- 1Ecodynamics Group, Department of Earth, Environmental and Physical Sciences, University of Siena, Siena, Italy
- 2Department of Engineering, University of Campania “Luigi Vanvitelli,” Aversa, Italy
- 3Inter-University National Consortium for Marine Sciences (CoNISMa), Rome, Italy
- 4Stazione Zoologica Anton Dohrn, Naples, Italy
Overtopping breakwater systems are among the most promising technologies for exploiting wave energy to generate electricity. They consist in water reservoirs, embedded in piers, placed on top of ramps, higher than sea-level. Pushed by wave energy, seawater fills up the reservoirs and produces electricity by flowing back down through low headhydro turbines. Different overtopping breakwater systems have been tested worldwide in recent years. This study focuses on the Overtopping BReakwater for Energy Conversion (OBREC) system that has been implemented and tested in the harbor of Naples (Italy). The Life Cycle Assessment of a single replicable module of OBREC has been performed for analyzing potential environmental impacts, in terms of Greenhouse Gas Emissions, considering construction, installation, maintenance, and the operational phases. The Carbon Footprint (i.e., mass of CO2eq) to build wave energy converters integrated in breakwater systems has been estimated, more specifically the “environmental investment” (i.e., the share of Carbon Footprint due to the integration of wave energy converter) needed to generate renewable electricity has been assessed. The Carbon Intensity of Electricity (i.e., the ratio between the CO2eq emitted and the electricity produced) has been then assessed in order to demonstrate the profitability and the opportunity to foster innovation in the field of blue energy. Considering the impact for implementing an operational OBREC module (Carbon Footprint = 1.08 t CO2eq; Environmental Investment = 0.48 t CO2eq) and the electricity production (12.6 MWh/year per module), environmental benefits (avoided emissions) would compensate environmental costs (i.e., Carbon Footprint; Environmental Investment) those provided within a range of 25 and 13 months respectively.
Introduction
The International Energy Agency (IEA) estimates that in 2017 global energy demand increased by 2.1% compared to previous years and the 72% of that increase has been met by deploying fossil fuels (International Energy Agency, 2018a). The electricity demand has grown by 3.1%, considerably higher compared to the overall increase in the energy demand. This increased demand resulted in an intensification of the global energy-related CO2 emissions by 1.4% in 2017, reaching a historic peak value of 32.5 gigatonnes (Gt), a detour from the three past years in which global emissions remained flat (International Energy Agency, 2018a). However, renewable energies have met a quarter of the global energy demand growth (International Energy Agency, 2018a).
In order to accomplish the Paris Agreement (United Nations Framework Convention on Climate Change, 2015), the IEA flagship publication, World Energy Outlook 2017, foresees a “Sustainable Development Scenario” (among 240 energy mix scenarios in 2100) according to which a mixture of technologies is considered a prerequisite to meet climate objectives (International Energy Agency, 2017). In this line, the BP Energy Outlook 2018 foresees, in 2040, an extremely diversified world energy mix with a significant increase of renewable energy (BP energy Outlook, 2018). Moreover, International Renewable Energy Agency (2018) remarked that renewable energy combined with improved energy efficiency are the cornerstone of climate solution and, by 2050 the share of renewable energy in the European Union could grow from about 17% to over 70%.
According to the Intergovernmental Panel on Climate Change (2011), the use of renewable energy sources is the solution to avoid greenhouse gas emissions and it can help to increase energy security, allow energy independency of communities and decrease air, water and soil pollution (Ellabban et al., 2014; Magagna and Uihlein, 2015; Melikoglu, 2018; Şener et al., 2018).
Among renewable energy sources, ocean energy represents the most promising because of the impressive energy potential stored in oceans (International Renewable Energy Agency, 2014; Khan et al., 2017). Oceans represent the 70% of Earth surface (Ressurreiçao et al., 2011) and, capturing the sun's thermal energy, they can be considered as the largest solar collectors (Khan et al., 2017), besides tides driven by the gravitational pull of the moon and waves generated by the wind. Major advantages of ocean energies, compared to other renewables, comprise predictability (Yaakob et al., 2016), availability and abundance (Homma, 1985) as well as high load factor (Benbouzid et al., 2017). The theoretical energy potential from oceans has been estimated to be more than sufficient to cope present and projected global electricity request (International Renewable Energy Agency, 2014; Hussain et al., 2017). Estimation of this potential ranges from 20,000 to 800,000 TWh electricity a year (International Renewable Energy Agency, 2014) or between 4 and 18 million tons of oil equivalent (toe) (de Andres et al., 2017a,b).
Supporting the deployment of ocean technologies would result in the accomplishment of recommendations and directives of the European Union regarding the promotion of renewable energies (European Commission, 2009; Sannino and Cavicchioli, 2013) and referring to the target set for climate and energy policies for 2030 and 2050 (European Commission, 2013; Sannino and Cavicchioli, 2013) and the objectives of the marine spatial planning and integrated coastal management directive (European Commission, 2014).
Ocean energy concerns the energy sector included in the definition of Blue Economy (Union for Mediterranean, 2017). As recognized by the United Nations Environmental Programme (UNEP): “a worldwide transition to a low-carbon, resource-efficient Green Economy will not be possible unless the seas and oceans are a key part of these urgently needed transformations” (UNEP, 2012). Moreover, the sustainable development of ocean energy will contribute to pursue the Sustainable Development Goals (SDGs) (United Nations, 2015) and the Mediterranean Strategy for Sustainable Development (MSSD) (United Nation Environmental Programme/Mediterranean Action plan, 2016). In particular, the deployment of ocean energy would contribute to accomplish the target 7.2 of the SDG 7, requiring a substantial increase of the share of renewable energy in the energy mix, and the objectives 4 and 5 of the MSDD, aimed at fostering the transition toward green and blue economy. The United Nations have declared the Decade of Ocean Science for Sustainable Development (2021–2030) in order to enhance sustainable use of oceans and marine resources and support the development of ocean economy (United Nations Educational, 2018).
Ocean energy sources include salinity gradient, onshore and offshore wave energy, tidal and marine currents, ocean thermal energy, marine biomass, and offshore wind (both floating and stable) (Lewis et al., 2012; Borthwick, 2016; Hussain et al., 2017; Melikoglu, 2018). Ocean energy converters exploit these renewable sources to generate useful energy—commonly electricity (International Renewable Energy Agency, 2014). To date, wave and tidal energy converters are at the most advanced stage (Lewis et al., 2012; International Renewable Energy Agency, 2014; Uihlein and Magagna, 2016). Wave energy technology development started on 1940 in Japan through the work of Yoshio Masuada (Falcão, 2010) with a serious academic attention gained around early 1970s (International Renewable Energy Agency, 2014). However, the technology development and proliferation of full-scale prototypes occurred in the last decades (Cruz, 2007). According to Magagna and Uihlein (2016), due to their availability and affluence of resources, wave and tidal energy are likely to mark the most significant contribution to the electricity production mix in EU in the near future. It has also been recognized that, wave energy has the potential to compete with the current use of fossil fuels thanks to its availability and predictability (Alamian et al., 2017; Mustapa et al., 2017).
Wave Energy Converters (WEC) concern different technologies, the 82% of which refers to five types: point absorber, wave overtopping reservoir, attenuator, oscillating water column, and oscillating surge (or inverted pendulum) (International Renewable Energy Agency, 2014; Magagna and Uihlein, 2015). In most of the cases, wave energy is converted into electricity by means of two steps: wave energy is firstly converted into a simplified form of mechanical energy (purely potential or kinetic energy) and then, through a proper power take-off system (hydro turbine, hydraulic piston, etc.), into electrical energy (Kim et al., 2017). According to Lewis et al. (2012) more than 50 types of WEC have been conceived and are under development. However, due to the high cost only few technologies are ready for the commercial stage (Contestabile et al., 2017a). However, WECs look to be the most cost-effective systems among blue energy converters (Contestabile et al., 2017b).
As recognized by International Energy Agency (2018b), Environmental Impact Assessments (EIA) of ocean energy converters are necessary to inform regulators on potential impacts due to ocean energy deployment. It has also been highlighted the necessity of environmental monitoring plan before, during and after the installation in order to minimize risks (Copping et al., 2013; International Energy Agency, 2018b). Effects on benthic communities, species-specific response to habitat changes, entanglement of marine mammals, turtles, fish, and marine birds are examples of direct environmental impacts due to ocean energy technologies (Azzellino et al., 2011; Frid et al., 2012). Moreover, impacts due to building, operating, maintenance, decommissioning and disposal of ocean energy converters should be also considered (Sannino and Cavicchioli, 2013; Uihlein and Magagna, 2016). Life Cycle Assessment (LCA) is widely recognized as useful tool to evaluate environmental burdens of energy produced from different renewable and non-renewable sources (Sannino and Cavicchioli, 2013; Amponsah et al., 2014). To date, only a small number of LCA on ocean energy converters have been carried out (e.g., Sørensen et al., 2006; Parker et al., 2007; Rule et al., 2009; Walker and Howell, 2011; Banerjee et al., 2013; Douziech et al., 2016; Uihlein, 2016; Elginoz and Bas, 2017; López-Ruiz et al., 2018; Thomson et al., 2019) tackling different aspects, from eco-design to end-of life of plants and evaluating different potential impacts.
The Interreg Med MAESTRALE is a cooperation project, co-financed by the European Regional Development Fund, involving 11 partners from 8 European countries. It aims to investigate strengths, weaknesses, opportunities and threats of blue energy technologies in order to inform and support their deployment in the Mediterranean area. A survey of the most promising solutions developed in Europe is available in the MAESTRALE webgis (http://maestrale-webgis.unisi.it). Among available WEC technologies, OBREC (Overtopping BReakwater for Energy Conversion), installed in the harbor of Naples (Italy), is a full-scale WEC prototype integrated into an existing breakwater. It has been designed to capture overtopping waves and produce electricity in poor and mild wave climate (Contestabile et al., 2016, 2017a).
This paper presents results of an LCA applied to OBREC, in order to evaluate environmental impacts and benefits in terms of Carbon Footprint. The LCA has been carried out to provide a measure of environmental impacts of OBREC implementation in terms of greenhouse gas emission in a real environment: the harbor of Naples (Italy). Since OBREC is integrated in an already functioning harbor, we identify and calculate the environmental investment (in terms of CO2eq) of renewable electricity production to capture the contribution of the additional inputs required by that technology to obtain electricity from an unexploited energy (wave energy in this case). Besides impacts, this study also focuses on environmental benefits given by renewable energy production (i.e., variation of the Carbon Intensity of Electricity of the Italian electricity mix). The electricity production can be estimated in terms of avoided emissions. Moreover, assuming that one OBREC module can replace 3–4 rows of two layers of antifers from the breakwater, the environmental cost-benefit balance concerns the environmental investment required to implement OBREC in place of antifers.
Materials and Methods
Overtopping BReakwater for Energy Conversion (OBREC)
The Overtopping BReakwater for Energy Conversion, namely OBREC, is a system completely embedded in a rubble mound breakwater designed to exploit wave energy potentials. It converts the wave overtopping process into potential energy by collecting seawater, pushed through a frontal ramp, in upper reservoirs to feed a set of mini hydro-turbines. Electricity is produced by means of a generator linked to the turbines converting potential energy of water stored in reservoirs (Contestabile et al., 2017a). Figure 1 reports the cross-section of OBREC highlighting geometrical parameters as showed in Contestabile et al. (2016).
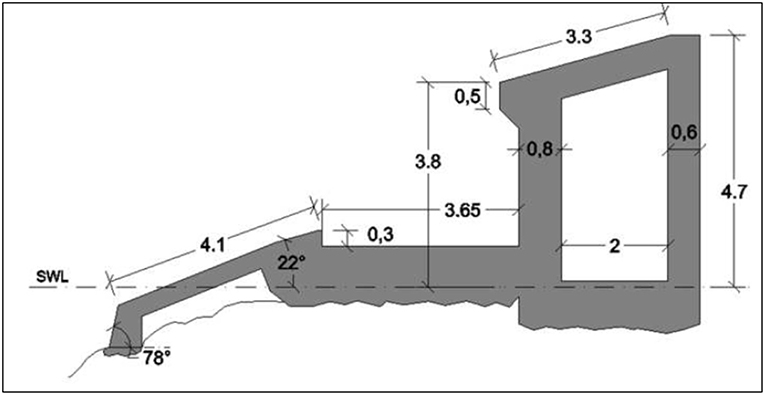
Figure 1. OBREC cross-section (from Contestabile et al., 2016).
The prototype implemented and tested in the harbor of Naples consists in a single module (5 m seafront length) that can be easily installed by assembling prefabricated elements, replicated and combined in rows along one side of a pier. The OBREC modules offer different solutions for the construction or refurbishment of breakwater systems and can potentially be used to partially or fully replace typical antifers, such as big stones or concrete tripods. The structure of one OBREC module, made of reinforced concrete (110 t concrete and 7 t iron), can likely replace 30–36 of 12-ton “antifers,” a kind of cyclopean grooved concrete cubes with hole (each side is 2 m long) or 13–16 42-ton “tetrapods,” (3.8 m high).
The module tested in the harbor of Naples embeds a set of pico hydro-turbine. The nominal power installed is about 3 kW. Based on ongoing monitoring campaign and numerical simulation by using a specifically-designed numerical model (OBRECsim, see Contestabile and Vicinanza, 2018), a 250 m pier in Naples is expected to generate more than 630 MWh/yr, corresponding to a wave-to-wire efficiency of 13.9%. The scenario simulated, take into account a new set of low head turbines, able to work with a wide spectrum of different incident wave conditions and water levels. Consistently, in this study, we assume an average electricity production of 12.6 MWh/yr for an OBREC single module 5 m long, in order to provide more reproducible results and considerations for other poor and mild wave climate.
Life Cycle Assessment (LCA) of the OBREC Module
The LCA has been applied in compliance with the International Standard Organization 14040 (2006a) and International Standard Organization 14044 (2006b). The life cycle of OBREC module has divided in three main phases of the production chain: (1) construction: production of structural elements and components; (2) building: assembly and on-site installation, including the transportation of the components to the building site; (3) maintenance: interventions for periodical check and maintenance (Figure 2). The end of life phase has not been included in this assessment, even if lifetime of structures and different components has been taken into account. Being OBREC able to replace, in mass terms, 3–4 rows of two layers of antifers from the breakwater and, considering that the main aim of this paper is the evaluation of the environmental investment required to implement OBREC, we assumed that decommission phase can be considered out of the system boundaries, as it would be equal both for OBREC and antifers.
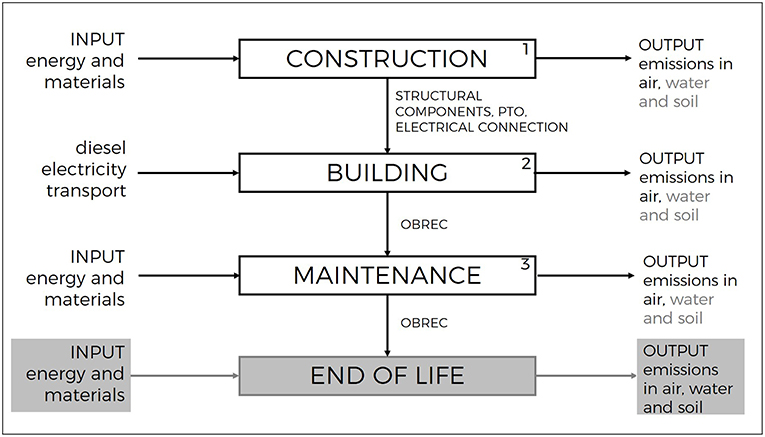
Figure 2. Flow chart with phases of the production chain. Phase 1: production of components; Phase 2: on site installation including transport; Phase 3: maintenance during operation. Gray boxes represent flows and phases outside the system boundaries.
The functional unit (FU) selected is represented by one single module (5 m seafront length) embedding the WEC (namely one module of OBREC). The system boundary includes the main lifecycle processes from cradle to gate, i.e., from cradle to a fully operating OBREC module.
Specific data regarding materials and energy needed to produce structural components (Phase 1) have been estimated based on metric computations (Contestabile et al., 2016) and considering main components: foundations, ramps and reservoirs made in reinforced concrete and pipes in PVC. The power take-off (PTO) system has been accounted as steel and PVC that are main materials of the pico hydro-turbine. Materials for electric connection (generator, stator, box and electrical cable) have been accounted as steel, PVC, copper, rubber, NdFeB (i.e., Neodimio-Ferro-Boro) alloy. A length of 1 km has been assumed for electric cable. Electricity losses have not been accounted.
The on-site installation (Phase 2) concerns energy use (electricity and diesel) for machineries (e.g., excavator) and materials (e.g., wood for the molds). A time span of 1 year (202 actual days considering work stoppage) for the whole building phase has been assumed. The average distance from the production site to the building site was assumed as 40 km for each component.
Maintenance (Phase 3) has been envisioned as 12 trips per year to the plant (average 30 km each) because there is no need of heavy interventions for ordinary maintenance. Nevertheless, the estimated time span for different components (e.g., 60 years for the concrete structure; 10 years for the turbine; 20 years for PVC pipes; 50 years for terrestrial cables and 25 years for marine ones), compared to the lifetime of the OBREC device (estimated 60 years), allowed to account for the replacement of components through maintenance.
The Life Cycle Inventory is modeled, and Life Cycle Impact Assessment performed by means of the LCA software tool SimaPro 8.4.0 (PRé Consultants, 2014). The Ecoinvent v3.1 (Wernet et al., 2016) database has been used for modeling the Life Cycle Inventory as source of secondary data. Table 1 reports input flows considered in the Life Cycle Inventory of the OBREC module, including both primary and secondary data sources.
The characterization method used in this study is the Global Warming Potential—GWP at the 100 year time horizon (Intergovernmental Panel on Climate Change, 2013), hereafter called also Carbon Footprint (CF).
In this analysis we focused the evaluation of the lifecycle processes and the production of electricity through OBREC limited to its contribution to climate change. A complete impact assessment, including other impact categories, will be the scope of further research.
Results and Discussions
Table 2 reports the energy and material flows per functional unit, i.e., one OBREC module, including the electricity production referred to a breakwater installed in poor and mild water climate in Italy.
The total CF of the OBREC module is 1.08 t CO2eq per FU is mainly due to construction elements (884.31 kg CO2eq) and minor contribution by building operations (85.28 kg CO2eq) and maintenance (113.57 kg CO2eq). Figure 3 shows the contribution of each single input to the total impacts, in terms of CO2eq, deriving from the life cycle of the OBREC module.
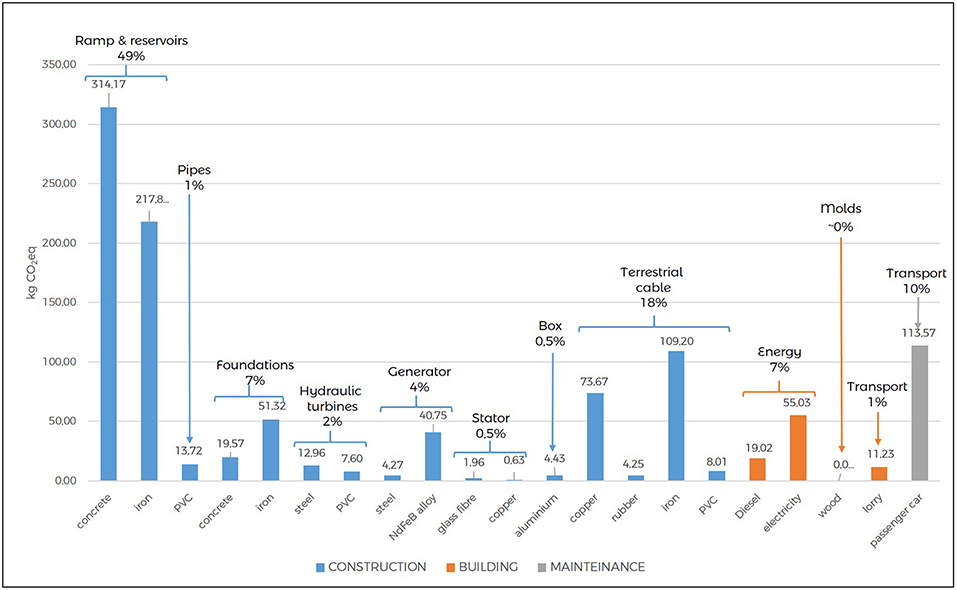
Figure 3. Breakdown of the CF of the OBREC module by single input flows (bottom). The percentage contribution of each construction element is reported in detail (up). Colors represent three Life Cycle Phases (Legend: blue=construction; orange=building; gray=maintenance).
Most of the impact throughout the production chain of OBREC is due to the use of materials for the construction of components (82%), including structural elements, i.e., ramp, reservoirs, foundations (56%), and the WEC system, especially electric cables for the connection to the grid (18%). Other impacts are due to operations for assembling and installing the OBREC system on site (8%) and its maintenance (10%). These results are in line with other LCA evaluations regarding ocean energy technologies (e.g., Dahlsten, 2009; Uihlein, 2016; Thomson et al., 2019) demonstrating that most of their impacts are related to materials even beyond the installation and maintenance of the devices. CF results are closely related to the mass flows (Table 2) in line with what outlined by Uihlein (2016) regarding the closed link between environmental impacts and material inputs. In particular the 56% of the total CF is due to concrete and iron needed for the foundations and construction of the ramp and reservoirs; these structural elements accounted for 95% of the total mass of the OBREC device. Input flows required during the building phase can be considered negligible (namely diesel and wood) except for the electricity that is responsible for the 5% of the total CF of the OBREC device. Finally, the use of a passenger car for the maintenance operations accounted for 11% of the total CF of the OBREC.
However, OBREC can be considered an upgrade of a traditional breakwater, thus we can consider the CF as a sort of “Environmental Investment” required for implementing a breakwater integrated with an OBREC module.
The Environmental Investment (EI) has been defined as the additional environmental impact produced to upgrade a system to a more integrated state, as stated by Patrizi et al. (2015) and Saladini et al. (2016). According to this definition, the environmental investment would specifically refer to the emissions provided to integrate the WEC system, made to produce renewable energy as additional function, in the breakwater, built to protect the port basin as primary function. Accordingly, processes included in the evaluation of the EI concerns the construction of WEC elements (generator, stator, box, and electric connection), their on-site assembling and maintenance, while we can assume that structural materials properly belong to the breakwater system (the OBREC module replaces 30–36 antifers likewise made of concrete with almost the same mass) and must not be taken into account.
Based on this observation the EI required for upgrading a breakwater with an OBREC module is represented by the portion of CF of the OBREC module assessed above (i.e., 1.08 t CO2eq) considering only the emission due to the implementation of the WEC system. In this way, the EI of OBREC is 0.48 t CO2eq, i.e., 44% of total CF (Table 3).
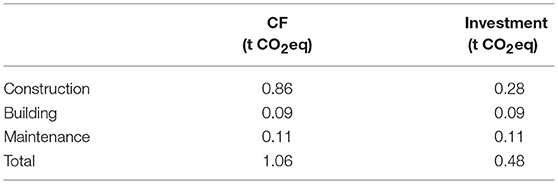
Table 3. Carbon Footprint compared to the “environmental investment” in terms of t CO2eq: “CF” refers to the comprehensive impact of the OBREC module; “investment” specifically concerns emissions for integrating the WEC system in the breakwater system instead of using traditional antifers.
The EI evaluation highlighted that the majority of CO2eq emissions are still due to construction elements being responsible for 59% of the total EI (i.e., 0.28 t CO2eq) of one module of OBREC. While the building and maintenance phases are responsible for the 18 and 23%, respectively of the total EI (i.e., 85.28 kg CO2eq and 113.57 kg CO2eq). In particular, 51% of the emissions are due to the electrical connection, more specifically to the terrestrial cable because of the copper and iron components. Therefore, a possible implementation to decrease emissions of OBREC can be represented by the use of electrical connection with higher environmental performances.
OBREC is expected to produce electricity with higher environmental performances then electricity produced from conventional resources. Results from the LCA allow for evaluating the Carbon Intensity of Electricity (CIE) of OBREC as the ratio between CO2eq emitted (CF) and the produced electricity (Moro and Lonza, 2018). This ratio can be included in the list of performance indicators: the lower its value, the better the performance (Ang and Su, 2016).
The annual productivity of electricity is highly dependent on the marine characteristic of the site in which OBREC is implemented. In this paper data on annual productivity has been taken from a study carried out for the extension of the Duca D'Aosta pier in Naples, considering an electricity production equal to 12.6 MWh/yr for one OBREC module (5 m length). Obviously, a detailed wave resource assessment would be necessary.
The CIE calculated as the amount of emissions divided by the electricity production of one OBREC module on a yearly basis reported a value of 0.086 t CO2eq/MWh when the total CF is considered. However, the estimation of the EI allowed for evaluating the CIE of OBREC in a more representative way. CIE calculated as the amount of invested emissions (EI) divided by the electricity production of one OBREC module on a yearly basis showed a value of 0.037 t CO2eq/MWh. Compared to CIE values of other renewable energies, we can see that the CIE of OBREC is quite similar to that of hydroelectric reservoir being CIE 0.01 t CO2eq/MWh (Sovacool, 2008). Both these values are much lower than the Italian electricity grid mix, i.e., 0.578 t CO2eq/MWh of electricity produced (Ecoinvent, 2014).
In order to assess environmental benefits, we considered avoided emissions due to the implementation of OBREC based on CIE, that corresponds to 0.49 t CO2eq/MWh (based on CF) and 0.54 t CO2eq/MWh (based on EI) per renewable electricity produced. The avoided emissions in producing 12.6 MWh per year therefore range from 6.20 to 6.81 t CO2eq/yr per OBREC module. Also, the carbon payback time for one module of OBREC (namely the time period of operation that is necessary to compensates emissions of total CF) was estimated to be 25 months. Focusing on the real EI the carbon payback time has been assessed to be 13 months.
This paper presents results of LCA of an innovative plant to produce renewable electricity by deploying wave energy obtained through an upgrading of a breakwater. Even if results of environmental benefits are very site specific (i.e., Naples, Italy), we can maintain that CF and more properly EI assessment are a prerequisite to foster the blue energy deployment. According to Pisacane et al. (2018) preliminary assessment on potential impacts are necessary to inform policy makers before any blue energy implementation (International Energy Agency, 2018b). Results of EI of OBREC, in fact, represented a first step for future research, these values will not be subject to variability on the contrary of wave potentials. While wave potential is linked to the localization, emissions necessary to upgrade a breakwater are not.
The crucial point in this evaluation is that a planned investment would allow for the conversion of energy embedded in waves into renewable electricity. We can interpret WEC systems integrated in harbors as a concretization of Herman Daly's quasi sustainability principle (Daly, 1990). According to Daly, quasi sustainability is a transition process during which the investment of non-renewable resources (such as structural components of the OBREC module) is a necessary condition to foster the production of a renewable resource, e.g., electricity (Bastianoni et al., 2009).
Finally, deploying untapped potential energy of waves can be viewed as a proper solution to prevent the so-called “tragedy of the commons” (Hardin, 1968). As affirmed by Lloyd (2007): “anthropogenic global warming and oil depletion can be seen as the traditional common grazing of the Hardin's paper” on the “tragedy of the commons.”
Conclusions
This paper presents an LCA of a Wave Energy Converter (WEC), namely OBREC (OBREC module installed and tested in the harbor of Naples) focusing on the Greenhouse Gases emission assessment, in order to evaluate environmental impacts and benefits of this blue energy technology. The implementation of a single module of OBREC provides 1.08 kg CO2eq/yr, considering the production and transport of constructive elements, their on-site assembling and maintenance. Most of the impact is due to structural parts, made of reinforced concrete; nevertheless, an OBREC module can replace several traditional antifers (i.e., artificial rocks for the breakwater armor layer).
This observation allowed for making assumptions for evaluating the Carbon Intensity of Electricity (CIE). The Environmental Investment can be defined as the emission provided to install a fully operating WEC system into the breakwater in place of traditional antifers and therefore add the function of producing renewable energy to that of protecting the port basin. Based on this assumption, the impact of the WEC is 0.48 t CO2eq/yr, i.e., 44% of the total CF, and the CIE is 0.037 t CO2eq/kWh. This value is much lower (i.e., 94%) than the CIE of current Italian electricity mix. The potential reduced CO2 emission due to the deployment of marine renewable energy for the electricity production have been considered as an “opportunity” for the blue energy technology development within a SWOT (Strengths, Weaknesses, Opportunities, and Threats) analysis regarding the deployment of Marine Energy in the Mediterranean Area carried out by Goffetti et al. (2018). Threats have been highlighted in economic and legal aspects that slow down the implementation of blue energy technologies coupled with lack of economic incentives (Goffetti et al., 2018).
This study demonstrates that breakwater integrated WECs, such as OBREC, are profitable solutions for exploiting renewable sources in the marine environment. Despite these are at the early stage, the environmental performance of existing devices and prototypes, based on a lifecycle approach, looks promising and supports the opportunity to further develop and test innovative blue energy technologies.
Author Contributions
NP, SB, and RMP conceived the paper. EN, NP, and VN elaborated and discussed data. DV, PC, and SB supervised the paper. All authors discussed the feedbak of the reviewers and contributed to the final manuscript.
Funding
This study has been developed in the framework of the Interreg Med MAESTRALE (2014–2020), project co-financed by the European Regional Development Fund. Website: https://maestrale.interreg-med.eu.
Conflict of Interest Statement
The authors declare that the research was conducted in the absence of any commercial or financial relationships that could be construed as a potential conflict of interest.
References
Alamian, R., Shafaghat, R., Shadloo, M. S., Bayani, R., and Amouei, A. H. (2017). An empirical evaluation of the sea depth effects for various wave characteristics on the performance of a point absorber wave energy converter. Ocean Eng. 137, 13–21. doi: 10.1016/j.oceaneng.2017.03.036
Amponsah, N. Y., Troldborg, M., Kington, B., Aalders, I., and Hough, R. L. (2014). Greenhouse gas emissions from renewable energy sources: a review of lifecycle considerations. Renew. Sustain. Energy Rev. 39, 461–475. doi: 10.1016/j.rser.2014.07.087
Ang, B. W., and Su, B. (2016). Carbon emission intensity in electricity production: a global analysis. Energy Policy 94, 56–63. doi: 10.1016/j.enpol.2016.03.038
Azzellino, A., Lanfredi, C., Contestabile, P., Ferrante, V., and Vicinanza, D. (2011). “Strategic environmental assessment to evaluate WEC projects in the perspective of the environmental cost-benefit analysis,” in The Twenty-first International Offshore and Polar Engineering Conference. Maui: International Society of Offshore and Polar Engineers.
Banerjee, S., Duckers, L., and Blanchard, R. E. (2013). An overview on green house gas emission characteristics and energy evaluation of ocean energy systems from life cycle assessment and energy accounting studies. J. Appl. Natur. Sci. 5, 535–540. doi: 10.31018/jans.v5i2.364
Bastianoni, S., Pulselli, R. M., and Pulselli, F. M. (2009). Models of withdrawing renewable and non-renewable resources based on Odum's energy systems theory and Daly's quasi-sustainability principle. Ecol. Model. 220, 1926–1930. doi: 10.1016/j.ecolmodel.2009.04.014
Benbouzid, M. E. H., Titah-Benbouzid, H., and Zhou, Z. (2017). “Ocean energy technologies”, in Encyclopedia of Sustainable Technologies, ed. M. Abraham (Oxford, Elsevier), 73–85.
Borthwick, A. G. L. (2016). Marine renewable energy seascape. Engineering 2, 69–78. doi: 10.1016/J.ENG.2016.01.011
BP energy Outlook (2018). BP energy Outlook 2018 edition. Available online at: https://www.bp.com/content/dam/bp/en/corporate/pdf/energy-economics/energy-outlook/bp-energy-outlook-2018.pdf (accessed July 19, 2018).
Contestabile, P., Di Lauro, E. D., Buccino, M., and Vicinanza, D. (2017a). Economic assessment of Overtopping BReakwater for Energy Conversion (OBREC): a case study in Western Australia. Sustainability 9:51. doi: 10.3390/su9010051
Contestabile, P., Di Lauro, E. D., Galli, P., Corselli, C., and Vicinanza, D. (2017b). Offshore wind and wave energy assessment around Malè and Magoodhoo Island (Maldives). Sustainability 9:613. doi: 10.3390/su9040613
Contestabile, P., Ferrante, V., Di Lauro, E., and Vicinanza, D. (2016). “Prototype overtopping breakwater for wave energy conversion at port of naples,” in Proceedings of the Twenty-sixth International Ocean and Polar Engineering Conference Rhodes, (Greece), 616–621.
Contestabile, P., and Vicinanza, D. (2018). Coastal defence integrating wave-energy-based desalination: a case study in Madagascar. J. Mar. Sci. Eng. 6:64. doi: 10.3390/jmse6020064
Copping, A., Smith, C., Hanna, L., Battey, H., Whiting, J., Reed, M., et al. (2013). Tethys: Developing a commons for understanding environmental effects of ocean renewable energy. Int. J. Marine Energy 3, 41–51. doi: 10.1016/j.ijome.2013.11.004
Dahlsten, H. (2009). Life Cycle Assessment of Electricity from Wave Power. [MSc Thesis]. Uppsala SLU: Swedish University of Agricultural Sciences.
Daly, H. E. (1990). Toward some operational principles of sustainable development. Ecol. Econ. 2, 1–6. doi: 10.1016/0921-8009(90)90010-R
de Andres, A., MacGillivray, A., Roberts, O., Guanche, R., and Jeffrey, H. (2017a). Beyond LCOE: a study of ocean energy technology development and deployment attractiveness. Sustain. Energy Technol. Assessments 19, 1–16. doi: 10.1016/j.seta.2016.11.001
de Andres, A., Medina-Lopez, E., Crooks, D., Roberts, O., and Jeffrey, H. (2017b). On the reversed LCOE calculation: design constraints for wave energy commercialization. Int. J. Mar. Energy 18, 88–108. doi: 10.1016/j.ijome.2017.03.008
Douziech, M., Hellweg, S., and Verones, F. (2016). Are wave and tidal energy plants new green technologies?. Env. Sci. Technol. 50, 7870–7878. doi: 10.1021/acs.est.6b00156
Ecoinvent (2014). Ecoinvent Database v3.3. Swiss Centre for Life-cycle Inventories. (Dübendorf). Available online at: http://www.ecoinvent.org/database/ (accessed July 19, 2018).
Elginoz, N., and Bas, B. (2017). Life Cycle Assessment of a multi-use offshore platform: combining wind and wave energy production. Ocean Eng. 145, 430–443. doi: 10.1016/j.oceaneng.2017.09.005
Ellabban, O., Abu-Rub, H., and Blaabjerg, F. (2014). Renewable energy resources: current status, future prospects and their enabling technology. Renew. Sust. Energ. Rev. 39, 748–764. doi: 10.1016/j.rser.2014.07.113
European Commission (2009). DIRECTIVE 2009/28/EC. Available online at: https://eur-lex.europa.eu/legal-content/EN/TXT/PDF/?uri=CELEX:32009L0028&from=EN (accessed July 19, 2018).
European Commission (2013). COM(2013) 169. GREEN PAPER - A 2030 Framework for Climate and Energy Policies. Available online at: https://eur-lex.europa.eu/legal-content/EN/TXT/PDF/?uri=CELEX:52013DC0169&from=EN (accessed July 19, 2018).
European Commission (2014). DIRECTIVE 2014/89/EU. Available online at: http://eur-lex.europa.eu/legal-content/EN/TXT/?uri=uriserv:OJ.L_.2014.257.01.0135.01.ENG%20 (accessed July 19, 2018).
Falcão, A. F. de O. (2010). Wave energy utilization: a review of the technologies. Renew. Sust. Energ. Rev. 14, 899–918. doi: 10.1016/j.rser.2009.11.003
Frid, C., Andonegi, E., Depestele, J., Judd, A., Rihan, D., Rogers, S. I., et al. (2012). The environmental interactions of tidal and wave energy generation devices. Environ. Impact. Assess. Rev. 32, 133–139. doi: 10.1016/j.eiar.2011.06.002
Goffetti, G., Montini, M., Volpe, F., Gigliotti, M., Pulselli, F. M., Sannino, G., et al. (2018). Disaggregating the SWOT analysis of marine renewable energies. Front. Energy Res. 6:138. doi: 10.3389/fenrg.2018.00138
Hardin, G. (1968). The tragedy of the commons. Science 162, 1243–1248. doi: 10.1126/science.162.3859.1243
Homma, T. (1985). S-1 prospects on ocean energy technology. Ocean Eng. 12:544. doi: 10.1016/0029-8018(85)90025-3
Hussain, A., Arif, S. M., and Aslam, M. (2017). Emerging renewable and sustainable energy technologies: state of the art. Renew. Sustain. Energy Rev. 71, 12–28. doi: 10.1016/j.rser.2016.12.033
Intergovernmental Panel on Climate Change (2011). Renewable Energy and Climate Change. Available online at: https://www.ipcc.ch/site/assets/uploads/2018/03/SRREN_FD_SPM_final-1.pdf (accessed July 19, 2018).
Intergovernmental Panel on Climate Change (2013). Fifth Assessment Report. The Physical Science Basis.
International Energy Agency (2018a). Global Energy & CO2 Status Report 2017. Available online at: https://www.iea.org/publications/freepublications/publication/GECO2017.pdf (accessed July 19, 2018).
International Energy Agency (2018b). The State of Knowledge for Environmental Effects. Driving Consenting/permitting for the Marine Renewable Energy Industry. Available online at: https://tethys.pnnl.gov/sites/default/files/publications/The%20State%20of%20Knowledge%20Driving%20Consenting%20Permiting%20for%20the%20MRE.pdf (accessed July 19, 2018).
International Renewable Energy Agency (2014). Ocean Energy- Technology Readiness, Patents, Deployment Status and Outlook. Available online at: http://www.irena.org/-/media/Files/IRENA/Agency/Publication/2014/IRENA_Ocean_Energy_report_2014.pdf (accessed July 19, 2018).
International Renewable Energy Agency (2018). Global Energy Transformation. A roadmap to 2050. Available online at: http://www.irena.org/publications/2018/Apr/Global-Energy-Transition-A-Roadmap-to-2050 (accessed July 19, 2018).
International Standard Organization 14040 (2006a). Environmental Management and Life Cycle Assessment e principles and Framework, Goal and Scope Definition and Life Cycle Inventory Analysis, Life Cycle Impact Assessment and Life Cycle Interpretation (Geneva).
International Standard Organization 14044 (2006b). Environmental Management and Life Cycle Assessment e requirements and Guidelines (Geneva).
Khan, N., Kalair, A., Abas, N., and Haider, A. (2017). Review of ocean tidal, wave and thermal energy technologies. Renew. Sustain. Energy Rev. 72, 590–604. doi: 10.1016/j.rser.2017.01.079
Kim, J., Koh, H. J., Cho, I. H., Kim, M. H., and Kweon, H. M. (2017). Experimental study of wave energy extraction by a dual-buoy heaving system. Int. J. Naval Architec. Ocean Eng. 9, 25–34. doi: 10.1016/j.ijnaoe.2016.07.002
Lewis, A., Estefen, S., Huckerby, J., Lee, K. S., Musial, W., Pontes, T., et al. (2012). “Ocean energy”, in Renewable Energy Sources and Climate Change Mitigation. Special Report of the Intergovernmental Panel on Climate Change, eds. O. Edenhofer, R. Pichs-Madruga, Y. Sokona, K. Seyboth, P. Matschoss, S. Kadner, et al. (Cambridge, NY: Cambridge University Press), 497–534.
Lloyd, B. (2007). The commons revisited: the tragedy continues. Energy Policy 35, 5806–5818. doi: 10.1016/j.enpol.2007.07.005
López-Ruiz, A., Bergillos, R. J., Raffo-Caballero, J. M., and Ortega-Sánchez, M. (2018). Towards an optimum design of wave energy converter arrays through an integrated approach of life cycle performance and operational capacity. Appl. Energy 209, 20–32. doi: 10.1016/j.apenergy.2017.10.062
Magagna, D., and Uihlein, A. (2015). Ocean energy development in Europe: current status and future perspectives. Int. J. Marine Energy 11, 84–104. doi: 10.1016/j.ijome.2015.05.001
Melikoglu, M. (2018). Current status and future of ocean energy sources: a global review. Ocean Eng. 148, 563–573. doi: 10.1016/j.oceaneng.2017.11.045
Moro, A., and Lonza, L. (2018). Electricity carbon intensity in European Member States: Impacts on GHG emissions of electric vehicles. Trans. Res. Part D Trans Environ. 64, 5–14. doi: 10.1016/j.trd.2017.07.012
Mustapa, M. A., Yaakob, O. B., Ahmed, Y. M., Rheem, C. K., Koh, K. K., and Adnan, F. A. (2017). Wave energy device and breakwater integration: a review. Renew. Sust. Energ. Rev. 77, 43–58. doi: 10.1016/j.rser.2017.03.110
Parker, R. P. M., Harrison, G. P., and Chick, J. P. (2007). Energy and carbon audit of an offshore wave energy converter. Proc. Inst. Mech. Eng. Part A J. Power. Energy 221, 1119–1130. doi: 10.1243/09576509JPE483
Patrizi, N., Pulselli, F. M., Morandi, F., and Bastianoni, S. (2015). Evaluation of the emergy investment needed for bioethanol production in a biorefinery using residual resources and energy. J. Cleaner Produc. 96, 549–556. doi: 10.1016/j.jclepro.2014.03.079
Pisacane, G., Sannino, G., Carillo, A., Struglia, M. V., and Bastianoni, S. (2018). Marine energy exploitation in the mediterranean region: steps forward and challenges. Front. Energy Res. 6:109. doi: 10.3389/fenrg.2018.00109
PRé Consultants (2014). SimaPro 8.4.0 Available online at: www.pre.nl/simapro/default (accessed July 19, 2018).
Ressurreiçao, A., Gibbons, J., Dentinho, T. P., Kaiser, M., Santos, R. S., and Edwards-Jones, G. (2011). Economic valuation of species loss in the open sea. Ecol. Econ. 70, 729–739. doi: 10.1016/j.ecolecon.2010.11.009
Rule, B. M., Worth, Z. J., and Boyle, C. A. (2009). Comparison of life cycle carbon dioxide emissions and embodied energy in four renewable electricity generation technologies in New Zealand. Env. Sci. Technol. 43, 6406–6413. doi: 10.1021/es900125e
Saladini, F., Patrizi, N., Pulselli, F. M., Marchettini, N., and Bastianoni, S. (2016). Guidelines for emergy evaluation of first, second and third generation biofuels. Renew. Sust. Energy Rev. 66, 221–227. doi: 10.1016/j.rser.2016.07.073
Sannino, G., and Cavicchioli, C. (2013). Overcoming Research Challenges for Ocean Renewable Energy. Available online at: https://setis.ec.europa.eu/energy-research/sites/default/files/library/ERKC_PB%20Ocean%20Renewable%20Energy.pdf (accessed July 19, 2018).
Şener, S. E. C., Sharp, J. L., and Anctil, A. (2018). Factors impacting diverging paths of renewable energy: a review. Renew. Sust. Energ. Rev. 81, 2335–2342. doi: 10.1016/j.rser.2017.06.042
Sørensen, H. C., Naef, S., Anderberg, S., and Hauschild, M. Z. (2006). “Life cycle assessment of the wave energy converter: Wave Dragon,” in International Conference Ocean Energy. From Innovation to Industry. Bremerhaven: OTTI.
Sovacool, B. K. (2008). Valuing the greenhouse gas emissions from nuclear power: a critical survey. Energy Policy 36, 2950–2963. doi: 10.1016/j.enpol.2008.04.017
Thomson, R. C., Chick, J. P., and Harrison, G. P. (2019). An LCA of the Pelamis wave energy converter. Int. J. Life Cycle Assess. 24, 51–63. doi: 10.1007/s11367-018-1504-2
Uihlein, A. (2016). Life cycle assessment of ocean energy technologies. Int. J. Life Cycle Assess. 21, 1425–1437. doi: 10.1007/s11367-016-1120-y
Uihlein, A., and Magagna, D. (2016). Wave and tidal current energy – A review of the current state of research beyond technology. Renew. Sust. Energ. Rev. 58, 1070–1081. doi: 10.1016/j.rser.2015.12.284
UNEP FAO, IMO, UNDP, IUCN, World Fish Center, GRIDArendal. (2012). Green Economy in a Blue World. Available online at: www.unep.org/greeneconomy and www.unep.org/regionalseas (accessed July 19, 2018).
Union for Mediterranean (2017). Blue Economy in the Mediterranean. Available online at: http://ufmsecretariat.org/wp-content/uploads/2017/12/UfMS_Blue-Economy_Report.pdf (accessed July 19, 2018).
United Nation Environmental Programme/Mediterranean Action plan (2016). Mediterranean Strategy for Sustainable Development 2016-2025. New York, NY; Valbonne: Plan Bleu, Regional Activity Centre. Available online at: http://planbleu.org/sites/default/files/publications/mssd_2016-2025_final.pdf (accessed July 19, 2018).
United Nations (2015). Transforming our world: the 2030 Agenda for Sustainable Development. General Assembly, Seventieth session, A/RES/70/1,2015. (New York).
United Nations Educational Scientific and Cultural Organization. (2018). The Science We Need for the Ocean We Want. Available online at: http://unesdoc.unesco.org/images/0026/002651/265198e.pdf (accessed July 19, 2018).
United Nations Framework Convention on Climate Change (2015). Paris Agreement. Available online at: https://unfccc.int/files/essential_background/convention/application/pdf/english_paris_agreement.pdf (accessed July 19, 2018).
Walker, S., and Howell, R. (2011). Life cycle comparison of a wave and tidal energy device. Proc. Inst. Mech. Eng. Part M J. Eng. Marit. Environ. 225, 325–337. doi: 10.1177/1475090211418892
Wernet, G., Bauer, C., Steubing, B., Reinhard, J., Moreno-Ruiz, E., and Weidema, B. (2016). The ecoinvent database version 3 (part I): overview and methodology. Int. J. Life Cycle Assess. 21, 1218–1230. doi: 10.1007/s11367-016-1087-8
Keywords: blue energy, Life Cycle Assessment, Carbon Footprint, Carbon Intensity of Electricity, environmental investment
Citation: Patrizi N, Pulselli RM, Neri E, Niccolucci V, Vicinanza D, Contestabile P and Bastianoni S (2019) Lifecycle Environmental Impact Assessment of an Overtopping Wave Energy Converter Embedded in Breakwater Systems. Front. Energy Res. 7:32. doi: 10.3389/fenrg.2019.00032
Received: 05 September 2018; Accepted: 08 March 2019;
Published: 12 April 2019.
Edited by:
Michel Feidt, UMR7563 Laboratoire d'énergétique et de Mécanique Théorique et Appliquée (LEMTA), FranceReviewed by:
Zhen Liu, Ocean University of China, ChinaJohn M. Polimeni, Albany College of Pharmacy and Health Sciences, United States
Copyright © 2019 Patrizi, Pulselli, Neri, Niccolucci, Vicinanza, Contestabile and Bastianoni. This is an open-access article distributed under the terms of the Creative Commons Attribution License (CC BY). The use, distribution or reproduction in other forums is permitted, provided the original author(s) and the copyright owner(s) are credited and that the original publication in this journal is cited, in accordance with accepted academic practice. No use, distribution or reproduction is permitted which does not comply with these terms.
*Correspondence: Riccardo M. Pulselli cHVsc2VsbGlAdW5pc2kuaXQ=