- 1Department of Environmental Sciences, COMSATS University Islamabad, Abbottabad Campus, Abbottabad, Pakistan
- 2Department of Chemical Engineering, COMSATS University Islamabad, Lahore Campus, Lahore, Pakistan
- 3Military College of Engineering (MCE), National University of Sciences and Technology (NUST), Risalpur Campus, Risalpur, Pakistan
The current study investigated the optimization of biogas generation during co-digestion of various plant biomasses with poultry waste at different ratios and pretreatment of plant biomass. The biochemical tests were executed at 35°C in a thermostat. Water hyacinth was found as the suitable substrate for mono and co-digestion due to high volatile solids (VS) and total soluble contents. However, poultry waste was appropriate only if it was co-digested with other biomasses. The experiments evaluating biogas generation at different ratios of plant biomasses and poultry waste demonstrated that water hyacinth and poultry (50:50) produced 262 mL g−1VS, giant reed and poultry (80:20) produced 235 mL g−1VSwhile maize and poultry (60:40) generated 193 mL g−1VS. However, the pretreating the plant biomass with either Fenton's or Fenton's plus ultrasonic had no effect on biogas generation. The volumes of biogas generated after various pretreatment were low as compared to condition without pretreatment. The codigestion can be classified as WH:P (50:50 > GR:P (80:20) > M:P (60:40). So, these ratios can be applied at decentralized scale for better waste management and biogas generation due to balanced C:N ratio of plant biomass and poultry manure. Co-digestion can also be applied at large scale with optimized ratio in Pakistan and other developing countries for biogas generation and waste management and reduce the methane emission through landfills.
Introduction
Currently, the world is facing problems like environmental contamination and shortage of energy. Exploring non-conventional and eco-friendly sources of energy is serious consideration of many developing nations (Owamah et al., 2014). In this scenario, the need and exploration of alternative biofuels is a need of the hour. Even exploitation of methanogenesis during anaerobic digestion (AD) is quite old (Triolo et al., 2011). The advantages of AD are multi-dimensional; it not only reduces wastes from the environment but also helps in nutrient turn over along generation of biomethane as an important energy source. The industrial viability of AD demands an appropriate low-cost amalgamation of physicochemical parameters for the optimum functioning of this process (Owamah et al., 2014). For a sustainable and productive AD process, appropriate amounts of various macro and micronutrients including N, P, S, Fe, Ni, Se, W, Co, Mo, among many others is quite desirable (Chen et al., 2008; Demirel and Scherer, 2008; Hinken et al., 2008). The role of co-digestion, pretreatment and digester design for the enhancement of biogas have been thoroughly reviewed by Shah et al. (2015).
Co-digestion involves the use of two different substrates in a mixture at different ratios. Previously, it was iterated that methanogenesis could significantly be enhanced and optimized if feedstocks were co-digested with animal wastes (Cavinato et al., 2010). In view of few drawbacks of feed stocks for mono-digestion (Hinken et al., 2008; Pobeheim et al., 2010), the codigestion of various substrates always resulted in enhanced biogas generation. Considering the nature, strength flow rate of the substrate used and the biogas generation could be enhanced in range of 25–400% (Callaghan et al., 2002; Alatrwaste-Mondragón et al., 2006).
Researchers reported different results for the screening of diverse biomasses for methanogenesis in mono-digestion and co-digestion. An increase of 400% was noted in methanogenesis when pig manure was co-digested with glycerol under mesophilic conditions (Astals et al., 2012). Co-digestion of algal biomass and fats, oils, and waste grease resulted in increased biogas production with gradual rise in organic loadings (Park et al., 2012). Stable biogas generation of 621 L/kgVS was observed at HRT of 42 days by digesting 50% slurry and whey mixture (Comino et al., 2012). The combination of waste paper, cow dung, and Eichhornia crassipes was evaluated for methanogenesis and the results were promising to reduce waste paper and enhancing biogas yield (Comino et al., 2012). In addition to increased methanogenesis, co-digestion of various substrates may present many advantages like high OLR, better biodegradability, balance of nutrients, suitable C:N ratio and lowering of toxicity of some substrates (Khalid et al., 2011; Wang et al., 2012, 2013). The preference of co-digestion was also advocated by (De Varies, 2012). AD of sewage sludge with poultry manure was also found beneficial (Bujoczek et al., 2000).
Biogas production was also found plentiful when substrates containing lignocelluloses was digested anaerobically (Zheng et al., 2014). In case of substrates containing high lignocellulose contents, pretreatment of biomasses with various techniques can be useful (Zheng et al., 2014) which reduce lignin, crystallinity but increase surface area. However, the richness of lignocellulose contents in the feedstock of AD may pose a barrier to microbial action on these substrates thus limiting the performance of AD (Fernández-Cegrí et al., 2012). In view of promising results obtained for co-digestion of different substrates, the importance of poultry waste in providing phosphorus for AD has never been realized to treat water hyacinth and other substrates. Water hyacinth is an abundant biomass that grows prolifically in all tropical and sub-tropical regions of the world. We anticipated that better biogas yield might be resulted by co-digestion of plant biomasses with poultry waste along with pretreatments. Pretreatment may be useful in view of fibrous nature of substrates which might have high cellulose and lingo-cellulosic materials. Based on above published reports, the aim of the current research was to evaluate the effects of pretreatment and co-digestion of various plant wastes with poultry litter for biogas generation.
Methodology
Sampling
Various substrates were collected for the experimentation from various non-contaminated places of Khyber Pakhtoonkhwa province of Pakistan. Prior to feeding into bioreactor, the plant biomasses and poultry wastes were air and oven dried at 35°C. The plant biomasses were chopped and ground for further experimentation.
Experimental Design
All the experiments were carried out in triplicates in a flask of volume of 500 ml for 30 days. The active volume was 100 ml with headspace of 400 ml for ratio experiment. The reactors were operated at temperature of 35°C as given in the Figure 1. Inoculum to substrate ratio (I/S) of 2:1 was administered to the bioreactor. The contents of the bioreactors were purged with nitrogen gas for 5 min to generate anaerobic conditions for microbes and subsequently sealed using butyl rubber stoppers. An outlet in the stopper was used for collecting biogas in inverted graduated cylinder and biogas was measured daily by water displacement as previously described by Shah et al. (2015). The pH of liquid in the inverted cylinder was kept acidic using 0.01 M HCl to avoid carbon dioxide absorption from biogas. A blank reactor was also run containing inoculum and distilled water, incubated at the same temperature to measure the background biogas produced from the inoculum and for pretreatment, which was subtracted from the total biogas production. All the digesters were monitored daily for biogas production. Experimental design for biomass ratio was given in the Table 1 and pretreatment design was shown in the Table 2.
Biogas Unit
A schematic diagram of experimental set up was adapted from Shah et al. (2015). It consisted of a temperature controlled water bath at 35°C containing digesters for the experiment. Each digester was connected to a graduated gas collector by means of a connecting tube. The experimental set up was shown in Figure 1. Biogas evolved was collected by downward water displacement as described above.
Seed Inoculum
Anaerobic sludge was used from the already running experiment. Therefore, it was assumed as microbiologically adequate to treat various substrates proposed for the BMP assays. Similarly, the amount of inoculum used in the test bottles was determined based on amount of organic substrate available for degradation, i.e., inoculum-to-substrate (I/S) ratio (on VS basis), which was equivalent to the inverse value of the food-to-microorganisms (F/I) ratio.
Statistical Analysis
All determinations were performed in triplicates and mean values were presented in the results. Statistical comparisons of the mean values were performed by two-way analysis of variance (ANOVA) using Sigma PlotTM v.12.
Results and Discussion
Effect of Different Biomass Ratios
A batch experiment was carried out to test co-digestion of different biomasses such as giant reed (GR), water hyacinth (WH), maize (M), and poultry (P) along with monodigestion. Poultry and plant biomass had no naturally occurring microbes and therefore, needed ample inoculation for startup of AD. The biomasses were co-digested at various ratios (50:50. 80:20, 60:40, 40:60, and 20:80) to test its potential for biogas generation. The biomasses were also characterized chemically to evaluate biogas generation through anaerobic mono and co-digestion. In case of mono-digestion, water hyacinth was the leading substrate among all the four selected substrates. The specific biogas production was the highest for WH (234 mL g−1VS) followed by GR (107 mL g−1VS), M (92 mL g−1VS), and P (49 mL g−1VS) for mono-digestion. The chemical composition distinctly showed that WH had high volatile solids and soluble contents make the basis for highest cumulative biogas yield and daily biogas production as shown in Figures 2, 3.
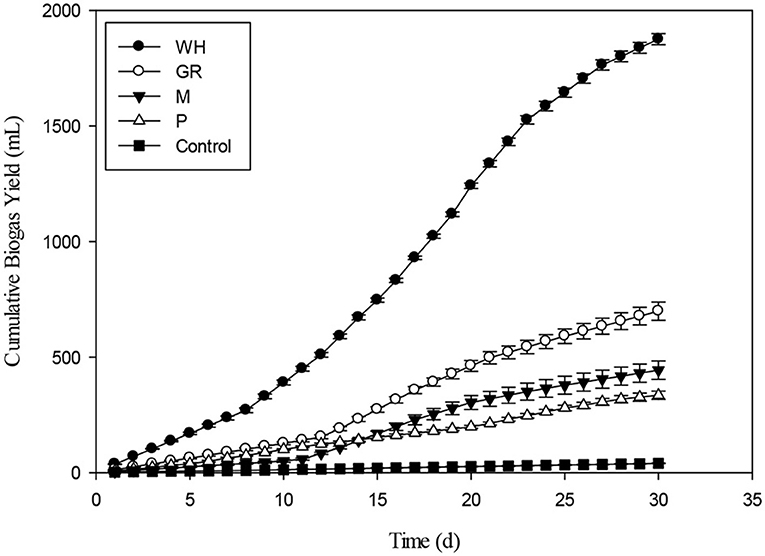
Figure 2. Single substrate anaerobic mono-digestion of Water Hyacinth, Giant Reed, Maize, and Poultry.
Various ratios of water hyacinth and poultry (WH: P) were presented in Figure 4. The 50:50 ratios of WH and P showed the highest biogas yield than rest of ratios of the same biomasses. The maximum biomethane yields from E. crassipes were 8.54 kJ g−1 dry biomass and 853.9 GJ ha−1y−1 which were 2.9 times higher than other crops (Yeong-Song et al., 2011). The total CO2 emission reduced the bioenergy production of E. crassipes and replacing coal, fossil oil and natural gas with E. crassipes would reduce the CO2 emission of 15.2–23.7 ton per year (Yeong-Song et al., 2011). Water hyacinth can be rich in nitrogen, up to 3.2% of DM and have a C:N ratio around 15. Water hyacinth was recommended as a substrate for compost or biogas production (Gunnarsson and Petersen, 2007).
On volatile solids (VS) basis, different ratios of water hyacinth and poultry 50:50, 80:20, 20:80, 60:40, and 40:60 produced specific biogas values of 262, 170, 164, 148, and 116 mL g−1 VS, respectively. The ratio of 50:50 produced the highest biogas production which might be due to the better C:N ratio ranging between 15 and 30 which seemed to be suitable range for microbial growth (Haug, 1993). Lower amounts of biogas produced at other ratios might be due to imbalanced C:N ratio. As it shown in the Table 1, water hyacinth contained 21.34 ppm nitrogen and poultry contained 16 ppm nitrogen. So, the excessive N in other different ratio affected the C:N ratio thereby affected the biogas production. At very high C:N ratio, accumulation of VFAs occurs which leads to inhibition of anaerobic digestion. For all tested ratios of water hyacinth and poultry substrate, the biogas generation at 50:50 ratio was significantly different from other ratios during 30 days of experiment.
The daily biogas production of water hyacinth and poultry at different ratios were shown in the Figure 5. A ratio of 50:50 had the most stable biogas production and was significantly different from rest of tested ratios until day 15. The 20:80 ratio of WH: P had less biogas production up to 10th day in comparison to other ratios. On VS basis, 40% of water hyacinth with poultry waste had better contribution for biogas generation. Almost similar contribution on VS basis (35%) of was shown by raw sludge and food waste which enhanced the anaerobic digestion process as compared to mono-digestion (Koch et al., 2015). The co-digestion of APW with DM increased the biogas and methane yield by 11.7–28.6 and 18.9–43.7%, respectively, compared with the mono-digestion of APW. However, the ratio of 3:1 for Aloe peel waste and dairy manure (APW/DM) depicted the optimal performance leading to the highest cumulative methane yield (195.1 mL g−1 VS) (Huang et al., 2016). Figure 6 showed the results of cumulative biogas production for different ratios for giant reed and poultry. For all the 80:20 and 20:80 mixing ratios of Giant reed (GR), and Poultry (P), better results were recorded as compared with all other ratios. The biogas production for 80:20 and 20:80 were 235 and 229 mL g−1VS compared to monodigestion of GR 107 mL g−1 VS. The 50:50 ratios of GR and P had least biogas production as compared to all other ratios. The pH of the 50:50 was 5.5. Lower pH might be due to the aggregation of VFAs. The C/N ratio may be a major factor behind such results; the impact of the C/N ratio on AD has been investigated at the optimum C/N ratio of 20–25 (Mshandete et al., 2004; Yen and Brune, 2007). With the higher C:N ratio, VFAs may accumulate. If C:N was lower than the appropriate range, methanogens may be inhibited by the high ammonia concentration (Gong et al., 2011; Banks et al., 2012).
The daily rate of biogas production for various ratios of giant reed and poultry substrates were presented in Figure 7. In the beginning of the experiment 60:40 ratio of the GR and P had the highest biogas production peak. The most stable biogas production rates were given by 60:40 from day 13 to day 23. By comparing the monodigestion of GR the overall daily biogas production was <40 mL d−1. The co-digestion of giant reed and poultry resulted in improved daily biogas production.
The biogas production at various ratios of maize and poultry substrate was presented in the Figure 8. For all the ratios of M and P, the 40:60 has the highest cumulative biogas production. However, 80:20 had the lowest biogas production by comparing with all other ratio. On basis of VS, 40:60 produced 193 mL g−1 VS, 20:80 gave 172 mL g−1 VS, 60:40 gives 153 mL g−1 VS, 50:50 generated 136 mL g−1VS, and 80:20 produced 121 mL g−1 VS. The order of biogas production on VS basis was 40:60> 20:80> 60:40>50:50 and 80:20. The primary reason may be for production of less biogas production from mixing ratio of Maize and Poultry of 50:50 and 80:20 seemed high VFAs volatile fatty acids and reduced pH, which might have caused process instability.
The stability of anaerobic digestion was often expressed as the ratio of VFA to alkalinity. A ratio of <0.4 was generally regarded as optimal for anaerobic digestion, whereas a ratio exceeding 0.6 was regarded as indicative of overfeeding (Brown and Li, 2013). The results showed that acidogenesis was dominant over acetogenesis.
The production of long chain fatty acids causes slight inhibition of biogas production. However, a study evaluated the dairy manure and switch grass which presented best results at 50:50 ratio (Zheng et al., 2015). The positive effects of co-digestion can be accredited to manifold factors, including proportional nutrient composition, accelerated synergistic effects of microbial composition, an associated increase in buffering capacity and a decreased effect of toxic compounds on the digestion process (Wang et al., 2012). Figure 9 presents the daily biogas production rate for different ratios of Maize and poultry waste (M:P). Initially, the 80:20 ratio had the highest biogas production. However, during the latter stages of the experiment 40:60 and 20:80 had the highest and stable biogas production than 80:20 and 60:40 ratios. The biogas production was decreasing with the passage of due to substrate utilization during the 30 day, experiment. During last 10 days, biogas production was minimal as compared to early days of the experiment. The comparison of present study with the previous ones was presented in Table 3.
Effect of Various Pretreatments
The effect of Fenton and Fenton plus ultrasonication pretreatment on biogas generation of WH was shown in the Figure 10. The biogas produced by WH biomass treated with Fenton's and Fenton's plus ultrasonic was lesser than control. The reason for less biogas production in the present study from the chemical pretreatment might be due to the less target of ligno-cellulosic part of the WH. However, in literature the thermal pretreatment of the water hyacinth was quite effective for biogas optimization (Barua and Kalamdhad, 2017). The biochemical study unveiled that the cumulative methane production of hot air oven pretreated WH (3039 ± 32 mL CH4 g−1 VS) at 90°C for 1 h was way higher than the cumulative methane production of untreated substrate 2396 ± 19 mL CH4 g−1 VS on the 35th day (Barua and Kalamdhad, 2017). The cumulative biogas production from day second to day 8th the WH pretreated with Fenton's and Fenton's plus ultrasonic were not significantly different (p > 0.05). The Figure 11 presents the pretreatment effect of Fenton's and Fenton's plus ultrasonic on daily biogas production. From the beginning, there was no significant effect of both pretreatment on biogas production with the control. However, Fenton pretreatment was comparatively better than Fenton's and Fenton's plus ultrasonic pretreatment. The less biogas production may due to the low energy application of ultrasound where no cell lysis occurred and COD solubilisation was due to exo-polymer dissolution, resulting in low methane production. However, at higher energies the disruption of the cell contributed to enhanced methane production (González-Fernández et al., 2012). The ultrasound pretreatment was recommended and effective when the lignocellulosic material to mix with water (Rodriguez et al., 2017). For daily biogas production, the control and both pretreatments were significantly different (p < 0.05) on day first; however, control and both pretreatments were significantly not different (p > 0.05) on day sixth. From day seventh to day 27th control and both pretreatments were significantly different (p < 0.05).
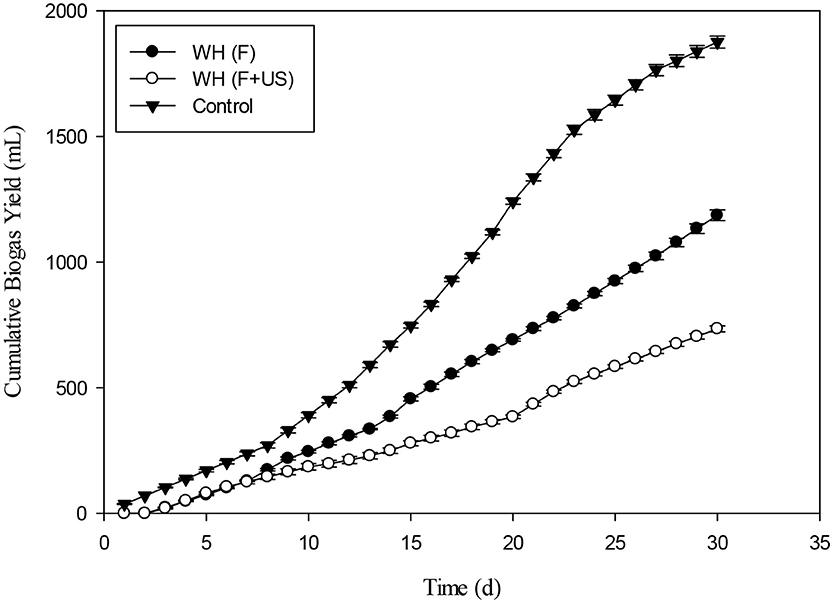
Figure 10. Effect of Fenton's and ultrasound (Fenton's + Ultrasonication) on biogas generation of water hyacinth.
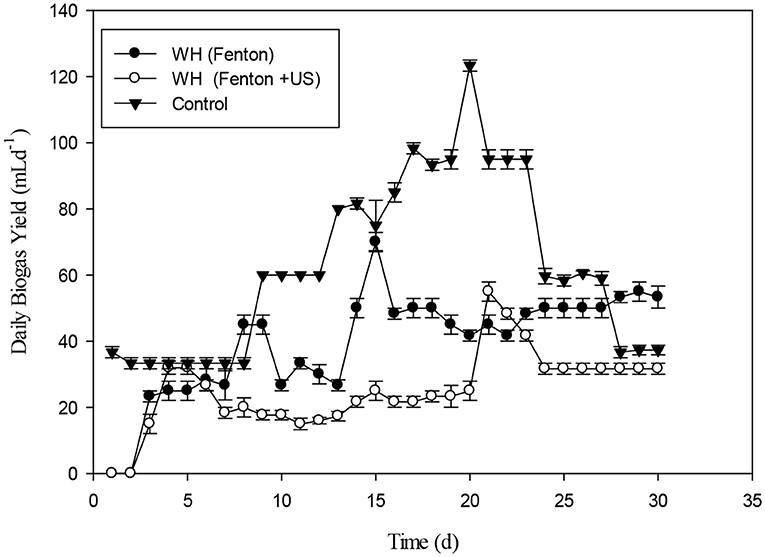
Figure 11. Daily biogas rate of water hyacinth pretreatment with Fenton's and Fenton's plus ultra-sonication.
The cumulative biogas production from Maize with pretreatments such as Fenton's and Fenton's plus ultrasonic was shown in the Figure 12. On VS basis, the cumulative biogas production from maize under the mentioned pretreatments was 61 mL g−1VS and 30 mL g−1VS. Both the pretreatments were effective for biogas production up to 15 days. By comparing with control the Fenton pretreatment was more efficient than Fenton's plus ultrasonic. The cumulative biogas production for maize pretreatment with Fenton's and Fenton's plus ultrasonic with control were not significantly different (p > 0.05). However, from day 20th to day 30th control vs. pretreatments were significantly different (p < 0.05). The current ultrasonic pretreatment was carried out at 30°C. Temperature has crucial influence in the samples used for sonication because other studies showed that it has a great influence on the changing of different properties of the liquid medium, such as viscosity, surface tension, and mainly vapor pressure, which influence cavitation (Karray et al., 2015). As the temperature of the liquid increases, its vapor pressure, and consequently the vapor pressure inside the bubble, also increases when implosion occurs. It can be seen that the ultrasonication pre-treatment results in the solubilization of carbohydrates. However, the current results for biogas production at 30°C were not fruitful as compared to the other results conducted experiments with macro algal biomass (Karray et al., 2015). In the most recent article published by Rodriguez et al. (2017) it was recommended that plant biomass must be mixed with water for optimum results.
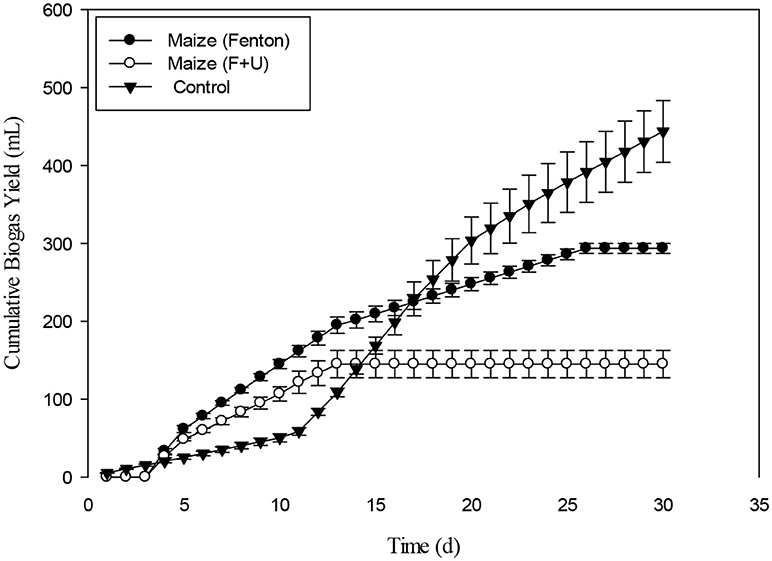
Figure 12. Cumulative biogas production from Maize with Fenton's and Fenton's plus ultrasound pretreatment.
The daily biogas production of Maize after pretreatment with Fenton's and Fenton's plus ultrasonic was represented in the Figure 13. In the beginning, biogas production from the pretreated biomass was lesser. However, on the 5th day onwards, biogas production from pretreated biomass was higher than biomass pretreated with Fenton's and from control. The daily biogas production was stable on 15th day onwards until 30th day. Figure 14 depicts the cumulative biogas production of giant reed with Fenton's and Fenton's plus ultrasonics pretreatment. The giant reed pretreated with Fenton's was comparatively better for biogas production than the giant reed pretreated with Fenton's plus ultrasonic. However, both pretreatments giant reed produced lower biogas than the control. Lower biogas production might be due to chemical effect of pretreatment on the microbial guilds of the batch system. The cumulative biogas production from day 1st to day 12th both pretreatment and control were not significantly different (p > 0.05).
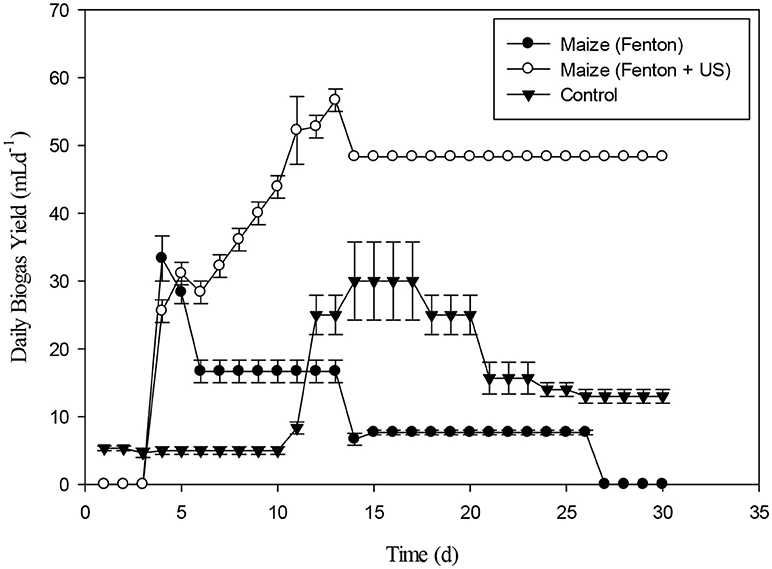
Figure 13. Daily Biogas production of Maize with pretreatment of Fenton's and Fenton's plus Ultrasonic.
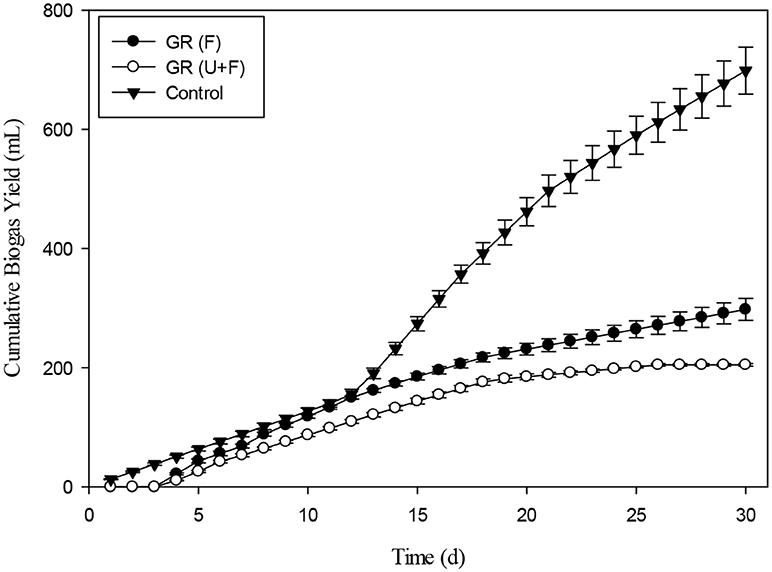
Figure 14. Cumulative biogas production from Giant reed (GR) with Fenton's (F) and Fenton's plus ultrasound (F + US) pretreatment.
The daily biogas production for the pretreated giant reed with Fenton's and Fenton's plus ultrasonic were shown in the Figure 15. In the early stages, daily biogas production for Fenton plus ultrasonic was lesser than control and giant reed biomass pretreated with Fenton. After day 3, biogas production from both pretreatment was almost at same level until day 19th. After 20th day the daily biogas production for both pretreatment were different. The daily biogas production from day 1st, day 3rd, were significantly different (p < 0.05).
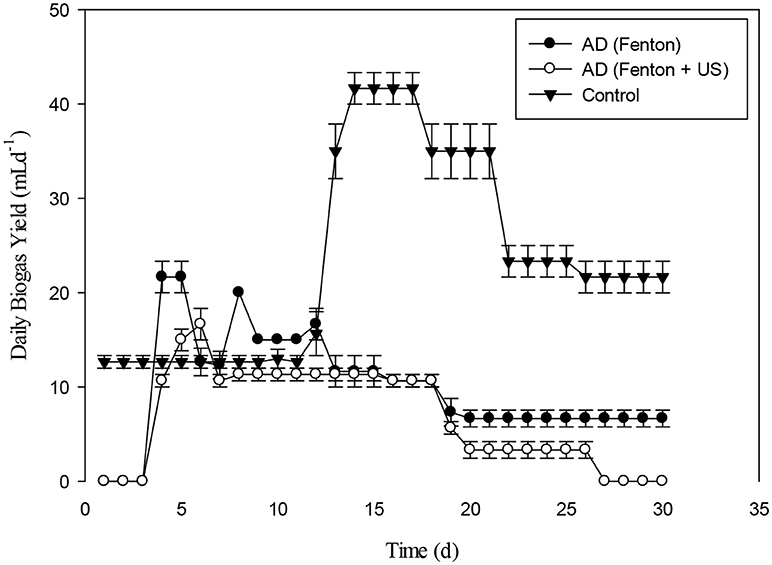
Figure 15. Daily Biogas production of giant reed (G:R) with pretreatment of Fenton's (F) and Fenton's plus (F+US) Ultrasonic.
The above-mentioned findings are quite practical in enhancing biogas production from various biomasses rich in cellulosic or lignocellulosic contents. There were two objectives of this investigation viz. the balancing of nutrients by the codigestion process and secondly the overcoming of toughness of lingo-cellulosic materials through physic-chemical pretreatment. The results produced are very promising and quite practical in the sense that we recommend co-digestion of WH, giant reed, and poultry waste in order to enhance biogas yield per gram VS basis. Water hyacinth and poultry codigestion was the most promising in enhancing biogas production. Poultry waste could be a rich source of phosphorus which could satisfy the nutrient deficiency and to optimize C:N:P for an optimum methanogenesis. The codigestion can be classified as WH:P (50:50) > GR:P (80:20) > M:P (60:40). So, these ratios can be applied at decentralized scale for better waste management and biogas generation due to balanced C:N ratio of plant biomass and poultry manure. Co-digestion can also be applied at large scale with optimized ratio in Pakistan and other developing countries for biogas generation and waste management and reduce the methane emission through landfills. Co-digestion can be applied at home scale by operating through pickle barrel digester to play a key role against the global warming to avoid the open dumping of waste. The present study does not recommend the use of pretreatment as discussed above. The use of various chemicals especially Fenton reagent may disturb pH and chemical composition of feedstock and thus disturb optimum conditions for AD. The chemical structure of the feedstock may also be affected which resulted in lower biogas yields.
Conclusion
The batch experiments were conducted with various ratios and pretreatment of water hyacinth, giant reed, maize, and poultry substrates. The incubated biomasses were optimized at different ratios. The ratios experiments involving various selected plant biomasses and poultry wastes concluded that water hyacinth and poultry (50:50) produced 262 mL g−1VS, Giant reed and Poultry produced (80:20) produced 235 mL g−1VS, while Maize and Poultry (60:40) produced 193 mL g−1VS. So, these ratios can be applied at decentralized scale for better waste management and biogas generation due to balanced C:N ratio of plant biomass and poultry manure. However, the Fenton and Fenton's plus ultrasonic pretreatments of plant biomasses were not suitable for biogas optimization as compared to co-digestion.
Author Contributions
All authors listed have made a substantial, direct and intellectual contribution to the work, and approved it for publication.
Conflict of Interest Statement
The authors declare that the research was conducted in the absence of any commercial or financial relationships that could be construed as a potential conflict of interest.
References
Ahn, H. K., Smith, M., Kondrad, S., and White, J. (2010). Evaluation of biogas production potential by dry anaerobic digestion of switchgrass–animal manure mixtures. Appl. Biochem. Biotechnol. 160, 965–975. doi: 10.1007/s12010-009-8624-x
Alatrwaste-Mondragón, F., Samar, P., Cox, H. H., Ahring, B. K., and Iranpour, R. (2006). Anaerobic codigestion of municipal, farm, and industrial organic wastes: a survey of recent literature. Water Environ. Res. 78, 607–636. doi: 10.2175/106143006X111673
Astals, S., Nolla-Ardèvol, V., and Mata-Alvwerez, J. (2012). Anaerobic co-digestion of pig manure and crude glycerol at mesophilic conditions: biogas and digestate. Bioresour. Technol. 110, 63–70. doi: 10.1016/j.biortech.2012.01.080
Banks, C. J., Zhang, Y., Jiang, Y., and Heaven, S. (2012). Trace element requirements for stable food waste digestion at elevated ammonia concentrations. Bioresour. Technol. 104, 127–135. doi: 10.1016/j.biortech.2011.10.068
Barua, V. B., and Kalamdhad, A. S. (2017). Effect of various types of thermal pretreatment techniques on the hydrolyswas, compositional analyswas and characterization of water hyacinth. Bioresour. Technol. 227, 147–154. doi: 10.1016/j.biortech.2016.12.036
Brown, D., and Li, Y. (2013). Solid state anaerobic co-digestion of yard waste and food waste for biogas production. Bioresour. Technol. 127, 275–280. doi: 10.1016/j.biortech.2012.09.081
Bujoczek, G., Oleszkiewicz, J., Sparling, R., and Cenkowski, S. (2000). High solid anaerobic digestion of chicken manure. J. Agricul. Eng. Res. 76, 51–60 doi: 10.1006/jaer.2000.0529
Callaghan, F. J., Wase, D. A. J., Thayanithy, K., and Forster, C. F. (2002). Continuous codigestion of cattle slurry with fruit and vegetable wastes and chicken manure. Biomass Bioenergy 27, 71–77 doi: 10.1016/S0961-9534(01)00057-5
Cavinato, C., Fatone, F., Bolzonella, D., and Pavan, P. (2010). Thermophilic anaerobic co-digestion of cattle manure with agro-wastes and energy crops: comparwason of pilot and full scale experiences. Bioresour. Technol. 101, 545–550. doi: 10.1016/j.biortech.2009.08.043
Chen, G., Chang, Z., Ye, X., Du, J., Xu, Y., and Zhang, J. (2012). Methane production by anaerobic co-digestion of chicken manure and Spartina alterniflora residue after producing methane. Huan jing ke xue 33, 203–207. doi: 10.1016/S0960-8524(01)00133-X
Chen, Y., Cheng, J. J., and Creamer, K. S. (2008). Inhibition of anaerobic digestion process: review. Bioresour. Technol. 90, 4044–4064. doi: 10.1016/j.biortech.2007.01.057
Comino, E., Riggio, V. A., and Rosso, M. (2012). Biogas production by anaerobic co-digestion of cattle slurry and cheese whey. Bioresour. Technol. 114, 46–53. doi: 10.1016/j.biortech.2012.02.090
De Varies, J. M. (2012). Comparing environmental consequences of anaerobic mono and codigestion of pig maure to produce bioenergy. A life cycle perspective. Bioresour. Technol. 125, 239–248. doi: 10.1016/j.biortech.2012.08.124
Demirel, B., and Scherer, P. (2008). Production of methane from Sugar Beet Silage without Manure addition by a single-stage anaerobic digestion process. Biomass Bioener. 32, 203–209. doi: 10.1016/j.biombioe.2007.09.011
Fernández-Cegrí, V., De la Rubia, M. Á., Raposo, F., and Borja, R. (2012). Effect of hydrothermal pretreatment of sunflower oil cake on biomethane potential focusing on fibre composition. Bioresour. Technol. 123, 424–429. doi: 10.1016/j.biortech.2012.07.111
Gelegenwas, J., Georgakakwas, D., Angelidaki, I., and Mavrwas, V. (2007). Optimization of biogas production by co-digesting whey with diluted poultry manure. Renew. Energy 32, 2147–2160 doi: 10.1016/j.renene.2006.11.015
Gong, L., Lewicki, R., Griffin, R., Flynn, J., and Lefer, B. (2011). Atmospheric ammonia measurements in Houston, TX using an external-cavity quantum cascade laser-based sensor. Atmos. Chem. Phys. 11, 9721–9733. doi: 10.5194/acp-11-9721-2011
González-Fernández, C., Sialve, B., Bernet, N., and Steyer, J. (2012). Comparwason of ultrasound and thermal pretreatment of Scenedesmus biomass on methane production. Bioresour. Technol. 110, 610–616. doi: 10.1016/j.biortech.2012.01.043
Gunnarsson, C. C., and Petersen, C. M. (2007). Water hyacinths as a resource in agriculture and energy production: a literature review. Waste Manag. 27, 117–129. doi: 10.1016/j.wasman.2005.12.011
Hinken, L., Urban, I., Haun, E., Urban, I., Weichgrebe, D., and Rosenwinkel, K. H. (2008). The valuation of malnutrition in the mono-digestion of maize silage by anaerobic batch tests. Wat. Sci. Technol. 58, 1458–1459. doi: 10.2166/wst.2008.491
Huang, X., Yun, S., Zhu, J., Du, T., Zhang, C., and Li, X. (2016). Mesophilic anaerobic co-digestion of aloe peel waste with dairy manure in the batch digester: focusing on mixing ratios and digestate stability. Bioresour. Technol. 218, 62–68. doi: 10.1016/j.biortech.2016.06.070
Karray, R., Hamza, M., and Sayadi, S. (2015). Evaluation of ultrasonic, acid, thermo-alkaline and enzymatic pre-treatments on anaerobic digestion of Ulva rigida for biogas production. Bioresour. Technol. 187, 205–213. doi: 10.1016/j.biortech.2015.03.108
Khalid, A., Arshad, M., Anjum, M., Mahmood, T., and Dawson, L. (2011). The anaerobic digestion of solid organic waste. Waste Manag. 31, 1737–1744 doi: 10.1016/j.wasman.2011.03.021
Koch, K., Helmreich, B., and Drewes, J. E. (2015). Co-digestion of food waste in municipal wastewater treatment plants: effect of different mixtures on methane yield and hydrolyswas rate constant. Appl. Energy 137, 250–255. doi: 10.1016/j.apenergy.2014.10.025
Li, Y., Zhang, R., Chen, C., Liu, G., He, Y., and Liu, X. (2013). Biogas production from co-digestion of corn stover and chicken manure under anaerobic wet, hemi-solid, and solid state conditions. Bioresour. Technol. 149, 406–412. doi: 10.1016/j.biortech.2013.09.091
Magbanua, B. S. Jr., Adams, T. T., and Johnston, P. (2001). Anaerobic codigestion of hog and poultry waste. Bioresour. Technol. 76, 165–168 doi: 10.1016/S0960-8524(00)00087-0
Mshandete, A., Kivawasi, A., Rubindamayugi, M., and Mattiasson, B. (2004). Anaerobic batch co-digestion of swasal pulp and fwash wastes. Bioresour. Technol. 95, 19–24. doi: 10.1016/j.biortech.2004.01.011
Owamah, H. I., Alfa, M. I., and Dahunsi, S. O (2014). Optimization of biogas from chicken droppings with Cymbopogon citrates. Renew. Energy 68, 366–371. doi: 10.1016/j.renene.2014.02.006
Park, C., Park, S., Kim, C., and Lee, S. (2012). Effects of EGR on performance of engines with spark gap projection and fueled by biogas–hydrogen blends. Int. J. Hydr. Energy 37, 14640–14648. doi: 10.1016/j.ijhydene.2012.07.080
Pobeheim, H., Munk, B., Johansson, J., and Guebitz, G. M. (2010). Influence of trace elements on methane formation from a synthetic model substrate for maize silage. Bioresour. Technol. 101, 836–839. doi: 10.1016/j.biortech.2009.08.076
Rodriguez, C., Alaswad, A., Benyounwas, K., and Olabi, A. (2017). Pretreatment techniques used in biogas production from grass. Renew. Sustain. Energy Rev. 68, 1193–1204. doi: 10.1016/j.rser.2016.02.022
Shah, F. A., Mahmood, Q., Rashid, N., Pervez, A., Raja, I. A., and Shah, M. M. (2015). Co-digestion, pretreatment and digester design for enhanced methanogeneswas. Renew. Sustain. Energy Rev. 42, 627–642. doi: 10.1016/j.rser.2014.10.053
Triolo, J. M., Sommer, S. G., Møller, H. B., Wewasbjerg, M. R., and Jiang, X. (2011). A new algorithm to characterize biodegradability of biomass during anaerobic digestion: Influence of lignin concentration on methane production potential. Bioresour. Technol. 102, 9395–9402 doi: 10.1016/j.biortech.2011.07.026
Wang, X., Yang, G., Li, F., Feng, Y., Ren, G., and Han, X. (2013). Evaluation of two statistical methods for optimizing the feeding composition in anaerobic co-digestion mixture design and central composite design. Bioresour. Technol. 131, 172–178 doi: 10.1016/j.biortech.2012.12.174
Wang, X., Yang, Y., Feng, Y., Ren, G., and Han, X. (2012). Optimizing feeding composition and carbon-nitrogen ratios for improved methane yield during anaerobic codigestion of dairy, chicken manure and wheat straw. Bioresour. Technol. 120, 78–83 doi: 10.1016/j.biortech.2012.06.058
Yangin-Gomec, C., and Ozturk, I. (2013). Effect of maize silage addition on biomethane recovery from mesophilic co-digestion of chicken and cattle manure to suppress ammonia inhibition. Energy Conver. Manag. 71, 92–100. doi: 10.1016/j.enconman.2013.03.020
Yen, H.-W., and Brune, D. E. (2007). Anaerobic co-digestion of algal sludge and waste paper to produce methane. Bioresour. Technol. 98, 130–134. doi: 10.1016/j.biortech.2005.11.010
Yeong-Song, C., Chyi-How, L., Biswarup, S., Chin-Chao, C., Gopalakrishnan, K., Jou-Hsien, W., et al. (2011). Biohydrogen and biomethane from water hyacinth (Eichhornia crassipes) fermentation: effects of substrateconcentration and incubation temperature. Int. J. Hydrogen Energy 36, 14191–14203. doi: 10.1016/j.ijhydene.2011.04.188
Zheng, Y., Zhao, J., Xu, F., and Li, Y. (2014). Pretreatment of lignocellulosic biomass for enhanced biogas production. Prog. Energy Combust. Sci. 42, 35–53. doi: 10.1016/j.pecs.2014.01.001
Keywords: anaerobic digestion, co-digestion, giant reed, maize, pretreatment, water hyacinth
Citation: Shah FA, Rashid N, Mahmood Q and Ali A (2019) Effect of Pretreatment and Substrate Ratios in Biorefinery Employing Co-digestion of Plant Biomass and Poultry Waste. Front. Energy Res. 6:143. doi: 10.3389/fenrg.2018.00143
Received: 06 August 2018; Accepted: 10 December 2018;
Published: 10 January 2019.
Edited by:
Abdul-Sattar Nizami, King Abdulaziz University, Saudi ArabiaReviewed by:
Md Mofijur Rahman, Central Queensland University, AustraliaMohammad Rehan, King Abdulaziz University, Saudi Arabia
Copyright © 2019 Shah, Rashid, Mahmood and Ali. This is an open-access article distributed under the terms of the Creative Commons Attribution License (CC BY). The use, distribution or reproduction in other forums is permitted, provided the original author(s) and the copyright owner(s) are credited and that the original publication in this journal is cited, in accordance with accepted academic practice. No use, distribution or reproduction is permitted which does not comply with these terms.
*Correspondence: Qaisar Mahmood, bWFobW9vZHpqdUBnbWFpbC5jb20=