- 1National Research Council, Washington, DC, United States
- 2American Society for Engineering Education, Washington, DC, United States
- 3Center for Biomolecular Science and Engineering, Naval Research Laboratory, Washington, DC, United States
The marine heterotroph, Marinobacter atlanticus strain CP1, was recently isolated from the electroautotrophic Biocathode MCL community, named for the three most abundant members: Marinobacter, an uncharacterized member of the Chromatiaceae, and Labrenzia. Biocathode MCL catalyzes the production of cathodic current coupled to carbon fixation through the activity of the uncharacterized Chromatiaceae, renamed as “Candidatus Tenderia electrophaga,” but the contribution of M. atlanticus is currently unknown. Here, we report on the electrochemical characterization of pure culture M. atlanticus biofilms grown under aerobic conditions and supplemented with succinate as a carbon source at applied potentials ranging from 160 to 510 mV vs. SHE, and on three different electrode materials (graphite, carbon cloth, and indium tin oxide). M. atlanticus was found to produce either cathodic or anodic current that was an order of magnitude lower than that of the Biocathode MCL community depending on the oxygen concentration, applied potential, and electrode material. Cyclic voltammetry, differential pulse voltammetry (DPV), and square wave voltammetry (SWV) were performed to characterize putative redox mediators at the electrode surface; however no definitive redox peaks were observed. No effect on current was observed when genes encoding a putative rubredoxin (ACP86_RS07295), as well as a putative NADH:flavorubredoxin oxidoreductase (ACP86_RS07290), were deleted to evaluate their role in EET. The addition of either riboflavin or excess trace mineral solution increased anodic current by ca. an order of magnitude under the conditions in which Biocathode MCL is typically grown. These results indicate that M. atlanticus has a non-negligible ability to utilize electrodes as an electron acceptor, which can be enhanced by the presence of excess trace minerals already available in the growth medium. The ability of M. atlanticus to utilize trace minerals as electron shuttles with extracellular electron acceptors may have broader implications for its natural role in biogeochemical cycling.
Introduction
Marinobacter spp. are considered “biogeochemical opportunitrophs” due to their metabolic flexibility (Singer et al., 2011), diverse substrate utilization, and wide geographic range including hydrocarbon-contaminated water (Gauthier et al., 1992), saline soil (Martin et al., 2003), and hot springs (Shieh et al., 2003). Based on their prevalence in subsurface environments (Edwards et al., 2003), demonstrated ability to oxidize iron (Edwards et al., 2003; Bonis and Gralnick, 2015), and identification in biocathode microbial communities (Erable et al., 2010; Debuy et al., 2015; Rowe et al., 2015; Wang et al., 2015b; Stepanov et al., 2016), Marinobacter spp. are thought to perform extracellular electron transfer (EET), however, no mechanism has been identified.
Marinobacter atlanticus strain CP1 was recently isolated from the Biocathode MCL community, named for the three most abundant members: Marinobacter, an uncharacterized member of the Chromatiaceae, and Labrenzia (Wang et al., 2015b; Bird et al., 2018). The Biocathode MCL community was originally enriched from seawater at 310 mV vs. SHE and is proposed to catalyze electron transfer from an electrode to oxygen and carbon dioxide for growth due to the activity of the uncharacterized Chromatiaceae [renamed “Candidatus Tenderia electrophaga” (Eddie et al., 2016)]. “Ca. Tenderia electrophaga” is an electroautotroph that cannot currently be cultivated in isolation and expresses proteins required for CO2 fixation through the Calvin-Benson-Bassham cycle. Marinobacter and Labrenzia are heterotrophs (unable to fix CO2) and presumably receive fixed carbon through the activity of “Ca. Tenderia electrophaga.” Metagenomic and 16S rRNA gene sequencing have shown that the relative abundance of M. atlanticus was negatively correlated with cathodic current (Malanoski et al., 2018), and relative activity of M. atlanticus tracked with relative abundance in a complementary study (Eddie et al., 2016). In addition, preliminary testing of M. atlanticus grown under the same conditions as Biocathode MCL and supplemented with an organic carbon source indicated some capacity to generate anodic current (Wang et al., 2015b), possibly indicating a competing electron transfer (ET) process to that of “Ca. Tenderia electrophaga.”
In the present study, we build on these previous observations to confirm whether M. atlanticus is an electroactive organism in order to further understand its electrochemical contribution, if any, to Biocathode MCL. M. atlanticus bioelectrochemical systems (BES) were established using oxygenated artificial seawater medium under identical conditions to those used for Biocathode MCL, but were supplemented with succinate (26 mM) as a carbon source and electron donor. Current production was evaluated at four different applied potentials (160, 260, 310, and 510 mV vs. SHE) and on three different electrode materials commonly used in BES [carbon cloth, graphite blocks, and indium tin oxide (ITO)]. We hypothesized that M. atlanticus biofilms would produce anodic current from succinate oxidation at potentials sufficiently positive to act as an electron acceptor once oxygen became limiting. At more negative potentials, we hypothesized that M. atlanticus may behave as a biocathode in the presence of O2 based on the observation that this strain can oxidize iron. When configured as a biocathode, M. atlanticus still requires supplemental carbon to grow since it cannot fix CO2, therefore succinate was also provided here.
The genome of M. atlanticus does not contain large multi-heme c-type cytochromes, such as those known to facilitate EET in well-known electroactive (EA) organisms like Geobacter sulfurreducens (Otero et al., 2018) and Shewanella oneidensis MR1 (Coursolle et al., 2010). Features ascribed to an Fe–S4-containing protein were previously observed in the Biocathode MCL biofilm (Yates et al., 2016) and within the Biocathode MCL community M. atlanticus expresses the gene for rubredoxin (Eddie et al., 2017), a class of small Fe–S4 proteins commonly involved in electron transfer events (Zanello, 2013; Liu et al., 2014). While rubredoxin has not yet been identified as a component of an EET conduit, synthetic biofilms employing rubredoxin as the charge mediator are capable of electron transfer between an electrode and a multi-copper enzyme and achieved even higher current densities than corresponding systems using cytochrome c (Altamura et al., 2017); therefore, its function as an ET mediator in M. atlanticus was explored.
For both the wild type (WT) and mutant strains, either anodic or cathodic current was generated, depending on the electrode potential and material used. No difference in current production was observed between the two strains. The presence of protein or small molecule redox mediators was investigated using cyclic voltammetry (CV), square wave voltammetry (SWV), and differential pulse voltammetry (DPV). While no definitive features corresponding to endogenous redox-active species were observed, the addition of exogenous redox-active species, riboflavin or excess trace mineral solution, resulted in approximately an order of magnitude increase in current production. This result suggests that soluble trace redox mediators available in the medium can facilitate EET in M. atlanticus BES. The use of soluble redox mediators may represent a more general role for M. atlanticus in biogeochemical cycles in the environment.
Materials and Methods
Construction of In-frame Deletion Mutants
M. atlanticus CP1 was previously isolated by plating cells from the Biocathode MCL electrode biofilm and restreaking for pure colonies (Wang et al., 2015b). Following isolation, the strain was stored as a glycerol stock at −80 °C. To cultivate this strain, an aliquot of the glycerol stock was struck on a fresh BB agar plate [18.7 g of Difco Marine Broth (MB) 2216 (Becton Dickinson, Franklin Lakes, NJ, USA), 5.0 g of tryptone, 2.5 g of yeast extract, 5.0 g of sodium chloride and 15.0 g of agar per one liter], and a single colony selected to inoculate overnight cultures. In-frame deletions of the M. atlanticus gene for a putative rubredoxin (locus: ACP86_RS07295) and a putative NADH:flavorubredoxin oxidoreductase (locus: ACP86_RS07290) were generated by overlap PCR (Warrens et al., 1997) according to an approach previously described for M. atlanticus (Bird et al., 2018). Briefly, 500 bp DNA fragments upstream and downstream of the target gene open reading frame were amplified from M. atlanticus genomic DNA using the primer pairs a/b and c/d (Table S1), respectively. The two PCR products were annealed at their overlapping region and amplified using primers a and d. The resulting 1 kb PCR fragment was digested with BamHI and SacI and cloned into the plasmid pSMV3 (Saltikov and Newman, 2003), which contains the kanamycin resistance gene for selection following conjugation, and the sacB gene for counterselection. The resulting gene deletion plasmids, pZW1207 for the rubredoxin gene and pZW2018 for the oxidoreductase gene, were transformed into Escherichia coli DH5α λpir strain (UQ950) for maintenance. Following growth and plasmid isolation, pZW1207 and pZW2018 were transformed into E. coli strain WM3064 by electroporation. Conjugation was carried out as previously described (Bird et al., 2018). Briefly, the M. atlanticus recipient strain was grown in Marine Broth (MB) 2216 (Becton Dickinson, Franklin Lakes, NJ, USA) overnight at 30 °C with shaking while the WM3064 donor strain was grown in lysogeny broth (LB) (10.0 g of tryptone, 5.0 g of yeast extract, and 10.0 g of sodium chloride per liter) supplemented with 50 μg/mL kanamycin and 600 μM diaminopimelic acid at 37 °C for 5 h with shaking. 400 μL of each strain was mixed, pelleted, and resuspended in 400 μL LB prior to selection on MB agar plates supplemented with 50 μg/mL kanamycin. The in-frame deletion mutants for the rubredoxin gene and NADH:flavorubredoxin oxidoreductase gene (hereafter denoted as Δrub and Δroo, respectively, for convenience) were further counter-selected on MB agar plates supplemented with 6% sucrose and verified by PCR with primers a/d and internal primers e/f (Table S1).
Description of Bioelectrochemical System and Inoculation
All biofilm electrochemical experiments were performed in 250 mL (total volume) water-jacketed bioelectrochemical systems (BES) (Pine Instruments, Durham, NC) set up under aerobic conditions except where specifically noted below. Reactors contained a Ag/AgCl reference electrode (3 M KCl, Bioanalytical Systems, Inc.) and carbon cloth counter electrode (4 × 6 cm). All potentials reported here are vs. the standard hydrogen electrode (SHE; +210 mV vs. Ag/AgCl [3 M KCl]). Working electrodes were either 3 × 6 cm carbon cloth (Zoltek PX30 woven carbon fabric, PW06 weave, Zoltek Corporation), 2 × 2.5 cm ITO (Corning 1737, Delta Technologies, Limited), or 3.5 × 1 × 0.3 cm graphite block (grade AXF-5Q, POCO Graphite, Inc.). Prior to use, ITO electrodes were cleaned in concentrated sulfuric acid (Trammell et al., 2011), rinsed with ultrapure deionized water (Millipore-Sigma, Burlington, MA), sandwiched between two titanium washers using a titanium nut and bolt to connect the ITO to a titanium wire, and autoclaved dry. Graphite electrodes were prepared as previously described (Yates et al., 2016). Briefly, electrodes were gently sanded, sonicated, and soaked in 1 N HCl overnight. Graphite electrodes were then rinsed with ultrapure deionized water and allowed to soak in fresh ultrapure water until use. Carbon cloth electrodes were soaked overnight in 1 N HCl, then rinsed repeatedly until all HCl was removed. Working and counter electrodes were connected to a potentiostat via titanium wire and titanium nuts, bolts, and washers. Current densities were reported using the geometric surface areas of 36, 9.7, and 5 cm2 for carbon cloth, graphite, and ITO electrodes, respectively. Reactors contained ca. 200 mL artificial seawater (ASW) medium (Emerson and Floyd, 2005) (per 1 L: 27.50 g NaCl, 3.80 g MgCl2•6H2O, 6.78 g MgSO4•7H2O, 0.72 g KCl, 0.62 g NaHCO3, 2.79 g CaCl2•2H2O, 1.00 g NH4Cl, 0.05 g K2HPO4) supplemented with 7 g/L sodium succinate dibasic hexahydrate as a carbon source, and 1 mL/L Wolfe's Mineral Solution (per 1 L: 1.5 g nitrilotriacetic acid, 3.0 g MgSO4•7H2O, 0.5 g MnSO4•H2O, 1.0 g NaCl, 0.1 g FeSO4•7H2O, 0.1 g CoCl2•6H2O, 0.1 g CaCl2, 0.1 g ZnSO4•7H2O, 0.01 g CuSO4•5H2O, 0.01 g AlK(SO4)2•12H2O, 0.01g H3BO3, 0.01 Na2MoO4•2H2O). The medium was brought to a final pH of 6.5–6.8 with CO2. BES temperature was maintained at 30 °C using a Haake AC200 (Thermo Scientific) recirculating water bath with continuous stirring (200–250 rpm).
A single colony of M. atlanticus WT, Δrub M. atlanticus, or Δroo M. atlanticus was used to inoculate a 3 mL culture of low calcium artificial seawater (lcASW) medium (Bird et al., 2018) (same as ASW except with a reduced concentration of calcium chloride, 0.05 g/L CaCl2•2H2O, to promote planktonic growth) supplemented with 7 g/L sodium succinate dibasic hexahydrate (carbon source and electron donor) and 1 mL/L Wolfe's Mineral Solution. The resulting culture was allowed to grow aerobically for ca. 40 h which resulted in an optical density at a wavelength of 600 nm (OD600) of ca. 0.9 for all strains. Approximately 1 mL was used to inoculate each BES, where the inoculum was normalized such that all BES received the same number of cells.
Electrochemical Measurements
Working electrodes (carbon cloth, graphite block, or ITO) were poised at 160, 260, 310, or 510 mV vs. standard hydrogen electrode (SHE) and chronoamperometry was recorded on a multichannel potentiostat (Solartron 1470E) under software control (Multistat; Scribner). Prior to inoculation, abiotic current was measured in all BES until it became steady; that is, it no longer increased in either the anodic or cathodic direction (3–24 h). Chronoamperometry is presented as the average of triplicate reactors overlaying the spread of high and low current.
For chronoamperometry of WT M. atlanticus reported in Figure 1, triplicate BES were measured at each potential. Following chronoamperometry, biofilm samples were preserved in RNAlater (ThermoFisher Scientific, USA) for future analysis. Laser scanning confocal microscopy (LSCM) of WT M. atlanticus biofilms reported in Figure 3 were obtained from a separate, independent set of BES grown on carbon cloth electrodes at all potentials. For comparison between WT and Δrub as shown in Figure 5, a separate independent set of triplicate BES were grown on carbon cloth electrodes at each potential and biofilm samples were again preserved in RNAlater (ThermoFisher Scientific, USA) for future analysis. Separate, independent BES were grown for SWV and DPV as described in the Supplementary Materials. For comparison of electrode materials, triplicate BES containing WT M. atlanticus were grown at 310 mV vs. SHE. At the conclusion of the experiment, biofilm samples were taken for LSCM or for cyclic voltammetry (CV) (Figure S3).
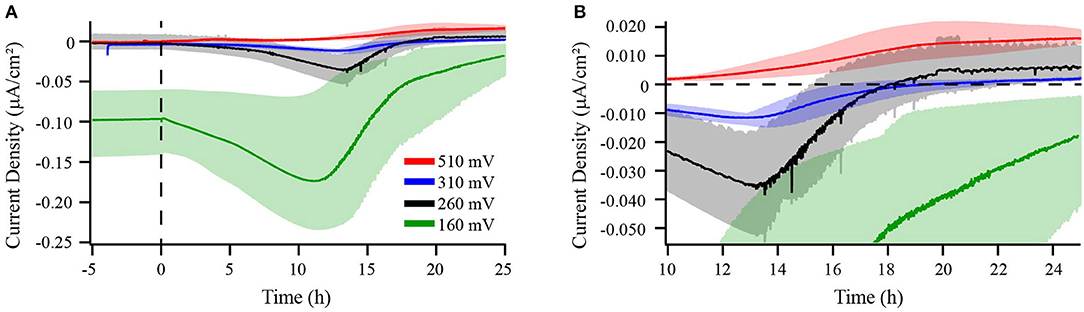
Figure 1. Chronoamperometry of M. atlanticus biofilms grown at 510 (red), 310 (blue), 260 (black), and 160 (green) mV vs. SHE. Current produced by both abiotic and biotic BES is shown in panel (A). Panel (B) shows a zoomed-in view of current production where 0 μA/cm2 is indicated by a dashed line. Bioelectrochemical systems (BES) equipped with carbon cloth working and counter electrodes were inoculated with wild type (WT) M. atlanticus and current was monitored over time. Solid lines represent an average of triplicate measurements and shaded areas represent the maximum and minimum values obtained.
Dissolved Oxygen Measurements
A NeoFox (Ocean Optics, Largo, FL, USA) small footprint phase fluorometer was coupled with the Foxy R-series oxygen sensor probe (Ocean Optics, Largo, FL, USA) and an O-series non-linear probe assembly for temperature correction (Ocean Optics, Largo, FL, USA). Oxygen sensors were factory calibrated and O2 concentration was recorded per the manufacturer's instructions. The oxygen sensor probe was sterilized using a 10% bleach solution and inserted through a septum into the BES. Oxygen measurements were recorded in two of the triplicate reactors grown on carbon cloth electrodes at 310 mV as described above.
Laser Scanning Confocal Microscopy
Electrodes for microscopy were collected at the conclusion of chronoamperometry measurements as noted above and submerged in Live/Dead staining solution [1 μL/mL 5 mM SYTO9 and 1.5 μL/mL 20 mM propidium iodide in ASW medium] for 30 min in the dark at room temperature, rinsed once with ASW medium, and then placed into a Nunc Lab-tek #1.0 borosilicate chambered cover glass (Thermo Fisher Scientific, Rochester, NY, USA). Fluorescence images of unfixed, stained cells from each electrode sample were acquired with a Nikon A1RSi confocal microscope using a CFI Plan Apochromat Lamba 20X, 0.75 numerical aperture objective over 184.32 μm to 245.76 μm square fields of view (0.18–0.24 μm/px) with 0.95–1.1 μm Z-step intervals. Pinhole widths varied from 20.43 to 26.82 μm depending upon the thickness of the Z-stack images for resolution of voxels deeper within the optical sections. SYTO9 and PI fluorescence spectra were excited with the LU-N4 laser unit at 489.4 nm (SYTO9) and 561.5 nm (propidium iodide). Emission data were collected using 525/50 (SYTO9) and 595/50 (PI) filters and detected with the DU4 GaAsP PMT detector. The images were recorded and processed into maximum intensity projections using identical settings with the NIS Elements software (Nikon Instruments, Melville, NY, USA). Three random fields of view were imaged from one electrode for each potential or electrode material and representative images are shown.
Addition of Electron Transfer Mediators
A third set of independent M. atlanticus electrode biofilms were grown as described above on carbon cloth electrodes at 310 mV vs. SHE. Riboflavin (3.5 μM, n = 3) or sterile-filtered excess trace minerals [1 mL of Wolfe's Mineral Solution (5X), n = 2] were added to either abiotic BES after 2.5–5 h, or to M. atlanticus electrode biofilms after ca. 40 h and chronoamperometry was recorded.
Results
Electrochemical Characterization of Wild Type Marinobacter atlanticus Grown on Carbon Cloth Electrodes
The electrochemical behavior of M. atlanticus was first characterized across a range of applied potentials (160, 260, 310, or 510 mV) for 25 h on carbon cloth electrodes in oxygenated artificial seawater medium where succinate (26 mM) was provided as both the electron donor and carbon source (M. atlanticus does not fix CO2) and O2 served as the electron acceptor in sealed BES. The 25 h experimental time frame was selected in order to (1) capture images of the biofilm that would reveal the morphology shortly after reaching peak current, and (2) save biofilms for future transcriptomics studies before cells entered into stationary phase where current no longer increased.
Carbon cloth electrodes were initially selected because they are commonly employed in BES and typically used by our lab to cultivate Biocathode MCL at 310 mV. The most positive potential of 510 mV was selected based on the typical applied potential for anode experiments by our group using G. sulfurreducens (Strycharz-Glaven and Tender, 2012). The potentials of 260 and 160 mV were selected to allow for characterization at more negative potentials while avoiding the onset of abiotic oxygen reduction observed in our system at ca. 150 mV. Prior to inoculation, abiotic current resulting from electrode interactions with the medium was measured until the current became steady (3–24 h) and is reported as negative time in Figures 1, 4, 5. Dashed lines represent the time point at which BES were inoculated. When biofilms were grown at 160, 260, or 310 mV, cathodic (negative) current immediately began to increase (Figure 1 and Figure S1). After 12–14 h, cathodic current reached a maximum and began to decrease. This transition occurred when the O2 concentration in the bulk medium of a BES with the electrode poised at 310 mV decreased to ca. 12% (Figure 2). Cathodic current is not observed in the absence of cells suggesting that it may be the result of biofilm-mediated electron transfer from the electrode to O2 which cannot be sustained as the surface environment of the electrode becomes anoxic due to increasing biomass from both electrode-attached and planktonic cell growth as described below. Biofilms in BES with electrodes at 260 and 310 mV generated anodic current after 16–18 h, once the bulk O2 concentration in the 310 mV reactor had decreased to ca. 4%. After approximately 20 h, anodic current production slowed, but remained steady, suggesting ET continues between the biofilm and electrode. Biofilms grown on electrodes at 160 mV never produced anodic current, indicating that this potential is too negative to act as an electron acceptor for the biofilm. M. atlanticus biofilms grown at 510 mV exclusively produced anodic current which increased steadily within the first 12 h after inoculation, followed by a more rapid increase after 12 h, corresponding to when O2 becomes limiting in the reactor (Figures 1, 2 and Figure S1). Similarly to biofilm electrodes poised at 260 and 310 mV, the anodic current began to level off around 20 h after which it increased more slowly. The coulombic efficiency estimated for complete oxidation of succinate (26 mM) in the highest anodic current-producing BES was 0.00064% (calculations available in Supplementary Materials). Loss of succinate in the BES due to anodic current would be in the nM range (total mol e- transferred/12 mol e- per mol succinate/volume of the BES), which would not be distinguishable from succinate oxidation coupled to oxygen reduction and therefore was not measured. M. atlanticus was not found to grow when a carbon cloth electrode poised at 310 or 510 mV was provided as the only available electron acceptor when the BES was made anoxic by sparging with CO2 and N2 prior to inoculation (data not shown). This finding, together with the observation that anodic current no longer increases once O2 is limiting, supports that idea that biofilm cells may access the electrode as O2 becomes limiting for maintenance, but not growth.
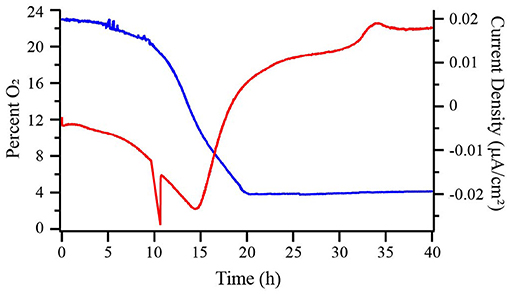
Figure 2. Percent dissolved oxygen (DO) in the bulk medium (blue) and chronoamperometry (red) of a wild type (WT) M. atlanticus bioelectrochemical system (BES) with a carbon cloth working electrode poised at 310 mV vs. SHE. Representative trace of duplicated experiments is shown.
Planktonic cell growth was observed in all BES due to the presence of succinate as the electron donor and carbon source and O2 as the electron acceptor. As has been previously demonstrated (Bird et al., 2018), planktonic growth was limited due to the concentration of calcium chloride in the artificial seawater medium. Final current was not correlated with the final planktonic OD600 of M. atlanticus (Table S2) indicating that planktonic cells do not make a significant contribution to current, and the effect of O2 limitation was not explored further. At all potentials, LSCM of M. atlanticus biofilms showed cells attached as a biofilm to the carbon cloth fibers (Figure 3). Biofilms grown at applied potentials of 260, 310, or 510 mV overwhelmingly stained green (Live/Dead) indicating that the cell membranes remain mostly intact. Qualitatively, biofilms grown at more negative electrode potentials contained a higher ratio of propidium iodide-stained cells (red), indicating compromised cell membranes which is often associated with cell death.
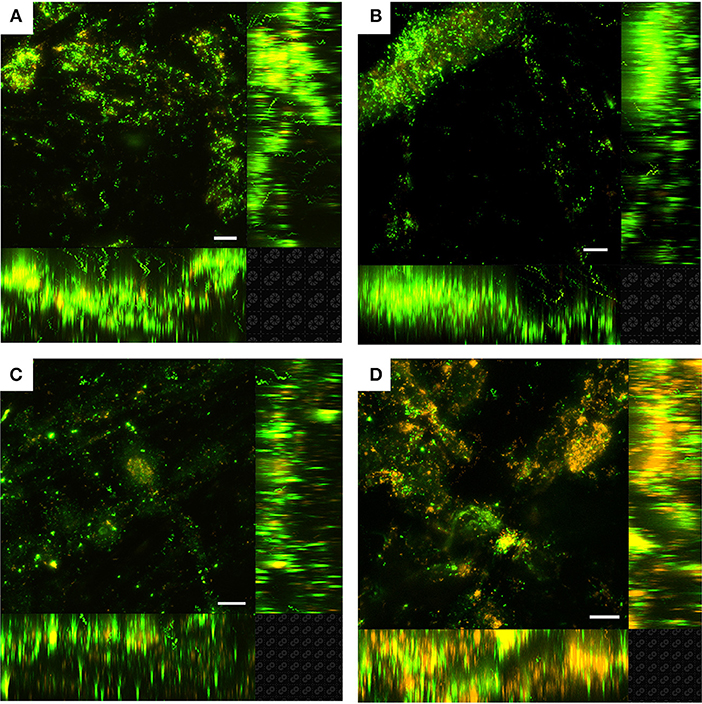
Figure 3. Laser scanning confocal microscopy (LSCM) of Live/Dead-stained M. atlanticus biofilms grown on carbon cloth electrodes poised at (A) 510 mV, (B) 310 mV, (C) 260 mV, and (D) 160 mV vs. SHE after ca. 25 h. Fluorescence associated with SYTO9 is shown in green (live) and fluorescence associated with propidium iodide is shown in red (dead). Representative images are shown from triplicate reactors. The white scale bar represents 20 μm.
To test whether observed current required M. atlanticus whole cells or cellular by-products only, sterile-filtered spent culture medium from Culture-grown cells was used to inoculate BES and current production monitored (Figure S2). No current production was observed above baseline abiotic current. Replacing the medium in M. atlanticus BES after maximum current was achieved had no effect on current (data not shown), indicating no contribution to current by planktonic cells.
Electrochemical Characterization of M. atlanticus on Different Electrode Materials Grown at 310 mV
In order to assess the electrochemical behavior of M. atlanticus across a range of materials commonly used in BES, chronoamperometry and cyclic voltammetry (CV) were performed on biofilms grown on carbon cloth, graphite block, and ITO electrodes at 310 mV. This electrode potential was selected because it is the potential at which Biocathode MCL was originally enriched and is typically used for Biocathode MCL electrochemical characterization. For both carbon cloth and graphite electrodes, anodic current increased over time (Figure 4A) in contrast to cathodic current observed for Biocathode MCL. The initial increase in cathodic current observed at 310 mV on carbon cloth electrodes (Figure 4A) was not observed on graphite block or ITO electrodes. Biofilms grown on ITO electrodes produced an order of magnitude less current than those on carbon cloth or graphite which never increased above the abiotic cathodic background and fewer cells were observed associated with the electrode surface (Figures 4B–D). Anodic current produced at 310 and 510 mV on graphite and carbon cloth electrodes was similar; however, it is difficult to assess whether biomass coverage on the electrode surface was comparable based on microscopy due to the 3-dimensional nature of the carbon cloth fibers. The majority of biofilm-associated cells stained green (Live/Dead) for all electrode materials, consistent with results presented above for biofilms grown at 310 mV and indicating living cells with intact cell membranes.
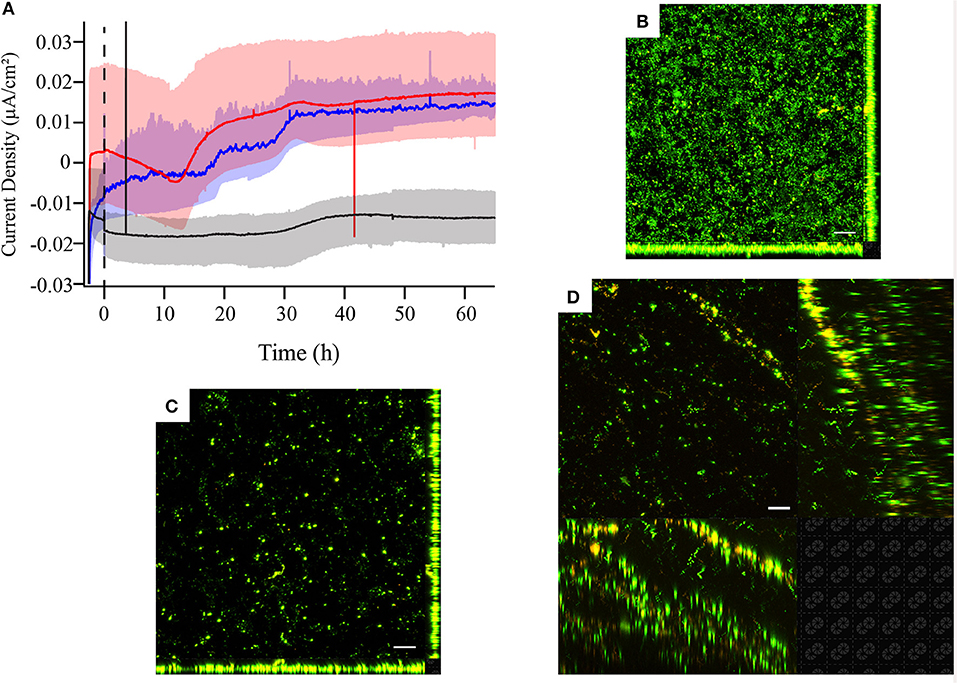
Figure 4. (A) Chronoamperometry and laser scanning confocal microscopy (LSCM) of Live/Dead-stained M. atlanticus biofilms grown on (B) graphite, (C) ITO, or (D) carbon cloth working electrodes poised at 310 mV vs. SHE. Microscopy was performed ca. 66 h after inoculation. Fluorescence associated with SYTO9 is shown in green (live) and fluorescence associated with propidium iodide is shown in red (dead). Representative images are shown from triplicate reactors. The white scale bar represents 20 μm.
In order to characterize putative redox mediators associated with M. atlanticus, CV was performed on abiotic and M. atlanticus-colonized carbon cloth, graphite block, and ITO electrodes (Figure S3). The appearance of redox peaks in the presence of cells was inconsistent between electrode materials and could not be distinguished from the abiotic controls. SWV (Figure S4) and DPV (Figure S5) were also applied in an attempt to further resolve redox peaks from the faradaic current by excluding the capacitive charging currents arising from the electrode; however, no consistent peak could be resolved.
Electrochemical Characterization of Wild Type and Rubredoxin Deletion Strains of M. atlanticus
To explore the possibility that small Fe–S4 proteins play a role in either EET or the survival of M. atlanticus as an electrode biofilm, biofilms of the Δrub M. atlanticus and Δroo M. atlanticus strains were electrochemically characterized vs. the WT strain on carbon cloth electrodes. No difference in the overall current trend was observed over 25 h between the Δrub strain and the WT strain at any potential (Figure 5), or the Δroo strain and the WT strain at 310 mV (Figures S1, S6). At the end of the 25–30 h growth period, OD600 was measured, found to be similar between reactor conditions, and was not correlated with current production (Table S2).
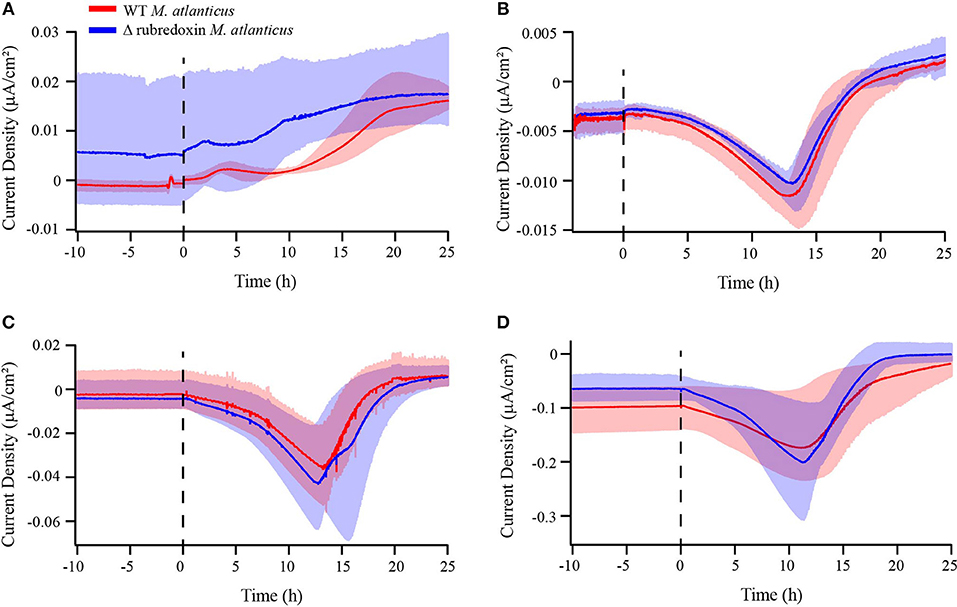
Figure 5. Chronoamperometry of wild type M. atlanticus (red) and rubredoxin deletion (Δrub) (blue) biofilms on carbon cloth working electrodes poised at (A) 510, (B) 310, (C) 260, and (D) 160 mV vs. SHE. The dashed line indicates the time of inoculation. Solid lines represent an average of triplicate measurements and shaded areas represent the maximum and minimum values obtained.
Addition of Exogenous Mediators Enhances Anodic Current in M. atlanticus Biofilms
The low current density and absence of redox features in voltammetry may indicate low abundance and/or low rate constants of redox mediators involved in the heterogeneous ET reaction, such as trace metals and minerals or small molecules found in the reactor medium. To test this possibility, a 5X higher concentration of trace mineral mixture was added to BES after WT M. atlanticus biofilms grown at 310 mV on carbon cloth electrodes reached maximum current (Figure 6). Current immediately began to increase and, after ca. 25 h, was an order of magnitude greater than the current produced prior to the addition of excess trace minerals. No change in the abiotic current was observed after adding excess minerals, either under aerobic conditions, or when the BES was made anoxic to mimic the conditions at the electrode surface once a biofilm is present. In all cases, the excess minerals were exposed to oxygen prior to addition to BES (Figure S7). When 5X trace mineral solution was added prior to inoculation, anodic current due to biofilm growth also increased to a maximum that was approximately an order of magnitude higher than is typically observed; however, current did not plateau until 60 h after inoculation (Figure S8D). Current was monitored for 40 h after the addition of excess minerals, at which point the experiment was discontinued and the working electrode of a representative BES that had 5X sterile-filtered trace minerals added was removed to test for cell viability by CLSM. Imaging by CLSM indicated that addition of trace minerals had some effect on cell membrane integrity as indicated by the increase in cells that stained red (Live/Dead) as compared to those observed for M. atlanticus grown at an applied potential of 310 mV under typical conditions (Figure S9). Qualitatively, the surface coverage of the electrode biofilms appears similar to those where no excess minerals were added, which might indicate the cells are limited in their use of the electrode as an electron acceptor by an electron shuttle. When CV was performed after the addition of minerals, a redox peak with a midpoint potential of ca. 180–200 mV was observed associated with M. atlanticus biofilms as well in abiotic BES under anaerobic conditions (Figure S10). Due to oxygen reduction features, it is unknown if this peak is also present in abiotic BES under aerobic conditions. The observation of this peak may be due to a change in the redox chemistry of charge carriers under anaerobic conditions, such as when a biofilm is present, as well as an increase in their concentration.
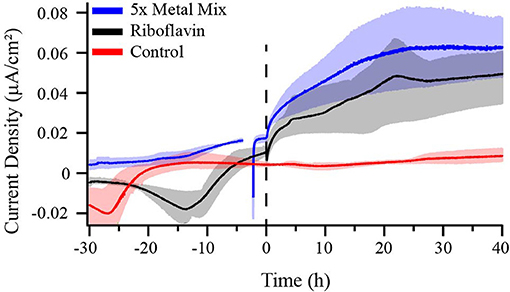
Figure 6. Chronoamperometry of M. atlanticus biofilms grown on carbon cloth electrodes poised at 310 mV vs. SHE with either no additions to the growth medium (red), 1 mL of sterile-filtered Wolfe's Mineral Solution added after ca. 48 h post inoculation (blue), or 3.5 μM riboflavin (final concentration) added after ca. 30 h post inoculation (black). Solid lines represent an average of triplicate (control and riboflavin addition) or duplicate (mineral mix addition) bioelectrochemical systems (BES) and the shaded areas represent the maximum and minimum values obtained.
Some iron-reducing bacteria, such as S. oneidensis MR-1 (Kotloski and Gralnick, 2013), access extracellular electron acceptors, including electrodes, via secreted small molecule redox shuttles including flavins. Flavins can interact with ET proteins in the periplasm or inner membrane of the cell, if such proteins are present, and facilitate EET. It has previously been shown that E. coli, which does not naturally participate in EET, is only able to use riboflavin as a shuttle when genetically engineered to express the S. oneidensis Mtr pathway (Jensen et al., 2016). In order to determine whether M. atlanticus can naturally use soluble redox shuttles for EET, riboflavin (3.5 μM) was added to BES at a concentration similar to that previously shown to stimulate EET in engineered E. coli. Addition of riboflavin resulted in roughly an order of magnitude increase in current (Figure 6). In comparison, when the same amount of riboflavin was added to BES containing WT E. coli modified electrodes (OD600 >0.600), no increase in current was observed (Figure S11), demonstrating that M. atlanticus has some innate capacity to perform EET with redox shuttles.
Discussion
The aim of this study was to electrochemically characterize M. atlanticus, recently isolated from the Biocathode MCL community. The relative abundance of M. atlanticus within Biocathode MCL has been shown to be negatively correlated with current, and previous work suggests that this strain has some capacity for EET on its own (Wang et al., 2015b; Bird et al., 2018); however no mechanism has been proposed. Results presented here show that M. atlanticus biofilms grown over a range of electrode potentials and materials resulted in the production of very low, but non-negligible, anodic and cathodic current which depended both on the electrode potential and concentration of O2. Cathodic current generated in the presence of O2 by M. atlanticus biofilms grown on carbon cloth at 310 mV was at least an order of magnitude lower than comparable Biocathode MCL biofilms grown at the same potential (Malanoski et al., 2018), and anodic current was 4 orders of magnitude lower than typical G. sulfurreducens bioanodes (Strycharz-Glaven and Tender, 2012), which would be expected if EET is not tied directly to the energy-generating processes of the cell. The production of cathodic current, even in the presence of the electron donor succinate, suggests that M. atlanticus could scavenge additional electrons from the electrode. Voltammetric analyses did not reveal any clear biofilm-associated redox peaks, differing markedly from the Nernstian current-voltage response observed during CV of Biocathode MCL (Yates et al., 2016). One explanation, inferred from the very low current, is that the redox signal associated with a redox mediator, either cell-associated or a component of the growth medium, is simply too low to detect.
Biofilms grown on carbon cloth electrodes at 260, 310, or 510 mV vs. SHE produced anodic current after a period of 12–18 h, consistent with a decrease in the concentration of O2 measured for BES at 310 mV. These results suggest that at more positive electrode potentials, M. atlanticus is able to use the electrode as an electron acceptor to relieve the buildup of reducing equivalents generated from succinate oxidation once O2 has been depleted. The absence of anodic current at 160 mV on carbon cloth electrodes, coupled with the noticeable increase in the number of propidium iodide-stained cells, supports the hypothesis that this potential is too negative for the electrode to act as a suitable electron acceptor. M. atlanticus biofilms achieve the highest anodic current density at 510 mV, whereas anodic current generated at 260 and 310 mV is about the same. Despite the ability of biofilms to transfer some electrons to an electrode, M. atlanticus was unable to grow under anaerobic conditions when the electrode was provided as the sole electron acceptor at 310 or 510 mV. This result is consistent with our previous observation that M. atlanticus is able to reduce small amounts of iron under anaerobic conditions in ASW medium, even though this metabolism does not appear to support growth (Bird et al., 2018). No difference in maximum anodic current was observed between the WT strain and Δrub and Δroo strains of M. atlanticus, indicating that neither of these proteins are involved in EET to an electrode. While there is a second rubredoxin present in the M. atlanticus genome, due to the degree of sequence similarity with AlkG of Pseudomonas putida it is likely to be part of the alkane degradation pathway (Kok et al., 1989; Wang et al., 2015a).
Electrochemical characterization of M. atlanticus biofilms on carbon cloth, graphite block, and ITO electrode surfaces showed that the electrode material has an effect on the maximum current production as well as whether biofilms initially behave as biocathodes at 310 mV. Graphite block electrodes resulted in the greatest anodic current density, followed by carbon cloth, then ITO. Some of this difference may be attributed to inaccuracies in calculated current density by using the geometric surface area, where ITO is a much smoother surface than carbon cloth or graphite. In addition, micro-features associated with the electrode topography may result in different chemical gradients (O2 or pH) at the electrode surface that affect current. The extent of M. atlanticus colonization, as measured by LSCM of Live/Dead-stained biofilms grown on these electrode surfaces, showed a robust biofilm on the graphite electrode, a fairly even biofilm coating on the carbon cloth fibers, and a sparse biofilm on the ITO electrodes. The biofilm coverage on these electrode surfaces agrees well with the overall current density produced in each case. These results suggest that current production is linked to the presence of metabolically active cells.
It is unclear why cathodic current generated by M. atlanticus biofilms at 310 mV only occurs on carbon cloth. The weave of the carbon cloth itself may contribute to this phenomenon if dissolved O2 is retained among the fibers, providing an electron acceptor that can be slowly released to the growing biofilm. Carbon cloth is a widely used electrode surface in microbial electrochemistry; however, the manufacturing process of carbon cloth only results in 99% carbon atoms (Zoltek Corporation) and surface modifications may be added to improve various properties. It is unknown whether such surface modifications may have an effect here.
The most significant observation was that the addition of excess trace minerals (5X) or riboflavin (3.5 μM) resulted in an order of magnitude increase in anodic current production at 310 mV on carbon cloth electrodes, the same conditions under which Biocathode MCL is typically grown. Conversely, the addition of riboflavin to E. coli biofilms resulted in no change in current production. It has previously been reported that the addition of nanomolar concentrations of Cu2+ or Cd2+ to S. oneidensis MR-1 grown in microbial fuel cells resulted in a 1.3-fold increase in power density due to the combined effects of increased biofilm formation and increased riboflavin production (Xu et al., 2016). M. atlanticus possesses the required genes for riboflavin biosynthesis (Wang et al., 2015a), but not for the riboflavin exporter found in S. oneidensis; therefore, it seems unlikely that a similar mechanism is responsible in this case.
The presence of cells was required for the observed increase in anodic current with the addition of either riboflavin or excess trace minerals, suggesting that sustained turnover of these redox shuttles is catalyzed by the cells at 310 mV. The addition of excess minerals in the presence of the biofilm or when the medium was made anaerobic in abiotic reactors resulted in a discernable redox peak with a midpoint potential (Em) around 180–200 mV. This peak is likely a component of the trace mineral solution and may be available to the biofilm as a redox mediator. If this is the case, the Em offers insight into biofilm current production at the various potentials tested here. At potentials above 200 mV, the unknown redox-active species may be partially oxidized by the electrode, and thus could serve as an electron-accepting shuttle between the cell and electrode. Below 200 mV, a higher proportion of the redox mediator would be maintained in the reduced state by the electrode and unable to accept electrons from cells. This is consistent with anodic current at potentials of 260 mV and above, and cathodic current only at 160 mV. Drawing from these results, we can imagine a scenario under which trace minerals serve as electron transfer mediators within the Biocathode MCL community between the electrode and heterotrophic constituents, contributing to the overall electron flux of the biofilm. Depending on the direction of electron flux at 310 mV generated by M. atlanticus, anodic or cathodic, this contribution may compete with, or enhance, cathodic ET by “Ca. Tenderia electrophaga.” If other heterotrophic constituents of Biocathode MCL also participate in mineral cycling, this effect could be amplified. The low availability of organic carbon in Biocathode MCL reactors (no organic carbon added), as well as microaerobic conditions at the electrode surface, limits heterotrophic growth. If mineral cycling can provide additional electron donors or acceptors it may explain the persistence of heterotrophic community members in Biocathode MCL even after many years of subculture. This finding may also have important implications for how Marinobacter spp. interact with minerals in the environment and should be further explored. For example, Marinobacter spp. may be able to take up oxidized or reduced trace minerals allowing for sustained cell maintenance during periods of electron donor or acceptor limitation.
In the BES configuration used in this study, it is not possible to determine if cells within the biofilm, but at a distance from the electrode surface, contribute to current as they do for conductive G. sulfurreducens biofilms. Because this system is not intentionally made anaerobic, cells at the periphery of the biofilm may utilize O2 as an electron acceptor, creating an anoxic environment near the electrode surface. LSCM imaging did not indicate the cells at the outer portion of the biofilm were compromised once O2 is depleted, suggesting that some other electron acceptor is available. Whether this is some oxidized component of the medium or other cells within the biofilm remains unclear and measurements of biofilm conductivity are warranted.
Conclusion
Marinobacter spp. are ubiquitous marine opportunitrophs identified in metal-rich environments and within electrode-associated biofilms. Here we demonstrated that M. atlanticus is able to generate very low levels of anodic and cathodic current, an indicator of its ability to perform EET outside of the Biocathode MCL community. Constituents of electrochemically active microbial communities that produce low levels of current on their own should not be discounted and may have important roles for the function of the community as a whole (Doyle and Marsili, 2018). Anodic current can be enhanced at 310 mV through addition of riboflavin and excess minerals. These findings have important implications for how Marinobacter spp. may interact with minerals in the environment and suggests that trace minerals could be scavenged for use as electron donors or acceptors under otherwise limiting conditions.
With respect to the role for M. atlanticus within Biocathode MCL, results presented here indicate the possibility that competing ET processes to “Ca. Tenderia electrophaga” occur at a poised electrode potential of 310 mV vs. SHE depending on the concentration of O2 present. Anodic current produced by M. atlanticus at 310 mV is low relative to Biocathode MCL, but it is possible that a cumulative effect may occur if other heterotrophic members of the community are also able to produce anodic current.
Data Availability
All datasets generated for this study are included in the manuscript and/or the Supplementary Files.
Author Contributions
EO and SG devised experiments and wrote the manuscript. EO performed electrochemical measurements on M. atlanticus. DP and BE performed microscopy and imaging. MY and LT helped perform SWV and DPV measurements and analysis of electrochemical data. ZW generated the M. atlanticus deletion strains used in this study. This research was performed while the author EO held an NRC Research Associateship award at The Naval Research Laboratory.
Funding
This work was funded through the NRL Base 6.1 program and the Office of the Assistant Secretary of Defense for Research and Engineering Applied Research for the Advancement of S&T Priorities program.
Conflict of Interest Statement
The authors declare that the research was conducted in the absence of any commercial or financial relationships that could be construed as a potential conflict of interest.
Supplementary Material
The Supplementary Material for this article can be found online at: https://www.frontiersin.org/articles/10.3389/fenrg.2019.00060/full#supplementary-material
References
Altamura, L., Horvath, C., Rengaraj, S., Rongier, A., Elouarzaki, K., Gondran, C., et al. (2017). A synthetic redox biofilm made from metalloprotein-prion domain chimera nanowires. Nat.Chem. 9, 157–163. doi: 10.1038/nchem.2616
Bird, L. J., Wang, Z., Malanoski, A. P., Onderko, E. L., Johnson, B. J., Moore, M. H., et al. (2018). Development of a genetic system for Marinobacter atlanticus CP1 (sp. nov.), a Wax Ester producing strain isolated from an autotrophic biocathode. Front. Microbiol. 9:03176. doi: 10.3389/fmicb.2018.03176
Bonis, B. M., and Gralnick, J. A. (2015). Marinobacter subterrani, a genetically tractable neutrophilic Fe(II)-oxidizing strain isolated from the Soudan Iron Mine. Front. Microbiol. 6:719. doi: 10.3389/fmicb.2015.00719
Coursolle, D., Baron, D. B., Bond, D. R., and Gralnick, J. A. (2010). The Mtr respiratory pathway is essential for reducing flavins and electrodes in Shewanella oneidensis. J. Bacteriol. 192, 467–474. doi: 10.1128/JB.00925-09
Debuy, S., Pecastaings, S., Bergel, A., and Erable, B. (2015). Oxygen-reducing biocathodes designed with pure cultures of microbial strains isolated from seawater biofilms. Int. Biodeterior. Biodegr. 103, 16–22. doi: 10.1016/j.ibiod.2015.03.028
Doyle, L. E., and Marsili, E. (2018). Weak electricigens: a new avenue for bioelectrochemical research. Bioresour. Technol. 258, 354–364. doi: 10.1016/j.biortech.2018.02.073
Eddie, B. J., Wang, Z., Hervey, W. J., Leary, D. H., Malanoski, A. P., Tender, L. M., et al. (2017). Metatranscriptomics supports the mechanism for biocathode electroautotrophy by “Candidatus Tenderia electrophaga”. Msystems 2. doi: 10.1128/mSystems.00002-17
Eddie, B. J., Wang, Z., Malanoski, A. P., Hall, R. J., Oh, S. D., Heiner, C., et al. (2016). ‘Candidatus Tenderia electrophaga', an uncultivated electroautotroph from a biocathode enrichment. Int. J. Syst. Evol. Microbiol. 66, 2178–2185. doi: 10.1099/ijsem.0.001006
Edwards, K. J., Rogers, D. R., Wirsen, C. O., and Mccollom, T. M. (2003). Isolation and characterization of novel psychrophilic, neutrophilic, Fe-oxidizing, chemolithoautotrophic alpha- and, gamma-Proteobacteria from the deep sea. Appl. Environ. Microbiol. 69, 2906–2913. doi: 10.1128/AEM.69.5.2906-2913.2003
Emerson, D., and Floyd, M. M. (2005). Enrichment and isolation of iron-oxidizing bacteria at neutral pH. Environ. Microbiol. 397, 112–123. doi: 10.1016/S0076-6879(05)97006-7
Erable, B., Vandecandelaere, I., Faimali, M., Delia, M. L., Etcheverry, L., Vandamme, P., et al. (2010). Marine aerobic biofilm as biocathode catalyst. Bioelectrochemistry 78, 51–56. doi: 10.1016/j.bioelechem.2009.06.006
Gauthier, M. J., Lafay, B., Christen, R., Fernandez, L., Acquaviva, M., Bonin, P., et al. (1992). Marinobacter-hydrocarbonoclasticus gen. nov., sp. nov., a New, Extremely Halotolerant, Hydrocarbon-Degrading Marine Bacterium. Int. J. Syst. Bacteriol. 42, 568–576. doi: 10.1099/00207713-42-4-568
Jensen, H. M., Teravest, M. A., Kokish, M. G., and Ajo-Franklin, C. M. (2016). CymA and exogenous flavins improve extracellular electron transfer and couple it to cell growth in Mtr-expressing Escherichia coli. Acs Synthetic Biol. 5, 679–688. doi: 10.1021/acssynbio.5b00279
Kok, M., Oldenhuis, R., Vanderlinden, M. P. G., Meulenberg, C. H. C., Kingma, J., and Witholt, B. (1989). The Pseudomonas oleovorans alkBAC operon encodes two structurally related rubredoxins and an aldehyde dehydrogenase. J. Biol. Chem. 264, 5442–5451.
Kotloski, N. J., and Gralnick, J. A. (2013). Flavin electron shuttles dominate extracellular electron transfer by Shewanella oneidensis. Mbio 4. doi: 10.1128/mBio.00553-12
Liu, J., Chakraborty, S., Hosseinzadeh, P., Yu, Y., Tian, S. L., Petrik, I., et al. (2014). Metalloproteins containing cytochrome, iron-sulfur, or copper redox centers. Chem. Rev. 114, 4366–4469. doi: 10.1021/cr400479b
Malanoski, A. P., Lin, B. C., Eddie, B. J., Wang, Z., Hervey, W. J., and Glaven, S. M. (2018). Relative abundance of “Candidatus Tenderia electrophaga' is linked to cathodic current in an aerobic biocathode community. Microb. Biotech. 11, 98–111. doi: 10.1111/1751-7915.12757
Martin, S., Marquez, M. C., Sanchez-Porro, C., Mellado, E., Arahal, D. R., and Ventosa, A. (2003). Marinobacter lipolyticus sp nov., a novel moderate halophile with lipolytic activity. Int. J. Syst. Evol. Microbiol. 53, 1383–1387. doi: 10.1099/ijs.0.02528-0
Otero, F. J., Chan, C. H., and Bond, D. R. (2018). Identification of different putative outer membrane electron conduits necessary for Fe(III) Citrate, Fe(III) Oxide, Mn(IV) Oxide, or electrode reduction by geobacter sulfurreducens. J. Bacteriol. 200:e00347–18. doi: 10.1128/JB.00347-18
Rowe, A. R., Chellamuthu, P., Lam, B., Okamoto, A., and Nealson, K. H. (2015). Marine sediments microbes capable of electrode oxidation as a surrogate for lithotrophic insoluble substrate metabolism. Front. Microbiol. 5:784. doi: 10.3389/fmicb.2014.00784
Saltikov, C. W., and Newman, D. K. (2003). Genetic identification of a respiratory arsenate reductase. Proc. Natl. Acad. Sci. U. S. A. 100, 10983–10988. doi: 10.1073/pnas.1834303100
Shieh, W. Y., Jean, W. D., Lin, Y. T., and Tseng, M. (2003). Marinobacter lutaoensis sp nov., a thermotolerant marine bacterium isolated from a coastal hot spring in Lutao, Taiwan. Can. J. Microbiol. 49, 244–252. doi: 10.1139/w03-032
Singer, E., Webb, E. A., Nelson, W. C., Heidelberg, J. F., Ivanova, N., Pati, A., et al. (2011). Genomic potential of Marinobacter aquaeolei, a biogeochemical “Opportunitroph”. Appl. Environ. Microbiol. 77, 2763–2771. doi: 10.1128/AEM.01866-10
Stepanov, V. G., Xiao, Y., Lopez, A. J., Roberts, D. J., and Fox, G. E. (2016). Draft genome sequence of Marinobacter sp. strain P4B1, an electrogenic perchlorate-reducing strain isolated from a long-term mixed enrichment culture of marine bacteria. Genome Announcements 4:e01617–01615. doi: 10.1128/genomeA.01617-15
Strycharz-Glaven, S. M., and Tender, L. M. (2012). Study of the mechanism of catalytic activity of G. Sulfurreducens biofilm anodes during biofilm growth. Chemsuschem 5, 1106–1118. doi: 10.1002/cssc.201100737
Trammell, S. A., Dressick, W. J., Melde, B. J., and Moore, M. (2011). Photocurrents from the direct irradiation of a donor-acceptor complex contained in a thin film on indium tin oxide. J. Phys. Chem. C 115, 13446–13461. doi: 10.1021/jp2023988
Wang, Z., Eddie, B. J., Malanoski, A. P., Hervey, W. J., Lin, B., and Strycharz-Glaven, S. M. (2015a). Complete genome sequence of Marinobacter sp. CP1, isolated from a self-regenerating biocathode biofilm. Genome Announc. 3:e01103–01115. doi: 10.1128/genomeA.01103-15
Wang, Z., Leary, D. H., Malanoski, A. P., Li, R. W., Hervey, W. J., Eddie, B. J., et al. (2015b). A previously uncharacterized, nonphotosynthetic member of the chromatiaceae is the primary CO2-fixing constituent in a self-regenerating biocathode. Appl. Environ. Microbiol. 81, 699–712. doi: 10.1128/AEM.02947-14
Warrens, A. N., Jones, M. D., and Lechler, R. I. (1997). Splicing by overlap extension by PCR using asymmetric amplification: an improved technique for the generation of hybrid proteins of immunological interest. Gene 186, 29–35. doi: 10.1016/S0378-1119(96)00674-9
Xu, Y. S., Zheng, T., Yong, X. Y., Zhai, D. D., Si, R. W., Li, B., et al. (2016). Trace heavy metal ions promoted extracellular electron transfer and power generation by Shewanella in microbial fuel cells. Bioresour. Technol. 211, 542–547. doi: 10.1016/j.biortech.2016.03.144
Yates, M. D., Eddie, B. J., Kotloski, N. J., Lebedev, N., Malanoski, A. P., Lin, B. C., et al. (2016). Toward understanding long-distance extracellular electron transport in an electroautotrophic microbial community. Energy Environ. Sci. 9, 3544–3558. doi: 10.1039/C6EE02106A
Zanello, P. (2013). The competition between chemistry and biology in assembling iron-sulfur derivatives. Molecular structures and electrochemistry. Part I. {Fe(SγCγs4)} proteins. Coordination Chem. Rev. 257, 1777–1805. doi: 10.1016/j.ccr.2013.02.006
Zoltek Corporation. Technical Datasheet ZOLTEK™ PX30 Fabric (PW03, PW06, SW08) [Online]. ZOLTEK Corporation. Available online at: http://zoltek.com/datasheets/ (accessed March 15, 2019).
Keywords: marinobacter, bioelectrochemical system (BES), biofilm, microbial electrochemistry, mineral cycling
Citation: Onderko EL, Phillips DA, Eddie BJ, Yates MD, Wang Z, Tender LM and Glaven SM (2019) Electrochemical Characterization of Marinobacter atlanticus Strain CP1 Suggests a Role for Trace Minerals in Electrogenic Activity. Front. Energy Res. 7:60. doi: 10.3389/fenrg.2019.00060
Received: 15 March 2019; Accepted: 03 June 2019;
Published: 26 June 2019.
Edited by:
Subba Rao Chaganti, University of Windsor, CanadaReviewed by:
Feng Zhao, Institute of Urban Environment (CAS), ChinaFalk Harnisch, Helmholtz Centre for Environmental Research (UFZ), Germany
Copyright © 2019 Onderko, Phillips, Eddie, Yates, Wang, Tender and Glaven. This is an open-access article distributed under the terms of the Creative Commons Attribution License (CC BY). The use, distribution or reproduction in other forums is permitted, provided the original author(s) and the copyright owner(s) are credited and that the original publication in this journal is cited, in accordance with accepted academic practice. No use, distribution or reproduction is permitted which does not comply with these terms.
*Correspondence: Sarah M. Glaven, c2FyYWguZ2xhdmVuQG5ybC5uYXZ5Lm1pbA==