ex situ studies. J. Mater. Chem. A 5, 16622–16629. doi: 10.1039/C7TA03484A
Yang, X., Wang, Y.-Y., Hou, B.-H., Liang, H.-J., Zhao, X.-X., Fan, H., et al. (2020). Nano-SnO2 Decorated Carbon Cloth as Flexible, Self-supporting and Additive-Free Anode for Sodium/Lithium-Ion Batteries. Acta Metallurgica Sinica. doi: 10.1007/s40195-020-01001-7
Yin, Y., Xiong, F., Pei, C., Xu, Y., An, Q., Tan, S., et al. (2017). Robust three-dimensional graphene skeleton encapsulated Na3V2O2(PO4)2F nanoparticles as a high-rate and long-life cathode of sodium-ion batteries. Nano Energy 41, 452–459. doi: 10.1016/j.nanoen.2017.09.056
Keywords: sodium-ion batteries, cathode, NaVPO4F, 3D carbon networks, nanocomposite
Citation: Guo J-Z, Yang A-B, Zhao X-X, Gu Z-Y and Wu X-L (2020) 3D Carbon Networks Constructed NaVPO4F/C/rGO as a Cathode Material for High-Performance Sodium-Ion Batteries. Front. Energy Res. 8:64. doi: 10.3389/fenrg.2020.00064
Received: 26 February 2020; Accepted: 01 April 2020;
Published: 23 April 2020.
Edited by:
Tianyu Liu, Virginia Tech, United StatesReviewed by:
Wei Luo, Tongji University, ChinaTeng Zhai, Nanjing University of Science and Technology, China
Copyright © 2020 Guo, Yang, Zhao, Gu and Wu. This is an open-access article distributed under the terms of the Creative Commons Attribution License (CC BY). The use, distribution or reproduction in other forums is permitted, provided the original author(s) and the copyright owner(s) are credited and that the original publication in this journal is cited, in accordance with accepted academic practice. No use, distribution or reproduction is permitted which does not comply with these terms.
*Correspondence: Jin-Zhi Guo, Z3VvanowNjUmI3gwMDA0MDtuZW51LmVkdS5jbg==; Xing-Long Wu, eGluZ2xvbmcmI3gwMDA0MDtuZW51LmVkdS5jbg==