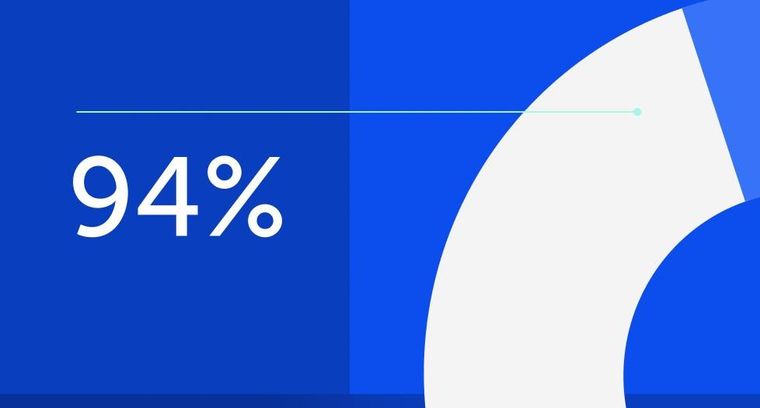
94% of researchers rate our articles as excellent or good
Learn more about the work of our research integrity team to safeguard the quality of each article we publish.
Find out more
ORIGINAL RESEARCH article
Front. Energy Res., 13 November 2018
Sec. Bioenergy and Biofuels
Volume 6 - 2018 | https://doi.org/10.3389/fenrg.2018.00107
This article is part of the Research TopicMicrobial Synthesis, Gas-Fermentation and Bioelectroconversion of CO2 and other Gaseous StreamsView all 13 articles
Domestic and industrial wastewaters contain organic substrates and nutrients that can be recovered instead of being dissipated by emerging efficient technologies. The aim of this study was to promote bio-hydrogen production and carbon fixation using a mixed culture of purple phototrophic bacteria (PPB) that use infrared radiation in presence or absence of an electrode as electron donor. In order to evaluate the hydrogen production under electrode-free conditions, batch experiments were conducted using different nitrogen (NH4Cl, Na-glutamate, N2 gas) and carbon sources (malic-, butyric-, acetic- acids) under various COD:N ratios. Results suggested that the efficiency of PPB to produce biogenic H2 was highly dependent on the substrates used. The maximum hydrogen production (H2_max, 423 mLH2/L) and production rate (H2_rate, 2.71 mLH2/Lh) were achieved using malic acid and Na-glutamate at a COD:N ratio of 100:15. Under these optimum conditions, a significant fixation of nitrogen in form of single-cell proteins (874.4 mg/L) was also detected. Under bio-electrochemical conditions using a H-cell bio-electrochemical device, the PPB were grown planktonic in the bio-cathode chamber with the optimum substrate ratio of malic acid and Na-glutamate. A redox potential of −0.5 V (vs. Ag/AgCl) under bio-electrochemical conditions produced comparable amounts of bio-hydrogen but significantly negligible traces of CO2 as compared to the biological system (11.8 mLCO2/L). This suggests that PPB can interact with the cathode to extract electrons for further CO2 re-fixation (coming from the Krebs cycle) into the Calvin cycle, thereby improving the C usage. It has also been observed during cyclic voltammograms that a redox potential of −0.8 V favors considerably the electrons consumption by the PPB culture, suggesting that the PPB can use these electrons to increase the biohydrogen production. These results are expected to prove the feasibility of stimulating PPB through bio-electrochemical processes in the production of H2 from wastewater resources, which is a field of special novelty and still unexplored.
Typical wastewater systems entail the dissipation of the contamination. However, the high content of organics and nutrients in industrial and domestic wastewaters is a valuable resource for energy and products recovery (Puyol et al., 2017a). Hence, upgrading of existing WWTP as resource recovery systems by implementing novel technologies, are mandatory steps considering economic and environmental benefits and recent policies within the circular economy.
Among the competing technologies, the biological accumulation of nutrients and their subsequent recovery, has received great attention as an environmental friendly and certainly cost-effective process (Batstone et al., 2015). Purple phototrophic bacteria (PPB) have shown significant accumulation of organics and nutrients from wastewater through assimilative processes (Batstone et al., 2015). PPB is a group of anaerobic facultative microorganisms, which can utilize infrared light (IR) as the main energy source. The use of PPB in the Partition-Release-Recovery concept proved to be far superior to other phototrophic organisms (as algae or cyanobacteria), since they achieve high growth rates and are not inhibited by O2 (Muñoz and Guieysse, 2006).
PPB are extremely versatile organisms due to their complex metabolic system, involving major C, N, S, P, and Fe pathways, which absorbs the IR energy through their photosystem, composed by carotenoids and bacteriochlorophyls (Hunter, 2008). Anoxygenic photosynthesis generates practically all the energy required for growth via the so-called cyclic electron flow (Klamt et al., 2008). In domestic wastewater treatment, the main metabolism follows photoheterotrophic growth on volatile fatty acids and sugars, although chemoheterotrophy (e.g., fermentation and anaerobic oxidation) can provide the necessary electrons for photoautotrophic growth (via hydrogen; Hülsen et al., 2014, 2016; Puyol et al., 2017a,b). The internal electron recycle, however, can be used for obtaining ammonium through dinitrogen gas fixation or directly dissipating electrons in the nitrogenase complex, which generates bio-hydrogen as the electron acceptor (Koku et al., 2002), or for direct internal accumulation of organic acids as poly-hydroxy-alcanoates (PHA) (Fulop et al., 2012). Moreover, the assimilative partitioning of wastewater macronutrients and organics through PPB leads to the production of one solid bacterial stream rich in proteins.
In this sense, PPB can be used for the extraction of high value-added products from waste sources, such as biofuels like bio-hydrogen, bioplastics as PHA and single-cell proteins. The metabolic pathways to obtain the valuable bioproducts are catalyzed by variant enzymes (McKinlay and Harwood, 2010). Monitoring the functionality of the involved bacteria and following-up their activity, can be of added value toward maximizing the bioproducts' formation. To add to the complexity of the above system, the end product depends greatly on the environmental conditions (IR light intensity, temperature, nutrients concentration, etc.). Thus, wastes rich in nitrogen are good sources for PPB growth producing biomass with high protein content (Verstraete et al., 2009), which can subsequently be used as additive animal food. In organic media lacking nutrients, PPB can accumulate high quantities of PHA, achieving up to 70–90% w/w (Mas and Van Gemerden, 1995). They are therefore an interesting alternative to fossil-fuels for plastics production. When the organic matter composition is quite high and is more reduced than biomass (i.e., butyrate), the excess of electrons (in absence of ammonium) are driven toward hydrogen production that can be used as a clean and renewable biofuel. Understanding the factors of importance and unraveling their relationship with the desired end bioproduct, remains one of the most important challenges in the ongoing research.
Finally, the internal electron recycling of PPB is a key issue, and an active modification of the electronic fluxes by means of artificial addition of electrons could drive toward different targeted bioproducts (Varfolomeyev, 1992). In this way, the concepts supporting microbial electrochemical technologies (METs) could be used to enhance the biochemical reactions of PPB by supplying electric current to microorganisms using electrodes as electron donors. In this context, METs have received great attention due to their potential applications in nitrate reduction (Pous et al., 2013; Tejedor et al., 2016), methanogenesis (Cheng et al., 2009) and microbial electrosynthesis (Logan and Rabaey, 2012). Likewise, the wise use of electricity to enhance PPB activity toward high value-added compounds (i.e., biohydrogen) through a bio-electrochemical system is undoubtedly an attractive challenge. PPB are highly electroactive organisms with high ability to generate bioelectricity through MFCs (Xing et al., 2008; Park et al., 2014). However, the use of electricity to enhance the PPBs metabolic activity aiming to produce high value-added bioproducts is an unexplored field with high growth potential in the short-term.
Based on the above-mentioned grounds, the aim of the present work was the assessment of PPB to enhance the formation of valuable bioproducts, such as biohydrogen, using electric and light energy as the driving forces. This was accomplished by identifying the biological and electrochemical conditions that influence the process of bio-hydrogen production from PPB. The wise use of electric energy to decontaminate wastewater and to produce bio-hydrogen is undoubtedly an attractive and novel challenge, yielding substantial ecological and economic benefits.
All the chemicals compounds used were purchased from Sigma-Aldrich. The organic compounds that were used were: L-malic acid (C4H6O5), butyric acid (C4H8O2), acetic acid (C2H4O2), propionic acid (C3H6O2) and ethanol (C2H6O). Stock solutions of individual organic compounds (20 gCOD/L) were prepared in ultra-pure water and stored at 4°C. The nitrogen sources used were: ammonium chloride (NH4Cl) as inorganic N-source, L-glutamic acid monosodium salt monohydrate (Na-glutamate, C5H8NNaO4·H2O) as organic N-source and nitrogen gas (N2) as external gaseous source. Stock solutions of both organic and inorganic nitrogen sources (5 gN/L) were prepared in ultra-pure water and stored at 4°C.
Finally, macro- and micro-nutrient solutions were prepared following the recipe proposed by Ormerod et al. (1961). The macro-nutrient solution contained 10.86 g K2HPO4·3H2O; 6.66 g KH2PO4; 2 g MgSO4·7H2O, 0.75 g CaCl2·2H2O; 69 mg FeCl2·4H2O and 0.2 g EDTA in 1 L ultra-pure water. The micro-nutrient solution contained 1.4 g H3BO3; 1.013 g MnCl2·4H2O; 274 mg (NH4)6Mo7O24·4H2O; 57 mg ZnCl2; 14 mg CuCl2·2H2O; 7.5 mg biotin and 1 g EDTA in 0.5 L ultra-pure water. The pH in all solutions was adjusted to 7.
All experimental tests were inoculated with a mixed culture of PPB. These bacteria were enriched from a wastewater influent taken from the pilot-scale WWTP located at the Rey Juan Carlos University (Mostoles, Madrid, Spain). Enrichment was performed by inoculating a 1 L suspended growth reactor (SGR) with sludge liquor, and subsequent incubation under near infra-red (NIR) light illumination and anaerobic conditions using a synthetic wastewater (SW) as growth medium. The SW (prepared with tap water) contained the 5 different organic carbon sources (acetic acid, malic acid, propionic acid, butyric acid and ethanol) with a total COD concentration of 2 gCOD/L, 0.26 gN/L as NH4Cl and 1 and 100 mL/L of micro- and macro-nutrient solutions, respectively. After the addition of SW the bioreactor liquor was flushed with argon gas in order to remove any presence of oxygen. The bioreactor was illuminated with LED lamps (850 nm) as IR light source. The reactor's surface was covered with UV-VIS absorbing foil (ND 1.2 299, Transformation Tubes, Banstead, UK). The foil absorbed around 90% of the wavelength below 750 nm. The average light intensity measured on the outside reactor's surface was 13 W/m2. The PPB mixed culture was continuously stirred and incubated at room temperature (25 ± 1°C). The liquor of the reactor was refreshed every week with fresh SW (99% volume exchange) to achieve final concentrations of 2 gCOD/L and 0.26 gNH4-N/L. The pH was weekly adjusted to 6.8 ± 0.1. The enrichment of PPB was evaluated by the detection of Bacteriochlorophylls (BChl) and carotenoids accumulation by performing VIS-NIR spectra analyses of the culture.
The ability of the PPB enriched culture to produce bio-hydrogen using different carbon and nitrogen sources was evaluated in batch assays. Initially, the capacity of the PPB culture to produce hydrogen using different organic and inorganic nitrogen sources was examined. The first set of experiments were conducted by using 2 gCOD/L of L-malic acid as the carbon source. Malic acid was chosen as a suitable carbon source that could favor hydrogen production by PPB (Assawamongkholsiri and Reungsang, 2015). Batch experiments were performed using: inorganic (NH4Cl) and organic (Na-glutamate) nitrogen, both with concentrations of 75, 150, and 300 mgN/L, and finally dinitrogen gas (60 mL of N2 in the headspace). Thereafter, two additional experiments were conducted using different carbon sources (butyric- and acetic- acid) at a concentration of 2 gCOD/L each, with 300 mgN/L of Na-glutamate as organic nitrogen source. A summary of the experimental conditions of the batch assays is shown in Table 1.
Table 1. Experimental runs of PPB biological experiments under different nitrogen and carbon sources.
All the experiments were conducted in 160 mL serum bottles with a working volume of 100 mL. The reactors contained 99 mL of SW medium (prepared as described above) with the corresponding COD and N contents and were inoculated with PPB enriched culture (1% v/v inoculum). The initial pH of the medium was adjusted to 6.8 ± 0.1 using NaOH or H2SO4. The liquid medium of each reactor was flushed with argon for 10 min. Thereafter, the bottles were closed with rubber stoppers and capped with aluminum seals. Subsequently, the headspace of the reactors was flushed again with argon for 2 min except from the reactors where nitrogen gas was used as nitrogen source that were flushed with N2 gas. The bottles were continuously shaken horizontally at 120 rpm at 25 ± 1°C (Orbital shaker, optic ivymen system) and illuminated at an average light intensity of 20 W/m2 using LED lamps for 7 days. The performance for H2 production using identical conditions but without PPB enriched culture was studied by conducting control experiments under sterilized conditions (all the glassware and media used were autoclaved). During these control experiments, no biomass growth as well as no H2 production or acid assimilation were detected. Both the liquid and the gas media were sampled periodically to evaluate, the carbon and nitrogen assimilation, the PPB growth and the hydrogen production. All the experiments were conducted in duplicate.
Bio-electrochemical experiments were performed in an H-cell device as shown in Figure 1. The device was consisted of two Duran glass bottles (8.6 cm diameter × 18.1 cm height) serving as two chambers. Each cell (cathode and anode) had a working volume of 500 mL. The cathode chamber was equipped with a working electrode of graphite of 10 × 100 mm and a reference electrode RE-5B Ag/AgCl. All potentials are quoted vs. Ag/AgCl. The anode chamber was equipped with a counter electrode of Ti/Pt (2.5 micro-m) 100 × 20 mm in a 10 × 5 mesh. The cathode and anode chambers were separated with a cationic membrane (RALEX, MEGA a.s., Straz pod Ralskem, Czechia). The working, counter and reference electrodes were connected to a potentiostat NEV4-V2 (Nanoelectra S.L., Alcala de Henares, Spain) with maximum current of ±100 mA and compliance voltage of ±10 V. A computer processed by specialized software (Potentiostat NEV4 software, Alcala de Henares, Spain) was used for the automatic recording of data.
As shown in Figure 1, the cathode chamber from the H-cell was employed as bio-cathode containing SW (495 ml). The bio-cathode was inoculated with PPB enriched culture (5 mL, 1% v/v inoculum). Malic acid (2 gCOD/L) and Na-glutamate at COD:N ratio of 100:15 were respectively used as carbon and nitrogen sources. The anode chamber was filled with 495 mL of tap water and 5 mL of the macro-nutrients solution. The initial pH in both chambers was adjusted to 6.8 ± 0.1. The bio-cathode was illuminated with LED lamps as NIR light source with an average light intensity of 20 W/m2. Bio-electrochemical experiments were performed at 25 ± 1°C and the cell of bio-cathode was continuously stirred at a speed of 200 rpm. The media in both cells were flushed with argon for 20 min. Subsequently, the cells were closed with butyl septa and capped with GL45 Duran caps. The headspaces of the cells were flushed again with argon for 3 min.
Experiments were conducted by setting the potential of bio-cathode at −0.5 V in order to force the PPB culture to be adapted to the electrochemical conditions. The reaction period among the PPB culture and the bio-cathode was chosen to be 1 week, similar to the biological experiments. Control electrochemical (abiotic) experiments were conducted using the same experimental conditions without PPB biomass. In order to determine whether the PPB culture interacted with the cathode or not by means of electron acceptance from PPB, cyclic voltametry (CV) in the range of −0.8 to 0.8 V was performed during the weekly reaction process.
All parameters except total chemical oxygen demand (COD) and total Kjeldahl Nitrogen (TKN) were determined after filtering with a 0.45 μm nylon filter (Chrodisc filter/syringe, CHMLab, Barcelona, Spain). Total and soluble COD were determined using a dichromate-reflux colorimetric method (APHA, 2005). The nitrogen contents of filtered and non-filtered samples were determined by the standard Kjeldahl procedure (Gerhardt TNK, Vapodest 450, Königswinter, Germany) using 20 mL of concentrated H2SO4 and K2SO4-CuSO4 as catalyst. Organic nitrogen content of PPB culture was determined as the difference between Kjeldahl nitrogen of filtered and non-filtered sample. The single cell protein (SCP) content of cell dry weight (CDW) was obtained by multiplying the obtained nitrogen value with a conversion factor of 5.33 (Salo-Vaananen and Koivistoinen, 1996). The inorganic nitrogen was analyzed as NH4Cl using Spectroquant Ammonium Test (Merck, Darmstadt, Germany). The optical density of PPB biomass was measured at 590 nm by UV-VIS spectrophotometer (V-630, Jasco, Madrid, Spain) and the concentration of biomass was calibrated using a standard curve of PPB optical density on the basis of volatile suspended solids (gVSS/L) concentration (Vasiliadou et al., 2008). The VSS concentration (gVSS/L) was measured according to standard methods (APHA, 2005). The detection of BChl and carotenoids of PPB was performed by determining the VIS-NIR spectra (400–950 nm) using a UV-vis spectrophotometer (V-630, Jasco, Madrid, Spain). The pH was measured using a pH meter (Crison GLP22, Hach Lange, Loveland, CO, USA). Illuminance was measured with a VIS-NIR spectroradiometer (STN-Bluewave-V, MTB, Madrid, Spain). The concentrations of VFAs (malic, acetic and butyric acids) in the liquid samples were analyzed using high performance liquid chromatography (HPLC) (Varian 356-LC, Agilent Technologies, Santa Clara, CA, USA), employing refractive index (RI) detector with a MetaCarb 67H 300 × 6.5 mm column (Agilent Technologies, Santa Clara, CA, USA). The oven temperature was 65°C. The mobile phase was 0.25 mM H2SO4 at a flow rate of 0.8 mL/min. The volume of the gas was determined by releasing pressure from the reactors headspace using a Boyle-Mariotte Apparatus (3B Scientific S.L., Hamburg, Germany). The composition of each reactors head-space was analyzed using a 7820A GC system equipped with a 3Ft 1/8 2 mm Poropak Q 80/100 SS column, a 6Ft 1/8 2 mm Poropak Q 80/100 SS column and a 6Ft 1/8 2 mm MolSieve 5A 60/80 SS column, a fitting external Luer lock and a thermal conductivity detector (TCD) (Agilent Technologies, Santa Clara, CA, USA). The mobile phase was Argon at a flow rate of 5 mL/min. The temperature of the oven and the detector were 45 and 220°C, respectively.
The following section include all results generated after exploring the physiology of PPB for selecting those culture conditions, including nitrogen and carbon sources, for achieving an optimal conversion of an extracellular source of electrons into hydrogen production and CO2 fixation.
The enrichment process was performed aiming to enhance the growth and acclimation of a mixed PPB culture from domestic wastewater, using a specific environment of NIR radiation. The organic mixture used for the enrichment was chosen on the basis that PPB can efficiently produce hydrogen from wastes that contain mixed VFAs (Wu et al., 2012). It should be noted that the optimum COD:N ratio for efficient C and N assimilation from domestic wastewater by PPB was reported to be 100:7.1 (Puyol et al., 2017b). However, during conventional DWW treatment operation, nutrients, such as N and P are usually in excess (Puyol et al., 2017b). Therefore, a COD:N ratio of 100:13.1 was chosen for the enrichment and acclimation process. Following a 2-weeks enrichment period, PPB biomass growth was evidenced through the BChl a accumulation as detected from the peaks with maximum absorbance at 590, 805, and 860 nm. This clearly indicated that the enrichment under anaerobic conditions and NIR light source could selectively enrich PPB from wastewater and express their photosynthetic apparatus via bacteriochlorophylls (Melnicki et al., 2008).
Figure 2 shows an example of PPB culture performance during a weekly operating cycle, after a 2-months acclimation period. Figure 2A shows the absorbance spectra of PPB culture aliquots that were taken at different time intervals during the weekly cycle (day 0–7). The PPB culture produced and accumulated with time BChl a as well as carotenoids that are naturally synthesized by photosynthetic organisms. The absorption spectrum of BChl a appeared in the spectral range between 560 and 930 nm, with maximum peaks at 590, 805, and 860 nm, respectively, while the spectrum of carotenoids appeared in the range between 400 and 550 nm. It has been previously reported that PPB produce molecules referred to as open-chain carotenoids and incorporate them into their photosynthetic system, such as light-harvesting complexes and the bacteriopheophytin-quinone type reaction center (Niedzwiedzki et al., 2017).
Figure 2. Performance of PPB culture during a weekly operating cycle: (A) BChl a and carotenoids absorption spectra due to PPB growth, (B) COD and NH4-N assimilation and PPB concentration as VSS.
As shown in Figure 2B, the PPB concentration reached 750 mg/L at the end of the weekly cycle, giving a growth yield of 0.75 ± 0.05 gCODPPB/gCODVFA. Moreover, the removal of COD and N by PPB culture over the whole acclimation period resulted to an average COD:N of 100:10, which was higher than the optimum ratio (100:7.1) previously reported for domestic wastewater. This high ratio suggested that the PPB enriched culture may have potential for enhancing nitrogen removal in order to achieve the discharge limits for total nitrogen (Hülsen et al., 2014).
Enriched PPB biomass was used for inoculum purposes in order to study the hydrogen formation from wastewater in presence and absence of an electrode as electron donor.
Hydrogen production under nitrogen fixation conditions is described by Equation (1) where molecular nitrogen (N2) is converted to ammonia (NH3) and protons (H+) to hydrogen (H2) (Rey et al., 2007).
Biological experiments were conducted in order to extract the optimum biological conditions to maximize hydrogen production while minimizing CO2 emission. Our first approach was to analysis how biohydrogen production depended on nitrogen substrate at different concentrations by using three different N sources (ammonium, glutamate and nitrogen gas) and malic acid as a model substrate of organic carbon. Interestingly, glutamate increased PPB growth rate by 2-fold in comparison with ammonium or nitrogen gas (see Figure S1 in Supplementary Information). This also is shown in Table 2, where the kinetic parameters of PPB metabolism are included.
Biohydrogen analysis revealed an interesting correlation of hydrogen with the ratio COD:N. So, hydrogen production was enhanced (451 ± 2.1 mLH2/L) when NH4Cl was used as inorganic nitrogen at a COD:N ratio of 100:3.75. In contrast, very low amount of hydrogen was produced when higher concentrations of NH4Cl (COD:N of 100:7.5 and 100:15) were tested (Figure 3A; Table 2), with 95% confidence values concurring with the zero value. This, in fact, indicates that zero hydrogen production cannot be statistically discarded under these conditions. This is in agreement with the results previously reported, stating that high NH4Cl concentration inhibits the function of the enzyme nitrogenase of PPB resulting in lower hydrogen production (Kim et al., 2012a). Alternatively, N2 gas as a nitrogen source was used to enhance hydrogen production under nitrogen fixation conditions without repressing the expression of nitrogenase genes.
Figure 3. Hydrogen (A) and CO2 (B) production by the PPB biological activity after 1 week of reaction with different nitrogen substrates and malic acid as organic carbon source. Error bars are standard deviations from triplicate experiments.
However, it was observed that the use of N2 gas as nitrogen source did not efficiently produce H2 (Figure 3A; Table 2). The low H2 production rate (0.12 ± 0.05 mLH2/Lh) observed in this experiment was probably attributed to a higher energy requirement for the process (16 ATP per mol of hydrogen produced in the nitrogen fixation case vs. 4 ATP per mol of hydrogen produced in the case of the hydrogen production with no nitrogen fixation in the nitrogenase; McKinlay and Harwood, 2010). Also, the extra consumption of reductants to conduct nitrogen fixation for the heterotrophic growth may be counteracted by an increase of the consumption of the produced H2 in autotrophic growth mode. The absence of CO2 evolution in the N2 experiments confirmed such a hypothesis. The PPB culture may use the H2 produced during the N2 fixation to re-fixate, in the Calvin-Benson-Bassham cycle (Calvin cycle, CBB), the CO2 produced during the malic acid assimilation. The analysis of the effect of an external electron source (e.g., from the cathode) would give light to this unsolved question and open possibilities for further research.
Finally, the results indicated that PPB culture produced large amounts of hydrogen when Na-glutamate was used as an organic nitrogen source (300–423 mLH2/L, Table 2). Na-glutamate enhanced the PPB growth and hydrogen production rate (2.1–2.7 mLH2/Lh). The results of this study are in agreement with those reported by other researchers, who have shown that Na-glutamate enhances hydrogen production without inhibiting the nitrogenase enzyme (Melnicki et al., 2008; Assawamongkholsiri and Reungsang, 2015). This is due to the fact that organic nitrogen can be directly assimilated into proteins and a less complex metabolic activity is required for the production of amino acids compared to inorganic sources (Merugu et al., 2010).
Considering Na-glutamate concentration, results showed that H2 production (mLH2/L) as well as its production rate was increased as the organic nitrogen concentration increased, achieving a maximum H2 production at a COD:N ratio of 100:15 (Figure 3A; Table 2). Moreover, it is noteworthy to mention that the CO2 production was reduced as the Na-glutamate concentration increased (Figure 3B). Therefore, the use of Na-glutamate at COD:N ratios of 100:3.75, 100:7.5, and 100:15 resulted to the production of 74.2 ± 11.6, 35.7 ± 30.7, and 11.8 ± 16.0 mLCO2/L, respectively. PPB that grow on oxidized organic substrates (as malic acid) produce CO2 due to the oxidation of these substrates. The released CO2 can then be fixed through the Calvin-Benson-Bassham cycle (Calvin cycle) into cell material as an electron accepting process (McKinlay and Harwood, 2010). This CO2 fixation via the Calvin cycle enabled PPB to accept excess of electrons and to maintain redox balance and to dispose extra electrons that are generated during use of extra carbon included in Na-glutamate. Therefore, the higher Na-glutamate concentration in the medium could result in a greater CO2 fixation and lower emission.
It should be highlighted that reducing the NH4Cl, levels (100:3.75 ratio) resulted in a high hydrogen production (Figure 3A) by minimizing the inhibitory effect of this compound on the activity of nitrogenase. In contrast, same conditions resulted in a significant emission of CO2 (56.7 ± 1.9 mLCO2/L). In addition, Na-glutamate in the ratio 100:15 could favor the PPB activity toward nitrogen assimilation. In conclusion, based on the above, Na-glutamate at a COD:N ratio of 100:15 was selected as the optimum culturing conditions for maximized H2 production with minimized CO2 emission.
Considering the importance of the internal electron recycling, an active modification of the electron fluxes through artificial addition of electrons by applying electrochemical technology may potentially enhance the PPB activity and drive toward an optimum H2 production process. In this sense, the bio-electrochemical capability of interaction between PPB and graphite-electrode has been explored, specially emphasizing the situation when graphite electrode behaves as an electron donor to PPB (setting graphite-electrode potential at −0.5 V) aiming to increase PPB metabolic paths activity by supplying electric current. Therefore, this study focused on the analysis of a bio-electrochemical device based on PPB, using malic acid as organic source and Na-glutamate as nitrogen source, compared to electrode-free biological systems.
Figure 4 presents the cyclic voltammograms (CV) of bio-electrochemical system as well as the bare graphite-electrode as a control electrochemical process, at different time intervals during a weekly operation. The electrochemical behavior for the bare graphite-electrode in the culture media do not exhibit any electrocatalytic behavior in the whole potential range of study (−0.8 to 0.8 V). As it can be seen in Figure 4, the cyclic voltammograms for bare graphite-electrode (electrochemical abiotic control) has no reductive currents that can be assigned to tentative hydrogen evolution or the malic acid reduction. As expected, only capacitive currents typical from bare graphite-electrode (ideally polarizable electrode, Bard and Faulkner, 2001) were detected in absence of PPB. Only small positive currents were observed at the more positive potentials explored, 0.8 V (vs. Ag/AgCl), probably due to slight water oxidation and graphite surface oxidation. Figure 4 shows the cyclic voltammograms for the bio-electrochemical system during the first 24 h, which are very similar to the bare graphite electrode. Only changes in the capacitive currents were observed, exhibiting a higher value for the interfacial pseudocapacitance under bio-electrochemical conditions, so suggesting an electrode surface modification by bacteria attachment. Just after inoculation no significant electroactive biofilm formed but the presence of bacteria in the interface increases the interfacial pseudocapacitance. This was probably due to the very low amount of PPB biomass existed at the beginning (Time 0 h) of the experiment (0.01 gVSS/L).
Figure 4. Cyclic voltammograms at different time intervals during bio-electrochemical (red line) and control electrochemical operation (blue line).
After 48 h of polarizing the electrode at −0.5 V (vs. Ag/AgCl), the cyclic voltammograms revealed the electroactivity of the PPB biofilm interacting with the graphite-electrode surface. These results suggested that PPB started to interact with the working electrode when sufficient amount of biomass (0.1 gVSS/L) and malic acid as carbon source were present in the cathode chamber (Figure 5A). It can be observed in Figure 4C, how the current was increased in correlation with a potential increase above 0.4 V. This result indicates that PPB biofilm used the electrode as an electron acceptor, probably for malic acid oxidation. This remarkably result indicates the use of PPB for anodic-based oxidations in MET applications. A less noticeable change in current in the negative potential region (between −0.2 and −0.8 V) is starting to develop after 48 h. In Figure 4D the negative currents in the potential region between −0.2 and −0.8 V results in a clear negative feature indicating processes related to the interaction of PPB with the graphite electrode as an electron donor. A detailed analysis of the cyclic voltammograms at 72 and 96 h suggests the presence of two processes responsible for the negative feature between −0.2 and −0.8 V. Two processes were may be identified: (a) between −0.2 and −0.4 V, there was a steady increase in the negative current (in absolute value), and (b) around −0.6 V there is a steep change in the slope of the negative current indicating the occurrence of a second process. In our experiments, the electrode was polarized at −0.5 V, a potential able to explore the first reductive process from electroactive PPB. Finally, it is noteworthy to mention that after the depletion of malic acid in the medium at 170 h (Figure 5A) the magnitude of redox reactions was changed (Figure 4F), showing an electrochemical behavior similar to this of Time 0 h, and suggesting low electroactivity of the PPB culture.
Figure 5. Culture of PPB under bio-electrochemical conditions at −0.5 V (vs. Ag/AgCl) (A) Malic acid removal and PPB growth and (B) production of H2, N2, and CO2.
The biological production of hydrogen by PPB was studied by testing different organic carbon sources, as malic acid, butyric acid and acetic acid, using Na-glutamate as optimum nitrogen source at a COD:N ratio of 100:15. Results indicated that the PPB culture was able to assimilate all the organic acids tested toward biomass growth as well as hydrogen production. Maximum PPB growth rate (7.56 mgVSS/Lh) was obtained when malic acid was used as compared to butyric (3.23 mgVSS/Lh) and acetic acid (3.96 mgVSS/Lh; Table 2). As a consequence of the higher C assimilation, the N assimilation into bacteria (as proteins) was also enhanced by using malic acid, giving a production of SCP of 874 mg/L as compared to 621 and 346 mg/L obtained with acetic and butyric acids, respectively. It was observed that the use of malic acid as carbon source achieved the highest hydrogen production (H2_max, mLH2/L) and the highest H2 production rate (H2_rate, mLH2/Lh; Table 2). Malic acid has widely used as optimum carbon source for H2 production, probably due to its capacity to directly enter the tricarboxylic acid cycle (Melnicki et al., 2008; Kim et al., 2012b; Assawamongkholsiri and Reungsang, 2015). Other evidences supporting malic acid as the optimum organic to conduct hydrogen production is shown in Supporting Information.
The experimental results obtained from the biological study (electrode-free) of hydrogen production indicated that the combination of malic acid and Na-glutamate was the optimum for maximizing the hydrogen production by PPB. The efficiency of H2 production from the PPB mixed culture enriched in this study is comparable to those of previous studies where pure or mixed PPB cultures were used (Table 3). In conclusion, the high H2 production rates achieved in this study showed that the PPB mixed culture could potentially be used for a feasible H2 production application during wastewater treatment processes. This supports the use of malic acid and Na-glutamate as the C and N sources for the bio-electrochemical production of H2.
Table 3. Comparison of hydrogen production rates by different cultures and systems with the one studied in the present work.
Results suggested that bio-electrochemical process of PPB resulted to similar H2 production rate and hydrogen yields (Table 2) compared to the PPB biological process under the same conditions. However, it was observed that after 1 week of bio-electrochemical reaction PPB fixed all the amount of CO2 that was produced during the first 50 h (Figure 5B). This resulted to zero CO2 emission as compare to the PPB biological process (see Supporting Information, Figure S2) that produced an average of 11.8 ± 16.0 mLCO2/L after 1 week of biological process (Figure 3B). Subsequently, results suggested that CO2 fixation was the main mechanism of PPB metabolism that was accepting electrons from the bio-cathode. This is in agreement with the negative current values observed during the bio-electrochemical process suggesting that there might be a consumption of electrons due to the PPB activity (Figure 6).
Figure 5 shows the malic acid assimilation, the PPB growth and the evolution of gas production during bio-electrochemical process. The bio-electrochemical setup revealed that PPB can effectively use the graphite-electrode as electron donor (Figures 4C-48 h, D-72 h, and E-96 h) and, subsequently, reduce the levels of CO2. Carbon dioxide fixation is not detected in such a high extension when system was run in absence of electrode (see Supporting Information, Figure S2). Carbon dioxide fixation seems to occur at the origin of the first reductive process detected between −0.2 and −0.6 V. The extra electron source provided by the electrode promoted carbon dioxide fixation by PPB beyond the standard activity of this bacterial genus in absence of electrodes under limited electrons availability.
It is well-reported that graphite electrodes, and generally carbon electrodes, exhibit a high overpotential for hydrogen evolution and carbon dioxide reduction (Sullivan et al., 1993; DuBois, 2006; Yang et al., 2016) and therefore poor electrocatalytic properties. The standard electrode potential for carbon dioxide reduction to formic acid and oxalic acid are −0.199 and −0.590 V (Sullivan et al., 1993; Eggins et al., 1998; DuBois, 2006; Yang et al., 2016), respectively. These values are reported in the SHE scale, and taking into account that we are working in pH = 7 solutions and Ag/AgCl reference scale (ca. 0.2 V vs. SHE), the standard electrode potential has to be recalculated according to Nernst equation. Nernst equation gives an equilibrium potential of −0.716 V for formate and −0.790 V in the case of oxalate vs. Ag/AgCl. In any case, these electrode potentials are more negative than −0.5 V vs. Ag/AgCl, potential value used in this study. Furthermore, these are the thermodynamic potential values, carbon dioxide reduction has been reported on graphite electrodes taking place at potentials more negative than −0.9 V vs. Ag/AgCl (Eggins et al., 1998). Actually, this fact can be clearly observed in the electrochemical control voltammograms reported in Figure 4. The cyclic voltammogram corresponding to the bare graphite electrode in the same solution but in absence of PPB, displays the classical voltammogram of an ideally polarized electrode, where there are no significant faradaic currents in the whole potential range explored (0.8 to −0.8 V), as it would be expected. Only pseudocapacitive electrochemical behavior is detected in the electrode interface in absence of PPB. Regarding the previous arguments, electrochemical carbon dioxide reduction on bare graphite electrode can be discarded, and the only contribution of PPB metabolism can explain the consumption of carbon dioxide.
In contrast, at −0.5 V, the capability of hydrogen production of the bio-electrochemical system was comparable to the exhibited by PPB in absence of electrode, and no electrode potential was observed in this process. The origin of the second bio-electrochemical process developed below −0.6 V, that can be tentatively assigned to hydrogen production, will require further investigation beyond the scope of this work. The capability of PPB for using graphite-electrode as electron donor was demonstrated. The extra electron donor source can be used in more than one metabolic pathway. Polarizing the electrode at −0.5 V, allows PPB to use electrode for carbon fixation reaching almost no carbon dioxide accumulation in contrast to the electrode-free biological system. This is the first study indicating that electroactive capture of CO2 by PPB is feasible.
Finally, the SCP production achieved by PPB during the bio-electrochemical process (81% mgSCP/mgVSS) was similar to this observed by PPB growing in absence of electrodes. Therefore, the bio-electrochemical process did not seem to affect proteins yields under the experimental conditions tested.
This work analyzed the optimum culturing conditions for maximizing the hydrogen production by a mixed culture of purple phototrophic bacteria. In addition, the effect of a negatively polarized bio-electrochemical device on the modification of the behavior of the culture in terms of metabolic shifts and current consumption was explored. The main conclusions extracted from this work are shown below:
- Among all the conditions tested in absence of electrodes, best results on the hydrogen production have been achieved by using malic acid as a carbon source (instead of acetic and butyric) and Na-glutamate as a N source (instead of ammonium and dinitrogen gas), in a COD/N relationship of 100/15. Under these conditions, the production of CO2 was also minimized.
- Cyclic voltammograms of the bio-electrochemical system shown the appearing of at least three potentials (two negative and one positive) with clear interaction between the PPB culture and the electrode. This makes evident the high electroactivity of PPB cultures and their potential as a MET microbial candidate.
- Negative polarization of the electrode at −0.5 V caused a detectable consumption of electrons associated with a depletion of the produced carbon dioxide, which indicates that the PPB culture was capable of using electrons from the cathode to capture the excess of C released as CO2 during the CBB cycle. This behavior was not observed before in an indigenous (non-genetically-modified) PPB culture.
- Results presented herein have shown that further in-depth research using different conditions (other polarization of the cathode) will be of extreme benefit and may enhance the H2 production rate.
IV designed and performed the experiments and wrote the manuscript, AB critically reviewed the manuscript, CM helped in the experimental stage, JM critically reviewed the manuscript, FM critically reviewed the manuscript and supervised the work, AE-N and DP designed the experiments, supervised the work, and corrected the manuscript. Both DP and AE-N are corresponding authors.
The authors declare that the research was conducted in the absence of any commercial or financial relationships that could be construed as a potential conflict of interest.
IV thanks the International Excellence Campus Smart Energy Program (CEISEP) for a Post-doctoral Fellowship. Financial support of Regional Government of Madrid provided through project REMTAVARES S2013/MAE-2716 and the European Social Fund as well as Spanish Ministry of Economy are acknowledged.
The Supplementary Material for this article can be found online at: https://www.frontiersin.org/articles/10.3389/fenrg.2018.00107/full#supplementary-material
Akkose, S., Gunduz, U., Yucel, M., and Eroglu, I. (2009). Effects of ammonium ion, acetate and aerobic conditions on hydrogen production and expression levels of nitrogenase genes in Rhodobacter sphaeroides O.U.00. Int. J. Hydrog. Energy 34, 8818–8827. doi: 10.1016/j.ijhydene.2009.08.040
APHA (2005). Standard Methods for the Examination of Water and Wastewater. Washington, DC: American Public Health Association.
Assawamongkholsiri, T., and Reungsang, A. (2015). Photo-fermentational hydrogen production of Rhodobacter sp. KKU-PS1 isolated from an UASB reactor. Elec. J. Biotechnol. 18, 221–230. doi: 10.1016/j.ejbt.2015.03.011
Bard, A. J., and Faulkner, L. R. (2001). Electrochemical Methods. Fundamentals and Aplications. Hoboken, NJ: John Wiley & Sons Inc.
Batstone, D. J., Hülsen, T., Mehta, C. M., and Keller, J. (2015). Platforms for energy and nutrient recovery from domestic wastewater: a review. Chemosphere 140, 2–11. doi: 10.1016/j.chemosphere.2014.10.021
Cheng, S., Xing, D., Call, D. F., and Logan, B. E. (2009). Direct biological conversion of electrical current into methane by electromethanogenesis. Environ. Sci. Technol. 43, 3953–3958. doi: 10.1021/es803531g
DuBois, D. L. (2006). “Electrochemical reactions of carbon dioxide,” in Encyclopaedia of Electrochemistry, eds A. J. Bard, and M. Stratmann (Weinheim: Wiley-VCH), 202–225.
Eggins, B. R., Brown, E. M., O'Neill, E. A., and Grinshaw, J. (1998). Carbon dioxide fixation by electrochemical reduction in water to oxalate and glyoxylate. Tetrahedron Lett. 29, 945–948.
Fulop, A., Beres, R., Tengolics, R., Rakhely, G., and Kovacs, K. L. (2012). Relationship between PHA and hydrogen metabolism in the purple sulfur phototrophic bacterium Thiocapsa roseopersicina BBS. Int. J. Hydrog. Energy 37, 4915–4924. doi: 10.1016/j.ijhydene.2011.12.019
Hu, C., Choy, S. Y., and Giannis, A. (2017). Evaluation of lighting systems, carbon sources, and bacteria cultures on photofermentative hydrogen production. Appl. Biochem. Biotechnol. 185, 257–269. doi: 10.1007/s12010-017-2655-5
Hülsen, T., Barry, E. M., Lu, K., Puyol, D., Keller, J., and Batstone, D. J. (2016). Domestic wastewater treatment with purple phototrophic bacteria using a novel continuous photo anaerobic membrane bioreactor. Water Res. 100, 486–495. doi: 10.1016/j.watres.2016.04.061
Hülsen, T., Batstone, D. J., and Keller, J. (2014). Phototrophic bacteria for nutrient recovery from domestic wastewater. Water Res. 50, 18–26. doi: 10.1016/j.watres.2013.10.051
Kim, M. S., Kim, D. H., and Cha, J. (2012b). Culture conditions affecting H2 production by phototrophic bacterium Rhodobacter sphaeroides KD131. Int. J. Hydrog. Energy 37, 14055–14061. doi: 10.1016/j.ijhydene.2012.06.085
Kim, M. S., Kim, D. H., Cha, J., and Lee, J. K. (2012a). Effect of carbon and nitrogen sources on photofermentative H2 production associated with nitrogenase, uptake hydrogenase activity, and PHB accumulation in Rhodobacter sphaeroides KD131. Bioresour. Technol. 116, 179–183. doi: 10.1016/j.biortech.2012.04.011
Klamt, S., Grammel, H., Straube, R., Ghosh, R., and Gilles, E. D. (2008). Modeling the electron transport chain of purple non-sulfur bacteria. Mol. Syst. Biol. 4:156. doi: 10.1038/msb4100191
Koku, H., Eroglu, I., Gunduz, U., Yucel, M., and Turker, L. (2002). Aspects of the metabolism of hydrogen production by Rhodobacter sphaeroides. Int. J. Hydrogen Energ. 27, 1315–1329. doi: 10.1016/S0360-3199(02)00127-1
Logan, B. E., and Rabaey, K. (2012). Conversion of wastes into bioelectricity and chemicals by using microbial electrochemical technologies. Science 337, 686–690. doi: 10.1126/science.1217412
Mas, J., and Van Gemerden, H. (1995). “Storage products in purple and green sulfur bacteria,” in Anoxygenic Photosynthetic Bacteria (Berlin: Springer), 973–990.
McKinlay, J. B., and Harwood, C. S. (2010). Carbon dioxide fixation as a central redox cofactor recycling mechanism in bacteria. Proc. Natl. Acad. Sci. U.S.A. 107, 11669–11675. doi: 10.1073/pnas.1006175107
Melnicki, M. R., Bianchi, L., De Philippis, R., and Melis, A. (2008). Hydrogen production during stationary phase in purple photosynthetic bacteria. Int. J. Hydrog. Energy 33, 6525–6534. doi: 10.1016/j.ijhydene.2008.08.041
Merugu, R., Girisham, S., and Reddy, S. M. (2010). Bioproduction of hydrogen by Rhodobacter capsulatus KU002 isolated from leather industry effluents. Int. J. Hydrog. Energy 35, 9591–9597. doi: 10.1016/j.ijhydene.2010.06.057
Merugu, R., Rudra, M. P., Badgu, N., Girisham, S., and Reddy, S. M. (2012). Factors influencing the production of hydrogen by the purple non-sulphur phototrophic bacterium Rhodopseudomonas acidophila KU001. Microb. Biotechnol. 5, 674–678. doi: 10.1111/j.1751-7915.2012.00346.x
Muñoz, R., and Guieysse, B. (2006). Algal-bacterial processes for the treatment of hazardous contaminants: a review. Water Res. 40, 2799–2815. doi: 10.1016/j.watres.2006.06.011
Niedzwiedzki, D. M., Dilbeck, P. L., Tang, Q., Martin, E. C., Bocian, D. F., Hunter, C. N., et al. (2017). New insights into the photochemistry of carotenoid spheroidenone in light-harvesting complex 2 from the purple bacterium Rhodobacter sphaeroides. Photosyn. Res. 131, 291–304. doi: 10.1007/s11120-016-0322-2
Ormerod, J. G., Ormerod, K. S., and Gest, H. (1961). Light-dependent utilization of organic compounds and photoproduction of molecular hydrogen by photosynthetic bacteria; relationships with nitrogen metabolism. Arch. Biochem. Biophys. 94, 449–463. doi: 10.1016/0003-9861(61)90073-X
Ozmihci, S., and Kargi, F. (2010). Bio-hydrogen production by photo-fermentation of dark fermentation effluent with intermittent feeding and effluent removal. Int. J. Hydrog. Energy 35, 6674–6680. doi: 10.1016/j.ijhydene.2010.04.090
Park, T. J., Ding, W., Cheng, S., Brar, M. S., Ma, A. P. Y., Tun, H. M., et al. (2014). Microbial community in microbial fuel cell (MFC) medium and effluent enriched with purple photosynthetic bacterium (Rhodopseudomonas sp.). AMB Express 4:22. doi: 10.1186/s13568-014-0022-2
Pous, N., Puig, S., Coma, M., Balaguer, M. D., and Colprim, J. (2013). Bioremediation of nitrate-polluted groundwater in a microbial fuel cell. J. Chem. Technol. Biotechnol. 88, 1690–1696. doi: 10.1002/jctb.4020
Puyol, D., Barry, E. M., Hülsen, T., and Batstone, D. J. (2017b). A mechanistic model for anaerobic phototrophs in domestic wastewater applications: photo-anaerobic model (PAnM). Water Res. 116, 241–253. doi: 10.1016/j.watres.2017.03.022
Puyol, D., Batstone, D. J., Hülsen, T., Astals, S., Peces, M., and Krömer, J. O. (2017a). Resource recovery from wastewater by biological technologies: opportunities, challenges, and prospects. Front. Microbiol. 7:2106. doi: 10.3389/fmicb.2016.02106
Rey, F. E., Heiniger, E. K., and Harwood, C. S. (2007). Redirection of metabolism for biological hydrogen production. Appl. Environ. Microbiol. 73, 1665–1671. doi: 10.1128/AEM.02565-06
Ryu, M. H., Hull, N. C., and Gomelsky, M. (2014). Metabolic engineering of Rhodobacter sphaeroides for improved hydrogen production. Int. J. Hydrog. Energy 39, 6384–6390. doi: 10.1016/j.ijhydene.2014.02.021
Salo-Vaananen, P. P., and Koivistoinen, P. E. (1996). Determination of protein in foods: comparison of net protein and crude protein (N X 6.25) values. Food Chem. 51, 21–31.
Sullivan, B. P., Krist, K., and Guard, H. E. (Eds.) (1993). Electrochemical and Electrocatalytic Reactions of Carbon Dioxide. Amsterdam: Elsevier.
Tawfik, A., El-Bery, H., Kumari, S., and Bux, F. (2014). Use of mixed culture bacteria for photofermentive hydrogen of dark fermentation effluent. Bioresour. Technol. 168, 119–126. doi: 10.1016/j.biortech.2014.03.065
Tejedor, S., Baccetti de Gregoris, T., Salas, J. J., Pastor, L., and Esteve-Nu-ez, A. (2016). Integrating a microbial electrochemical system into a classical wastewater treatment configuration for removing nitrogen from low COD effluents. Environ. Sci. Water Res. Technol. 2, 884–896. doi: 10.1039/C6EW00100A
Varfolomeyev, S. D. (1992). Bioelectrosynthesis as an alternative to photosynthesis. Appl. Biochem. Biotechnol. A Enz. Engin. Biotechnol. 33, 145–155. doi: 10.1007/BF02950783
Vasiliadou, I. A., Tziotzios, G., and Vayenas, D. V. (2008). A kinetic study of combined aerobic biological phenol and nitrate removal in batch suspended growth cultures. Int. Biodeterior. Biodegrad. 61, 261–271. doi: 10.1016/j.ibiod.2007.09.002
Verstraete, W., Van de Caveye, P., and Diamantis, V. (2009). Maximum use of resources present in domestic “used water”. Bioresour. Technol. 100, 5537–5545. doi: 10.1016/j.biortech.2009.05.047
Wu, T. Y., Hay, J. X. W., Kong, L. B., Juan, J. C., and Jahim, J. M. D. (2012). Recent advances in reuse of waste material as substrate to produce biohydrogen by purple non-sulfur (PNS) bacteria. Renew Sustain Energy Rev. 16, 3117–3122. doi: 10.1016/j.rser.2012.02.002
Xing, D., Zuo, Y., Cheng, S., Regan, J. M., and Logan, B. E. (2008). Electricity generation by Rhodopseudomonas palustris DX-1. Environ. Sci. Technol. 42, 4146–4151. doi: 10.1021/es800312v
Keywords: purple phototrophic bacteria, biolectrochemical, high value-added products, bio-hydrogen, carbon fixation, proteins
Citation: Vasiliadou IA, Berná A, Manchon C, Melero JA, Martinez F, Esteve-Nuñez A and Puyol D (2018) Biological and Bioelectrochemical Systems for Hydrogen Production and Carbon Fixation Using Purple Phototrophic Bacteria. Front. Energy Res. 6:107. doi: 10.3389/fenrg.2018.00107
Received: 15 May 2018; Accepted: 25 September 2018;
Published: 13 November 2018.
Edited by:
Sebastià Puig, University of Girona, SpainReviewed by:
Ioannis Andrea Ieropoulos, University of the West of England, United KingdomCopyright © 2018 Vasiliadou, Berná, Manchon, Melero, Martinez, Esteve-Nuñez and Puyol. This is an open-access article distributed under the terms of the Creative Commons Attribution License (CC BY). The use, distribution or reproduction in other forums is permitted, provided the original author(s) and the copyright owner(s) are credited and that the original publication in this journal is cited, in accordance with accepted academic practice. No use, distribution or reproduction is permitted which does not comply with these terms.
*Correspondence: Daniel Puyol, ZGFuaWVsLnB1eW9sQHVyamMuZXM=
Abraham Esteve-Nuñez, YWJyYWhhbS5lc3RldmVAdWFoLmVz
Disclaimer: All claims expressed in this article are solely those of the authors and do not necessarily represent those of their affiliated organizations, or those of the publisher, the editors and the reviewers. Any product that may be evaluated in this article or claim that may be made by its manufacturer is not guaranteed or endorsed by the publisher.
Research integrity at Frontiers
Learn more about the work of our research integrity team to safeguard the quality of each article we publish.