- Scion, Te Papa Tipu Innovation Park, Rotorua, New Zealand
This mini-review considers the densification of biomass blended with plastic wastes as an approach for waste management and sustainable fuel production from two perspectives; (1) We overviewed the pollutants generated during plastics combustion and their hazards. The control of these pollutants can be achieved as both reported in literature and by currently in-service municipal waste plants. (2) Advantages from densifying biomass/plastic blends as a solid fuel are indicated. Biomass/plastic briquettes or pellets are a potentially promising solid fuel with low costs, high volumetric heating values, high resistance to mechanical damage, and good durability performance under humid conditions. Moreover, the combustion of biomass/plastic blends with <10% plastics has no substantial negative effect on pollutants emission compared with that of biomass. Perspectives on densifying biomass/plastic blends as a solid fuel are proposed to realize the scale-up of this technique.
Introduction
The cascading use of biomass to achieve a circular bioeconomy has been considered as a sustainable solution for an environmental friendly world (Patermann and Aguilar, 2018). Producing bioenergy and biofuels to replace fossil fuels has been widely considered as an option of cascading biorefinery (Gelfand et al., 2013; Guo et al., 2015; Bose et al., 2020; Ding et al., 2020). Compared with traditional fuels such as coal, natural gas and petroleum, biomass fuel is a carbon-neutral, sustainable alternative that has manageable pollutant emissions (Gao and Wu, 2011; Van Loo and Koppejan, 2012; Gao et al., 2017). However, the low density (e.g., ~0.6 kg/L for wood), high moisture absorption, and comparatively low heating values of biomass (gross calorific values at 15.9–20.3 MJ/kg) limit the transportation, conversion, and combustion of biomass as solid fuels (Friedl et al., 2005). To enhance both the volumetric density and energy density of biomass, densification such as briquetting and pelletizing is required (Mostafa et al., 2019). Previous studies on biomass briquetting have increased the density of densified biomass to >1.1 kg/L by optimizing the densification pressure, temperature and moisture content (Li et al., 2015; Wongsiriamnuay and Tippayawong, 2015; Kudo et al., 2019).
To transport, store, and use densified biomass as fuel, the resistance of biomass briquettes or pellets to mechanical damage and durability under humid storage conditions are of critical importance. Binders such as bentonite, starch, glycerine, and lignin have been recommended to enhance the durability of densified biomass (Kaliyan and Morey, 2010; Yahaya and Ibrahim, 2012; Sakkampang and Wongwuttanasatian, 2014). However, the use of these binders does not show significant improvement in the energy density of biomass briquettes or pellets, due to the low heating values of these materials. Thus, binders with higher calorific values are suggested to be used.
Plastics are one of the major solid wastes that need to be treated properly both for waste management to reduce their environmental effect and for the valorisation of wastes with high organic contents (Subramanian, 2000). There are different chemical forms of plastic wastes (e.g., PE, PP, PVC, PA, PS, and PET) with distinct physicochemical properties and compositions (Table S1). Considering the superior heating values of some plastic wastes (e.g., 41.80 and 30.90 MJ/kg for PE and PP, respectively, compared with ~20–21 MJ/kg for sub-bituminous thermal coal ~28 MJ/kg for bituminous coal and 19.2 MJ/kg for oven dry softwood) (Eng et al., 2008), the combustion of plastics is regarded as a promising way to partially replace the use of fossil fuels; while disposal of plastics into landfills implies an irreversible loss of valuable raw materials and energy. Moreover, the excellent flowability, tensile strength, and hydrophobicity of plastics suggest that they are ideal binders for biomass and coal densification (Shenoy et al., 1983; Chang et al., 2012; Chen et al., 2018). The co-densification of biomass and plastic wastes can be considered as a step closer to a circular bioeconomy that can achieve a reduction of over 80% (assuming 90% wood and 10% plastics) of greenhouse gas emission compared with the use of coal (Eriksson and Finnveden, 2009). Meanwhile, combusting plastic wastes as a fuel can also achieve the rapid degradation of these non-biodegradable polymers, rather than degradation in the environment with long-lasting pollution (Chamas et al., 2020).
However, the “life-threatening” pollution associated with plastic wastes combustion has been widely considered as a problem, which is why plastic combustion is criticized in many areas. To address worldwide concerns about the pollution caused by the combustion of plastic wastes and municipal solid wastes containing plastics, thus achieving the production of biomass/plastic solid fuel with high heating values, this mini-review: firstly, briefly describes the pollutants generated during plastics combustion and the corresponding techniques used for pollution control; secondly, highlights densified biomass/plastic blends as a sustainable solid fuel based on its advantages; and thirdly, proposes some perspectives on future work.
Pollution From the Combustion of Plastics and Wastes Containing Plastics
Major Pollutants and Their Hazards
Multiple techniques have been used to find the best use of plastic wastes. The direct combustion of high heating value plastics (PP, PE, PS) or solid wastes containing plastics has been widely studied as an effective approach for waste plastic management (Wasilewski and Siudyga, 2013). However, the combustion of plastic generates multiple pollutants including gases, particulates (or airborne particles), and solid residues (ash). The detailed chemical composition of these pollutants is correlated to various factors including the composition of plastics and combustion conditions (e.g., temperature, air flow, and time). In general, the following pollutants shown in Figure 1 were identified based on the representative studies listed in Table S2.
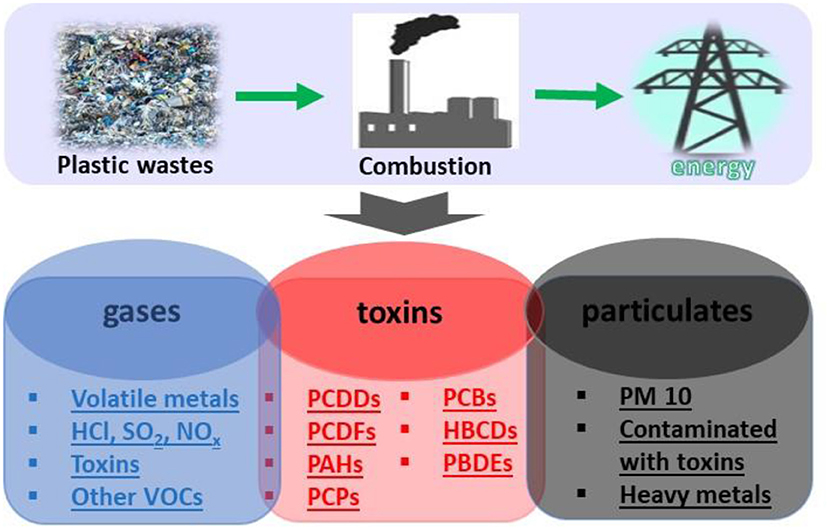
Figure 1. Major pollutants generated during the incineration of waste plastics. Chemicals and abbreviations: PCDDs, Polycholorinated dibenzo-p-dioxins; PCDFs, Dibenzofurans; PAHs, Polycyclic aromatic hydrocarbons; PCPs, Pentachlorophenols; PCBs, Polychlorobenzenes; HBCDs, hexabromocyclodedecanes; PBDEs, polybrominated diphenyl ethers.
Gases
Acid gases are some of the major gaseous pollutants generated from the combustion of various feedstocks including coal, gasoline, as well as plastics. Major acid gases including HCl, SO2, and even HF or HCN were previously reported as pollutants produced from plastic combustion (Werther, 2007). Other gaseous pollutants such as NOx (i.e., NO, NO2) and CO can also be produced depending on the combustion conditions (Takasuga et al., 2003; Werther, 2007). The acid gases generated from combustion are responsible for acid rain, which causes severe effects to soil, plants, buildings, animals, and human beings. The gases NOx and CO are poisonous to human beings and animals, these chemicals are also greenhouse gases, and serve as catalysts for the degradation of ozone when transferred to the Ozone layer (De Nevers, 2010).
Particulates
The combustion of plastics (or any organic matter) also generates particulates with various chemical compositions and particle sizes. The particulates, especially PM2.5 and PM10, can directly enter the lungs of human beings and animals and cause breathing problems (Xing et al., 2016). The emission of particles also causes other complex problems such as reducing visibility and severing the greenhouse effect (De Nevers, 2010; Feng et al., 2018). Moreover, particulates contaminated with toxic chemicals produced during plastic combustion can raise further concerns.
Toxins
The generation of various toxins (Table S2) from plastics combustion is one of the major reasons that the combustion of plastics is banned in many areas. These chemicals are recalcitrant, toxic and unbiodegradable (Yasuhara et al., 2005). Many hazards are associated with toxins generated during plastics combustion. For instance, volatiles from polystyrene (PS) combustion are harmful to the central nervous system. Open-air burning of PS leads to severe health risks such as heart diseases, aggravates respiratory ailments such as asthma and emphysema, and causes rashes, nausea or headaches, damages the nervous system, kidney or liver, and the reproductive and development system. Dioxins settle on crops and in our waterways where they eventually enter our food-chain and get into human body. Polycyclic aromatic hydrocarbons (PAHs) and polychlorinated dibenzofurans (PCDFs) are also common toxins generated from plastic combustion. These chemicals can go into the human body via the food-chain or by inhalation, thus causing various diseases (Valavanidis et al., 2008; Verma et al., 2016).
Moreover, because of the widely use of metal-containing additives during the production of plastic products, there are also risks of producing metal pollutants (Dimitrakakis et al., 2009). Metal pollutants, in particulates or fly ash (e.g., Cd, Pb, and Cu), gases (e.g., Hg), and solid residues (ash and clinker), are also produced during the combustion of plastic wastes (Dimitrakakis et al., 2009). These metals mainly exist in the solid residue in the form of water-soluble salts (~40%), which are not allowed to go to direct disposal (Forsgren, 2019) in land fill because the leaching of these salts pollutes the underground water. Mono-filling and separate management of the ash generated during municipal solid wastes (MSW) combustion is required (Forsgren, 2019). The hazards of these metals and corresponding salts are correlated to the specific chemical compositions. For instance, heavy metals present in wastes can be volatilized during the combustion process, and then condense in flue gas cooling processes to form sub-micrometer metal particulates with the size ranging from 0.5 to 10 nm. If inhaled, these metal particles can cause significant respiratory problems (Chen and Yang, 1998).
Techniques Applied for Controlling Pollutants During the Combustion of Plastics and Wastes Containing Plastics
The combustion of plastics produces pollutants in various ways, but there are also opportunities to incinerate them under the conditions where negligible pollution is caused. There are emission regulations which refer to the air quality standards of various countries and areas. The revised National Environmental standards for Air Quality (2004) of New Zealand has given details of the emission standards of particulates, gases and volatile organic carbons (VOCs). More detailed emission limits for toxins can refer to the EU waste gas emission standards, where detailed chemicals including metals, gases, and toxins are listed (Werther, 2007; Broadbent et al., 2010). In general, the environmental and health effects of plastic combustion should not be exaggerated, given that the emissions from the process can be controlled to lower than the prescribed levels in the environmental standards.
Some of the techniques for controlling the emissions from plastic combustion are described in Table S3. As shown, it is possible to control the emission of pollutants during plastic combustion with appropriate controlling techniques. Precipitation methods including gravitational precipitation, filtration, cyclone, electrostatic precipitators (ESPs) and filters/baghouses are highlighted as methods to suppress the emission of particulates because these techniques are widely used and suitable for combustion derived emissions (MacKenna and Turner, 1989; De Nevers, 2010). Absorption with porous materials is widely used in industry for the control of dioxins from sources with high gas flows (Andersson and Lindgren, 2006; Liu et al., 2014). Catalytic combustion is recommended to minimize the emission of various organic toxins, because these chemicals are poisonous and most of them have recalcitrant structures and cannot be decomposed under moderate conditions. Therefore, it would be better to eliminate or minimize their formation during the combustion process (Zhao and Wang, 2018). In-situ combustion under on-site temperatures with high efficiencies is desirable and can be achieved with the use of appropriate catalysts (Wang and Zhao, 2016). Moreover, gaseous pollutants such as acids SO2, and NOx can be controlled via water/chemical capture methods as these gases are highly soluble and can be easily captured via chemical reactions under moderate conditions (Ghosh-Dastidar et al., 1996; Gunter et al., 2000; Vosteen et al., 2005). In addition, biological techniques such as biofilters, composting, and anaerobic digestion are also capable of controlling various gaseous pollutants (VOCs) and/or organic compounds in aqueous phases (Verma et al., 2006; Hough et al., 2010; Gopinath et al., 2018; Wu et al., 2018). The heavy metals, generally exists as water-soluble salts in the residue, can be recycled via chemical precipitation (Forsgren, 2019).
With the employment of appropriate combustion techniques, waste-to-energy projects incinerating municipal wastes (containing plastics) as fuels have been widely accepted in European countries and there are many plants distributed in urban areas such as Vienna (Spittelau's Incineration Plant), Munich (WtE plant Munich North), Berlin, Greater Copenhagen, Zurich, Amsterdam (Waste-fired Power Plant), Brescia (Bresica WtE Plant), Barcelona (Tersa WtE Plant), and Mallorca (Son Reus WtE plant) (Chaliki et al., 2014).
Advantages of Biomass/Plastic Blends as a Fuel
The combustion of plastic wastes generates various pollutants and these pollutants can be controlled via proper techniques as discussed above. Thus, combusting biomass/plastics with a majority amount of biomass is unlikely causing more pollutants than the singular combustion of plastics. Previous studies have investigated the emission of pollutants during the co-combustion of plastics and other feedstocks such as coal, coke and biomass, suggesting that the pollutants emission was not enhanced compared with the combustion of singular feedstocks. For instance, compared with the combustion of coal, the addition of 20% plastic wastes containing municipal wastes showed no considerable enhancement on pollutants emission (Frankenhaeuser et al., 1994). Combustion of straw/LDPE (90/10) pellets showed less emission of ash content compared with that of straw pellets (Emadi et al., 2017). However, compared with the combustion of biomass and biomass/PE, the combustion of biomass/PET had shown more emission of PAHs and particulates, suggesting that the choose of plastic types is of critical importance (Tomsej et al., 2018). Current studies at least suggest that the addition of PE at <10% is unlikely causing more pollutant emission. Moreover, some of the previous studies have demonstrated the advantages of using plastics as both a co-fuel for municipal wastes and as a binder for coal or biomass. We identify three advantages of utilizing biomass/plastic blends as a fuel based on the recent studies listed in Table 1.
First, co-combustion of plastics and biomass is preferred to simplify the separation of municipal waste and save process costs. In industrial waste-to-energy plants, plastics are generally co-incinerated with other feedstocks (mainly lignocellulosic biomass or its derived products) (Chaliki et al., 2014). In general, the major composition of municipal wastes are plastics (~4–23%) and biomass derived wastes (~15–68%) such as paper and wood. Direct co-combustion means saving the cost used for pre-separation (Karak et al., 2012).
Second, densification of biomass/plastic blends can produce a solid fuel with higher volumetric heating values than lignocellulosic biomass. Many plastic wastes (e.g., LDPE, PP, PE) have high heating values (>30 MJ/kg) as shown in Table S1, while that of biomass is essentially lower (<20 MJ/kg). Moreover, because of the low bulk density of both plastics and biomass, their volumetric energy densities are not suitable for direct combustion. In contrast, after densification of biomass with plastics such as 10% LDPE, studies have demonstrated that the volumetric heating values of biomass/plastic were significantly enhanced to ~18 MJ/L, which is comparable to bituminous coal (Simoneit et al., 2005; Qin et al., 2016; Wang and Zhao, 2016). Moreover, plastics have high volatile contents and low ignition points that can promote the combustion efficiencies of biomass/plastic blends (Sahajwalla et al., 2009).
Third, using plastics as binders enhances the durability of biomass/plastic briquettes or pellets to both mechanical damage and humidity. Plastics are primarily used as binders in the coal industry. The addition of plastics in coal briquettes can strengthen the physical durability by taking advantage of the tensile physical properties of plastics (Massaro et al., 2014; Emadi et al., 2017; Tomsej et al., 2018). Recent studies on densification of biomass/plastic indicated that densified biomass/plastic briquettes or pellets have higher hydrophobicity and physical strength than biomass briquettes or pellets, thus benefiting the transportation, storage, and combustion of biomass as a solid fuel (Bhoumick et al., 2016; Tomsej et al., 2018).
Perspectives on Preparing Biomass/Plastic Solid Fuels
Based on the above review, using plastics and biomass mixtures as solid fuels has emerged as a promising approach for plastics waste management and sustainable use of forestry wastes. To produce densified biomass/plastic solid fuels, the following perspectives are proposed.
Current coal boiler infrastructure requires fuels with volumetric energy densities of 18–22 GJ per m3. This limits the use of many biomass based solid fuels in these boilers. Densification is suggested as a means to convert biomass/plastic into briquettes or pellets with volumetric energy density comparable to coal, because both biomass and plastic wastes have low volumetric density, which is not suitable for transportation, storage and combustion as fuels.
Methodologies for densifying biomass/plastic blends should be investigated and developed. Parameters such as the blend ratios of biomass and plastic feedstocks, moisture content, densification pressure, and densification temperature should be optimized.
The selection of appropriate plastic waste types should also be considered. Plastics such as PVC and PS should always be excluded from combustion due to their chemical compositions and emissions generated during combustion. Also, the high melting points of PET (>250°C) and PS (~240°C) make these materials less suitable for densification via briquetting or pelletizing. Moreover, plastics such as PET have a viable recycling route available, and these materials should be reused. Other plastic wastes such as PP and LDPE can be used as binders in biomass densification.
The mixing and binding mechanisms of biomass/plastic blends during their densification should be researched in order to optimize the mixing ratios of biomass/plastic and improve the durability of produced briquettes or pellets to both mechanical damage and humid condition.
Both laboratory-scale and pilot-scale combustion tests are required to determine the emission of pollutants during the combustion of densified biomass/plastic fuels, to identify the optimal control techniques. Also, an overall techno-economic assessment of densifying plastic wastes and biomass as a fuel is required.
Conclusion
Pollutants will inevitably be produced during the combustion of plastic wastes, as with any waste, and many of them are toxic. However, given proper control techniques, the combustion of plastics and wastes containing plastics is viable as a way for both waste management and energy production. Densifying biomass/plastic with certain plastics such as polyethylene at ~10% has shown no increase in pollutant emissions but significant improvement in the physiochemical properties of biomass/plastic briquettes or pellets. To achieve the use of biomass/plastic as a solid fuel, more efforts on studying the fundamentals of biomass/plastic densification and the combustion of densified biomass/plastic as a fuel are encouraged.
Author Contributions
All authors listed have made a substantial, direct and intellectual contribution to the work, and approved it for publication.
Funding
This study was supported by the Ministry of Business, Innovation, and Employment, through the Crown Research Institute (CRI) Strategic Science Investment Fund.
Conflict of Interest
The authors declare no competing financial interest.
Acknowledgments
The authors acknowledge the helpful comments and edits from Suren Wijeyekoon, Katharine Challis, Paul Bennett, Gregor MacDonald, and Elspeth MacRae.
Supplementary Material
The Supplementary Material for this article can be found online at: https://www.frontiersin.org/articles/10.3389/fenrg.2020.00058/full#supplementary-material
References
Andersson, S., and Lindgren, P. (2006). Air pollution—wet, semi-wet or dry Adiox absorber technology targets dioxin removal. Filtr. Separat. 43, 30–30. doi: 10.1016/S0015-1882(06)70919-3
Bhoumick, M. C., Sarker, N. C., Hasan, M. M., and Roy, B. K. (2016). Conversion of waste plastic into solid briquette in combination with biomass: Bangladesh perspective. Int. Adv. Res. J. Sci. Eng. Technol. 3, 142–146. doi: 10.17148/IARJSET.2016.3332
Boavida, D., Abelha, P., Gulyurtlu, I., and Cabrita, I. (2003). Co-combustion of coal and non-recyclable paper and plastic waste in a fluidised bed reactor. Fuel, 82, 1931–1938. doi: 10.1016/S0016-2361(03)00151-0
Bose, A., O'Shea, R., Lin, R., and Murphy, J. D. (2020). A perspective on novel cascading algal biomethane biorefinery systems. Bioresour. Technol. 304:123027. doi: 10.1016/j.biortech.2020.123027
Broadbent, A., Cullen, N., and Zawar-Reza, P. (2010). Wintertime numerical modelling of PM10 air pollution in Milton, Otago, New Zealand: boundary layer structure, effects of data assimilation, and reaching national environmental standards. Air Qual. Clim. Change, 44:22. Available online at: https://search.informit.com.au/documentSummary;dn=624224151734979;res=IELNZC
Chaliki, P., Psomopoulos, C., Themelis, N., and Stavroulakis, C. (2014). WTE plants installed in 10 European Cities. 12th International Conference on Protection and Restoration of the Environment (Skiathos Island). pp. 493–500.
Chamas, A., Moon, H., Zheng, J., Qiu, Y., Tabassum, T., Jang, J. H., et al. (2020). Degradation rates of plastics in the environment. ACS Sus. Chem. Eng. 8, 3494–3511. doi: 10.1021/acssuschemeng.9b06635
Chang, C., Oyang, T., Hwang, F., Chen, C., and Cheng, L. (2012). Preparation of polymer/silica hybrid hard coatings with enhanced hydrophobicity on plastic substrates. J. Non-Cryst. Solids 358, 72–76. doi: 10.1016/j.jnoncrysol.2011.08.024
Chen, C., and Yang, W. (1998). Metal volatility during plastic combustion. J. Environ. Sci. Heal. A 33, 783–799. doi: 10.1080/10934529809376762
Chen, S., Zhang, L., Zhang, G., Zhong, G., Li, J., Zhang, X., et al. (2018). An investigation and comparison of the blending of LDPE and PP with different intrinsic viscosities of PET. Polymers 10:147. doi: 10.3390/polym10020147
Dimitrakakis, E., Janz, A., Bilitewski, B., and Gidarakos, E. (2009). Determination of heavy metals and halogens in plastics from electric and electronic waste. Waste Manag. 29, 2700–2706. doi: 10.1016/j.wasman.2009.05.020
Ding, L., Cheng, J., Lin, R., Deng, C., Zhou, J., and Murphy, J. D. (2020). Improving biohydrogen and biomethane co-production via two-stage dark fermentation and anaerobic digestion of the pretreated seaweed Laminaria digitata. J. Clean. Prod. 251:119666. doi: 10.1016/j.jclepro.2019.119666
Distler, T., and Sitzmann, W. (2018). An investigation on additives for pelletizing highly torrefied biomass. Biofuels Bioprod. Bior. 12, 958–965. doi: 10.1002/bbb.1919
Emadi, B., Iroba, K. L., and Tabil, L. G. (2017). Effect of polymer plastic binder on mechanical, storage and combustion characteristics of torrefied and pelletized herbaceous biomass. Appl. Energy, 198, 312–319. doi: 10.1016/j.apenergy.2016.12.027
Eng, G., Bywater, I., and Hendtlass, C. (2008). New Zealand Energy Information Handbook. Christchurch: New Zealand Centre for Advanced Engineering.
Eriksson, O., and Finnveden, G. (2009). Plastic waste as a fuel-CO 2-neutral or not? Energy Environ. Sci. 2, 907–914. doi: 10.1039/b908135f
Feng, C., Zhang, M., and Wu, H. (2018). Trace elements in various individual and mixed biofuels: abundance and release in particulate matter during combustion. Energy Fuels 32, 5978–5989. doi: 10.1021/acs.energyfuels.8b00503
Forsgren, C. (2019). Recycling experiences from Scandinavia, focus on ash from waste incineration and plastics. AIP Conference Proceedings. Brisbane, QLD: AIP Publishing. pp. 020062. doi: 10.1063/1.5117122
Frankenhaeuser, M., Hiltunen, M., Manninen, H., Palonen, J., Ruuskanen, J., and Vartiainen, T. (1994). Emissions from co-combustion of used packaging with peat and coal. Chemosphere 29, 2057–2066. doi: 10.1016/0045-6535(94)90373-5
Friedl, A., Padouvas, E., Rotter, H., and Varmuza, K. (2005). Prediction of heating values of biomass fuel from elemental composition. Anal. Chimi. Acta 544, 191–198. doi: 10.1016/j.aca.2005.01.041
Gao, X., Rahim, M. U., Chen, X., and Wu, H. (2017). Inorganic PM10 emission from the combustion of individual mallee components and whole-tree biomass. P. Combust. Inst. 36, 3313–3319. doi: 10.1016/j.proci.2016.08.072
Gao, X., and Wu, H. (2011). Combustion of volatiles produced in situ from the fast pyrolysis of woody biomass: direct evidence on its substantial contribution to submicrometer particle (PM1) emission. Energy Fuels 25, 4172–4181. doi: 10.1021/ef2008216
Gelfand, I., Sahajpal, R., Zhang, X., Izaurralde, R. C., Gross, K. L., and Robertson, G. P. (2013). Sustainable bioenergy production from marginal lands in the US Midwest. Nature 493, 514–517. doi: 10.1038/nature11811
Ghosh-Dastidar, A., Mahuli, S. K., Agnihotri, R., and Fan, L.-S. (1996). Investigation of high-reactivity calcium carbonate sorbent for enhanced SO2 capture. Ind. Eng. Chem. Res. 35, 598–606. doi: 10.1021/ie950342r
Gopinath, M., Pulla, R. H., Rajmohan, K., Vijay, P., Muthukumaran, C., and Gurunathan, B. (2018). “Bioremediation of volatile organic compounds in biofilters,” in Bioremediation: Applications for Environmental Protection and Management, eds S. Varjani, A. Agarwal, E. Gnansounou, and B. Gurunathan (Singapore: Springer), 301−330. doi: 10.1007/978-981-10-7485-1_15
Gunter, W., Perkins, E., and Hutcheon, I. (2000). Aquifer disposal of acid gases: modelling of water–rock reactions for trapping of acid wastes. Appl. Geochem. 15, 1085–1095. doi: 10.1016/S0883-2927(99)00111-0
Guo, M., Song, W., and Buhain, J. (2015). Bioenergy and biofuels: history, status, and perspective. Renew. Sust. Energy Rev. 42, 712–725. doi: 10.1016/j.rser.2014.10.013
Hough, R. L., Crews, C., White, D., Driffield, M., Campbell, C. D., and Maltin, C. (2010). Degradation of yew, ragwort and rhododendron toxins during composting. Sci. Total Environ. 408, 4128–4137. doi: 10.1016/j.scitotenv.2010.05.024
Kaliyan, N., and Morey, R. V. (2010). Natural binders and solid bridge type binding mechanisms in briquettes and pellets made from corn stover and switchgrass. Bioresour. Technol. 101, 1082–1090. doi: 10.1016/j.biortech.2009.08.064
Karak, T., Bhagat, R., and Bhattacharyya, P. (2012). Municipal solid waste generation, composition, and management: the world scenario. Crit. Rev. Env. Sci. Tech. 42, 1509–1630. doi: 10.1080/10643389.2011.569871
Kudo, S., Okada, J., Ikeda, S., Yoshida, T., Asano, S., and Hayashi, J.-,i. (2019). Improvement of pelletability of woody biomass by torrefaction under pressurized steam. Energy Fuels 33, 11253–11262. doi: 10.1021/acs.energyfuels.9b02939
Li, H., Jiang, L.-B., Li, C., Liang, J., Yuan, X., Xiao, Z., et al. (2015). Co-pelletization of sewage sludge and biomass: the energy input and properties of pellets. Fuel Process. Technol. 132, 55–61. doi: 10.1016/j.fuproc.2014.12.020
Liu, X., Qin, X., Chen, L., and Sun, F. (2015). CO2 emission optimization for a blast furnace considering plastic injection. Int. J. Energy Env. 6:175. Available online at: https://www.ijee.ieefoundation.org/vol6/issue2/IJEE_07_v6n2.pdf
Liu, Z., Li, W., and Hung, M. (2014). Simultaneous removal of sulfur dioxide and polycyclic aromatic hydrocarbons from incineration flue gas using activated carbon fibers. J. Air Waste Manag. 64, 1038–1044. doi: 10.1080/10962247.2014.922519
MacKenna, J. D., and Turner, J. H. (1989). Fabric Filter-Baghouses: Theory, Design, and Selection. Roanoke, VA: ETS.
Massaro, M., Son, S., and Groven, L. (2014). Mechanical, pyrolysis, and combustion characterization of briquetted coal fines with municipal solid waste plastic (MSW) binders. Fuel 115, 62–69. doi: 10.1016/j.fuel.2013.06.043
Mostafa, M. E., Hu, S., Wang, Y., Su, S., Hu, X., Elsayed, S. A., et al. (2019). The significance of pelletization operating conditions: an analysis of physical and mechanical characteristics as well as energy consumption of biomass pellets. Renew. Sust. Energy Rev. 105, 332–348. doi: 10.1016/j.rser.2019.01.053
Patermann, C., and Aguilar, A. (2018). The origins of the bioeconomy in the European Union. New Biotechnol. 40, 20–24. doi: 10.1016/j.nbt.2017.04.002
Qin, L., Han, J., Chen, W., Yao, X., Tadaaki, S., and Kim, H. (2016). Enhanced combustion efficiency and reduced pollutant emission in a fluidized bed combustor by using porous alumina bed materials. Appl. Therm. Eng. 94, 813–818. doi: 10.1016/j.applthermaleng.2015.10.153
Sahajwalla, V., Zaharia, M., Kongkarat, S., Khanna, R., Saha-Chaudhury, N., and O'Kane, P. (2009). Recycling plastics as a resource for electric arc furnace (EAF) steelmaking: combustion and structural transformations of metallurgical coke and plastic blends. Energy Fuels 24, 379–391. doi: 10.1021/ef900875r
Sakkampang, C., and Wongwuttanasatian, T. (2014). Study of ratio of energy consumption and gained energy during briquetting process for glycerin-biomass briquette fuel. Fuel 115, 186–189. doi: 10.1016/j.fuel.2013.07.023
Shenoy, A., Chattopadhyay, S., and Nadkarni, V. (1983). From melt flow index to rheogram. Rheol. Acta 22, 90–101. doi: 10.1007/BF01679833
Simoneit, B. R., Medeiros, P. M., and Didyk, B. M. (2005). Combustion products of plastics as indicators for refuse burning in the atmosphere. Environ. Sci. Technol. 39, 6961–6970. doi: 10.1021/es050767x
Subramanian, P. (2000). Plastics recycling and waste management in the US. Resour. Conserv. Recy. 28, 253–263. doi: 10.1016/S0921-3449(99)00049-X
Takasuga, T., Makino, T., Umetsu, N., and Senthilkumar, K. (2003). Quantitative analysis of toxic compounds formed from combustion of some plastic materials and newspaper. Organohalogen Compd. 63, 86–89. Available online at: http://ww.burnbarrel.org/Science/Dioxin2003_papers/TTakasuga424.pdf
Tomsej, T., Horak, J., Tomsejova, S., Krpec, K., Klanova, J., Dej, M., et al. (2018). The impact of co-combustion of polyethylene plastics and wood in a small residential boiler on emissions of gaseous pollutants, particulate matter, PAHs and 1,3,5-triphenylbenzene. Chemosphere 196, 18–24. doi: 10.1016/j.chemosphere.2017.12.127
Valavanidis, A., Iliopoulos, N., Gotsis, G., and Fiotakis, K. (2008). Persistent free radicals, heavy metals and PAHs generated in particulate soot emissions and residue ash from controlled combustion of common types of plastic. J. Hazard. Mater. 156, 277–284. doi: 10.1016/j.jhazmat.2007.12.019
Van Loo, S., and Koppejan, J. (2012). The Handbook of Biomass Combustion and Co-firing. London, UK: Earthscan.
Verma, M., Brar, S., Blais, J., Tyagi, R., and Surampalli, R. (2006). Aerobic biofiltration processes—advances in wastewater treatment. Pract. Period. Hazard. Toxic, Radioact. Waste Manag. 10, 264–276. doi: 10.1061/(ASCE)1090-025X(2006)10:4(264)
Verma, R., Vinoda, K., Papireddy, M., and Gowda, A. (2016). Toxic pollutants from plastic waste-a review. Procedia Environ. Sci. 35, 701–708. doi: 10.1016/j.proenv.2016.07.069
Vosteen, B., Beyer, J., Bonkhofer, T.-G., Fleth, O., Wieland, A., Pohontsch, A., Kanefke, R., Standau, E., Mueller, C., and Nolte, M. (2005). Process for Removing Mercury From Flue Gases. Google Patents.
Wang, J., and Zhao, H. (2016). Application of CaO-decorated iron ore for inhibiting chlorobenzene during in situ gasification chemical looping combustion of plastic waste. Energy Fuels 30, 5999–6008. doi: 10.1021/acs.energyfuels.6b01102
Wasilewski, R., and Siudyga, T. (2013). Energy recovery from waste plastics. Chemik 67:435. Available online at: https://journals.indexcopernicus.com/search/article?articleId=544027
Werther, J. (2007). Gaseous emissions from waste combustion. J. Hazard. Mater. 144, 604–613. doi: 10.1016/j.jhazmat.2007.01.116
Wongsiriamnuay, T., and Tippayawong, N. (2015). Effect of densification parameters on the properties of maize residue pellets. Biosyst. Eng. 139, 111–120. doi: 10.1016/j.biosystemseng.2015.08.009
Wu, H., Yan, H., Quan, Y., Zhao, H., Jiang, N., and Yin, C. (2018). Recent progress and perspectives in biotrickling filters for VOCs and odorous gases treatment. J. Environ. Manag. 222, 409–419. doi: 10.1016/j.jenvman.2018.06.001
Xing, Y., Xu, Y., Shi, M., and Lian, Y. (2016). The impact of PM2. 5 on the human respiratory system. J. Thorac. Dis. 8:E69. doi: 10.3978/j.issn.2072-1439.2016.01.19
Yahaya, D., and Ibrahim, T. (2012). Development of rice husk briquettes for use as fuel. Res. J. Eng. Appl. Sci. 1, 130–133. Available online at: http://citeseerx.ist.psu.edu/viewdoc/download?doi=10.1.1.676.5258&rep=rep1&type=pdf
Yasuhara, A., Katami, T., and Shibamoto, T. (2005). Dioxin formation during combustion of nonchloride plastic, polystyrene and its product. Bull. Environ. Contam. Toxicol. 74, 899–903. doi: 10.1007/s00128-005-0666-3
Keywords: plastic wastes, biomass, solid fuels, combustion, hazards, densification
Citation: Song B and Hall P (2020) Densification of Biomass and Waste Plastic Blends as a Solid Fuel: Hazards, Advantages, and Perspectives. Front. Energy Res. 8:58. doi: 10.3389/fenrg.2020.00058
Received: 01 March 2020; Accepted: 25 March 2020;
Published: 16 April 2020.
Edited by:
Richen Lin, University College Cork, IrelandCopyright © 2020 Song and Hall. This is an open-access article distributed under the terms of the Creative Commons Attribution License (CC BY). The use, distribution or reproduction in other forums is permitted, provided the original author(s) and the copyright owner(s) are credited and that the original publication in this journal is cited, in accordance with accepted academic practice. No use, distribution or reproduction is permitted which does not comply with these terms.
*Correspondence: Bing Song, bing.song@scionresearch.com; Peter Hall, peter.hall@scionresearch.com