- 1Department of Chemical Engineering and Materials Science, Michigan State University, East Lansing, MI, United States
- 2DOE Great Lakes Bioenergy Research Center, Michigan State University, Madison, MI, United States
- 3Department of Chemical Engineering, Michigan Technological University, Houghton, MI, United States
- 4Department of Biochemistry & Biophysics, Texas A&M University, College Station, TX, United States
- 5Department of Chemical & Biological Engineering, Montana State University, Bozeman, MT, United States
- 6Division of Chemical Engineering, Luleå University of Technology, Luleå, Sweden
Sorghum (Sorghum bicolor L. Moench) offers substantial potential as a feedstock for the production of sugar-derived biofuels and biochemical products from cell wall polysaccharides (i. e., cellulose and hemicelluloses) and water-extractable sugars (i.e., glucose, fructose, sucrose, and starch). A number of preprocessing schemes can be envisioned that involve processes such as sugar extraction, pretreatment, and densification that could be employed in decentralized, regional-scale biomass processing depots. In this work, an energy sorghum exhibiting a combination of high biomass productivity and high sugar accumulation was evaluated for its potential for integration into several potential biomass preprocessing schemes. This included counter-current extraction of water-soluble sugars followed by mild NaOH or liquid hot water pretreatment of the extracted bagasse. A novel processing scheme was investigated that could integrate with current diffuser-type extraction systems for sugar extraction. In this approach, mild NaOH pretreatment (i.e., <90°C) was performed as a counter-current extraction to yield both an extracted, pretreated bagasse and a high-concentration mixed sugar stream. Following hydrolysis of the bagasse, the combined hydrolysates derived from cellulosic sugars and extractable sugars were demonstrated to be fermentable to high ethanol titers (>8%) at high metabolic yields without detoxification using a Saccharomyces cerevisiae strain metabolically engineered and evolved to ferment xylose.
Introduction
Ethanol derived from sucrose and starch crops has seen substantial growth in recent years (Babcock, 2012), and a non-trivial fraction of the agricultural output of some countries such as the U.S. and Brazil are currently devoted to supply these first-generation fuel ethanol processes. Continued growth in the global capacity for renewable biofuels and bio-based products requires alternative feedstocks such as lignocellulosic biomass. Technologies have begun to be commercialized that are able to utilize the polysaccharides that comprise cell walls of plants (i.e., lignocellulose) as a feedstock for fermentation-derived ethanol (Schwab et al., 2016). However, the nascent cellulosic biofuel industry faces challenging economics, with a high cost of cellulosic sugars relative to starch and sucrose due to the high capital and operating costs associated with large centralized lignocellulosic biorefineries (Dale, 2017). Approaches to address this challenge include improving process economics through novel processing approaches, novel or improved biomass feedstocks, and the production of additional co-products from the biomass. The low bulk density of herbaceous feedstocks such as corn stover and switchgrass represents an important challenge for the logistics of feedstock transport and storage for commercial-scale biorefineries. A lignocellulosic biorefinery that uses a biological process consisting of pretreatment such as dilute acid or dilute aqueous ammonia, enzymatic hydrolysis, and fermentation to produce cellulosic ethanol will need to process on the order of 2,000 dry tons per day of biomass to generate comparable ethanol yields as a typical dry grind corn ethanol plant (50 MM gal/yr). This presents an enormous set of logistical and storage challenges for these low bulk density feedstocks. One processing approach that addresses this challenge is decoupling select components, such as feedstock handling and potentially processing such as pretreatment, from the centralized biorefinery (Thompson et al., 2013). Decentralized biomass aggregation and preprocessing facilities that feed into larger centralized biorefineries have been proposed as solutions to the challenges associated with feedstock logistics. As one example, decentralized, depot-scale preprocessing that employs AFEX pretreatment and pelletization could provide a higher bulk-density feedstock that facilitates transportation and storage, enabling year-round supply, decreasing the sensitivity to supply chain disruptions, and potentially yielding a product with additional applications, such as a high-digestibility ruminant feed (Campbell et al., 2013; Lamers et al., 2015).
Another approach to addressing the economic challenges of cellulosic ethanol is by developing feedstocks with improved agronomic or processing attributes. Sorghum (Sorghum bicolor L. Moench) is one potential advanced feedstock that has been proposed due to a number of positive attributes. Based on whether the plant partitions significant stocks of carbon into grain, stem sucrose, or structural biopolymers, sorghum is classified as grain sorghum, sweet sorghum, and forage/silage/energy sorghum, respectively (Rooney et al., 2007). Proposed feedstock benefits of sorghum include its genetic tractability and diversity (Mullet et al., 2014), high biomass productivity (Rooney et al., 2007; Olson et al., 2012), and, when utilized for biofuel production, substantial greenhouse gas reduction benefits relative to gasoline (Cai et al., 2013). Additionally, like sugarcane, some sorghums (e.g., sweet and forage/silage/energy) accumulate water-extractable sugars (sucrose, fructose, glucose, and starch) in stems that are readily fermentable to ethanol (Eggleston et al., 2013). Unlike sugarcane, however, some cultivars of sorghum are suitable for cultivation in temperate climates, including much of the continental U.S. (Rooney et al., 2007). Sorghum cultivars/hybrids may be highly suitable for marginal lands as they can require less than two thirds of the water and less fertilizer compared to corn, with some varieties showing high drought tolerance (Eggleston et al., 2013). Further environmental benefits include advantages in process water utilization relative to starch-derived ethanol or other cellulosic ethanol technologies in that, as with sugarcane ethanol, high-moisture biomass is transported to the processing plant, while water and nutrients can be returned to field as vinasse (Chum et al., 2014).
The ability of sucrose-accumulating sorghum varieties to partition a significant fraction of the fixed carbon into water-extractable sugars suggests that hybrid processing schemes can be developed that integrate first- and second-generation biofuels technologies. Like sugarcane, sucrose-accumulating sorghum varieties can lose sucrose content quickly post-harvest, often within hours of being cut (Eggleston et al., 2013), which would require that stalks be processed immediately, and sugars be stabilized or potentially fermented at decentralized biomass processing depots before being transported to a centralized biorefinery for further processing. Another consideration for these processes is that, like sugarcane, the harvest period defines a limited window of operation, therefore many sugarcane processing mills only operate for a portion of the year. This limited feedstock lifetime, low bulk density, and high moisture all suggest that sorghum may lend itself to decentralized processing. A number of biofuels processing technologies have been proposed specifically for sweet sorghum utilization, which could be integrated into decentralized depot-scale processing facilities, making use of both the soluble sugars and structural polysaccharides within the biomass. Examples of sweet sorghum utilization include sucrose extraction and fermentation followed by ensiling the bagasse prior to anaerobic digestion (Zegada-Lizarazu and Monti, 2015), solid-state fermentation of soluble sugars to ethanol followed by alkaline pretreatment, enzymatic hydrolysis, and fermentation (Li et al., 2013), direct anaerobic digestion (Matsakas et al., 2014), and integration of ensiling with enzymatic hydrolysis of cell wall polysaccharides for ethanol production (Henk and Linden, 1994).
Sorghum harvest and processing for sugar extraction has been demonstrated using technologies developed for sugarcane (Noah and Linden, 1989; Woods, 2000; Webster et al., 2004), however maximum juice purities (i.e., sucrose, glucose, and fructose as a percentage of total solubles) tend to be lower, 75% for sorghum (Eggleston et al., 2013), relative to 88% for sugarcane (Webster et al., 2004) although this depends on feedstock properties. Commercial sugar or ethanol mills utilizing sugarcane employ counter-current extraction of sucrose from shredded stalks using either tandem roller-mills or diffuser extraction (Koster, 1995). Diffuser extraction is the most common technology, with extraction efficiencies as high as 98% for sugarcane, however it has some disadvantages compared to other methods, including higher levels of impurities and clarification requirements for sucrose production, and longer start-up and shut-down periods. However, extraction using diffusers also has lower capital costs, operating costs, and energy requirements relative to extraction by tandem milling (Rein, 1995). The most utilized sugarcane diffuser commercially is the counter-current moving bed diffuser with typical design capacities ranging from 1,000 to 40,000 wet tons sugarcane/day. For these processes, the sugarcane stems are first subjected to combinations of crushing, shredding, and/or milling with some diffuser designs extracting soluble sugar at this stage. The prepared sugarcane is next introduced to a porous screen and is conveyed along the screen while juice is collected under the screen in a series of 10 to 18 collection troughs. This juice is reintroduced to the moving bed of solids in the region one stage upstream from where it was collected, yielding a counter-current extraction process. Upon leaving the screen, the extracted bagasse is dewatered in roller mills (Baikow, 1967; Rein, 1995).
Besides their application for sugar extraction, counter-current solid-liquid extraction technologies have a wide range of existing and potential applications in biofuels processes, where the counter-counter action provides processing benefits over other process configurations such as sequential batch or co-current continuous processes. Examples include counter-current pretreatment by dilute acid (Lee et al., 1999) or liquid hot water (Thomsen et al., 2006) that have the benefits of minimizing soluble xylan exposure to high temperature and acid, reducing sugar degradation. Counter-current enzymatic hydrolysis of pretreated biomass has also been demonstrated as a concept to minimize product inhibition by high concentrations of sugars (Lonkar et al., 2017). Counter-current sucrose extraction from sugarcane is performed to maximize both sucrose concentrations and extraction efficiency by minimizing the soluble sugars remaining in the extracted bagasse. Recently, counter-current diffuser technology has been proposed as a pretreatment process (Borden et al., 2015). However, to our knowledge, combination of sucrose extraction and pretreatment using an integrated counter-current extraction has not been previously investigated and will be addressed in the present work.
The motivation for the current work is the development of a hybrid route for pretreatment and soluble sugar extraction that is suitable for decentralized biorefining. This work investigates the integration of mild NaOH pretreatment with counter-current soluble sugar extraction from an energy sorghum, utilizing both extractable and structural carbohydrates for biofuel production. Considering that minimal work has been published on this topic, this work is intended as a proof of concept to demonstrate the potential for this approach. Specifically, we compare the separate extraction and pretreatment using LHW and NaOH with the integrated approach and finally demonstrate the fermentability of the undetoxified combined juice extract, pretreatment liquor, and enzyme hydrolysate utilizing a yeast strain metabolically engineered to ferment xylose.
Materials and Methods
Lignocellulosic Biomass Feedstock
Sorghum bioenergy hybrid TX08001 was used in this work. This bioenergy feedstock is a photoperiod-sensitive hybrid exhibiting high nitrogen use efficiency (Olson et al., 2013) and extended vegetative growth duration due to delayed flowering resulting in high biomass yields (Olson et al., 2012; Gill et al., 2014). Sorghum was grown at the Texas A&M University Farm, College Station, Texas as previously described (Olson et al., 2013) and was harvested prior to floral initiation after 120–150 days of vegetative growth. Following harvest, sorghum stems that account for ~80% of total shoot biomass, were isolated, milled to pass a 5-mm screen, and dried to a moisture content of 7.3% and stored at 4°C in sealable bags. Composition analysis of the untreated sorghum stems was performed based on the NREL procedures for biomass analysis (Hames et al., 2008; Sluiter et al., 2008a,b,c, 2012), with alterations as specified by Ong et al. (2016). Extractives-free bagasse for sequential extraction, pretreatment, and hydrolysis was prepared by extensive washing of ~15 g of sorghum with 600 mL water at 80°C followed by filtration through a plastic funnel fitted with a 200-mesh porous base. The bagasse was then allowed to air dry before composition analysis and further use in batch pretreatment and hydrolysis experiments. Structural carbohydrate and lignin content of the bagasse and pretreated biomass were determined using the NREL analytical protocols NREL/TP-510-42618 with modifications as described previously (Li et al., 2012).
Liquid Hot Water and Alkaline Pretreatments of Extractives-Free Bagasse
Following extraction, air-dried bagasse was pretreated using two levels for pretreatment “severity” for both liquid hot water (LHW) and NaOH pretreatment. Rather than attempting to optimize the pretreatment conditions, these two levels were chosen to gauge the range of responses to pretreatment. The LHW pretreatments were performed at 15% solids (w/v) by adding 6 g (dry basis) of bagasse and a total of 40 mL of water (including biomass moisture) into a pressure tube (Ace Glass part number 8648-162) and sealed with a Teflon screwcap. For the “low severity” LHW pretreatment, the pressure tubes were placed in an autoclave for 1 h at 120°C. For the “high severity” LHW pretreatment, a 10 L M/K Systems digester (M/K Systems, Inc., Peabody, MA) was used as described in our prior work (Stoklosa and Hodge, 2015). For this, the reactor was filled with 5 L of water and pressure tubes were immersed in this water. The reactor was heated at a rate of 1°C/min until the target temperature of 160°C was reached, whereupon the reactor was held at 160°C for 1 h. After 1 h of reaction, the reactor was cooled at a rate of ~1.3°C/min until a temperature of 80°C was reached. The LHW-pretreated bagasse was then diluted to 10% solids (w/v) without washing and placed in flasks in preparation for hydrolysis. NaOH pretreatment was performed at two levels of pretreatment “severity” (based on NaOH loading) in shake flasks at 15% solids (w/v) and NaOH loadings of 0.1 and 0.06 g NaOH/g bagasse. The flasks were incubated at 80°C for 1 h in a water bath without stirring, and then diluted to 10% (w/w) solids without washing. The pH was adjusted to 5.5 using concentrated sulfuric acid in preparation for enzymatic hydrolysis.
Counter-Current Sugar Extraction and Alkali Pretreatment
Counter-current sugar extraction and sugar extraction plus NaOH pretreatment were performed using a series of five funnels and filter flasks (Supplemental Figure 1). The 3.8 cm diameter funnels were fitted with a 200-mesh stainless steel porous base. For counter-current extraction, 5.0 g of milled sorghum (dry, total mass basis) was introduced at one end of the series of funnels, designated Stage 1, washed with the filtrate from the following stage (Stage 2), and then moved to the next stage (Stage 2). This process was repeated sequentially until the biomass reached Stage 5, at which point fresh imbibition water (50 mL) was passed through the extracted bagasse and subjected to filtration under mild vacuum. The filtrate from each stage was moved sequentially to lower stage numbers during the process (Supplemental Figure 1). For example, the filtrate collected from Stage 5 was used to extract the biomass on Stage 4 and the final recovered filtrate was collected after extraction of biomass on Stage 1. A complete cycle was performed (biomass passing from Stage 1 to Stage 5) before the process was assumed to be at “steady-state.” Once this steady-state was reached, the process was continued until enough juice and bagasse had accumulated to perform the subsequent hydrolysis and fermentation experiments. For the integrated NaOH pretreatment and extraction, the filtrate from Stage 5 was mixed with 5 M NaOH to yield an alkali loading of 0.06 g NaOH/g extractives-free biomass, slurried with the bagasse from Stage 3, and incubated for 1 h at 80°C before being subjected to filtration at Stage 4. The bagasse exiting Stage 5 was immediately prepared for enzymatic hydrolysis. The extraction juice recovered from Stage 1 was filter-sterilized (250 mL Stericup-GP, 0.22 μm membrane, EDM Millipore) and stored at 4°C until fermentation was performed. The concentrations of glucose, fructose, and sucrose in each of the filtrates was determined by HPLC (Agilent 1,100 Series) equipped with an Aminex HPX-87H column (Bio-Rad, Milford, MA) and operated at 65°C and a flowrate of 0.6 mL/min with a mobile phase of 0.005 M H2SO4 and detection by refractive index.
Enzymatic Hydrolysis
For the batch LHW- and alkali-pretreated bagasse samples, pretreated material was diluted to 10% (w/w) solids and the pH adjusted to 5.5 as needed. Following this, sodium citrate buffer (50 mM, pH 5.5), tetracycline (10 mg/L), and cycloheximide (10 mg/L), respectively, were added to the hydrolysates to inhibit microbial growth during hydrolysis. Cellic® CTec2 and HTec2 (Novozymes A/S, Bagsværd, Denmark) were added in a protein ratio of 2:1 for an enzyme loading of 15 mg enzyme/g glucan (12.6 FPU/g glucan for the CTec2). The total protein contents of enzyme cocktails used in determining enzyme loadings on biomass were quantified using the Bradford Assay (Sigma-Aldrich). Samples were then incubated at 50°C for 7 days. For the combined sugar extraction and pretreatment process, the bagasse leaving Stage 5 was diluted to ~18% solids (w/v) and the pH adjusted to 5.5 using concentrated sulfuric acid. Citrate buffer, antibiotics and enzymes were added and samples were incubated as described above without the cycloheximide, and then filter-sterilized and stored at 4°C until fermentation was performed. Sugar concentrations in the hydrolysates were determined by HPLC using the method described above and converted to glucose and xylose yields based on the glucan and xylan contents of the untreated bagasse.
Fermentation
Fermentation of the combined extracted juice from the integrated extraction and NaOH pretreatment and enzymatic hydrolysate was performed using Saccharomyces cerevisiae strain GLBRCY73 expressing xylose reductase (XYL1), xylitol dehydrogenase (XYL2), and xylulokinase (XYL3) from Scheffersomyces (Pichia) stipitis and evolved to grow on xylose as described in our prior work (Sato et al., 2014). Juice and hydrolysate were mixed in a 60:40 v/v ratio, which is equivalent to the relative abundance of these liquors from the procedure. A second liquor was generated by vacuum evaporation (Buchi Rotavapor R114) of the combined extraction liquor and hydrolysate to yield a sugar stream concentrated by ~6-fold with 325 g/L of total mixed monosaccharides (primarily glucose, xylose, fructose, and sucrose). Yeast nitrogen base (YNB) and urea were added to sterile, mixed-sugar hydrolysates at concentrations of 1.67 and 2.27 g/L, respectively. Yeast seed cultures were prepared by inoculating 50 mL of YPD medium (10 g/L yeast extract, 20 g/L peptone and glucose) with the glycerol stock of GLBRCY73 and incubating for 24 h at 30°C with 150 rpm orbital shaking. After 24 h, 10 mL of culture was transferred aseptically to 60 mL of the liquor/hydrolysate mixture in a shake flask, in duplicate. The flasks were covered with fermentation locks, sparged with N2, and incubated at 30°C with orbital shaking at 150 rpm for 7 days. Samples were collected every 24 h to determine OD600 by spectrophotometer and sugar and ethanol concentrations by HPLC (Agilent 1100 series) as described above.
Results and Discussion
Sorghum Composition
The composition of the TX08001 sorghum on a total dry mass basis is presented in Table 1 and shows the content of both extractives as well as cell wall biopolymers. Prior research on sorghum stems showed that significant compositional and structural heterogeneity among cell and tissue types and stage of plant development (Li et al., 2018). The current analysis averages these diverse sub-cellular compositions and examines sugar content only during vegetative phase growth when stem sucrose levels are relatively low compared to levels that accumulate post-anthesis (McKinley et al., 2016). At the growth stage analyzed, the combined structural and extractable carbohydrate contents account for 64% of the dry mass of the plant and that 18% of this sugar is water-extractable: primarily sucrose, glucose, fructose, and polymeric glucan (i.e., starch and mixed-linkage glucan). Notably, the water-extractable polymeric glucan represents 7.7% of the water-extractable carbohydrates. This composition can be contrasted to other graminaceous feedstocks such as corn stover and switchgrass that contain comparable or slightly less total carbohydrates on a dry mass basis, but which is overwhelmingly comprised of structural polysaccharides (Ong et al., 2016; Williams et al., 2017). The implication is that a significant fraction of the sorghum plant mass is in the form of readily assimilable carbohydrates for microbial conversion to biofuels, which would not require pretreatment and enzymatic hydrolysis for utilization, although requiring immediate utilization or stabilization following harvest. Another key result is that 57% of the extractable sugars were monosaccharides (glucose and fructose), while only 33% was sucrose. This is consistent with prior studies showing that during the vegetative phase, sorghum stems accumulate high levels of glucose and fructose compared to sucrose (McKinley et al., 2018). Some sucrose may also be hydrolyzed/interconverted during processing, however, relative to sugar mills, preventing sucrose inversion and high sucrose purity are not critical for processes fermenting these sugars.
Integration Concepts for Counter-Current Carbohydrate Extraction and Cell Wall Deconstruction
Two potential concepts for integrating recovery and utilization of water-extractable sugars and plant cell wall-derived polysaccharides were evaluated (Figure 1). The first concept uses separate unit operations for the extraction of sugars and subsequent deconstruction of the structural polysaccharides in the bagasse during pretreatment and enzymatic hydrolysis (Figure 1A). Utilizing counter-current extraction in this processing approach would yield a relatively clean, high-concentration of water-extractable sugars (and extractable starch) and, depending on the how the process is integrated, either separate or combined pretreatment liquors and hydrolysate sugar streams derived from the enzymatic hydrolysis of the pretreated bagasse. The water-insoluble hydrolysis residue would be primarily lignin with some unhydrolyzed carbohydrates that could be burned for process energy. The pretreatment liquors would contain a significant number of pretreatment-solubilized compounds including xylan/xylose, lignin (minimal for LHW), acetate, and degradation products derived from sugar and lignin depending on the pretreatment process.
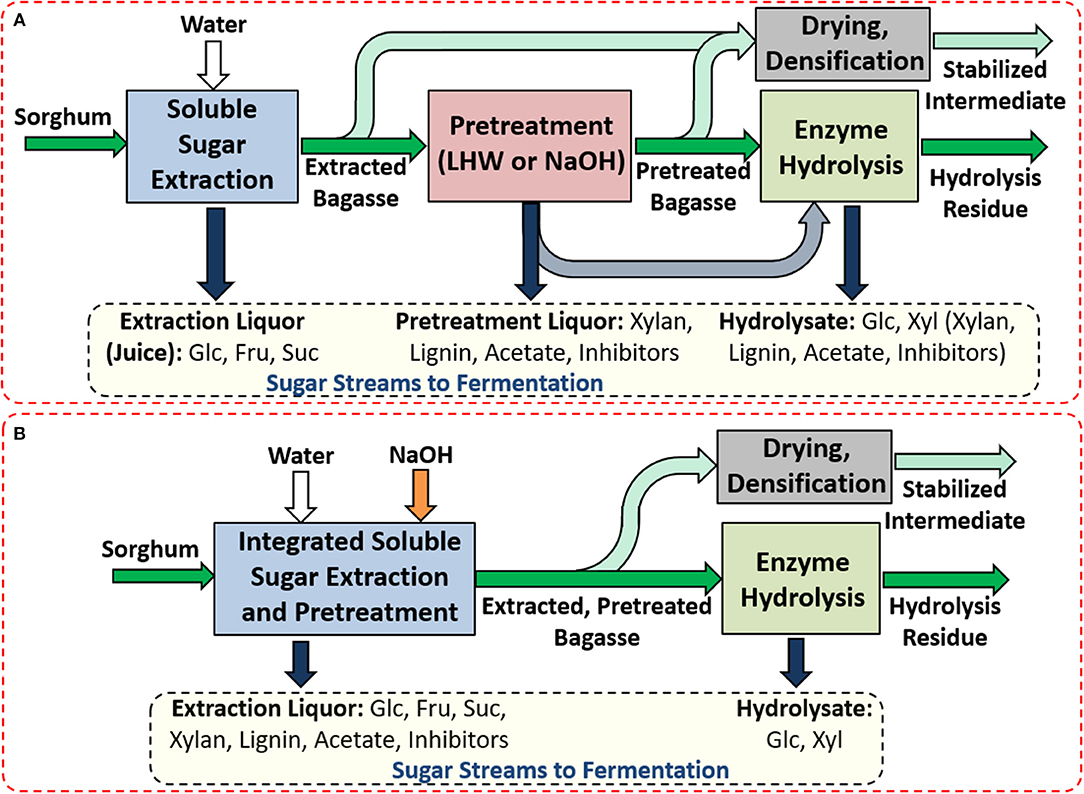
Figure 1. Potential processing schemes for integrating sugar extraction and bagasse pretreatment and hydrolysis of sorghum to generate sugar streams that can be utilized for fuel production, or other sugar-based products with potential stabilization for storage and subsequent transportation to a centralized biorefinery by drying and densification. These processing configurations include (A) separate extraction of water-extractable compounds and deconstruction and (B) integrated deconstruction and extraction. The separate deconstruction employs either alkali pretreatment (0.10 g NaOH or 0.06 g NaOH per g extractives-free biomass at 80°C) or liquid hot water pretreatment (120°C or 160°C for 1 h), while the integrated deconstruction and extraction employs an alkali pretreatment with the equivalent of 0.06 g NaOH per g extractives-free biomass at 80°C as one of the extraction stages during counter-current sucrose extraction.
The second concept integrates sugar extraction with mild alkali pretreatment in the same unit operation (Figure 1B). In this configuration, the process acts as a combined extraction process for soluble sugars and a leaching/lixiviation process of the extracted cell wall biopolymers. This process would yield an extraction liquor that would contain, in addition to water-extractable sugars and starch, pretreatment-derived compounds such as Na+, lignin, acetate, and xylan, which would not be desirable in conventional sugar mill, but are not necessarily problematic for ethanol fermentation. A second stream would comprise the hydrolysate of cell wall polysaccharides (glucose and xylose) that would be relatively free from pretreatment-derived contaminants. If these approaches were performed at decentralized bioprocessing depots rather than at a centralized biorefinery, the extraction and pretreatment could be coupled with a subsequent drying and densification of the biomass (Figure 1). This approach would enable the conversion of a high-moisture, low-bulk density, unstable (due to the degradable sugar content), seasonally available feedstock into a high-bulk density, stable, storable intermediate product that is amenable to further enzymatic deconstruction in a cellulosic biofuels process or, alternatively, may serve as a high-digestibility feed for ruminants or as a feedstock for anaerobic digestion as suggested in the prior literature (Bals and Dale, 2012).
Integration of the pretreatment with the soluble sugar extraction has the potential to yield a number of advantages relative to other processing configurations. First, by performing this approach in a counter-current manner with the sugar extraction primarily taking place in the initial stages and the pretreatment taking place on one or several of the later stages, the high concentrations of water-extractable carbohydrates are not subjected to the high pH values that would degrade reducing sugars (i.e., containing an anomeric carbon not involved in a glycosidic bond) such as glucose and fructose (De Bruijn et al., 1986). One advantage of this process is that three outcomes are achieved simultaneously: (1) the soluble sugars are extracted at high yields and concentrations, (2) the biomass is pretreated, and (3) the pretreated biomass is washed prior to enzymatic hydrolysis. Additionally, relative to a sequential extraction and pretreatment process (Figure 1A), the integrated process (Figure 1B) offers the potential for substantial savings in process water use as the water used for sugar extraction and pretreatment are the same and can be derived in part from high moisture biomass such that additional concentration of an extraction juice with a pretreatment liquor would not be necessary to achieve the same sugar concentrations.
A commercial sugarcane diffuser may be comprised of more than 10 extraction stages with typical residence times of 5 min per stage, for total material residence times in the diffuser ranging from 40–60 min at an extraction temperature of 80 to 85°C (Buchanan, 1967; Love and Rein, 1980; Breward et al., 2012). Mild NaOH pretreatment may be performed in this temperature range with residence times of 1 h (Stoklosa and Hodge, 2012). The time required for the mild alkaline pretreatment is a combination of reaction rate and diffusion of alkali into the cell walls where diffusion has been proposed to be the rate-limiting step during the initial stages of delignification at low temperatures (Olm and Tistad, 1979). As the name implies, molecular diffusion of sucrose out of the biomass is the primary mechanism for mass transfer during extraction in a diffuser (Buchanan, 1968), and it is possible that the pretreatment may be complete after only 10–20 min. This could take place over multiple stages or stage hold-up times could be modified for longer times in the pretreatment section. Finally, in addition to the single concept outlined, many variations can be envisioned, such as inclusion of enzymes during extraction to hydrolyze starch, xylans, or mixed-linkage glucans (i.e., β-glucans) or further integrate with enzymatic hydrolysis of cell wall polysaccharides in stages following the pretreatment.
Separate Carbohydrate Extraction and Deconstruction
Counter-current extraction of water-extractable carbohydrates from the sorghum was performed in a laboratory approximation of a diffuser extraction process using 5 stages (Figures 1A, 2C). The sugar concentration profiles using this extraction approach demonstrate that relatively high sugar concentrations (i.e., 80.1 g/L sucrose, glucose, and fructose) can be achieved (Figure 2B). It should be noted that 7.7% of the extractable carbohydrates were polymeric glucan (i.e., starch) that was not quantified in this set of studies. The mass of extractable sugars estimated to remain entrained in the bagasse in stream S5 was >3% of the mass of the extractable sugars entering the process in stream S0. Thus, the calculated extraction efficiency of this process is high (i.e., 97%) and is comparable to that of commercial diffuser systems (Rein, 1995). One factor that contributes the high sugar concentrations in the extraction liquor is that dried sorghum at a moisture of ~7% was used in this work, resulting in significant sorption of water in Stage 1 and a low volume of extraction juice recovery in stream L0 (13.5 mL) relative to the volumes of juice in all other streams (43.5–49.5 mL for streams L1-L4, and 50 mL of imbibition water in stream L5). The estimated water content of the biomass for each stage following extraction (% of total mass) was found to be relatively consistent and ranged from 89% on the first stage of the extraction (i.e., Stage 1) to a maximum of 91% on the last stage (i.e., Stage 5). This can be contrasted to commercial diffuser processes for sucrose extraction from sugarcane, where the biomass is fed at 70% moisture (rather than 7%), reaches a moisture content of ~85% during diffusion process (comparable to the results in the present study), leaves diffuser, and is dewatered to ~30% moisture (Breward et al., 2012). The low moisture content of the entering sorghum (S0) that results in the substantial sorption of juice from stream L1 is why there is the increase in sugar mass (but not concentration) between streams L0 and L1. As the moisture content of the bagasse leaving the last stage (i.e., stream S5) in our work is 90%, pressing out more juice would obviously result in even high sugar extraction yields. It should also be noted that based on mass balances, the extraction process had not reached steady-state and consequently the estimated sugar extraction yields (Figure 2B) are higher than the theoretical yields based on the original composition (Table 1).
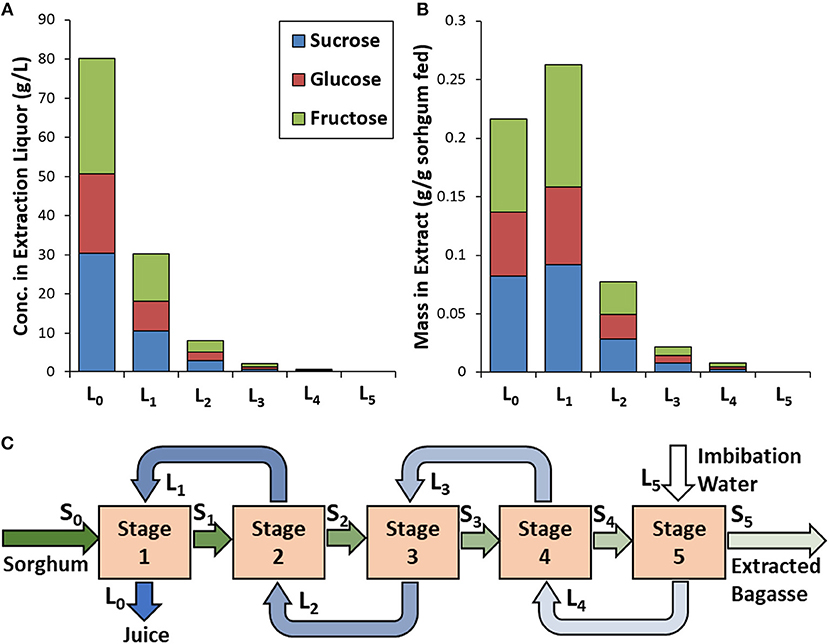
Figure 2. Example of counter-current extraction of sorghum sugars for the separate extraction and deconstruction approach showing results for (A) extractable sugar concentration, (B) mass yield of extractable sugar concentration in the extraction liquor as a function of extraction stage, and (C) identification of processing streams. Starch content in extraction liquors was not quantified.
For biological deconstruction and conversion of plant cell wall polysaccharides, pretreatments are a necessary step to improve the accessibility of these polysaccharides to hydrolytic enzymes for the enzymatic generation of cellulosic sugars (Ong et al., 2014). While a wide range and combination of solvents, pH, and temperatures can and have been used in the past to effectively pretreat biomass, any chemical inputs into the process can become a liability. The pretreatment chemicals must be either minimized or recovered with high efficiency to minimize process costs, integration of pretreatment chemicals/solvents with downstream processes can be challenging, and any inorganics added during pretreatment may prevent significant recycling of process water.
Hydrothermal pretreatments have economic, technological, and environmental advantages in that, compared to all other pretreatments, these require no chemical inputs other than water or steam. These technologies have been extensively researched as pretreatments for the biochemical production of biofuels from bioenergy grasses and agricultural residues (Mosier et al., 2005; Ruiz et al., 2013, 2017). Hydrothermal pretreatments are typically performed at temperatures between 150 and 220°C with either liquid hot water (LHW, also called autohydrolysis), or steam, depending on whether the pressure is above or below the vapor pressure of water at operating temperature. One of the main outcomes of hydrothermal pretreatments is lignin melting and redistribution throughout the cell wall and the solubilization of a portion of the hemicellulose fraction as oligomers and acetic acid (Ong et al., 2014). Alkaline pretreatments are another class of promising pretreatments for the liberation of cell wall polysaccharides. Soda pulping of graminaceous feedstocks such as de-pithed sugarcane is practiced commercially, NaOH pretreatments are known to be effective at delignifying graminaceous biomass at relatively mild (i.e., <130°C) conditions, and these processes have been adapted to facilitate high sugar yields during enzymatic hydrolysis (Karp et al., 2014; Liu et al., 2014; Li et al., 2018). An additional advantage of mild alkaline pretreatments is that pretreatment-generated inhibitors are benign enough that a detoxification is not required to facilitate fermentation (Sato et al., 2014) as in other pretreatments such as dilute acid pretreatment (Jönsson et al., 2013). Furthermore, these pretreatments are effective at temperatures below 100°C, which can overcome the operational challenges of feeding biomass into a pressurized reactor. Alkali also lends itself to integration with diffuser technologies as lime is typically used in these processes for pH control. However, as a disadvantage, the use of NaOH during pretreatment necessitates the use of strategies such as alkali recovery to prevent accumulation of inorganics in recycled process water streams.
In the present work, the response of the extracted sorghum bagasse to deconstruction was assessed for separate extraction and either LHW or NaOH pretreatment (Figure 1A). For this, extractives-free sorghum bagasse was subjected to pretreatment at two levels each for LHW (1 h and either 120°C or 160°C) and NaOH pretreatment (1 h, 80°C, and either 0.06 or 0.10 g NaOH/g biomass) followed by enzymatic hydrolysis at an enzyme loading of 15 mg/g glucan for 7 days. As can be observed, the hydrolysis yields for the more severe conditions for both the NaOH and LHW pretreatments were higher than the lower severity conditions, while the NaOH pretreatment at an alkali loading of 0.10 g/g resulted in the highest glucose hydrolysis yields (Figure 3A). These hydrolysis yields are within the range identified in our prior work with TX08001 sorghum (Li et al., 2018), where mild NaOH pretreatment of sorghum fractionated by tissue type could result in glucose hydrolysis yields ranging from 53% to the theoretical maximum. It is well-understood that the general mechanisms of reducing recalcitrance in alkaline pretreatments is by delignification as well as minor xylan solubilization (Ong et al., 2014) and not surprisingly, the levels of delignification were highest for the NaOH pretreatment and increased with increasing severity (Figure 3B). In contrast, LHW pretreatment decreases plant cell wall recalcitrance by hydrolyzing xylan and melting and redistributing lignin throughout the cell wall (Ong et al., 2014) and results for mass loss (Figure 3C) and lignin removal (Figure 3B) agree with this mechanism whereby more mass is lost with increasing pretreatment severity although minimal lignin is removed.
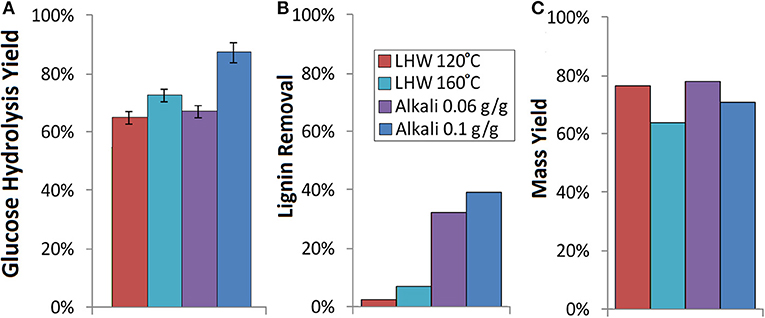
Figure 3. Results for separate extraction and deconstruction for two conditions each for alkali (0.06 or 0.10 g NaOH/g biomass at 80°C for 1 h) and liquid hot water (LHW) pretreatment (120°C or 160°C for 1 h) demonstrating differences in (A) glucose hydrolysis yields based on structural glucan in extractives-free biomass, (B) lignin removal based on quantified Klason lignin content and mass yields, and (C) mass yields from pretreatment based on extractives-free original mass. Enzymatic hydrolysis was performed using CTeC2 and HTec2 at a 2:1 ratio based on protein content at a protein loading of 15 mg/g glucan for a total hydrolysis time of 7 days.
Counter-Current Integrated Extraction and Pretreatment
As a proof of concept, an integrated extraction and pretreatment was performed at one experimental condition corresponding to the configuration previously outlined (Figures 1B, 4C) using 0.06 g NaOH/g extractives-free sorghum. For the integrated approach, sugar concentrations (Figure 4A) are slightly lower than in the extraction-only study (Figure 2A). Potential reasons for this discrepancy may be a combination of both the system not yet reaching steady-state and differences in water sorption between untreated and alkali-pretreated biomass. It is known that alkali-pretreated graminaceous biomass is capable of sorbing substantially more water than unpretreated biomass as demonstrated in our prior work with maize (Li et al., 2015) and sorghum (Li et al., 2018). Another important finding from this study is that the high pH (i.e., >12) reached during the pretreatment (Stage 4 and leaving in stream L3) is not propagated through the other process stages. That is, stream L3 is partially neutralized during Stage 3 by a combination of dilution with the entrained liquor in stream S2 as well as consumption of alkali by reaction with the biomass in stream S2 (e.g., through saponification of acetyl and hydroxycinnamoyl ester xylan and lignin). Furthermore, the high pH liquor entrained in the biomass entering Stage 5 (stream S4) is clearly partially neutralized by dilution with the fresh imbibition water in stream L5. The implications of this are (1) that neutralization with a strong mineral acid is not required for this process performed under these conditions and (2) as the majority of the soluble sugar extraction takes place in the first two stages, high concentrations of soluble sugars are never in contact with the high pH liquors. This is important as it is well-understood that under alkaline conditions, reducing sugars in the extraction liquors (i.e., glucose and fructose) are highly susceptible to enolization and subsequent degradation or aldol condensation (e.g., with phenolics) (Horváth et al., 2005), which could represent a significant loss of fermentable sugar. Finally, it was observed that the extraction liquor leaving the process (stream L0) dropped to a pH of 5.5 (Figure 4B). Fortuitously, this is the appropriate pH for fermentation by S. cerevisiae, indicating that no additional pH adjustment of this extraction liquor is necessary prior to fermentation.
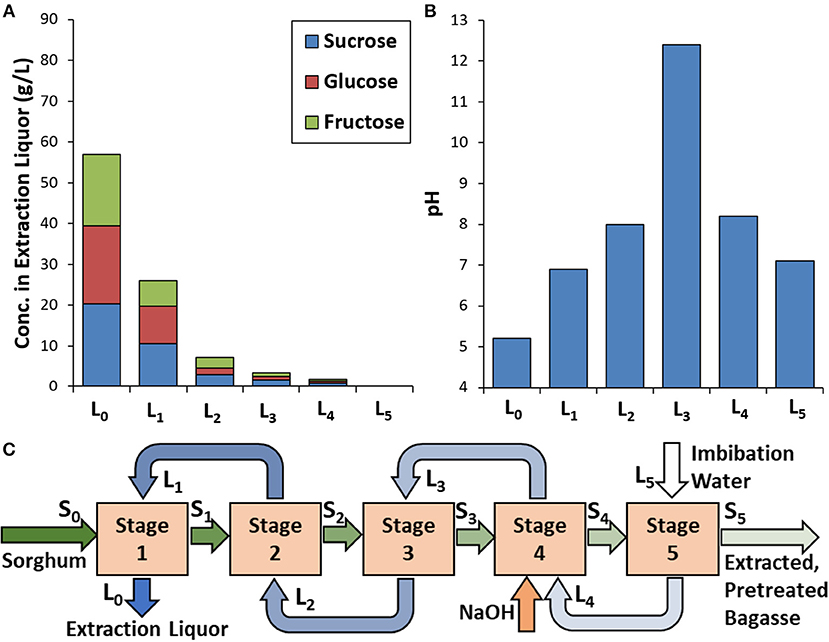
Figure 4. Results for (A) sugar concentrations and (B) pH in the extraction liquor as a function of extraction stage together with the (C) identification of processing streams during integrated counter-current extraction and mild NaOH pretreatment. Xylan, acetate, lignins, and starch in extraction liquors were not quantified.
Fermentation of Combined Hydrolysate and Extracted Sugars
The sugar streams derived from the integrated extraction and deconstruction of bioenergy sorghum (Figure 1B) were next subjected to fermentation by engineered S. cerevisiae strain GLBRCY73. This strain was previously developed through a combination of rational metabolic engineering to incorporate a xylose fermentation pathway into a background strain demonstrated to exhibit superior growth in the presence of pretreatment-derived inhibitors and evolutionary engineering to improve the xylose utilization rate (Sato et al., 2014). Challenges in performing fermentation of lignocelluosic hydrolysates include hydrolysate toxicity, the need to co-ferment glucose and xylose, and the need to generate hydrolysates at high sugar concentrations to minimize separation costs and process water usage. Hydrolysate toxicity to fermentation depends on the pretreatment technology employed and even the feedstock used where inhibitors include organic acids, phenolics, furans and inorganics (Palmqvist and Hahn-Hagerdal, 2000; Luo et al., 2002). Major fermentation inhibitors in pretreatment liquors derived from the mild alkaline pretreatment of grasses include hydroxycinnamic acids (p-coumaric and ferulic acid), acetate, Na+ as well as other unknown and poorly characterized and quantified extractives (Sato et al., 2014). As an example, prior research has clearly demonstrated that weak acids such as acetic acid are inhibitors of the rates of cell growth and ethanol fermentation and cell biomass yields in S. cerevisiae under anaerobic conditions (Narendranath et al., 2001). A key finding from prior work is that sugar hydrolysates combined with alkali pretreatment liquors are completely fermentable by yeast without detoxification (Liu et al., 2014; Sato et al., 2014).
In the present work, the sugar streams derived from the integrated pretreatment and sugar extraction were combined with the hydrolysate derived from the enzymatic hydrolysis of the extracted and pretreated sorghum bagasse. Combining extracted juice or syrup from sweet sorghum with lignocellulosic hydrolysate has the advantage of further diluting the pretreatment-derived inhibitors in the hydrolysate with the juice. Two sugar hydrolysates derived from these combined sugar streams were tested in this study. The first hydrolysate comprised the combined sugar streams at their original concentrations that were combined at an extraction juice to hydrolysate ratio of 60:40 (v/v), which dilutes both liquors to yield a total of 48.8 g/L mono- and disaccharides (not including starch). A second, more concentrated hydrolysate was generated by subjecting the first hydrolysate subjected to vacuum evaporation. Using this approach, a sugar syrup was produced with a more than 6-fold increase in concentration (i.e., 332 g/L total mono- and disaccharides) relative to the first, more dilute hydrolysate and was employed to test the limits of fermentation in terms of ethanol tolerance. Importantly, fermentations were performed without detoxification directly on these hydrolysates with only nitrogen supplementation.
Fermentation kinetics for the two hydrolysates (i.e., the combined extraction filtrate plus the hydrolysate and the concentrated filtrate plus hydrolysate) (Figure 5) reveal several key findings. First, for the low-concentration combined hydrolysate (Figure 5A), all the sucrose, glucose, and fructose are utilized within the first 16 h, while ~80% of the xylose was utilized after 7 days. This indicates that the inhibition of the fermentation by organic solubles as well as some sodium derived from the pretreatment in the combined juice/hydrolysate is minimal. This can be contrasted with fermentation of lignocellulose-derived hydrolysates utilizing other pretreatments such as dilute acid that typically require extensive detoxification or dilution (Jönsson et al., 2013) or organosolv pretreatments whereby the solvent must be removed from the biomass prior to biological conversion. Furthermore, a final ethanol titer of 21 g/L was obtained corresponding to a yield of 0.44 g ethanol generated per g sugar consumed, or ~85% of the theoretical maximum for ethanol fermentation. This lower value for the metabolic product yield is consistent with our prior work that found yields slightly lower than typical yeast fermentations potentially due to higher glycerol yields in this strain (Liu et al., 2014; Sato et al., 2014).
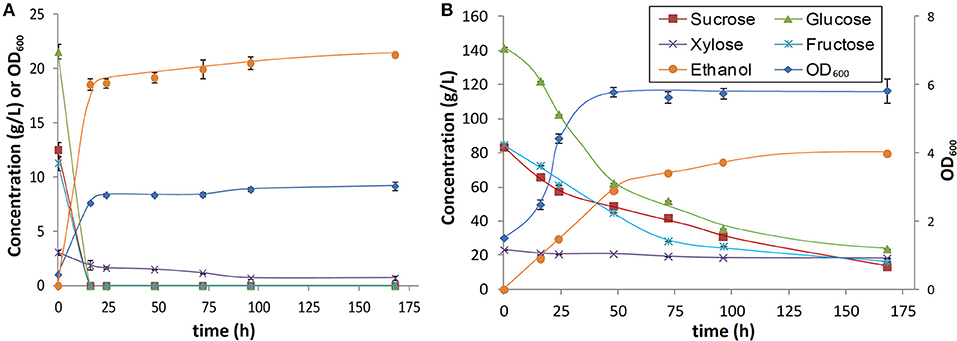
Figure 5. Kinetics for fermentation using S. cerevisiae GLBRCY73 strain of combined juice/hydrolysate at the (A) original concentration and (B) following 6-fold concentration by vacuum evaporation.
Typical challenges for industrial “very high gravity” yeast fermentation of starch hydrolysates or sucrose include high initial osmotic stress for cells resulting in slow initial growth and inhibited growth and fermentation when high ethanol titers are reached later in the fermentation for batch fermentations (Häggström et al., 2014). Both these phenomena are apparent in the kinetic profile of the high-sugar hydrolysate (Figure 5B). It can be observed that yeast growth was notably slower than in the more dilute hydrolysate and 3 days was required for the cell density to reach the maximum level achieved in the low (i.e., an OD600 of ~6). This slower growth as well as the longer lag phase can presumably be attributed to the osmotic stress exerted by the high sugar concentrations as well as potentially the increased concentration of pretreatment-derived inhibitors. Sugar consumption was also significantly slowed and ~15–25 g/L each of sucrose, fructose and glucose were still remaining after 7 days of fermentation, compared to complete consumption of these sugars in >18 h for the more dilute hydrolysate. These results for fermentation of the undetoxified high-sugar syrup presumably demonstrate the limits of ethanol tolerance for this strain and the final ethanol titer of 80 g/L was sufficient to halt growth and fermentation. The ethanol yield per consumed sugar is slightly lower than the yield for the low sugar concentration hydrolysate and may be attributed to the diversion of carbon in the substrate to glycerol, which is known to occur in S. cerevisiae to offset the inhibitory effects of high osmotic pressure (Nevoigt and Stahl, 1997).
Conclusions
An innovative hybrid approach to sugar extraction and pretreatment of energy sorghum was investigated in this work to demonstrate the potential of integrating mild alkaline pretreatment with counter-current sugar extraction. Hybrid energy sorghum TX08001 was found to be comprised of 64% carbohydrates as a percentage of the total dry mass of the sorghum, of which 27% of this sugar is water-extractable sucrose, fructose, glucose, and unidentified glucan. Laboratory counter-current extraction approximating diffuser extraction used for sugarcane processing was demonstrated to be capable of extracting 97% of the water-extractable sugars in 5 extraction stages, yielding extractives-free bagasse and an extraction juice containing 80.1 g/L of sucrose, fructose, and glucose. Extractives-free bagasse resulting from this process was susceptible to deconstruction by both NaOH and LHW pretreatments, with subsequent glucose hydrolysis yields ranging from 64.9% for the low severity LHW pretreatment (1 h at 120°C) to 87.3% for the most severe NaOH pretreatment condition (1 h at 80°C and 0.10 g NaOH/g biomass). It can be highlighted that these yields were achieved with the moderate enzyme of loadings of 15 mg protein/g glucan in the presence of all pretreatment-derived solubles. Not surprisingly, it was found that increasing NaOH loading during alkaline pretreatment improved lignin removal and hydrolysis yields and increasing temperature during LHW pretreatment improved hydrolysis yields.
Combined counter-current soluble sugar extraction and NaOH pretreatment at the mild conditions of 0.06 g NaOH/g extractives-free biomass (1 h at 80°C) was next demonstrated. It was identified that, again, high sugar extraction yields and titers could be achieved while simultaneously pretreating the biomass and generating a pretreated, extractives-free biomass pulp that was susceptible to enzymatic hydrolysis. Interestingly, the high pH (>12) of the liquors extracted from the pretreatment stage of the process was completely neutralized over the subsequent extraction stages, such that the final liquor pH exiting the process was only 5.5 and that the high concentrations of water-extractable sugars were never subjected to alkaline pH at elevated temperatures, which would degrade any free reducing sugars in the extract. Furthermore, the pH of the extraction liquor is already optimal for fermentation and no additional acidification is necessary. Finally, the extraction/pretreatment liquor from the integrated process was combined with the enzymatic hydrolysate to yield a mixed sugar solution with a concentration of 48.8 g/L total fermentable sugars (sucrose, glucose, fructose, and xylose). This was found to be completely fermentable by a S. cerevisiae strain engineered for xylose fermentation. Following a more than 6-fold concentration of this sugar stream by vacuum evaporation, an ethanol titer of 80 g/L could be achieved, however a significant fraction (21%) of the sugars remained unfermented, presumably due to inhibition of yeast growth and fermentation due to ethanol toxicity, potentially in combination with pretreatment-derived inhibitors. Additional variations of this integrated approach for soluble sugar extraction and pretreatment can be envisioned and may offer potential for novel depot-scale processing configurations such as subsequent drying and densification of extracted, pretreated sorghum to convert an unstable, transiently available feedstock into a stable intermediate product that can be stored to provide a continuous supply to a centralized biorefinery.
Author Contributions
DH and DW planned the experiments, analyzed the results, and wrote the manuscript. JM provided critical insights and ideas into sorghum processing that were used in this study. RO performed the detailed compositional analysis of the unextracted sorghum. All authors read, edited, and approved the final draft of the manuscript.
Funding
Research carried out in the laboratories of JM and RO was funded in part by the Great Lakes Bioenergy Research Center, U.S. Department of Energy, Office of Science, Office of Biological and Environmental Research under Award Numbers DE-SC0018409 and DE-FC02-07ER64494. The authors thank Dr. Trey Sato (GLBRC) for provision of the yeast strain used in this work, Bill Rooney (Crop & Soil Sciences, Texas A&M) for providing and planting the energy sorghum hybrid TX08001, and Brian McKinley (Biochemistry and Biophysics, Texas A&M) for collecting the stem samples used for the analysis.
Conflict of Interest Statement
The authors declare that the research was conducted in the absence of any commercial or financial relationships that could be construed as a potential conflict of interest.
Supplementary Material
The Supplementary Material for this article can be found online at: https://www.frontiersin.org/articles/10.3389/fenrg.2018.00133/full#supplementary-material
References
Babcock, B. A. (2012). Outlook For Ethanol and Conventional Biofuel Rins in 2013 and 2014. Iowa State University, Center for Agricultural and Rural Development Policy Brief 12-PB 9. Ames, IA.
Bals, B., and Dale, B. E. (2012). Developing a model for assessing biomass processing technologies within a local biomass processing depot. Biores. Technol. 106, 161–169. doi: 10.1016/j.biortech.2011.12.024
Borden, J., Garrett, J. B., and Shabaker, J. W. (2015). Counter-Current Diffuser Technology for Pretreatment of Lignocellulosic Substrates. U.S. Patent Application No US20150047629A1. Washington, DC: U.S. Patent and Trademark Office.
Breward, C., Hocking, G., Ockendon, H., Please, C., and Schwendeman, D. (2012). Modelling the extraction of sugar from sugarcane in a diffuser. Proc. 2012 South African MISG, Capetown, South Africa, 31–57.
Buchanan, E. (1967). The appraisal of diffusion performance without confusion. Proc. S. Afr. Sug. Technol. Assoc. 41, 94–100.
Buchanan, E. (1968). The calculation of stage efficiency and its application to diffuser design. Proc. S. Afr. Sug. Technol. Assoc. 42, 65–72.
Cai, H., Dunn, J. B., Wang, Z., Han, J., and Wang, M. Q. (2013). Life-cycle energy use and greenhouse gas emissions of production of bioethanol from sorghum in the United States. Biotechnol. Biofuels 6:141. doi: 10.1186/1754-6834-6-141
Campbell, T. J., Teymouri, F., Bals, B., Glassbrook, J., Nielson, C. D., and Videto, J. (2013). A packed bed ammonia fiber expansion reactor system for pretreatment of agricultural residues at regional depots. Biofuels 4, 23–34. doi: 10.4155/bfs.12.71
Chum, H. L., Warner, E., Seabra, J. E. A., and Macedo, I. C. (2014). A comparison of commercial ethanol production systems from Brazilian sugarcane and US corn. BioFPR 8, 205–223. doi: 10.1002/bbb.1448
Dale, B. (2017). A sober view of the difficulties in scaling cellulosic biofuels. BioFPR 11, 5–7. doi: 10.1002/bbb.1745
De Bruijn, J. M., Kieboom, A. P. G., and van Bekkum, H. (1986). Reactions of monosaccharides in aqueous alkaline solutions. Carb. Res. 74, 157–175.
Eggleston, G., Cole, M., and Andrzejewski, B. (2013). New commercially viable processing technologies for the production of sugar feedstocks from sweet sorghum (Sorghum bicolor L. Moench) for manufacture of biofuels and bioproducts. Sugar Technol. 15, 232–249. doi: 10.1007/s12355-013-0229-6
Gill, J., Burks, P., Staggenborg, S., Odvody, G., Heiniger, R., Macoon, B., et al. (2014). Yield results and stability analysis from the sorghum regional biomass feedstock trial. Bioenerg. Res. 7, 1026–1034. doi: 10.1007/s12155-014-9445-5
Häggström, C., Rova, U., Brandberg, T., and Hodge, D. B. (2014). “Integration of ethanol fermentation with second generation biofuels technologies,” in Biorefineries: Integrated Biochemical Processes for Liquid Biofuels, eds. N. Qureshi, D.B. Hodge, and A.A. Vertès (Amsterdam: Elsevier), 161–187.
Hames, B., Scarlata, C., and Sluiter, A. (2008). Determination of Protein Content in Biomass. National Renewable Energy Laboratory Technical Report NREL/TP-510–42625.
Henk, L. L., and Linden, J. C. (1994). “Silage processing of forage biomass to alcohol fuel,” in Enzymatic Conversion of Biomass for Fuels Production, eds Himmel, M.E., Baker, J.O., and Overend, R.P. (Washington DC, ACS Symposium Series 566, American Chemical Society), 391–410. doi: 10.1021/bk-1994-0566.ch020
Horváth, I. S., Sjöde, A., Alriksson, B., Jönsson, L. J., and Nilvebrant, N.-O. (2005). Critical conditions for improved fermentability during overliming of acid hydrolysates from spruce. Appl. Biochem. Biotechnol. 124, 1031–1044. doi: 10.1385/ABAB:124:1-3:1031
Jönsson, L. J., Alriksson, B., and Nilvebrant, N.-O. (2013). Bioconversion of lignocellulose: inhibitors and detoxification. Biotechnol. Biofuels. 6:16. doi: 10.1186/1754-6834-6-16
Karp, E. M., Donohoe, B. S., O'Brien, M. H., Ciesielski, P. N., Mittal, A., Biddy, M. J., et al. (2014). Alkaline pretreatment of corn stover: Bench-scale fractionation and stream characterization. ACS Sus. Chem. Eng. 2, 1481–1491. doi: 10.1021/sc500126u
Koster, K. (1995). Some downstream effects resulting from diffusion compared with milling as published by the South African sugar industry. Proc. S. Afr. Sug. Technol. Assoc. 69, 201–204.
Lamers, P., Roni, M. S., Tumuluru, J. S., Jacobson, J. J., Cafferty, K. G., Hansen, J. K., et al. (2015). Techno-economic analysis of decentralized biomass processing depots. Biores. Technol. 194, 205–213. doi: 10.1016/j.biortech.2015.07.009
Lee, Y. Y., Iyer, P., and Torget, R. W. (1999). Dilute-acid hydrolysis of lignocellulosic biomass,” in Recent Progress in Bioconversion of Lignocellulosics, ed G.T. Tsao. (New York, NY: Springer), 93–115. doi: 10.1007/3-540-49194-5_5
Li, J., Li, S., Han, B., Yu, M., Li, G., and Jiang, Y. (2013). A novel cost-effective technology to convert sucrose and homocelluloses in sweet sorghum stalks into ethanol. Biotechnol. Biofuels 6:174. doi: 10.1186/1754-6834-6-174
Li, M., Heckwolf, M., Crowe, J. D., Williams, D. L., Magee, T. D., Kaeppler, S. M., et al. (2015). Cell-wall properties contributing to improved deconstruction by alkaline pre-treatment and enzymatic hydrolysis in diverse maize (Zea mays L.) lines. J. Exp. Bot. 66, 4305–4315. doi: 10.1093/jxb/erv016
Li, M., Yan, G., Bhalla, A., Maldonado-Pereira, L., Russell, P. R., Ding, S.-Y., et al. (2018). Physical fractionation of sweet sorghum and forage/energy sorghum for optimal processing in a biorefinery. Ind. Crops Prod. 124, 607–616. doi: 10.1016/j.indcrop.2018.07.002
Li, Z., Chen, C. H., Liu, T., Mathrubootham, V., Hegg, E. L., and Hodge, D. B. (2012). Catalysis with CuII(bpy) improves alkaline hydrogen peroxide pretreatment. Biotechnol. Bioeng. 110, 1078–1086. doi: 10.1002/bit.24793
Liu, T., Williams, D. L., Pattathil, S., Li, M., Hahn, M. G., and Hodge, D. B. (2014). Coupling alkaline pre-extraction with alkaline-oxidative post-treatment of corn stover to enhance enzymatic hydrolysis and fermentability. Biotechnol. Biofuels 7:48. doi: 10.1186/1754-6834-7-48
Lonkar, S., Liang, C., Bond, A., Darvekar, P., Brooks, H., Derner, J., et al. (2017). Countercurrent saccharification of lime-pretreated corn stover–Part 1. Biomass Bioenergy 96, 28–37. doi: 10.1016/j.biombioe.2016.10.013
Love, D., and Rein, P. (1980). Percolation behaviour of a cane diffuser. Proc. XVII Con. Int. Soc. Sugar Cane Technol. 17, 1900–1924.
Luo, C., Brink, D. L., and Blanch, H. W. (2002). Identification of potential fermentation inhibitors in conversion of hybrid poplar hydrolyzate to ethanol. Biomass Bioenergy 22, 125–138. doi: 10.1016/S0961-9534(01)00061-7
Matsakas, L., Rova, U., and Christakopoulos, P. (2014). Evaluation of dried sweet sorghum stalks as raw material for methane production. BioMed Res. Intern. 2014:731731. doi: 10.1155/2014/731731
McKinley, B. A., Olson, S. N., Ritter, K. B., Herb, D. W., Karlen, S. D., Lu, F., et al. (2018). Variation in energy sorghum hybrid TX08001 biomass composition and lignin chemistry during development under irrigated and non-irrigated field conditions. PLoS ONE 13:e0195863. doi: 10.1371/journal.pone.0195863
McKinley, B. A., Rooney, W., Wilkerson, C., and Mullet, J. E. (2016). Dynamics of biomass partitioning, stem gene expression, cell wall biosynthesis, and sucrose accumulation during development of Sorghum bicolor. Plant J. 88, 662–680. doi: 10.1111/tpj.13269
Mosier, N., Hendrickson, R., Ho, N., Sedlak, M., and Ladisch, M. R. (2005). Optimization of pH controlled liquid hot water pretreatment of corn stover. Biores. Technol. 96, 1986–1993. doi: 10.1016/j.biortech.2005.01.013
Mullet, J., Morishige, D., McCormick, R., Truong, S., Hilley, J., McKinley, B., et al. (2014). Energy sorghum—a genetic model for the design of C4 grass bioenergy crops. J. Exp. Bot. 65, 3479–3489. doi: 10.1093/jxb/eru229
Narendranath, N. V., Thomas, K. C., and Ingledew, W. M. (2001). Effects of acetic acid and lactic acid on the growth of Saccharomyces cerevisiae in a minimal medium. J. Ind. Microbiol. Biotechnol. 26, 171–177. doi: 10.1038/sj.jim.7000090
Nevoigt, E., and Stahl, U. (1997). Osmoregulation and glycerol metabolism in the yeast Saccharomyces cerevisiae. FEMS Microbiol. Rev. 21, 231–241. doi: 10.1111/j.1574-6976.1997.tb00352
Noah, K. S., and Linden, J. C. (1989). Extraction of ensiled sweet sorghum with a continuous countercurrent diffuser. Trans. ASAE 32, 1419–1425. doi: 10.13031/2013.31165
Olm, L., and Tistad, G. (1979). Kinetics of the initial stage of kraft pulping. Svensk. Papperstidn. 82, 458–464.
Olson, S. N., Ritter, K., Medley, J., Wilson, T., Rooney, W. L., and Mullet, J. E. (2013). Energy sorghum hybrids: functional dynamics of high nitrogen use efficiency. Biomass Bioenergy 56, 307–316. doi: 10.1016/j.biombioe.2013.04.028
Olson, S. N., Ritter, K., Rooney, W., Kemanian, A., McCarl, B. A., Zhang, Y., et al. (2012). High biomass yield energy sorghum: developing a genetic model for C4 grass bioenergy crops. BioFPR 6, 640–655. doi: 10.1002/bbb.1357
Ong, R. G., Chundawat, S. P. S., Hodge, D. B., Keskar, S., and Dale, B. E. (2014). “Linking plant biology and pretreatment: understanding the structure and organization of the plant cell wall and interactions with cellulosic biofuel production,” in Plants and BioEnergy, eds M.C. McCann, M.S. Buckeridge, and N.C. Carpita. (New York, NY: Springer), 231–253. doi: 10.1007/978-1-4614-9329-7_14
Ong, R. G., Higbee, A., Bottoms, S., Dickinson, Q., Xie, D., Smith, S. A., et al. (2016). Inhibition of microbial biofuel production in drought-stressed switchgrass hydrolysate. Biotechnol. Biofuels 9:237. doi: 10.1186/s13068-016-0657-0
Palmqvist, E., and Hahn-Hagerdal, B. (2000). Fermentation of lignocellulosic hydrolysates. II: inhibitors and mechanisms of inhibition. Biores. Technol. 74, 25–33. doi: 10.1016/S0960-8524(99)00161-3
Rein, P. (1995). A comparison of cane diffusion and milling. Proc. South Afr. Sugar Technol. Assoc. 69, 196–200.
Rooney, W. L., Blumenthal, J., Bean, B., and Mullet, J. E. (2007). Designing sorghum as a dedicated bioenergy feedstock. BioFPR 1, 147–157. doi: 10.1002/bbb.15
Ruiz, H. A., Rodríguez-Jasso, M. A., Fernandes, B. D., Vicente, A. A., and Teixeira, J. A. (2013). Hydrothermal processing, as an alternative for upgrading agriculture residues and marine biomass according to the biorefinery concept: a review. Renew. Sust. Energ. Rev. 21, 35–51. doi: 10.1016/j.rser.2012.11.069
Ruiz, H. A., Thomsen, H. T., and Trajano, H. L. (2017). Hydrothermal Processing in Biorefineries: Production of Bioethanol and High Added-Value Compounds of Second and Third Generation Biomass. Cham: Springer. doi: 10.1007/978-3-319-56457-9
Sato, T. K., Liu, T., Parreiras, L. S., Williams, D. L., Wohlbach, D. J., Bice, B. D., et al. (2014). Harnessing genetic diversity in Saccharomyces cerevisiae for fermentation of xylose in hydrolysates of alkaline hydrogen peroxide-pretreated biomass. Appl. Environ. Microbiol. 80, 540–554. doi: 10.1128/AEM.01885-13
Schwab, A., Warner, E., and Lewis, J. (2016). 2015 Survey of Non-starch Ethanol and Renewable Hydrocarbon Biofuels Producers. National Renewable Energy Laboratory Technical Report NREL/TP- 6A10–65519. doi: 10.2172/1236956
Sluiter, A., Hames, B., Ruiz, R., Scarlata, C., Sluiter, J., and Templeton, D. (2008a). Determination of Ash in Biomass. National Renewable Energy Laboratory Technical Report NREL/TP-510–42622.
Sluiter, A., Hames, B., Ruiz, R., Scarlata, C., Sluiter, J., and Templeton, D. (2008b). Determination of Sugars, Byproducts, and Degradation Products in Liquid Fraction Process Samples. National Renewable Energy Laboratory Technical Report NREL/TP-510–42623.
Sluiter, A., Hames, B., Ruiz, R., Scarlata, C., Sluiter, J., Templeton, D., et al. (2012). Determination of Structural Carbohydrates and Lignin in Biomass. National Renewable Energy Laboratory Technical Report NREL/TP-510–42618.
Sluiter, A., Ruiz, R., Scarlata, C., Sluiter, J., and Templeton, D. (2008c). Determination of Extractives in Biomass. National Renewable Energy Laboratory Technical Report NREL/TP-510–42619.
Stoklosa, R. J., and Hodge, D. B. (2012). Extraction, recovery, and characterization of hardwood and grass hemicelluloses for integration into biorefining processes. Ind. Eng. Chem. Res. 51, 11045–11053. doi: 10.1021/ie301260w
Stoklosa, R. J., and Hodge, D. B. (2015). Fractionation and improved enzymatic deconstruction of hardwoods with alkaline delignification. Bioenerg. Res. 8, 1224–1234. doi: 10.1007/s12155-015-9579-0
Thompson, D. N., Campbell, T., Bals, B., Runge, T., Teymouri, F., and Ovard, L. P. (2013). Chemical preconversion: application of low-severity pretreatment chemistries for commoditization of lignocellulosic feedstock. Biofuels 4, 323–340. doi: 10.4155/bfs.13.15
Thomsen, M. H., Thygesen, A., Jørgensen, H., Larsen, J., Christensen, B. H., and Thomsen, A. B. (2006). Preliminary results on optimization of pilot scale pretreatment of wheat straw used in coproduction of bioethanol and electricity. Appl. Biochem. Biotechnol. 129-132, 448–460. doi: 10.1007/978-1-59745-268-7_37
Webster, A., Hoare, C., Sutherland, R., and Keating, B. A. (2004). “Observations of the harvesting, transporting and trial crushing of sweet sorghum in a sugar mill,” in 2004 Conference of the Australian Society of Sugarcane Technology 26, (Brisbane, QLD) 1–10.
Williams, D. L., Crowe, J. D., Ong, R. G., and Hodge, D. B. (2017). Water sorption in pretreated grasses as a predictor of enzymatic hydrolysis yields. Biores. Technol. 245, 242–249. doi: 10.1016/j.biortech.2017.08.200
Woods, J. (2000). Integrating Sweet Sorghum and Sugarcane for Bioenergy: Modelling the Potential for Electricity and Ethanol Production in SE Zimbabwe. Dissertation. King's College London, University of London.
Keywords: sorghum, sucrose extraction, decentralized biorefining, pretreatment, cellulosic biofuels
Citation: Williams DL, Ong RG, Mullet JE and Hodge DB (2019) Integration of Pretreatment With Simultaneous Counter-Current Extraction of Energy Sorghum for High-Titer Mixed Sugar Production. Front. Energy Res. 6:133. doi: 10.3389/fenrg.2018.00133
Received: 25 May 2018; Accepted: 21 November 2018;
Published: 14 January 2019.
Edited by:
Allison E. Ray, Idaho National Laboratory (DOE), United StatesReviewed by:
Héctor A. Ruiz, Universidad Autónoma de Coahuila, MexicoNing Sun, Lawrence Berkeley National Laboratory (LBNL), United States
Copyright © 2019 Williams, Ong, Mullet and Hodge. This is an open-access article distributed under the terms of the Creative Commons Attribution License (CC BY). The use, distribution or reproduction in other forums is permitted, provided the original author(s) and the copyright owner(s) are credited and that the original publication in this journal is cited, in accordance with accepted academic practice. No use, distribution or reproduction is permitted which does not comply with these terms.
*Correspondence: David B. Hodge, ZGF2aWQuaG9kZ2UzQG1vbnRhbmEuZWR1