- 1Department of Forest and Natural Resources Management, SUNY College of Environmental Science and Forestry, Syracuse, NY, United States
- 2Department of Biomedical and Chemical Engineering, College of Engineering and Computer Science, Syracuse University, Syracuse, NY, United States
- 3Department of Manufacturing and Mechanical Engineering Technology, College of Engineering Technology, Rochester Institute of Technology, Rochester, NY, United States
Dry matter losses (DML) and fuel quality changes occurring in storage piles are important parameters for the management of any biomass supply system. This study evaluates the effect of a hot water extraction pretreatment, harvest season, depth in storage pile and initial moisture content on willow biomass fuel quality [moisture, ash, higher (HHV) heating value and lower (LHV) heating value] during storage, and models DML in storage piles based on experimental data. For the summer storage (SS) pile, mesh bags containing freshly harvested chips (FC) were inserted at 0.5–1 m deep in the pile. For the winter storage pile (WS), the mesh bags were filled with FC and hot water extracted chips (HC) with three different initial moisture contents inserted in the shell (<0.45 cm) and the core (1–1.5 m) of the pile. The ash contents through all sampling periods were in the range of 1.1–2.2% for FC and 0.6–2.1% for HC from both the shell and core of the WS pile. Higher ash contents, in the range of 2.1–3.4%, were observed in SS pile. Moisture contents of the storage piles had differing patterns over time. DML was the highest in the SS pile, reaching up to 33.6% after 140 days in storage; in contrast, there was no significant increase in DML over the first winter season. Although DML of FC and HC were in the same range during the initial storage period, DML of HC was 40% lower than FC after 180 days of storage. Higher DML was observed in the core (e.g., 17.3% for FC) compared to the shell (e.g., 12.1% for FC) at the end of the WS trial. There was no particular trend observed between initial moisture and DML. This study suggests that a linear model is sufficient to estimate DML, but a non-linear model may be needed for chips stored in SS piles for 6 months or longer. It also suggests that DML is reduced in storage piles created in winter, and that willow chips kept in SS should be utilized within 2 months for a DML below a 10% threshold.
Introduction
Short rotation woody crops (SRWC), such as shrub willow (Salix spp.) and hybrid poplar (Populus spp.), are being developed in North America and Europe for bioenergy (Mola-Yudego et al., 2016; Volk et al., 2016, 2018). In the Northeast United States, willow chips are currently mixed with other wood chips to generate heat and power (Volk et al., 2016). Storage is an essential component of the biomass supply system because harvesting windows are limited while demand for feedstock for power, biofuels and biobased chemicals is required year-round. Maintaining feedstock quality during storage is essential for end users and the success of the entire system.
Dry matter loss (DML) can occur at multiple places along the supply chain such as during harvesting, loading and unloading of vehicles, transportation, and storage. DML is an important factor as it can translate into loss of revenue (Routa et al., 2018; Therasme et al., 2019), waste of resources, higher greenhouse gas emissions per unit of energy delivered, and challenges at conversion facilities. A DML of 10% during the storage of willow biomass for heat production would result in 6% increase of greenhouse gas emissions per unit of delivered heat and 9% decrease of the net energy yield per unit of land (Whittaker et al., 2016).
DML in storage piles is caused by biological decomposition and chemical reaction (Krigstin and Wetzel, 2016) and is influenced by numerous factors, including composition of the material, particle size, cover system, storage duration, pile size, harvest season, species, oxygen availability, weather conditions, and moisture content (Manzone et al., 2013; Barontini et al., 2014; He et al., 2014; Hofmann et al., 2017; Pari et al., 2017; Pecenka et al., 2018; Whittaker et al., 2018). Additional questions remain to be addressed to understand the effect of biomass preprocessing on DML during storage and the variation of DML at different depth within a storage pile.
The season of harvest influences the initial quality of harvested biomass and will impact reactions in storage piles. Whether leaves are included in the harvested material or not has an influence on the quality of the material as well as potential implications for nutrient cycling at the site. During spring and summer, the harvested biomass dries naturally as a result of high air temperature. But, moisture content increases during autumn and winter storage (Filbakk et al., 2011). The moisture content of uncovered willow chip storage piles harvested in late spring with a moisture content of 46% decreased to 37–26% after 60 days of storage and increased thereafter until the end of the storage trial in mid-autumn (Therasme et al., 2019). However, Eisenbies et al. (2016) reported an increase of moisture from 42% at harvest in February to 49% in May and 44% in July. Bark and foliage have higher organic nitrogen and moisture content than wood and higher spore counts, both of which can stimulate bacterial and fungal growth (Krigstin and Wetzel, 2016).
Most recent SRWC storage studies in the United States do not report the amount of DML in outdoor storage pile of wood chips (Ergül and Ayrilmis, 2014; Lin and Pan, 2015; Eisenbies et al., 2016; Therasme et al., 2019). However, DML reported in European studies ranges from 0.9 to 4.5% per month and total DML can reach up to 47% after 18 months of storage in open outdoor piles (Table 1). While these studies contribute to a better understanding of the rate of DML and factors that influence it, the direct use of these values in modeling, such as techno-economic assessment or life cycle assessment of biomass storage systems, is only valid for storage under similar conditions. Also, while some studies show linear increase of DML over time others report positive but diminishing rate where DML approaches some asymptotic maximum (Mooney et al., 2012).
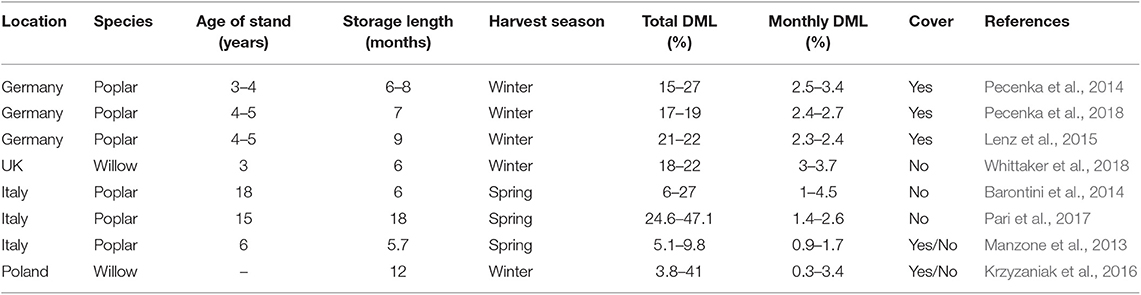
Table 1. Reported values for dry matter loss (DML) for outdoor storage piles of willow and poplar chips.
Hot water extraction (HWE) of wood chips is a preprocessing step that removes predominately hemicellulose along with smaller amounts of other compounds and ash. Hemicellulose can be hydrolyzed to generate fermentable C-5 sugars and organic acids, while leaving behind a solid residue. The solid residue that remains after HWE contains a higher fraction of lignin and cellulose than the non-extracted chips, resulting in an increase in the higher heating value (Therasme et al., 2018). The removal of a fraction of the original weight of the wood chips increases the porosity and pore size of the pulp fibers, which could affect water absorption and retention (Duarte et al., 2011). These changes in the structure and composition of the HWE wood chips raise questions about the dynamics of this material in storage piles and how DML is impacted.
The first objective of this study is to determine the dry matter loss, and changes in moisture, ash, and heating value of hot water extracted and non-extracted willow chips stored in a pile created at the start of winter and non-extracted willow chips in a pile created in the summer. The second objective is to evaluate the relationship between DML and storage time, moisture content, ash content, and harvest season of willow chips. Moisture content, ash content, and heating value are key parameters, especially for thermochemical conversion processes, because they influence the conversion efficiency and the cost of production. Conversion facilities will operate year-round so the changes in quality and DML associated with storage are important to understanding how feedstock systems for these facilities need to be designed and managed.
Materials and Methods
To address the objectives of this study, we established two storage piles with freshly harvested willow biomass that were monitored on a regular basis. The first pile was constructed with leaf-on freshly harvested materials in summer 2017. A second pile was made with leaf-off materials harvested during the winter 6 months later. Bags of fresh chips (FC) and HWE chips (HC) with three levels of initial moisture contents were inserted in the core and shell of the winter pile. The bags were collected from the piles over the entire storage period to determine DML, moisture, ash, higher and lower heating values.
Summer Storage Pile
The summer storage (SS) trial took place in Solvay, NY (43°03′56.0″N, 76°15′42.4″W). The site was a former industrial site containing high level of calcium, sodium, and chloride ions (Effler, 1996). The area harvested for this experiment was planted in 2012 with the following willow cultivars: Fish Creek, SX61, Millbrook, Sherburne, SX64, and Canastota. They were coppiced after the 2012 growing season and harvested for the first time on 21–22 June 2017 with a New Holland FR9080 forage harvester equipped with a New Holland 130FB coppice header. The harvester was set to produce the largest chips size (33 mm). The leaf content determined by manual sorting of six random samples of harvested material was 7.4 ± 4.2%. The particle size distributions of the harvested chips are listed in Table 2.
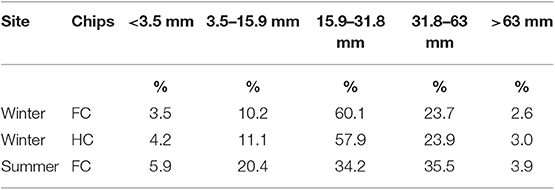
Table 2. Particle size distribution of fresh (FC) and hot water extracted (HC) willow chips that were placed in bags and inserted into storage piles.
The SS pile was constructed at the edge of the field on the same day that harvesting was conducted. Loads of willow chips were dumped in a rough linear pile on open ground and shaped to produce an even contour using a tractor equipped with a front loader. The pile was 21.8 m long, 8.8 m wide, and 2.6 m tall. A total of 68 mesh bags (45 × 55 cm) made of polypropylene and containing 1.2–2.2 kg of FC willow biomass were inserted (~2 m intervals) at about 0.5–1 m from the surface of the storage pile. HC was not included in this trial because this material was not available at that time. The exact locations of the samples were identifiable with a colored rope that was tied to the bags and brought to the surface of the pile. The weight of wood chips inserted in each net bag was recorded. While filling the bags, a total of 38 samples of approximately 1 kg were collected for initial moisture content determination. Finally, up to 12 temperature sensors connected to three data loggers (HOBO U12-008) were placed at 0.5–1 m deep in the storage pile to record pile temperatures automatically every 30 min. Air temperature and precipitation for the site were extrapolated from PRISM (Parameter-elevation Regressions on Independent Slopes Model) climate data for the site1.
Winter Storage Pile
The winter storage pile (WS) experiment took place in Tully, NY (42°47′50″N, 76°07′09″W). Both sites—Tully and Solvay—classify as “Dfb” (snow, fully humid, warm summer) under the Köppen–Geiger climate classification (Kottek et al., 2006). A mixture of different cultivars of 3-year-old aboveground stems was harvested with the same settings on the New Holland harvester after leaf fall. The harvesting operation for this trial occurred in 2017 at two separate dates; on December 17th to collect the materials for the HWE run and December 22nd for the pile construction.
A fraction of the FC biomass was preprocessed via HWE prior to being included in the storage piles. The extraction of FC equivalent to 263 kg OD was performed in a 1.8 m3 digester for 2 h at 160°C. The liquid to wood ratio was 4.7:1. At the end of the process the liquor was drained and the HC were washed by adding water equivalent to the volume of liquor removed into the digester and re-cooking at 80°C for 15 min. After draining the remaining liquor, the HC were removed from the digester. The total weight of chips was recorded before and after HWE, and samples were taken prior and after the HWE for moisture content determination. The HWE process removed 23.5% of the dry weight of the starting biomass. Two thirds of the HC were sent to the drying room and the remaining fraction was kept as is with no further processing.
For the WS trial, the bags were filled with two types of chips—HC and FC—with three moisture levels each (Table 3). The groups of HC and FC with lower moisture content were obtained by drying. The drying occurred in a kiln connected with a computer for automatic control of the relative air humidity and drying temperatures. For each samples group, the chips were homogenized by mixing then transferred into net bags. There were 54 net bags per group of samples for a total of 324 net bags filled with leaf off HC and FC willow biomass.
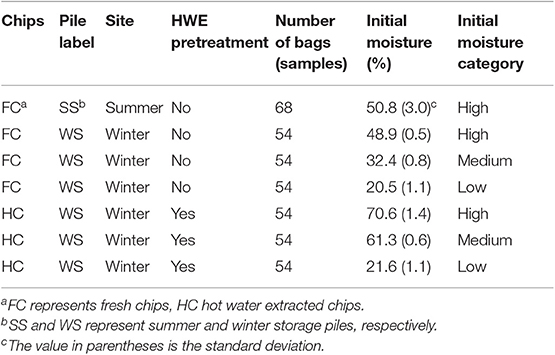
Table 3. Initial moisture content of freshly harvested and hot water extracted (HWE) willow chips that were inserted into storage piles in the summer (SS) with leaf material and in the winter (WS) when no leaves were present.
The winter pile was constructed on an open area near the harvesting site (Figure 1). Chips collected in a dump wagon were deposited on the ground in a rough linear pile and then molded into a consistent contour using a tractor equipped with a front loader. The height of this pile was 2.5 m, the width was 5.4 m, and the length was 19.1 m. At each sampling point, three bags of each treatment were placed in the core (1–2 m from the surface) and the shell (<45 cm from the surface) of the pile. The exact locations of the samples in the pile were marked using colored flags for the shell samples and willow stems for the core samples. The sampling points were placed at 1.5–2.5 m apart of each other along the length of the pile. A total of eight temperatures probes connected to two data loggers (HOBO U12-008) were inserted in the shell and core of the piles following the description provided by Eisenbies et al. (2016) and Therasme et al. (2019). Data loggers inserted in this storage pile automatically recorded the pile temperature every 30 min.
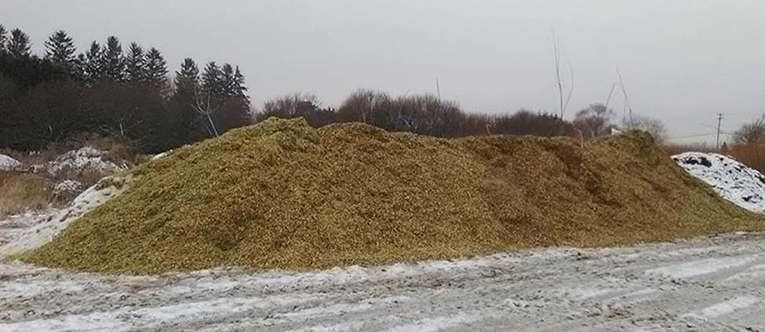
Figure 1. A winter storage pile of willow chips created at an experimental field station in Tully, NY.
Sample Collection and Laboratory Analysis
Samples were collected on a regular basis to monitor the loss of dry matter and changes of other characteristics of willow chips during storage. Up to four net bags were removed from the SS pile every 1–2 weeks starting on June 30th until November 11th 2017. Two additional sets of samples were removed months later to test if there would be additional increase of DML during the winter and summer seasons; one set of samples in April 2018 and another one in August 2018. For the WS pile, three samples from each group of bags were pulled out from the shell and the core of the pile on a monthly basis. Sampling started at the south end of the pile and at each sampling date we move to the next sample location to the north. This process was followed to minimize disturbances to bags that remained in the pile. During sampling, disturbance to the pile was minimized and localized because the location of the bags was marked. Hand tools were used to remove the samples and refilled the holes as soon as the bags were recovered.
The samples were taken to the lab immediately after being pulled out of the piles and dried at 65°C to a constant weight. The amount of DML was calculated based on the dry weight of samples before and after storage. Dried samples were split to reduce the size of the samples to about 200 g, then ground in a Wiley mill equipped with a 0.5-mm screen. The ash content was determined by combustion in a thermolyne muffle furnace (Model F30400) equipped with a ramping program in accordance with the National Renewable Energy Laboratory NREL/TP-510-42622 method (Sluiter et al., 2008). The results of ash contents are reported relative to the 105°C oven dry weight of the sample. The HHV was determined according to the ASTM method D5865-13: Standard test method for gross calorific value of coal and coke by using a Parr 6200 Oxygen bomb calorimeter (ASTM, 2013). The HHV results are reported on a dry basis. The LHV represents the maximum potential energy available in an as-received biomass fuel and was calculated using the formula described by Krigstin and Wetzel (2016).
Regression Analysis
The statistical analysis was conducted for DML by application of linear mixed models and non-linear regression models using Statistical Analysis System version 9.4 (SAS Institute, Cary, NC). The data for the SS and WS piles were combined together for this analysis. The data from the last two sampling points (284 and 417 days) of the SS pile were included only for the non-linear models. The non-linear models have the capability to represent curves with decreasing slope. However, the linear models apply for storage of biomass for a period of 1–6 months.
Linear Mixed Models
A full model (Equation 1) defines DML as a function of harvest season, initial moisture, moisture, ash, depth, HWE treatment and storage period. From the full model, three candidate models (Equations 2–4) were selected by using R2 and Mallows Cp criteria. The MIXED procedure was used to fit the selected models to the data by considering harvest season, depth and HWE treatment as fixed effects while allowing random deviation from one sampling period to another. A first-order autoregressive AR(1) variance structure was chosen to take into account the correlation between measurements on bags from the same pile's depth at adjacent periods and an unstructured structure for the random terms. Since bags were not returned to the pile and the requirement for the levels of the repeated effect to be different for each observation within a subject, the averages of the replicates were used to fit the models. This step was necessary to avoid singularity of the variance of the unobserved random errors. Statistical significance for parameter coefficients was claimed for p < 0.05.
Where αi, βij, and γi are regression model parameters, Ai and Aj are the covariates (i.e., harvest season, initial moisture, moisture, ash, depth, hot water extraction, and period), and A1 is storage period.
Model 1:
Model 2:
Model 3:
Non-linear Mixed Models
Considering that DML increases at a decreasing rate and eventually approaches some asymptotic maximum, two non-linear models were fit using the NLMIXED procedure. Model 4 (Equation 5) is an exponential decay model that gives rise to a maximum value (k0). However, model 5 (Equation 6) is based on a logistic function where the maximum DML (k0) depends on harvest season.
Model 4:
With k0 = α0 + α1 Season + α2 Depth + α3 Extraction + α4 Airtemp + α5 Precipitation, and c = γ0 + γ1 Season.
Model 5:
With k0 = α0 + α1Season + α2 Season × Depth + α3 Season × Extraction and
Where:
Period: number of days in storage;
Season: dummy variable designating summer or winter storage pile (0, 1);
Depth: dummy variable designating samples from the shell or the core of a storage pile (0, 1);
Extraction: dummy variable designating storage of freshly harvested willow biomass or storage of HWE willow biomass (0, 1);
Inimoisture: initial moisture content prior to storage (%wb);
Airtemp: average daily air temperature of the current month of storage (°C);
Precipitation: average daily precipitation of the current month of storage (mm);
Ash: ash content of samples when pulled out of the storage pile (%).
Results and Discussion
Weather Conditions and Pile Temperatures
For the SS, mean daily air temperature ranged from −19.1 to 24.4°C over the first 284 days, with an average air temperature of 7.5°C. During the first 2 months the mean daily air temperature was consistently above 15°C (Figure 2) creating favorable conditions for natural drying. Total precipitation was 913 mm over 284 days and the site received daily precipitation above 20 mm for a total of 9 days over the same period of time. The temperature in the SS pile increased rapidly to 47°C within 7 days and remained higher than the daily mean air temperature for 3 consecutive months; the pile temperature equilibrated with air temperature for the remaining of the SS trial.
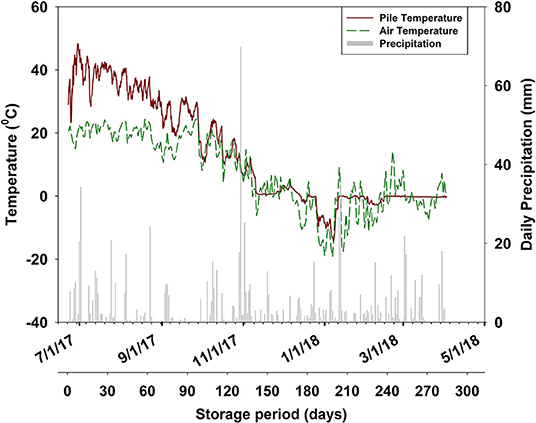
Figure 2. Daily mean air temperature, precipitation, and pile temperature measured at 0.5–1 meter from the surface of a summer willow chips storage pile in Solvay, NY (the full dataset for air temperature is available at http://prism.oregonstate.edu/explorer/).
For the WS pile the mean daily air temperature ranged from −19.8 to 26.4°C for a period of 207 days (Figure 3), with an average air temperature of 7.4°C. The air temperature during the WS pile trial was continuously below 15°C for more than 3 months. The site received a total of 585.5 mm of precipitation and a total of 5 days with daily precipitation exceeding 20 mm. Temperatures recorded in the shell and core of the WS pile showed differing patterns. The core temperature rose to 53°C in 3 weeks and remained higher than the air temperature for more than 3 months. However, the shell temperature followed the ambient air temperature for the entire storage trial and was below 0°C 65% of the time during the first 3 months.
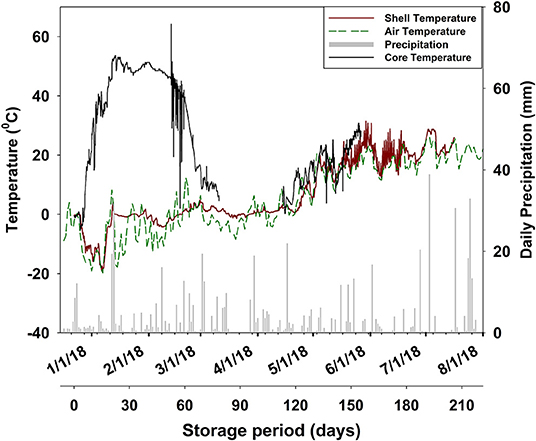
Figure 3. Daily mean air temperature, precipitation, and temperature in the shell and core of a winter willow chips storage pile in Tully, NY.
The rapid increase of temperature immediately after the construction of chips storage piles was expected and has been reported previously in the literature (Jirjis, 1995; Krzyzaniak et al., 2016; Whittaker et al., 2018; Therasme et al., 2019). This increase of temperature could be associated with heat released through wood respiration and microbial activities. The season of the pile construction seems to play a role in the differing temperature patterns observed between the shell and the core of the storage piles. Because the air temperature was below zero degree celsius most of the time during the first 2 months of storage (Figure 3) in a chips pile created in winter, as opposed to a pile created in late spring or summer, the outside layer (shell) of the pile froze, thus inhibiting any microbial activity that would generate additional heat and cause an increase of temperature in the shell of the WS pile.
Dry Matter Loss and Quality Change During Storage
Initially DML in the SS pile was slow, reaching 5.6% by day 43 and then it increased over time and reached 33.6% after 141 days (Table 4, Figure 4). Determination of DML at two additional sampling periods at much later dates showed no additional loss of dry matter between storage days 141–284, which includes the winter season, and slight increase of DML at a rate of 0.7% per month for the remaining of the storage. The results from this trial are in line with previous findings that indicate a decline in the rate of DML after long storage time (Jirjis, 1995; Anerud et al., 2018). However, the DML found in the SS trial were significantly higher than the 7.3% reported for a 7 month storage of logging residues chips in a SS pile (Anerud et al., 2018). During the first 2 months, the moisture content in the SS pile was the same or slightly lower than the initial moisture content of the harvested FC, but after 2 months it was consistently higher and reached up to 80%. This increased moisture content later during the storage trial was consistent with data reported in the literature for storage pile studies conducted in the region (Eisenbies et al., 2016; Therasme et al., 2019). There were also small changes in ash content within and from one sampling period to another, with mean ash content by sampling period in the range of 2.1–3.4%. The mean HHV by period was consistent, varying from 18.7 to 19.4 MJ/kg. The higher end for both ash and HHV were recorded at 141 days and they were, respectively, 35 and 3% higher than the corresponding values at the beginning of the storage. Because the moisture content of the chips in the pile started to increase after 2 months, the LHV also declined and was as low as 3.3 MJ/kg after 141 days.
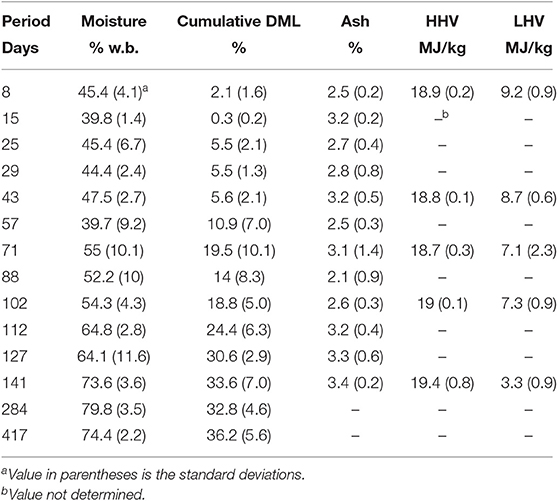
Table 4. Changes (mean and standard deviation) in dry matter loss (DML), moisture, ash, and higher heating value (HHV) and lower heating value (LHV) in a leaf-on willow summer storage (SS) pile in Solvay, NY (first day is June, 22nd 2017).
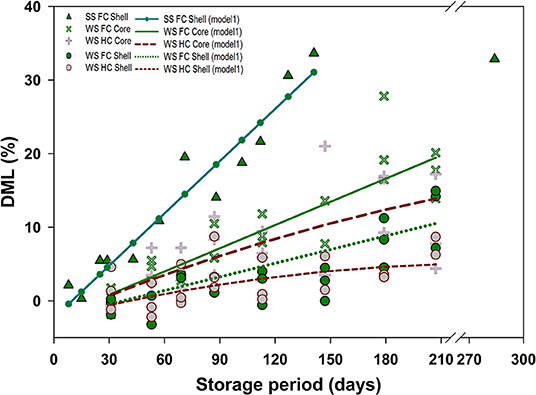
Figure 4. Representation of predicted dry matter loss (DML) (model 1) and DML value for fresh (FC) and hot water extracted (HC) willow biomass chips that were stored at various initial moisture in outdoor piles created in the winter (WS) and summer (SS).
The results reported in Tables 5, 6 depict the variability of DML, moisture, ash and heating values of FC and HC in the shell and core of the WS pile. After 207 days, DML in FC bags across the three moisture treatments were 7.2–14.2% in the shell and 13.9–20.1% DML in the core. For HC bags, DML were in the range of 6.3–8.7% in the shell and 7.7–17.2% in the core. The DML reported here for WS pile correspond to a rate of 1.0–2.9% per month, and are within the range of reported DML from WS pile (see Table 1). But, they were lower than the rates of 3.0–3.7% per month that were found in storage trials of shrub willow in UK (Whittaker et al., 2018) and the rate of 3.4% per month from another trial conducted in Poland (Krzyzaniak et al., 2016). The lower rate of DML from the current study is mostly due, despite the difference in geographic locations and local climate conditions, to the fact that the pile for the current study was created in the beginning of the winter season (December) while the other trials from UK and Poland were started in mid to late winter (February and March). Therefore, the higher ambient air temperatures in the UK and Poland trials could foster more the microbial activities. On average the DML was higher in the core of the WS pile than the shell throughout the storage trial. Perhaps, this may also be linked to the fact that the core of the WS pile was warmer than the shell during the first 2 months. However, the difference between FC and HC was relatively small during the first 150 days but was on average 6% higher in FC than HC at the end of the storage period. It is unclear why the difference between FC and HC become more evident only at the later stage of the storage, but it seems to be related with the structural and morphological changes of the wood chips during the extraction. HC has higher surface area than FC which may accelerate HC decays (Duarte et al., 2011). However, HC have higher proportion of lignin than FC (Therasme et al., 2018), therefore, preferential decomposition of carbohydrates may result in lower DML for HC in the long run.
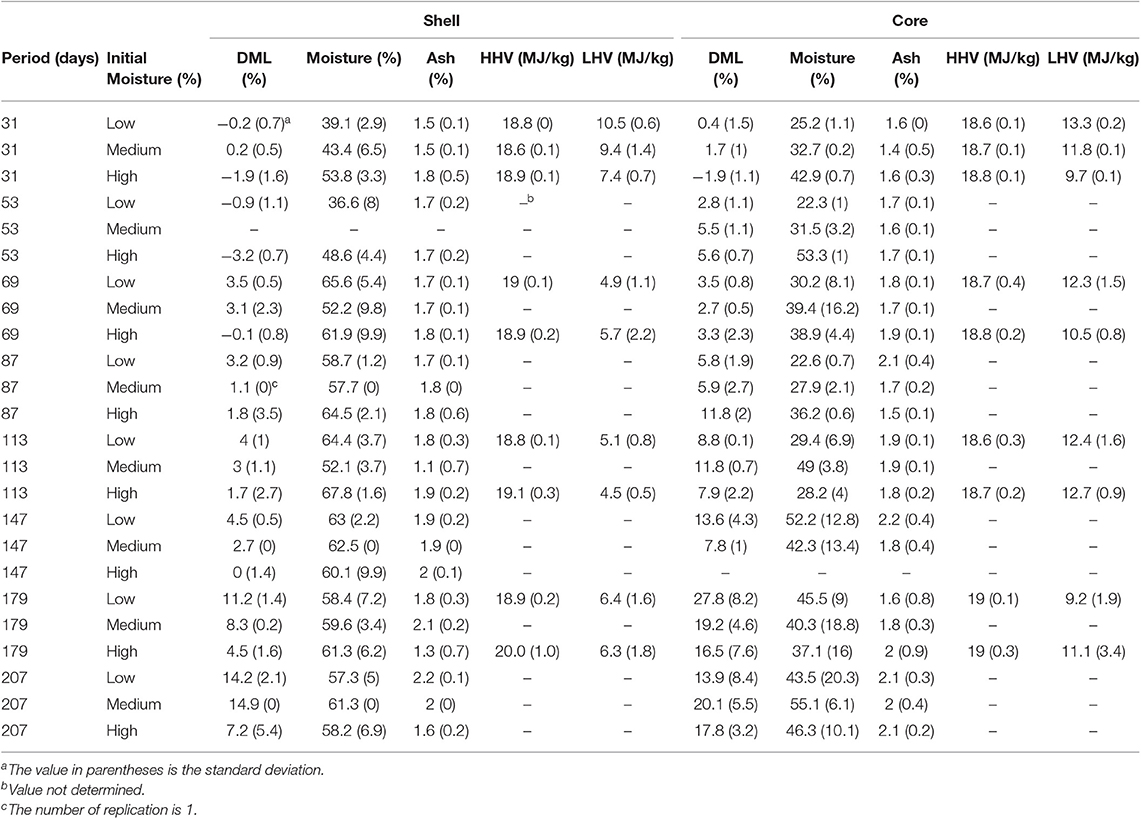
Table 5. Changes in dry matter loss (DML), moisture, ash, higher (HHV), and lower (LHV) heating value for three initial moisture contents of fresh chips (FC) in the core and shell of a leaf-off willow winter storage pile (first day is December, 22nd 2017).
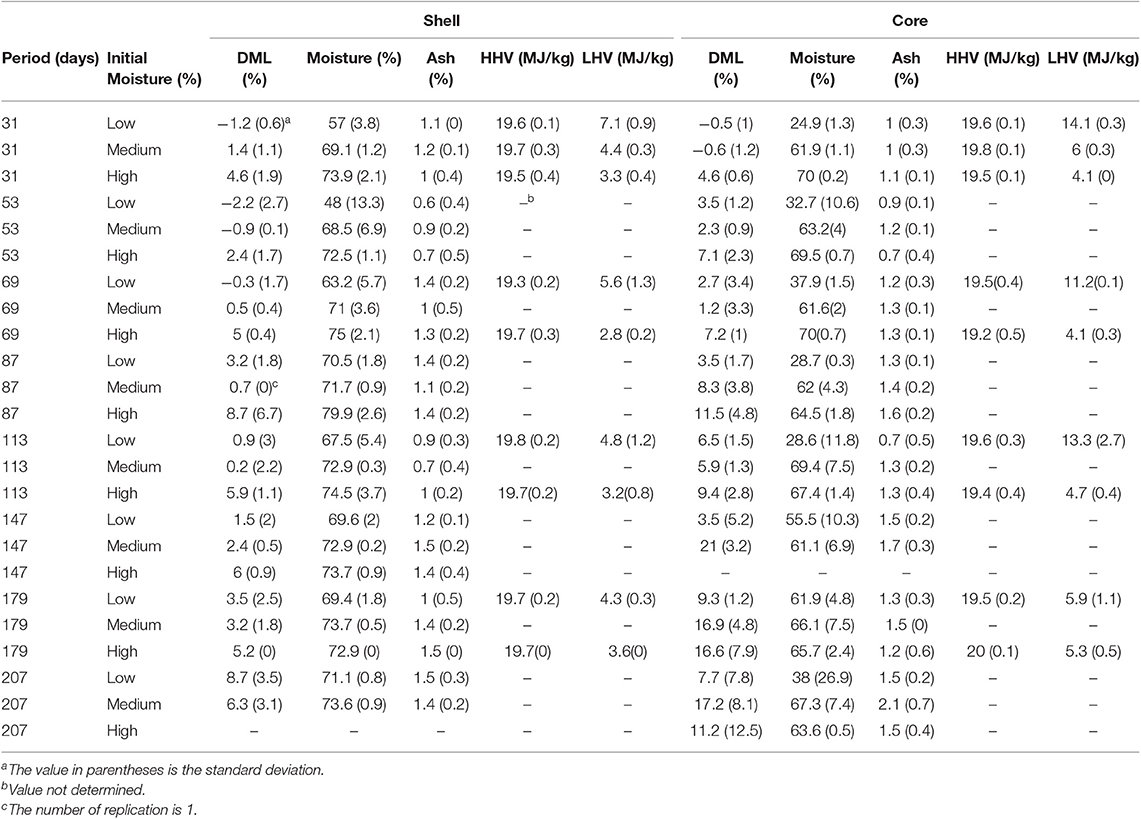
Table 6. Changes in dry matter loss (DML), moisture, ash, and higher (HHV) and lower (LHV) heating value for three initial moisture contents of hot water extracted chips (HC) in the core and shell of a leaf-off willow winter storage pile (first day is December, 22nd 2017).
In the absence of significant decay, it is possible to find negative but small values for DML as it was the case for some observations during the first 2 months in the shell of the WS pile. Similar observations were reported previously in the literature (Pecenka et al., 2014, 2018; Lenz et al., 2016). These results could be attributed to natural variations in the estimation of the true value.
The moisture content of FC (Table 5) and HC (Table 6) from the shell of the WS pile was constantly higher than the initial moisture (Table 3) for the entire storage period. The initial gradient of moisture among the different groups of bags that were inserted in the WS pile did not last for long in the shell of the WS pile. The low moisture FC bags, which had moisture differences at the beginning of the storage of 12% with medium moisture FC and 28% with high moisture FC, ended after 31 days of storage with moisture differences of only 4% with medium moisture FC and 15% with high moisture FC. For low moisture HC bags the differences were, respectively 12 and 17% with medium moisture HC and high moisture HC. At the end of the study period, the average moisture contents within all three initial moisture treatments were 57–61% for FC bags and 71–74% for HC bags from the shell of the pile. The higher moisture content observed for the HC could be explained in part by their increased cell wall porosity after hot water extraction (Duarte et al., 2011).
Similar to the shell, the moisture content of bags from the core of the WS pile was generally higher than the initial moisture content except for high moisture FC (Table 5) and high moisture HC (Table 6). The high moisture FC had up to 21% point decrease in moisture (113 days) while the highest decrease for low moisture HC was 7% after 207 days. These observations are the results of two processes: (1) dried and low moisture chips absorbing moisture from humid air and wetter chips in the surrounding, and (2) wetter chips releasing moisture as part of the natural drying process in the core of the pile. Both FC and HC from the core of the WS pile were drier than those from the shell. The moisture content of FC in the core was on average across the storage period 15–20% point lower than the shell, while it was 26% for low moisture HC and 7% for medium moisture HC and low moisture HC. At the end of the storage the moisture content in the core of the WS pile was 43–45% for all three FC groups. Differing moisture contents across different layers of the pile have been reported previously in the literature (Jirjis, 2005; Anerud et al., 2018; Therasme et al., 2019). For this trial, the differences between the moisture content in the shell and the core could be attributed to: (1) the high temperature recorded in the core of the WS during the first 2 months, thus facilitating the evaporation in the core, (2) low ambient air temperature limiting the evaporation in the outside layer (shell) of the pile, (3) addition of moisture to the shell of the pile through precipitation and exposure to air humidity.
Compared to FC from the SS pile, the FC and HC bags from the WS pile had lower ash content because of the absence of foliage in the winter and the removal of mineral elements during the HWE process. The ash content across all sampling periods was in the range of 1.1 to 2.2% for FC bags and 0.6 to 2.1 for HC from the shell and core of the WS pile. There was no clear trend for the HHV of the WS pile over time. However, the HC samples had slightly higher HHV than the FC samples. The HHV of FC samples for all the sampling periods ranged from 18.6 to 20 MJ/kg with an average of 18.9 MJ/kg. For HC, the HHV ranged from 19.2 to 20 MJ/kg with an average value of 19.6 MJ/kg. These differences can be explained by the structural changes that occurred on the chips during the HWE. When applied to bamboo, the HWE process decreases the oxygen/carbon ratio from 0.43 to 0.34 in the exterior and 0.37 in the interior surface (Ma et al., 2013). These observations corroborate with other findings that indicate a decrease of the percentage of hemicellulose, which has higher oxygen/carbon ratio and lower heating value than both lignin and cellulose, in HWE biomass (Demirbaş, 2001; Pu et al., 2011; Corbett et al., 2015; Therasme et al., 2018).
Linear and Non-linear Mixed Regression Models
Model 1 shows that harvest season and depth in the storage pile affect the slope of DML while HWE treatment affects the curvature of DML (Table 7). All the variables included in this model were significant. The coefficients of the equations to calculate the DML in the SS pile and WS pile (Table 8) were determined by replacing the dummy variables in model 1 by their assumed values. Model 1 predicts the highest rate of DML (7.1% per month) in SS piles. For WS pile, higher rate of DML is expected from the core (3.1% per month) than the shell (1.9% per month), and HC will end up with lower DML than FC at the end of the storage (Figure 4). The model predicts that after 3 months in storage, FC would lose 3.2% dry matter in the shell and 7.1% in the core while for HC it would be 2.2% in the shell and 6.1% in the core of a WS pile. DML of the pile can be obtained by the weighted average of DML in the shell and DML in the core. Considering a 45 cm shell (Eisenbies et al., 2016), the mean ratio between core and shell of the WS would be 60:40 which would result in 5.5% DML for FC after 3 months. The data for SS pile was not detailed enough to estimate the DML at different depth in the pile. However, it is expected that the predicted DML for SS piles represent the average DML. The bags in the SS piles were located at the interface of the core-shell line. Furthermore, it was previously found that the temperatures in core of unprotected piles were only 9.9°C higher than the shell (<45 cm) during the first month of a storage trial of willow starting late spring to November (Therasme et al., 2019).
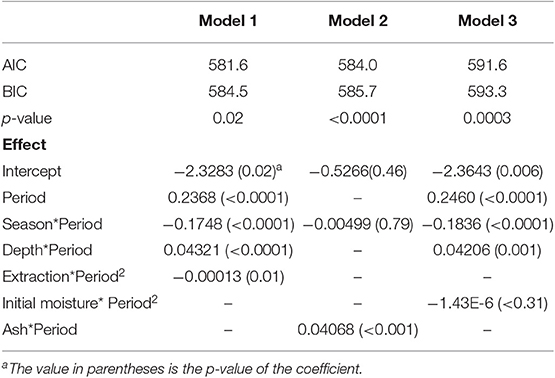
Table 7. Coefficients of three mixed model candidates for dry matter loss of willow biomass when stored in piles, and Akaike Information Criterion (AIC) and Bayesian Information Criterion (BIC).
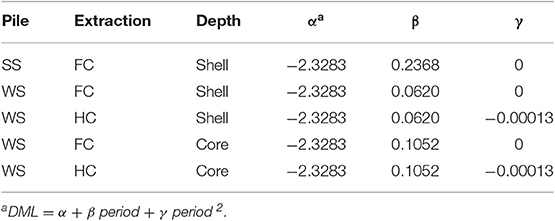
Table 8. Coefficients of model 1 to estimate the dry matter Loss (DML) in a summer storage (SS) pile and a winter storage (WS) pile of freshly harvested willow chips (FC) and hot water extracted willow chips (HC).
Model 2 shows the relationship between DML, number of days in storage and ash content (Table 7). At a given storage period higher ash content was related to higher DML. Although model 2 is defined by different parameters that model 1, it corroborates with the conclusion that was drawn from model 1. Ash content was 35% lower in harvested leaf-off willow than leaf-on willow. Also, HC has lower ash content than FC (Tables 5, 6). Therefore, model 2 indicates lower rate of DML for HC than FC and lower rate for leaf-off chips than leaf-on. The increased proportion of ash alone could have been used to estimate the amount of DML, however preliminary screening using R2 and CP Mallow criteria suggested that model 2 was a better option.
According to model 3, the initial moisture of the chips did not have a significant contribution to the curvature of the DML curve. However, studies in Sweden reported that initial moisture correlates well with observed DML in wood chips storage; for initial moisture in the range of 20–58%, monthly DML ranges from 0.23–2.6%, with the highest monthly loss being associated with the highest initial moisture content (Wihersaari, 2005). Another storage trial on sweet sorghum bagasse, a non-woody biomass, showed that initial moisture strongly affected observed DML; 31% DML for storage at 26% moisture and 4% DML for storage at 12% moisture (Athmanathan et al., 2015). The differences of the findings with this study may be explained by the fact that the sweet sorghum biomass was stored indoor in a controlled environment, the bales with the 12% moisture were below the fiber saturation point, and that the moisture gradient in the willow chips between the different initial moisture treatments did not hold long enough to favor differing rate of DML.
The coefficients for model 4 and model 5 are reported in Table 9. Model 4 predicts a maximum DML of 33.4% after 140 days in the SS pile. The variables depth, harvest season, temperature and precipitation were all significant. Although model 4 predicts lower maximum DML for HC, the coefficient for the extraction term is not significant. This model suggests that for a given period higher air temperature and precipitation within the last 30 days leads to higher DML. Ambient air temperature regulates the temperature inside of the pile, which regulates the population of microorganisms inhabiting the pile. Suitable temperatures for most fungi ranges from 15 to 60°C; mesophilic fungi thrive at 20–30°C and thermophilic fungi show optimal growth at 40–50°C (Krigstin and Wetzel, 2016). However, the magnitude of the effect of temperature on DML declines after long storage period. For example, DML of SS pile increased rapidly during the first summer, but a year later (next spring/summer season) the rate of DML slowed down despite the recorded pile temperature was in the optimal range for microbial growth. This might be the result of low pile temperature during the winter season, so the fungi population did not survive, and maybe the initially available part of the wood for these microorganisms is already largely consumed and different group of microorganisms is needed to breakdown when the optimal temperature is reached again.
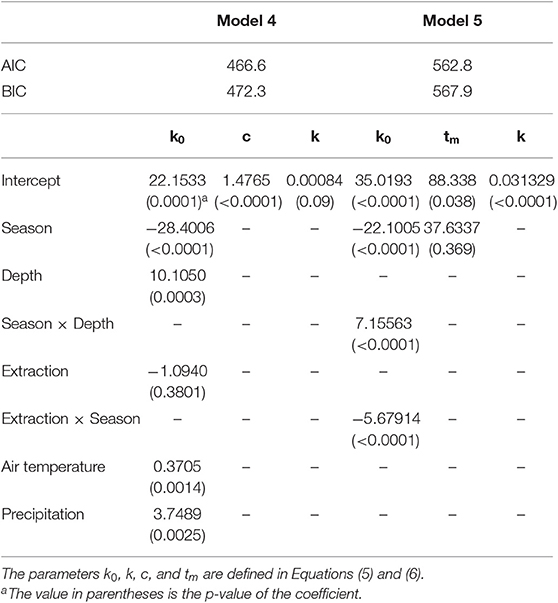
Table 9. Coefficients of two non-linear models that predict dry matter loss of hot water extracted chips (HC) and fresh chips (FC) when stored in outdoor summer and winter pile, and Akaike Information Criterion (AIC) and Bayesian Information Criterion (BIC).
According to model 5, the rate of DML is maximal at the end of the third month of storage for SS pile and after the fourth month for WS pile. So, chips stored in winter pile could last longer in pile before they are being processed. It is unclear whether a storage pile constructed in early fall would show the same pattern observed for SS or WS pile or not, because after about 3 months in storage the difference between the pile temperature will be small, thus, the temperature inside a fall pile would not be favorable for microbial decays after three or more months in storage (during the winter season). However, one could hypothesize that a spring storage pile could still show the fastest mass loss after about 3–4 months because the pile temperature would be high enough for mesophilic fungi to thrive.
Scatter plots of actual DML vs. predicted DML (Figure 5) indicate that the selected models fit the data with high level of accuracy. The predicted DML from all the models, except model 2, have an overall 1:1 relationship (with R2 > 0.75) with the actual DML. Also, the null likelihood ratio test is highly significant for models 1, 2, and 3, which indicates that the first order autoregressive structured covariance matrix is preferred to the diagonal matrix of the ordinary least squares null models. Model 1 has the lowest AIC and BIC among the linear models while the AIC and BIC for model 4 was lower than model 5 suggesting that model 1 would be the preferred linear model and model 4 the preferred non-linear model. Nevertheless, with an R-square of 0.76, model 5 can be very useful particularly when air temperature and precipitation data are not available. Simplified but accurate DML models are crucial for biomass supply chain logistics, techno-economic analysis, and life cycle assessment of bioenergy systems. For example, Mooney et al. (2012) used a DML model and storage cost of switchgrass bale to illustrate the breakeven prices for optimal outdoor storage. Another study (Routa et al., 2018) uses field experiment DML data, to evaluate the cost effects of DML of delimbed small diameter energy wood stems of pine during the storage in pile.
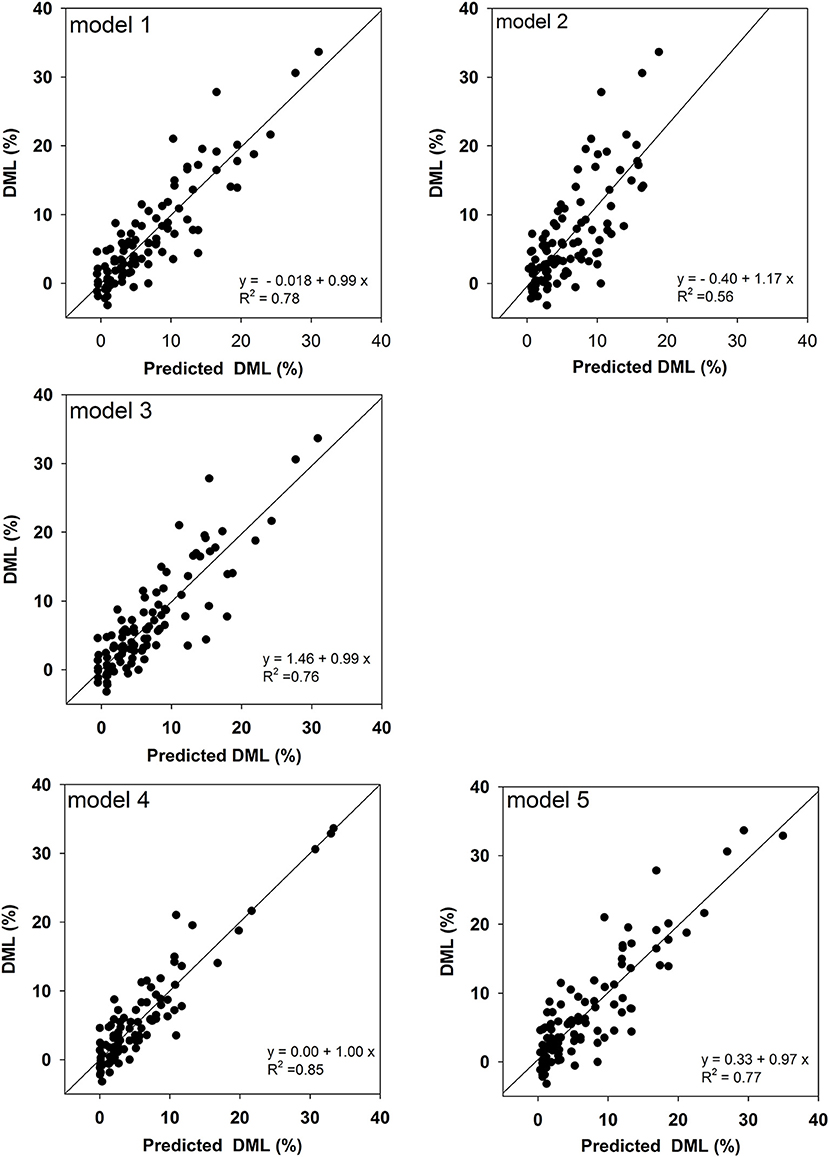
Figure 5. Scatter plots and regression lines between dry matter loss (DML) and predicted DML from linear models 1, 2, and 3 and non-linear models 4 and 5 for willow chips stored in the shell and core of outdoor piles at different time of the year.
Predicted DML during storage can be used for the screening of optimal biomass storage pile size. For example, because DML in the shell differs from the core, one can vary the ratio between the volume of core and shell of the pile to minimize the overall DML for the entire pile. In the case of WS pile, it is evident that the ratio between core and shell should be kept low i.e., small size pile rather than large pile. However, for conversion pathways that are more dependent on the LHV of the fuel (e.g., biopower), the moisture content of the chips will also drive the selection of the optimal pile size. The change of the amount of net energy, i.e., excluding latent heat of water vaporization, on a dry basis, simulated for varying pile core volume ratio shows multiple patterns (Figure 6). Considering case A as a control, increased DML in the shell and core (case B) would result in reduced net energy per unit of initial mass of chips while a simultaneous increase of DML and decrease of moisture (case D) can show increased net energy. Considering the predicted DML and approximate moisture in the core and shell of a WS pile after 3 months (Case C) and 6 months (case E), the net energy increases when the fraction of the core volume increases. Therefore, there would be more advantages creating larger storage piles for scenario C and E.
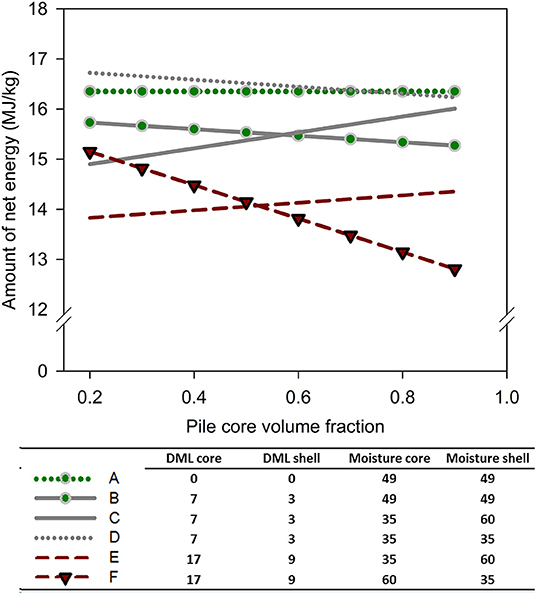
Figure 6. Calculated net energy from 1 kg (oven dry) harvested willow chips for different pile core volume fractions for selected dry matter loss (DML) and moisture content. Case A represents the situation when the harvested biomass is combusted immediately after harvest with no DML. Cases B–F represent different hypothetical situations depicting different DML and moisture values for stored chips. The higher heating value (HHV) for this calculation is 18.7 MJ/kg.
End user facilities (e.g., biorefineries) will need biomass supplies all year round to be productive. This research study reports on storage piles created in the winter and summer seasons and provides useful insights on how the quality of willow biomass that is harvested at two different points and then delivered to an end user will change over time. This is important information to more accurately model willow biomass quality and costs for year-round supply. However, there are only two discrete harvesting events in this study and the occurred in 1 year so the variability of weather patterns is not captured. So, further storage trials with piles constructed at different points in fall and late summer and over different years are still needed to accurately reflect how DML will impact changes in both quality and quantity of biomass for year-round operations. While the discretization of the pile into shell and core in this study may suffice for relatively small piles created at the edge of the field, a rather more complex discretization method may be required for larger piles created at the end user facility or piles that have been submitted to compaction.
Conclusions
This study determined the changes in dry matter loss, moisture, ash, and heating value during the storage of shrub willow chips in piles built in summer and winter. Of the two storage pile experiments, it was shown that the rate of DML was higher in SS piles than WS piles; DML in a SS pile increased at a rate of 7.1% per month during the first summer and fall season of the storage, then decreased to a rate of 0.7% in the spring and the following summer while DML in a WS pile created with freshly harvested willow chips increased by only 1.0% per month in the shell and 2.6% in the core of the pile. This study presents three linear and two non-linear DML models that could be used in techno-economic analysis, environmental life cycle assessment, and supply planning of willow biomass for bioenergy applications in the northeast United States or other regions with similar climate patterns. The linear models apply to SS pile not exceeding 140–150 days as the slope of the DML in the SS pile will decrease and tend toward zero during the winter season. However, for a longer storage period, the non-linear models have the feature to capture the decreasing rate of DML over time. This study demonstrates also that while the DML of hot water extracted chips were in the same range with non-extracted chips in the early period of storage, the hot water extracted chips had lower DML at the end of the WS storage (207 days). Pile configuration (e.g., core/shell fraction) could be an important factor to consider in order to increase the net amount of energy from stored chips.
Data Availability Statement
All datasets generated for this study are included in the article/supplementary material.
Author Contributions
OT, TV, and ME conceptualized and designed the experiments and revised the manuscript. OT, HS, and NU performed the experiments. OT performed the data analysis and drafted the manuscript.
Funding
This research was funded by the US Department of Energy Bioenergy Technologies Office under award number DE-EE0006638, and the Agriculture and Food Research Initiative Competitive Grant No. 2012-68005-19703 from the USDA National Institute of Food and Agriculture. HS and NU received support from MercyWorks SYNERGY Summer Internship Program to participate in this project.
Conflict of Interest
The authors declare that the research was conducted in the absence of any commercial or financial relationships that could be construed as a potential conflict of interest.
Acknowledgments
The authors would like to thank Karl Hallen for harvesting the biomass used in this study and his contribution in the construction of the storage piles, Qian Wang, Daniel P. L. de Souza, Justin Heavey, and Kaitlin C. Knutson for their contribution at different stages of this project.
Footnote
1. ^PRISM Climate Group, Oregon State University Data Explor. Time Ser. Values Individ. Locat. Available online at: http://prism.oregonstate.edu (accessed December 27, 2018).
References
Anerud, E., Jirjis, R., Larsson, G., and Eliasson, L. (2018). Fuel quality of stored wood chips – Influence of semi-permeable covering material. Appl. Energy 231, 628–634. doi: 10.1016/j.apenergy.2018.09.157
ASTM (2013). Standard Test Method for Gross Calorific Value of Coal and Coke. West Conshohocken, PA: American Society for Testing and Materials.
Athmanathan, A., Emery, I. R., Kuczek, T., and Mosier, N. S. (2015). Impact of temperature, moisture, and storage duration on the chemical composition of switchgrass, corn stover, and sweet sorghum bagasse. BioEnergy Res. 8, 843–856. doi: 10.1007/s12155-014-9563-0
Barontini, M., Scarfone, A., Spinelli, R., Gallucci, F., Santangelo, E., Acampora, A., et al. (2014). Storage dynamics and fuel quality of poplar chips. Biomass Bioenerg. 62, 17–25. doi: 10.1016/j.biombioe.2014.01.022
Corbett, D., Kohan, N., Machado, G., Jing, C., Nagardeolekar, A., and Bujanovic, B. (2015). Chemical composition of apricot pit shells and effect of hot-water extraction. Energies 8, 9640–9654. doi: 10.3390/en8099640
Demirbaş, A. (2001). Relationships between lignin contents and heating values of biomass. Energy Convers. Manag. 42, 183–188. doi: 10.1016/S0196-8904(00)00050-9
Duarte, G. V., Ramarao, B. V., Amidon, T. E., and Ferreira, P. T. (2011). Effect of hot water extraction on hardwood kraft pulp fibers (Acer saccharum, Sugar Maple). Ind. Eng. Chem. Res. 50, 9949–9959. doi: 10.1021/ie200639u
Effler, S. W. (1996). Limnological and Engineering Analysis of a Polluted Urban Lake: Prelude to Environmental Management of Onondaga Lake, New York. New York, NY: Springer. doi: 10.1007/978-1-4612-2318-4
Eisenbies, M. H., Volk, T. A., and Patel, A. (2016). Changes in feedstock quality in willow chip piles created in winter from a commercial scale harvest. Biomass Bioenerg. 86, 180–190. doi: 10.1016/j.biombioe.2016.02.004
Ergül, E., and Ayrilmis, N. (2014). Effect of outdoor storage conditions of wood chip pile on the technological properties of wood-based panel. Biomass Bioenerg. 61, 66–72. doi: 10.1016/j.biombioe.2013.11.025
Filbakk, T., Høib,ø, O. A., Dibdiakova, J., and Nurmi, J. (2011). Modelling moisture content and dry matter loss during storage of logging residues for energy. Scand. J. For. Res. 26, 267–277. doi: 10.1080/02827581.2011.553199
He, X., Lau, A. K., Sokhansanj, S., Lim, C. J., Bi, X. T., and Melin, S. (2014). Investigating gas emissions and dry matter loss from stored biomass residues. Fuel 134, 159–165. doi: 10.1016/j.fuel.2014.05.061
Hofmann, N., Mendel, T., Schulmeyer, F., Kuptz, D., Borchert, H., and Hartmann, H. (2017). Drying effects and dry matter losses during seasonal storage of spruce wood chips under practical conditions. Biomass Bioenerg. 111, 196–205. doi: 10.1016/j.biombioe.2017.03.022
Jirjis, R. (1995). Storage and drying of wood fuel. Bioenerg. Biomass 9, 181–190. doi: 10.1016/0961-9534(95)00090-9
Jirjis, R. (2005). Effects of particle size and pile height on storage and fuel quality of comminuted Salix viminalis. Biomass Bioenerg. 28, 193–201. doi: 10.1016/j.biombioe.2004.08.014
Kottek, M., Grieser, J., Beck, C., Rudolf, B., and Rubel, F. (2006). World Map of the Köppen-Geiger climate classification updated. Meteorol. Z. 15, 259–263. doi: 10.1127/0941-2948/2006/0130
Krigstin, S., and Wetzel, S. (2016). A review of mechanisms responsible for changes to stored woody biomass fuels. Fuel 175, 75–86. doi: 10.1016/j.fuel.2016.02.014
Krzyzaniak, M., Stolarski, M. J., Niksa, D., Tworkowski, J., and Szczukowski, S. (2016). Effect of storage methods on willow chips quality. Biomass Bioenerg. 92, 61–69. doi: 10.1016/j.biombioe.2016.06.007
Lenz, H., Idler, C., Hartung, E., and Pecenka, R. (2015). Open-air storage of fine and coarse wood chips of poplar from short rotation coppice in covered piles. Biomass Bioenerg. 83, 269–277. doi: 10.1016/j.biombioe.2015.09.018
Lenz, H., Pecenka, R., Hartung, E., and Idler, C. (2016). Development and test of a simplified method to calculate dry matter loss during open-air storage of poplar wood chips by analysing ash contents. Biomass Bioenerg. 94, 258–267. doi: 10.1016/j.biombioe.2016.09.011
Lin, Y., and Pan, F. (2015). Monitoring woody biomass chips quality change during field storage in Michigan. For. Prod. J. 65, 327–336. doi: 10.13073/FPJ-D-14-00069
Ma, X. J., Cao, S. L., Lin, L., Luo, X. L., Chen, L. H., and Huang, L. L. (2013). Surface characterizations of bamboo substrates treated by hot water extraction. Bioresour. Technol. 136, 757–760. doi: 10.1016/j.biortech.2013.03.120
Manzone, M., Balsari, P., and Spinelli, R. (2013). Small-scale storage techniques for fuel chips from short rotation forestry. Fuel 109, 687–692. doi: 10.1016/j.fuel.2013.03.006
Mola-Yudego, B., Rahlf, J., Astrup, R., and Dimitriou, I. (2016). Spatial yield estimates of fast-growing willow plantations for energy based on climatic variables in northern Europe. GCB Bioenerg. 8, 1093–1105. doi: 10.1111/gcbb.12332
Mooney, D. F., Larson, J. A., English, B. C., and Tyler, D. D. (2012). Effect of dry matter loss on profitability of outdoor storage of switchgrass. Biomass Bioenerg. 44, 33–41. doi: 10.1016/j.biombioe.2012.04.008
Pari, L., Scarfone, A., Santangelo, E., Gallucci, F., Spinelli, R., Jirjis, R., et al. (2017). Long term storage of poplar chips in Mediterranean environment. Biomass Bioenerg. 107, 1–7. doi: 10.1016/j.biombioe.2017.09.007
Pecenka, R., Lenz, H., and Idler, C. (2018). Influence of the chip format on the development of mass loss, moisture content and chemical composition of poplar chips during storage and drying in open-air piles. Biomass Bioenerg. 116, 140–150. doi: 10.1016/j.biombioe.2018.06.005
Pecenka, R., Lenz, H., Idler, C., Daries, W., and Ehlert, D. (2014). Development of bio-physical properties during storage of poplar chips from 15 ha test fields. Biomass Bioenerg. 65, 13–19. doi: 10.1016/j.biombioe.2014.04.017
Pu, Y., Treasure, T., Gonzalez, R., Venditti, R., and Jameel, H. (2011). Autohydrolysis pretreatment of mixed hardwoods to extract value prior to combustion. Bioresources 6, 4856–4870. doi: 10.15376/biores.6.4.4856-4870
Routa, J., Kolström, M., and Sikanen, L. (2018). Dry matter losses and their economic significance in forest energy procurement. Int. J. For. Eng. 29, 53–62. doi: 10.1080/14942119.2018.1421332
Sluiter, A., Hames, B., Scarlata, C., Sluiter, J., and Templeton, D. (2008). Determination of Ash in Biomass. Golden, CO: National Renewable Energy Laboratory (NREL).
Therasme, O., Eisenbies, M. H., and Volk, T. A. (2019). Overhead protection increases fuel quality and natural drying of leaf-on woody biomass storage piles. Forests 10:390. doi: 10.3390/f10050390
Therasme, O., Volk, T. A., Cabrera, A. M., Eisenbies, M. H., and Amidon, T. E. (2018). Hot water extraction improves the characteristics of willow and sugar maple biomass with different amount of bark. Front. Energy Res. 6:93. doi: 10.3389/fenrg.2018.00093
Volk, T. A., Berguson, B., Daly, C., Halbleib, M. D., Miller, R., Rials, T. G., et al. (2018). Poplar and shrub willow energy crops in the United States: field trial results from the multiyear regional feedstock partnership and yield potential maps based on the PRISM-ELM model. GCB Bioenerg. 10, 735–751. doi: 10.1111/gcbb.12498
Volk, T. A., Heavey, J. P., and Eisenbies, M. H. (2016). Advances in shrub-willow crops for bioenergy, renewable products, and environmental benefits. Food Energy Secur. 5, 97–106. doi: 10.1002/fes3.82
Whittaker, C., Yates, N. E., Powers, S. J., Misselbrook, T., and Shield, I. (2016). Dry matter losses and greenhouse gas emissions from outside storage of short rotation coppice willow chip. BioEnergy Res. 9, 288–302. doi: 10.1007/s12155-015-9686-y
Whittaker, C., Yates, N. E., Powers, S. J., Misselbrook, T., and Shield, I. (2018). Dry matter losses and quality changes during short rotation coppice willow storage in chip or rod form. Biomass Bioenerg. 112, 29–36. doi: 10.1016/j.biombioe.2018.02.005
Keywords: willow biomass, hot water extraction, bioenergy, storage, dry matter loss, fuel quality
Citation: Therasme O, Volk TA, Eisenbies MH, San H and Usman N (2020) Hot Water Extracted and Non-extracted Willow Biomass Storage Performance: Fuel Quality Changes and Dry Matter Losses. Front. Energy Res. 7:165. doi: 10.3389/fenrg.2019.00165
Received: 29 October 2019; Accepted: 23 December 2019;
Published: 21 January 2020.
Edited by:
Mukesh Kumar Awasthi, Northwest A&F University, ChinaReviewed by:
Surendra Sarsaiya, Zunyi Medical University, ChinaYu Min Duan, Northwest A&F University, China
Copyright © 2020 Therasme, Volk, Eisenbies, San and Usman. This is an open-access article distributed under the terms of the Creative Commons Attribution License (CC BY). The use, distribution or reproduction in other forums is permitted, provided the original author(s) and the copyright owner(s) are credited and that the original publication in this journal is cited, in accordance with accepted academic practice. No use, distribution or reproduction is permitted which does not comply with these terms.
*Correspondence: Obste Therasme, b3RoZXJhc20mI3gwMDA0MDtzeXIuZWR1
†ORCID: Timothy A. Volk orcid.org/0000-0002-6969-9281