- 1Center for Renewable Carbon, The University of Tennessee, Knoxville, TN, United States
- 2U.S. Forest Service (USDA), Auburn, AL, United States
Non-structural components, such as inorganics and organic extractives, present in switchgrass were extracted with water and ethanol, and the resulting non-structural components-free materials were pyrolyzed to investigate the effect of the inorganic species on the pyrolytic products. The extraction was performed for switchgrass materials harvested from three consecutive growing seasons, removing 8.5 wt% of the organic extractives in the first season biomass, and 5.8 and 6.3 wt% in the second and third season, respectively, on total dry basis of biomass. In addition to organic extractives, from 0.7 to 2.7 wt% of ash were extracted. Specifically, 99% and 59% of total K and Mg were removed from the switchgrass harvested in the second and third growing season. Thermogravimetric analysis demonstrated that a predominant reduction of K and Mg content in the biomass increased temperature at which mass loss rate is maximized in the decomposition of cellulose, hemicellulose, and lignin. The reduction of K and Mg content also affected pyrolytic products generated at 450°C. The chromatographic peak area percentage of levoglucosan from the extracted samples in the second and third growing season was two to three times higher than that from the extracted samples in the first growing season, showing a strong negative correlation with K and Mg content, whereas most of the light oxygenated and furanic/pyranic/cyclopentanic products exhibited a strong positive correlation with K and Mg content. We concluded that the removal of non-structural components prior to thermochemical conversion processes such pyrolysis can potentially produce a valuable extractives stream while removing catalytic inorganics that negatively impact downstream pyrolysis process.
Introduction
A wide variety of lignocellulosic biomass is under consideration as renewable resources, including woody and herbaceous biomass, to produce chemicals, biomaterials, and biofuels using various thermochemical and biochemical conversion technologies. Of these technologies, pyrolysis is a thermochemical conversion that liquefies biomass in the absence of oxygen at mild operating temperatures (450–600°C) (Kim et al., 2014). Conventionally, pyrolysis is used to directly convert biomass to a high yield bio-oil (60–75%) as well as a biochar fraction (15–25%) and non-condensable gases (10–20%) similar to syngas (Bridgwater, 2012). Bio-oil can be upgraded to biofuels and chemicals through hydrotreating or catalytic processes while non-condensable gases can be burned for combined heat and power (CHP). In addition, biochar can be used as soil amendment or alternatively be combusted for CHP. However, during thermochemical conversion, ash naturally present in the biomass can damage processing systems by slagging and fouling of reactors and pipes as well as influence the composition and quality of the pyrolytic products (Froment et al., 2013). The most abundant alkali and alkaline earth metallic species, such as potassium, calcium, sodium, and magnesium in ash, act as a catalyst for cellulose and hemicellulose depolymerization and fragmentation, and generate a high yield of light oxygenated compounds such as acids, ketones, aldehydes, and furans (Lian et al., 2012). The large amount of oxygenated compounds present in bio-oils has been found to deactivate catalysts by coke deposition during refinery upgrading processes (Bridgwater, 2012) and to restrain ethanol production by inhibiting the growth of fermentative microorganisms (Lian et al., 2012). Therefore, many efforts have been devoted to reduce inorganics present in biomass via water, mild, or strong acid pretreatment prior to fast pyrolysis and to promote the yield and quality of bio-oil in the aspect of heating value, viscosity, and water content. Bio-oil also contains high amount of levoglucosan due to the decrease of glycolaldehyde yield after water or acid pretreatment (Mourant et al., 2011; Wang et al., 2012). Levoglucosan is a useful starting chemical that can be converted into platform chemicals such as levulinic acid, levulinic esters, and 5-(hydroxymethyl) furfural (Yin et al., 2016). Levoglucosan can also serve as a precursor for bioethanol production through hydrolysis followed by fermentation (Luque et al., 2016). However, high severity pretreatment conditions, including high temperature, long reaction time, and high acid concentration, lead to the decomposition of the biomass matrix through the hydrolysis of cellulose and hemicellulose (Eom et al., 2011).
Switchgrass (Panicum virgatum), a perennial grass native to North America, is an attractive candidate for bioenergy production (Kline et al., 2013). Compared to woody biomass, switchgrass is a fast-growing crop that can reach up to three meters in height in one growing season (Parrish and Fike, 2005). Switchgrass can be harvested either annually or semi-annually for ten years before it needs to be replanted, leading to one of the most dependable feedstocks with low production costs and high renewability (Parrish and Fike, 2005). The quality of biomass reflected by its chemical composition is a key factor that affects efficiency of bioenergy production. The chemical constituents of switchgrass, are generally structural polymers such as cellulose (30.0–43.9 wt%), hemicellulose (24.4–30.5 wt%), and lignin (18.6–25.4 wt%), and non-structural components such as extractives (4.7–15.6 wt%) and inorganic ash-forming elements (1.8–7 wt%) (Lemus et al., 2002; Kline et al., 2015). Interestingly, the content of extractives in switchgrass is similar or higher than that of woody biomass and is mostly composed of lipophilic and hydrophilic types such as waxes, oils, fats, resins, free sugars, chlorophyll, organic acids, alditols, and polyphenolics, making them a potential source of value-added co-products (Sannigrahi et al., 2010). These extractives are generally extracted with water and organic solvents such as ethanol, acetone, hexane, dichloromethane, or diethyl ether (Thammasouk et al., 1997; Uppugundla et al., 2009). For example, a standard non-structural components extraction procedure using water and ethanol according to National Renewable Energy Laboratory (NREL) analytical procedure (NREL, 2008) extracts water soluble compounds such as non-structural sugars, inorganics, and ethanol soluble compounds such as chlorophyll, phytosterols, terpenes, and phenolic compounds, which have been reported to have a potential antimicrobial and antioxidant activity (Proestos et al., 2006; Uppugundla et al., 2009; Labbé et al., 2016).
Most of the aforementioned studies, however, have investigated the effect of removal of inorganics present in biomass via water or acid treatment on pyrolytic products, but have not taken the loss of non-structural components such as extractives into consideration although this biomass fraction, extractives, could be a potential high-value co-product if an integrated biorefinery is considered (Labbé et al., 2016). Therefore, the objective of this study was to evaluate the effect of non-structural inorganic species on pyrolytic products after the biomass, switchgrass, was subjected to an extraction step that was specifically designed to extract the organic extractives fraction of switchgrass. Switchgrass materials with a wide extractives and ash content range were initially treated with both water and ethanol, then analyzed by thermogravimetric analysis (TGA) and pyrolysis-gas chromatography/mass spectrometry (Py-GC/MS). The inorganic composition of switchgrass before and after extraction was determined to evaluate the catalytic effects of existing inorganic species on pyrolytic products using statistical methods such as principal component and Pearson's correlation analyses.
Materials and Methods
Material
Alamo switchgrass samples were collected from a University of Tennessee-Knoxville experiment field established in 2011. Eight switchgrass samples were harvested for three consecutive growing seasons in December of 2011 (year 1), 2012 (year 2), and 2013 (year 3). A total of 24 switchgrass samples were collected, dried, then ground to less than 0.42 μm particle size using a Wiley mill (Thomas Scientific). The samples are referred as S1 to S8 for year 1, S9 to 16 for year 2, and S17 to S24 for year 3.
Sample Extraction and Characterization
The ground samples were extracted using an accelerated solvent extractor (ASE 350, Dionex) to remove non-structural components, i.e., extractives and inorganics, according to the National Renewable Energy Laboratory (NREL) protocol (NREL, 2008). Approximately 5 g of the ground material were loaded with glass beads (1:5 wt/wt) in a 34 mL extraction cell. The sample-loaded cell was heated up to 100°C, then extracted with water by 3 cycles for 10 min/cycle, and followed by ethanol with 3 cycles for 10 min/cycle at 1600 psi of pressure. After extraction, the extractives-free samples were dried at 40°C for 7 days before further analyses.
Ash content of the unextracted and the extracted samples was measured according to ASTM D 1762-84. The inorganic composition was measured by inductively coupled plasma-optical emission spectroscopy (ICP-OES, Optima 7300 DV spectrometer, PerkinElmer) after acid microwave digestion of the material (Kim et al., 2011).
The thermal behavior of the sample was investigated by thermogravimetric analysis (TGA) and pyrolysis-gas chromatography/mass spectrometry (Py-GC/MS). A thermogravimetric analyzer (TGA Q50 TA Instrument) was employed to monitor weight loss as a function of temperature and to evaluate thermal decomposition of the unextracted and extracted switchgrass over time. Approximatively 6–9 mg of the sample were heated from 30 to 110°C at a rate of 20°C/min and held for 10 min to remove moisture from the sample under nitrogen gas flow (20 mL/min). Then, the sample was heated to 550°C at 20°C/min and held for 15 min. The resulting TG and differential TG (DTG) curves described the thermal characteristics of each sample.
The unextracted and extracted switchgrass samples were also analyzed using a micro-pyrolyzer (Frontier EGA/Py-3030 D) fitted with a Perkin Elmer Clarus 680 Gas Chromatograph coupled with a Clarus SQ 8C Mass Spectrometer. Approximatively, 0.2 mg of sample in a stainless-steel pan was injected into the pyrolysis furnace which was heated to 450°C for 12 s of residence time. The vapor produced in the furnace was swept into a GC-injection port (temperature 270°C) by the GC carrier gas (helium, 1 mL/min) that passes through the furnace with a split ratio of 80:1. The vapor was then separated using a DB-1701 Agilent capillary column (60 m × 0.25 mm ID × 0.25 μm film thickness). The GC oven temperature program was as follows: 4 min at 50°C, ramp 5°C per minute to 280°C, and hold at 280°C for 4 min. The generated compounds were analyzed using a mass spectrometer with a source temperature of 270°C and a 70 eV electron ionization. Chromatographic peaks with S/N > 2000, including identifiable and non-identifiable peaks, were extracted using the TurboMass GC/MS software. These peaks were normalized to the peak area percentage based on the sum of the total peak areas. A total of 91 organic compounds were selected for statistical analysis.
Statistical Analysis
The relationships between the inorganics present in switchgrass and the resulting pyrolytic organic products were investigated using multivariate statistical analysis as well as Pearson's correlation analysis. Normalized peak areas % of the 91 organic compounds identified in each pyrogram were analyzed using principal component analysis (PCA, Unscrambler software ver. 9.0 CAMO) to determine similarities and differences in the pyrolytic organic compounds produced from the samples containing various levels of inorganics. PCA is a statistical procedure that transforms a large set of variables into a smaller set in a new system of axes of principal components (PCs). The PCA results show the trend of similarity and difference of the samples and the variables in the plot (Saporta and Niang, 2009). The scores plot visualizes the samples and helps to interpret the relationships among the samples while the loadings plot presents the contribution of each variable (chromatographic peaks) to each new PC and describes the relationship among the variables. The Pearson correlation coefficient (r) was calculated using the SigmaPlot 12 software and was considered significant when the p < 0.05.
Results and Discussion
Non-structural Components of Switchgrass
A total of twenty four switchgrass samples, eight samples collected for three consecutive years, were extracted with water and ethanol (Figure 1). Non-structural components, including organic and inorganic constituents, were extracted from the biomass. The averaged extraction was 9.6 wt% for the first growing season biomass, 6.8, and 7.5 wt% for the second and third growing season biomass, respectively, on a dry basis of total biomass. Organic extractives constituted a large portion of the extracts, with an average of 8.5 wt% in the first year, then 5.8 and 6.3 wt% in the second and third year, respectively. Many researchers have been highly interested in biomass extractives, including flavonoids such as rutin and quercetrin and phenolic compounds such as p-coumaric, sinapic, and ferulic acids, that are potential sources of valuable co-products and can be used in the pharmaceutical and cosmetic industries providing an additional income stream for biorefineries producing biofuel in a more integrated manner (Uppugundla et al., 2009; Michels and Wagemann, 2010). In addition to these valuable organic components, inorganics naturally present in switchgrass were also extracted, which corresponds to a mass loss ranging from 0.7 to 2.7 wt% (Figure 1). The content and composition of these fractions are known to vary with the age, genetics, tissue types, and harvesting method and time of the biomass (Kline et al., 2013). The switchgrass samples harvested for three consecutive years had very similar chemical composition including cellulose of 39.1–39.3%, hemicellulose of 29.4–31.2%, and lignin of 21.4–21.7%, respectively. The reduction of non-structural components in switchgrass samples over the course of three years can be explained by the fact that switchgrass reached a more steady-state growth pattern in the second and third year (Baxter et al., 2016).
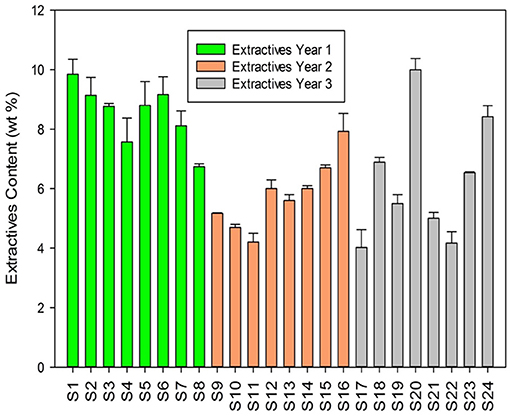
Figure 1. Yield of non-structural components extracted from switchgrass samples harvested for 3 consecutive years.
Inorganic Compounds in Switchgrass
As expected, the unextracted (untreated) switchgrass samples had a higher ash content with an average of 7.0 wt% in the first year and a 3.5 and 3.2 wt% in the second and third year, respectively (Figure 2A and Supporting Information Table A1). The extracted switchgrass (treated) samples had an average ash content of 5.9 wt% with a decrease of 16% compared to the unextracted samples in the first year, and 2.4 and 2.1 wt% with a removal of 33% in the second and third year, respectively. These data demonstrated that the solvent extraction could remove a fraction of the inorganic compounds, between 16 and 33% of the total ash present in switchgrass. Alkali and alkaline earth metals (AAEM), such as calcium, magnesium, and potassium, were the most abundant constituents found in the untreated switchgrass samples (Figure 2B and Supporting Information Table A1). For example, the first growing season switchgrass contained an average of 7,103 mg of K per kg of biomass, 5,261 mg/kg of Ca, and 2,559 mg/kg of Mg whereas the biomass in the second and third year had lower of K (an average of 4,923 and 3,931 mg/kg), Ca (2,018 and 1,522 mg/kg), and Mg (1,590 and 1,411 mg/kg), respectively. After the solvent extraction, the concentration of K in the extracted switchgrass samples was predominantly reduced to an average of 370 mg/kg, a 95% removal compared the untreated samples in the first year, and to 40 and 28 mg/kg with 99% removal for the second and third year biomass, respectively. The concentration of Mg decreased by more than 50% after the extraction; with 55% removal in the first year and 58% removal for the second and third year biomass. However, Ca was slightly extracted from the switchgrass with only a 12 and 9% removal for the second and third year, respectively.
The concentration of other minor inorganics, such as Al, Fe, and Mn present in switchgrass was slightly reduced with removal ranging between less than 1 and 28%. The extraction of silica (Si) was also low with the highest removal at 8%. These findings indicated that salts present in biomass could be extracted with water and ethanol extraction, whereas inorganics structurally bound to the carboxylic and/or phenolic groups in the organic matrix still remain in biomass (Nik-Azar et al., 1997).
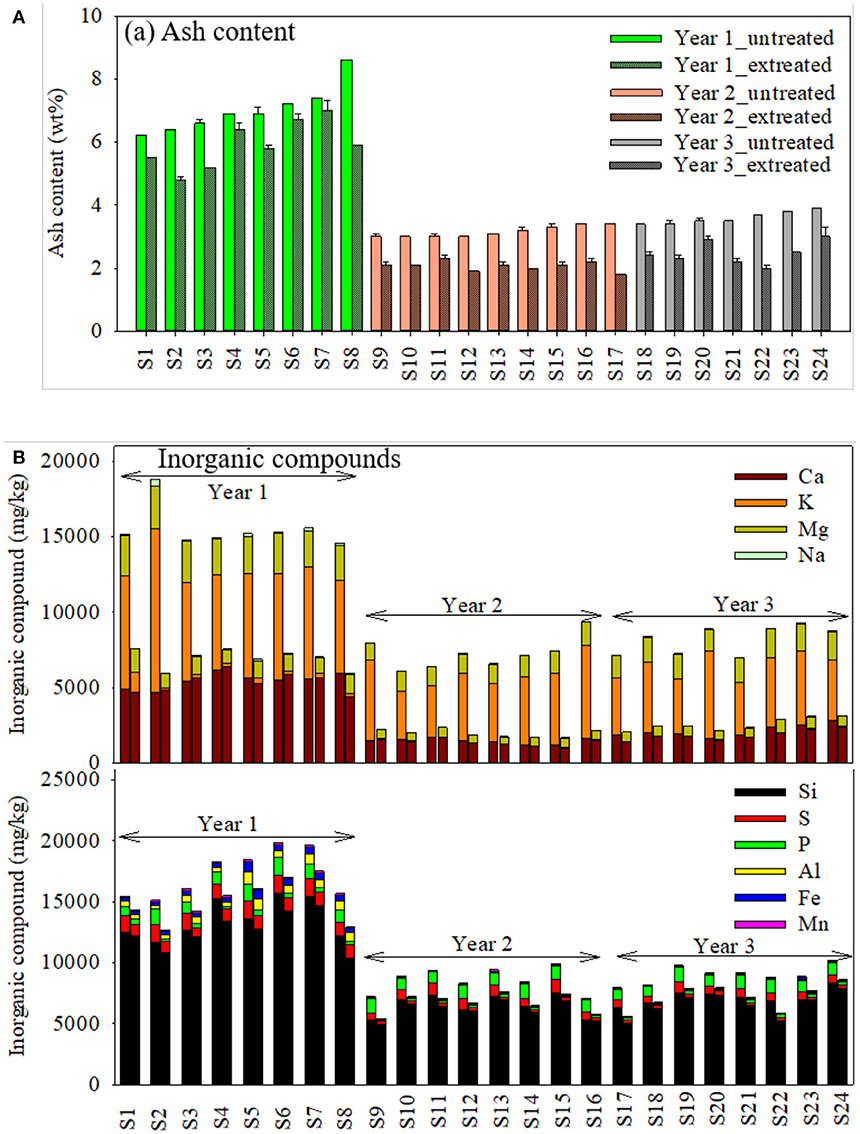
Figure 2. Ash content and inorganic compounds in switchgrass (untreated and the corresponding treated) samples harvested for three consecutive years. (A) Ash content and (B) inorganic species in the untreated (first stack) and treated switchgrass (second stack).
Thermogravimetric Analysis
The thermal degradation profile of switchgrass and the corresponding extractives-free biomass were obtained by TGA from 30 to 550°C in order to investigate the biomass decomposition temperature in function of ash content (Figure 3). The TG thermograms exhibited a shift of initial degradation temperature, at which drastic mass loss started, toward higher TG temperatures as the unextracted samples were extracted with water and ethanol (Figure 3A). Derivative TG (DTG) curves were calculated from the TG thermograms and presented the thermal decomposition peaks corresponding to temperatures at which the mass loss rate was maximum (Figure 3B) according to the devolatilization temperatures determined as in Grønli et al. (2002). The initial degradation temperature (Tonset) at which hemicellulose decomposition starts (Figure 4A) demonstrated that as ash content in the unextracted switchgrass samples decreased from 7.0 wt% in the first year to 3.1–3.6 wt% in the second and third year, Tonset shifted to higher temperature, from 223 to 251°C showing a strong negative correlation (r = −0.85) between the two parameters (Table 1). The decomposition temperature (Tshoulder) of hemicellulose (Figure 4B), at which hemicellulose decomposition is maximum, moved to higher temperature (from 284 to 312°C), with a mild negative correlation of −0.56 with ash content. The decomposition temperature (Tpeak) (Figure 4B), where the maximum cellulose decomposition rate occurs, shifted from 323 to 346°C also showing a weaker negative correlation (r = −0.42) with ash content. The beginning temperature, Toffset, of the final tailing region, dominated by the lignin decomposition, slightly moved from 364 to 379°C with no correlation with ash content (Figure 4A).
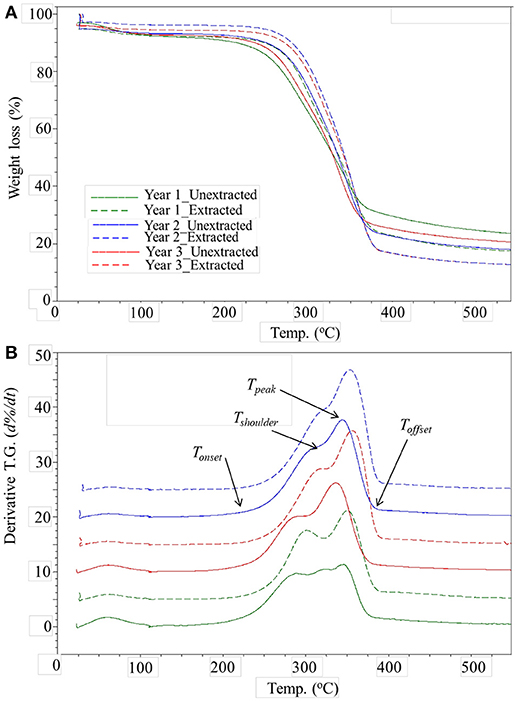
Figure 3. Representative thermograms (A) and relative derivative TG curves (B) of the unextracted and extractives-free switchgrass samples of 3 consecutive harvesting years, with points noted for the onset, shoulder, peak, and offset temperatures, as defined by Grønli et al. (2002).
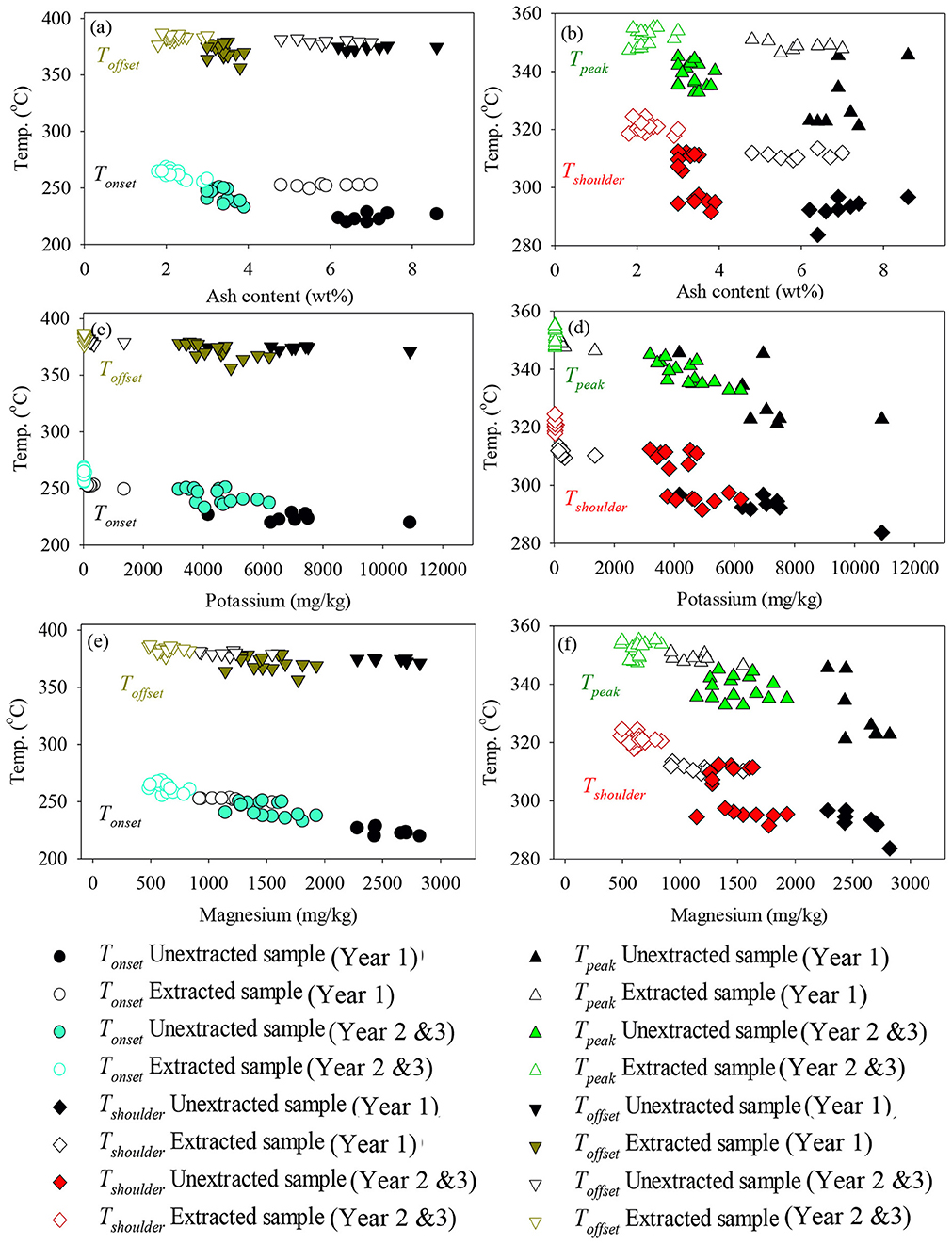
Figure 4. Degradation temperature at which switchgrass decomposition is maximum in the DTG curve associated with ash content, potassium, and magnesium. (A) Toffset and Tonset with ash content, (B) Tpeak and Tshoulder with ash content, (C) Toffset and Tonset with potassium, (D) Tpeak and Tshoulder with potassium, (E) Toffset and Tonset with magnesium, and (F) Tpeak and Tshoulder with magnesium.
The DTG curves of the extracted switchgrass samples exhibited a shift of hemicellulose (Tonset and Tshoulder), cellulose (Tpeak), and lignin (Toffset) decomposition peaks toward higher temperatures due to the reduction in ash content, demonstrating strong negative correlations between ash and these decomposition temperatures (Table 1). Interestingly, the extracted samples with high ash content of 5.9 wt% in the first year biomass exhibited a shift of the DTG peaks to temperatures higher than the unextracted switchgrass samples with low ash content ranging from 3.1 and 3.6 wt% in the second and third year (Figures 4A,B). These results confirm that some specific inorganic species present in the unextracted switchgrass significantly affected the thermal decomposition of switchgrass structural components. Of the inorganic species affected by the solvent treatment, the major alkali and alkaline earth metals (AAEM), such as potassium and magnesium, were remarkably extracted with their content decreasing by 95–99% and 43–62 %, respectively. The drastic reduction of potassium and magnesium could be responsible for the shift of hemicellulose (Tonset and Tshoulder), cellulose (Tpeak), and lignin (Toffset) decomposition peaks toward higher temperatures (Figures 4C–F, Table 1). Therefore, it could be concluded that a reduction of potassium and magnesium content during an extractives extraction process significantly impacts the thermal decomposition of biomass confirming that alkali and alkaline earth metals act as catalyst that affect the rate of thermal degradation of biomass components in thermochemical processes (Kleen and Gellerstedt, 1995).
Py-GC/MS Analysis
Switchgrass samples with varying ash content and their corresponding extracted samples were pyrolyzed using a Py-GC/MS at 450°C. A total of 91 organic compounds were identified to primarily estimate the changes of the yield of these volatile organic products (Table 2). Chromatographic peak area percentage of the identified organic compounds for the unextracted and extractives-free samples were subjected to a multivariate statistical technique, principal component analysis (PCA) (Figure 5). The PCA scores plot featured the distribution of the samples along PC1 and PC2 which accounted for 90 and 6% of the total variance of the chromatographic data set, respectively. The PCA scores plot (Figure 5A) demonstrated that the extractives-free switchgrass samples with low ash, including potassium and magnesium, clustered together in the negative quadrant of PC1 whereas the unextracted samples with high ash, potassium, and magnesium content, grouped in the positive section of PC1 (Figure 5A). The extracted samples from the first year biomass closely clustered to the untreated samples from the first year. This result can be explained by the presence of high ash content (5.9 wt%) and potassium (370 mg/kg), magnesium (1147 mg/kg), calcium (5187 mg/kg) in the extracted samples in the first year, which still participates in catalytic pyrolysis similar to that of the untreated samples in the first year. Meanwhile, the untreated and extracted samples in the second and third year were far apart from each other due to a significant reduction of inorganic compounds with low ash content (2.1–2.4 wt%), potassium (33–35 mg/kg), and magnesium (577–686 mg/kg) in the extracted samples.
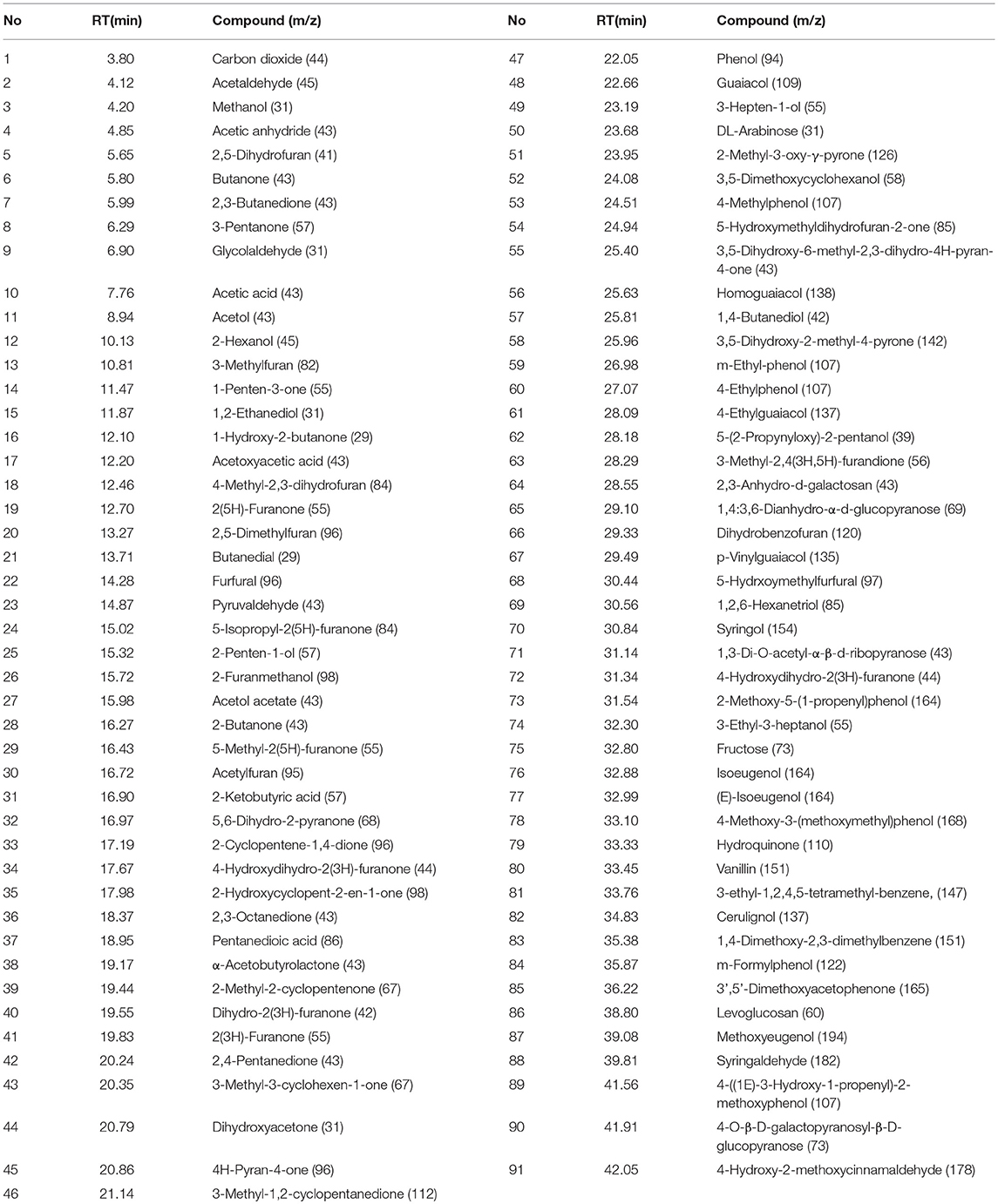
Table 2. Organic compounds identified by Py-GC/MS from the unextracted and the corresponding extractives-free switchgrass samples.
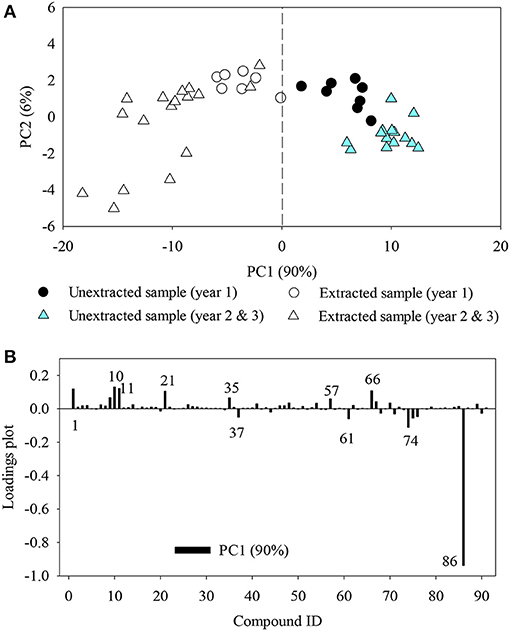
Figure 5. PCA of the Py-GC/MS chromatographic peak area % of the unextracted and corresponding extractives-free switchgrass samples. (A) Scores and (B) loadings plots of the unextracted and extracted switchgrass samples.
The loadings plot of PC1 identified the chromatographic peaks that contributed the most to the separation along PC1 (Figure 5B) and revealed that during pyrolysis the extractives-free samples with lower potassium and magnesium generated higher amount of mainly pentanedioic acid (37), 4-ethylguaiacol (61), 3-ethyl-3-heptanol (74), and levoglucosan (86). Whereas, the unextracted samples with higher ash content produced higher amount of low molecular weight species such as carbon dioxide (1), acetic acid (10), acetol (11), butanedial (21), 1,4-butanediol (57), furans/pyrans/cyclopentenones such as 2-hydroxycyclopent-2-en-1-one (35), and dihydrobenzofuran (66) (Figure 5B) confirming again the catalytic role of the inorganics during switchgrass pyrolysis (Shen and Gu, 2009).
Chromatographic peaks of all identified pyrolytic compounds, accounting for higher than 90% of total peak area in all chromatograms, were integrated, expressed as a percentage of the total pyrogram area, and classified into light oxygenates, furans/pyrans/cyclopentenones, anhydrosugars, phenolics, and carbon dioxides (Figure 6). The first thermal decomposition step of carbohydrates (cellulose/hemicellulose fraction) during switchgrass pyrolysis is the generation of anhydrosugars such as mainly levoglucosan and 1,4:3,6-dianhydro-α-d-glucopyranose, which are produced by competitive reactions through dehydration and cleavage of glycosidic bond during pyrolysis (Patwardhan et al., 2009). The anhydrosugars yield of the extracted switchgrass samples was higher than that of unextracted samples (Figure 6). In particular, the amount of anhydrosugars from the extracted samples in the second and third year became two to three times more than that of the untreated samples in the same years. This finding can be explained by various amounts of inorganics present in the switchgrass samples. As aforementioned in the Table 1, the extracted samples in the first year still contained high ash content (5.9 wt%) after the solvent extraction whereas the extracted samples in the second and third year had low ash content of 2.1 and 2.4 wt%, respectively. Pearson's correlation analysis also demonstrated that the yield of levoglucosan (Table 3 and supporting information Figure A1) increased with a reduction of K and Mg content showing a negative correlation (r = −0.70) whereas the dehydrated levoglucosan, 1,4:3,6-diahydro-a-D-glucopyranose (Gardiner, 1966), decreased with a reduction of K and Mg contents with a positive correlation value of 0.80. Furthermore, the extracted samples in the second and third year had a small residual amount of K and Mg with a significant removal efficiency, which only interacts with the formation of levoglucosan from carbohydrates and do not have any effect on its secondary degradation into lower molecular compounds such as light oxygenated compounds and furans/pyrans/cyclopentenes (Figure 6). In particular, significant removal (97–99%) of potassium, which is known to possess a stronger catalytic strength than magnesium and calcium (Muller-Hagedorn et al., 2003), from switchgrass harvested in the second and third year via water-ethanol extraction significantly promoted the yield of levoglucosan (Figure 6).
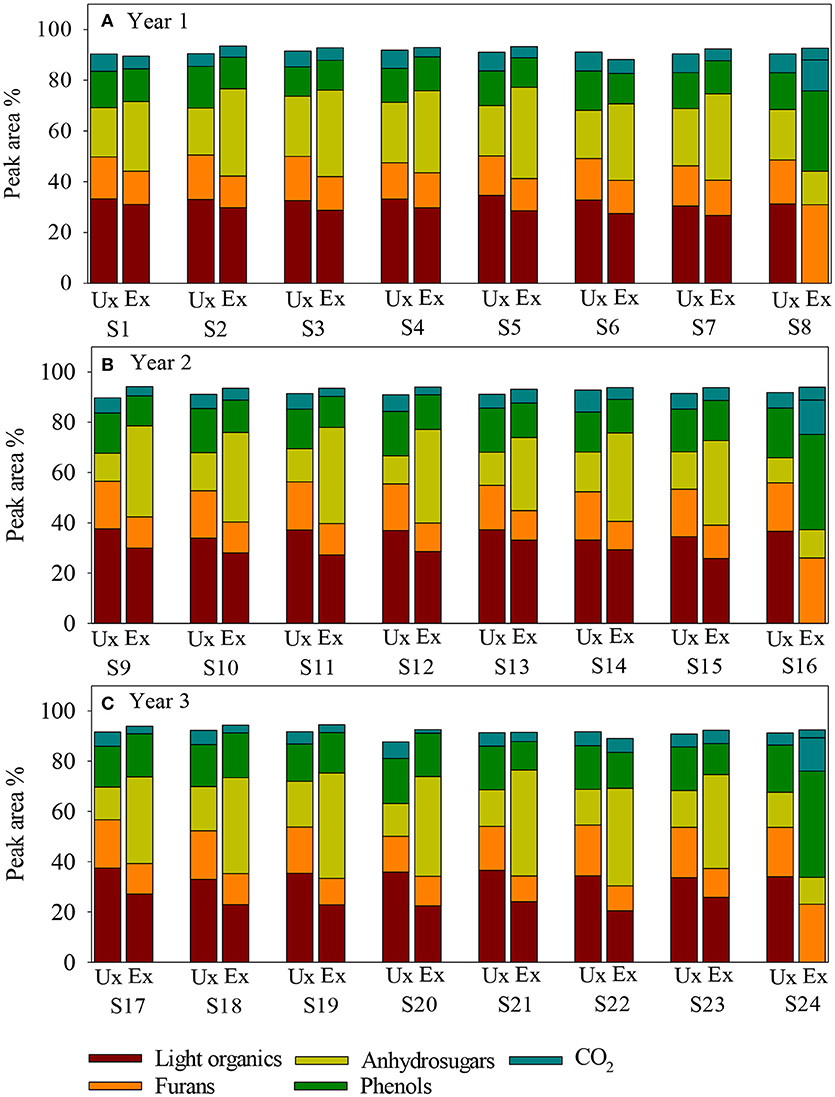
Figure 6. Distribution of chromatographic peak area % of pyrolytic products in the untreated (Ux) and extractives-free (Ex) switchgrass samples harvested for 3 consecutive years. (A) year 1, (B) year 2, (C) year 3.
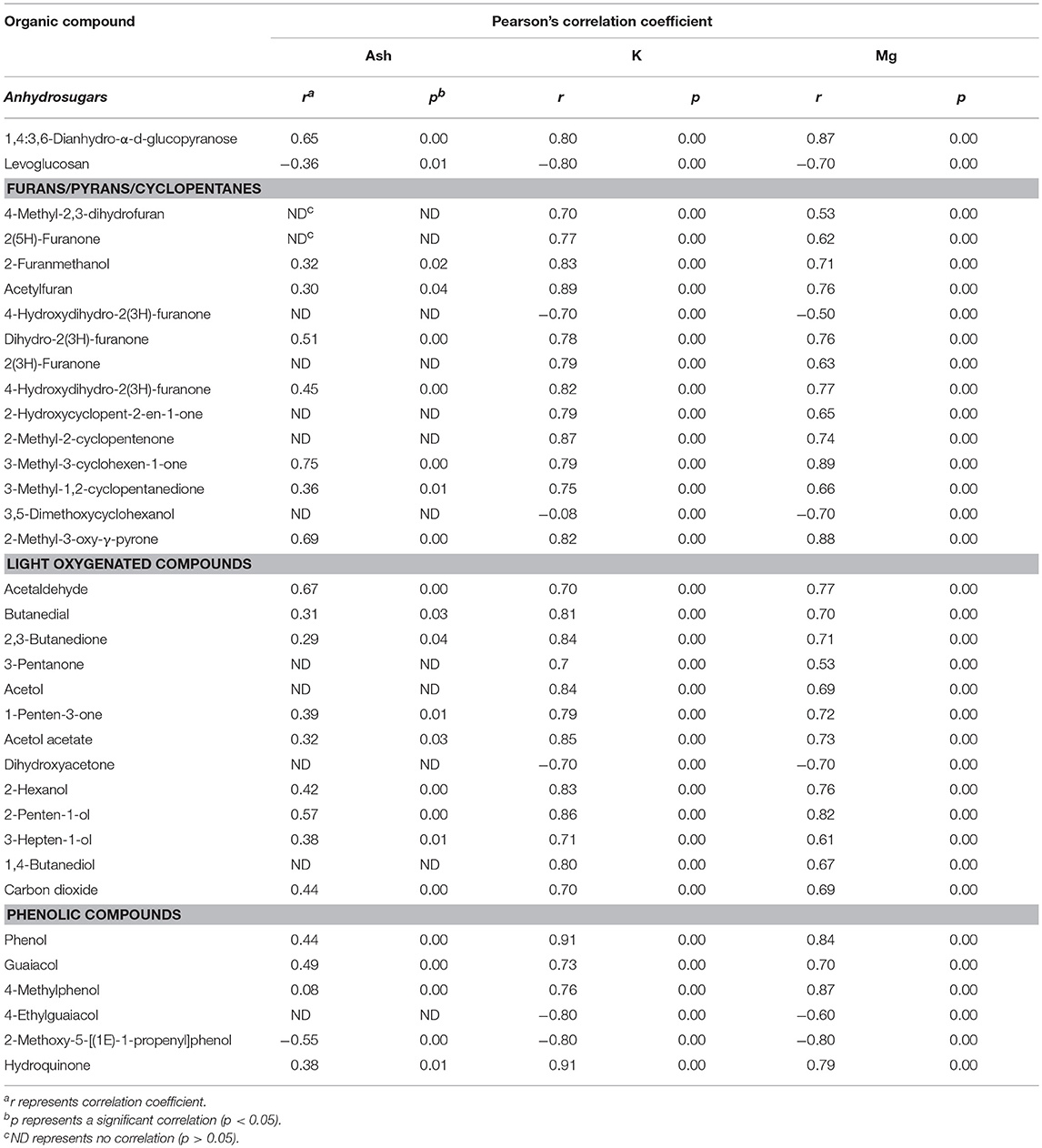
Table 3. Pearson's correlation between inorganic compounds in the unextracted and extracted switchgrass samples and the pyrolytic organic compounds produced by Py-GC/MS.
As the yield of anhydrosugars increased with decreasing inorganics content, the amount of low molecular weight oxygenates such as acetic acid, butanedial, acetol, and other low carbon-atom fragments gradually decreased, exhibiting a stronger positive correlation with K (r = 0.70) than with Mg (r = 0.53) content (Figure 6, Table 3, and supporting information Figure A2). These light organic compounds are promoted by fragmentation of glucose rings, known as ring-scission (Zhou et al., 2014), at the loss of glycosidic bond breakage for levoglucosan formation in the presence of alkali and alkaline earth metals. The yield of furanic/pyranic/cyclopentanic compounds such as 2-furanmethanol, 3-methyl-1,2-cyclopentanediion, 2-methyl-3-oxy-γ-pyrone, and other compounds decreased with a reduction of inorganics content (Figure 6) showing a stronger positive correlation with K (r = 0.70) than Mg (r = 0.53) (Table 3 and supporting information Figure A3). Moreover, 4-hydroxydihydro-2(3H)-furanone and 3,5-dimethoxycyclohexanol exhibited a strong negative correlation with K and Mg indicating the fate of the catalytic depolymerization reaction of carbohydrates in the presence of these inorganic species (Eom et al., 2011). Non-condensable gases (NCGs), such as carbon dioxide, also exhibited a strong positive correlation with K and Mg (Table 3). Therefore, a reduction of K and Mg via a simple solvent extraction process resulted in increasing yields of anhydrosugar-derivatives and decreasing low molecular weight species and NCGs. The yield of lignin-derived phenolic compounds decreased with a reduction of inorganic compounds (Figure 6). Specifically, monomeric phenolic compounds, such as phenol, guaiacol, and other compounds (Supporting information Figure A4), showed a strong positive correlation with K (r = 0.73) and Mg (r = 0.70), whereas 4-ethylguaiacol and 2-methoxy-5-[(1E)]-1-propenyl]phenol had a strong negative correlation (Table 3). These results indicated that inorganics promote the decomposition of the side chains of lignin structures via demethylation, demethoxylation, and dehydration reactions (Kleen and Gellerstedt, 1995; Mourant et al., 2011).
Conclusions
This study concluded that a non-structural components extraction step using water and ethanol produced valuable extractives while simultaneously resulting in removing undesirable catalytic inorganics, which ultimately improves pyrolytic bio-oil quality. The organic extractives were extracted up to 8.5 wt% in the first season biomass and between 5.8 and 6.3 wt% in the second and third season biomass, respectively, on the biomass dry basis. During the extraction step, ash removal ranged from 0.7 to 2.7 wt% with a predominant reduction of potassium and magnesium content in switchgrass. Pyrolysis of the extractives-free switchgrass harvested from the second and third season produced anhydrosugars two and three times more than that from the first season and reduced the yield of furanic/pyranic/cyclopentanic and light oxygenated compounds. From this study, we demonstrated that the removal of non-structural components increases the quality of switchgrass by decreasing its total ash content, specifically potassium and magnesium content. This approach could be applied to any biomass that contained detrimental amount of inorganics. The implementation of such step prior to a pyrolysis process of lignocellulosic biomass has a potential to produce valuable extractives while improving the quality of the feedstock for thermochemical processes.
Author Contributions
PK performed the extraction, collected the PyGC/MS data, investigated the correlation between the different set of data, and wrote the first draft of the manuscript. CH collected the ash and inorganic data and edited the manuscript. TE collected the TGA data and assisted in writing the manuscript. NL designed the study, contributed in data analysis, and was heavily involved in revising the manuscript. All authors approved the final document.
Conflict of Interest Statement
Presently, PK is employed by TerraPower, LLC but he performed all the work while being employed by the University of Tennessee.
The remaining authors declare that the research was conducted in the absence of any commercial or financial relationships that could be construed as a potential conflict of interest.
Acknowledgments
The authors would like to acknowledge the Southeastern Partnership for Integrated Biomass Supply Systems (IBSS), which is supported by Agriculture and Food Research Initiative Competitive Grant no. 2011-68005-30410 and 2013-67021-21158 from the USDA National Institute of Food and Agriculture, for support of this work.
Supplementary Material
The Supplementary Material for this article can be found online at: https://www.frontiersin.org/articles/10.3389/fenrg.2018.00096/full#supplementary-material
References
Baxter, H. L., Mazarei, M., Fu, C., Cheng, Q., Turner, G. B., Sykes, R. W., et al. (2016). Time course field analysis of COMT-downregulated switchgrass: lignification, recalcitrance, and rust susceptibility. Bioenerg. Res. 9, 1087–1100. doi: 10.1007/s12155-016-9751-1
Bridgwater, A. V. (2012). Review of fast pyrolysis of biomass and product upgrading. Biomass Bioenerg. 38, 68–94. doi: 10.1016/j.biombioe.2011.01.048
Eom, I. Y., Kim, K. H., Kim, J. Y., Lee, S. M., Yeo, H. M., Choi, I. G., et al. (2011). Characterization of primary thermal degradation features of lignocellulosic biomass after removal of inorganic metals by diverse solvents. Bioresour. Technol. 102, 3437–3444. doi: 10.1016/j.biortech.2010.10.056
Froment, K., Seiler, J. M., Defoort, F., and Ravel, S. (2013). Inorganic species behaviour in thermochemical processes for energy biomass valorisation. Oil Gas Sci. Technol. 68, 725–739. doi: 10.2516/ogst/2013115
Gardiner, D. (1966). The pyrolysis of some hexoses and derived di-, tri-, and poly-saccharides. J. Chem. Soc. C. 1473–1476. doi: 10.1039/j39660001473
Grønli, M. G., Várhegyi, G., and Di Blasi, C. (2002). Thermogravimetric analysis and devolatilization kinetics of wood. Ind. Eng. Chem. Res. 41, 4201–4208. doi: 10.1021/ie0201157
Kim, P., Johnson, A., Edmunds, C. W., Radosevich, M., Vogt, F., Rials, T. G., et al. (2011). Surface functionality and carbon structures in lignocellulosic-derived biochars produced by fast pyrolysis. Energy Fuels 25, 4693–4703. doi: 10.1021/ef200915s
Kim, P., Weaver, S., Noh, K., and Labbé, N. (2014). Characteristics of bio-oils produced by an intermediate semipilot scale pyrolysis auger reactor equipped with multistage condensers. Energy Fuels 28, 6966–6973. doi: 10.1021/ef5016186
Kleen, M., and Gellerstedt, G. (1995). Influence of inorganic species on the formation of polysaccharide and lignin degradation products in the analytical pyrolysis of pulps. J. Anal. Appl. Pyrolysis 35, 15–41. doi: 10.1016/0165-2370(95)00893-J
Kline, L. M., Johnson, A., Kim, P., Jackson, S., and Labbé, N. (2013). Monitoring switchgrass composition to optimize harvesting periods for bioenergy and value-added products. Biomass Bioenerg. 56, 29–37. doi: 10.1016/j.biombioe.2013.04.023
Kline, L. M., Labbé, N., Boyer, C., Yu, T. E., English, B. C., and Larson, J. A. (2015). Investigating the impact of biomass quality on near-infrared models for switchgrass feedstocks. AIMS Bioeng. 3, 1–22. doi: 10.3934/bioeng.2016.1.1
Labbé, N., Ownley, B. H., Gwinn, K. D., Moustaid-Moussa, N., and D'Souza, D. H. (2016). Antimicrobial and Anti-Inflammatory Activity of Switchgrass-Derived Extractives. United States Patent No 9,282,747. Knoxville, TN: University of Tennessee Research Foundation.
Lemus, R., Brummer, E. C., Moore, K. J., Molstad, N. E., Burras, C. L., and Barker, M. F. (2002). Biomass yield and quality of 20 switchgrass populations in southern Iowa, USA. Biomass Bioenerg. 23, 433–442. doi: 10.1016/S0961-9534(02)00073-9
Lian, J. N., Garcia-Perez, M., Coates, R., Wu, H. W., and Chen, S. L. (2012). Yeast fermentation of carboxylic acids obtained from pyrolytic aqueous phases for lipid production. Bioresour. Technol. 118, 177–186. doi: 10.1016/j.biortech.2012.05.010
Luque, L., Oudenhoven, S., Westerhof, R., van Rossum, G., Berruti, F., Kersten, S., et al. (2016). Comparison of ethanol production from corn cobs and switchgrass following a pyrolysis-based biorefinery approach. Biotechnol. Biofuels 9, 242–256. doi: 10.1186/s13068-016-0661-4
Michels, J., and Wagemann, K. (2010). The german lignocellulose feedstock biorefinery project. Biofuels Bioprod. Biorefin. 4, 263–267. doi: 10.1002/bbb.216
Mourant, D., Wang, Z., He, M., Wang, X. S., Garcia-Perez, M., Ling, K., et al. (2011). Mallee wood fast pyrolysis: effects of alkali and alkaline earth metallic species on the yield and composition of bio-oil. Fuel 90, 2915–2922. doi: 10.1016/j.fuel.2011.04.033
Muller-Hagedorn, M., Bockhorn, H., Krebs, L., and Muller, U. (2003). A comparative kinetic study on the pyrolysis of three different wood species. J. Anal. Appl. Pyrolysis 68–9, 231–249. doi: 10.1016/S0165-2370(03)00065-2
Nik-Azar, M., Hajaligol, M. R., Sohrabi, M., and Dabir, B. (1997). Mineral matter effects in rapid pyrolysis of beech wood. Fuel Process. Technol. 51, 7–17. doi: 10.1016/S0378-3820(96)01074-0
NREL (2008). “Determination of structural carbohydrates and lignin in biomass,” in Biomass Program Analysis Technology Team Laboratory Procedure (Golden, CO: NREL).
Parrish, D. J., and Fike, J. H. (2005). The biology and agronomy of switchgrass for biofuels. Crit. Rev. Plant Sci. 24, 423–459. doi: 10.1080/07352680500316433
Patwardhan, P. R., Satrio, J. A., Brown, R. C., and Shanks, B. H. (2009). Product distribution from fast pyrolysis of glucose-based carbohydrates. J. Anal. Appl. Pyrolysis 86, 323–330. doi: 10.1016/j.jaap.2009.08.007
Proestos, C., Boziaris, I. S., Nychas, G. J. E., and Komaitis, M. (2006). Analysis of flavonoids and phenolic acids in Greek aromatic plants: investigation of their antioxidant capacity and antimicrobial activity. Food Chem. 95, 664–671. doi: 10.1016/j.foodchem.2005.01.049
Sannigrahi, P., Ragauskas, A. J., and Tuskan, G. A. (2010). Poplar as a feedstock for biofuels: a review of compositional characteristics. Biofuels Bioprod. Biorefin. 4, 209–226. doi: 10.1002/bbb.206
Saporta, G., and Niang, N. (2009). “Principal component analysis: application to statistical process control,” in Data Analysis, ed G. Govaert (London: John Wiley & Sons), 1–23.
Shen, D. K., and Gu, S. (2009). The mechanism for thermal decomposition of cellulose and its main products. Biores. Technol. 100, 6496–6504. doi: 10.1016/j.biortech.2009.06.095
Thammasouk, K., Tandjo, D., and Penner, M. H. (1997). Influence of extractives on the analysis of herbaceous biomass. J. Agric. Food Chem. 45, 437–443. doi: 10.1021/jf960401r
Uppugundla, N., Engelberth, A., Vandhana Ravindranath, S., Clausen, E. C., Lay, J. O., Gidden, J., et al. (2009). Switchgrass water extracts: extraction, separation and biological activity of rutin and quercitrin. J. Agric. Food Chem. 57, 7763–7770. doi: 10.1021/jf900998q
Wang, H., Srinivasan, R., Yu, F., Steele, P., Li, Q., Mitchell, B., et al. (2012). Effect of acid, steam explosion, and size reduction pretreatments on bio-oil production from sweetgum, switchgrass, and corn stover. Appl. Biochem. Biotechnol. 167, 285–297. doi: 10.1007/s12010-012-9678-8
Yin, W., Tang, Z. C., Venderbosch, R. H., Zhang, Z., Cannilla, C., Bonura, G., et al. (2016). A one-step synthesis of C6 sugar alcohols from levoglucosan and disaccharides using a Ru/CMK-3 catalyst. ACS Catal. 6, 4411–4422. doi: 10.1021/acscatal.6b00296
Zhou, X. W., Nolte, M. W., Mayes, H. B., Shanks, B. H., and Broadbelt, L. J. (2014). Experimental and mechanistic modeling of fast pyrolysis of neat glucose-based carbohydrates. 1. Experiments and development of a detailed mechanistic model. Ind. Eng. Chem. Res. 53, 13274–13289. doi: 10.1021/ie502259w
Keywords: switchgrass, non-structural components, extractives, catalytic pyrolysis, ash, inorganics
Citation: Kim P, Hamilton C, Elder T and Labbé N (2018) Effect of Non-Structural Organics and Inorganics Constituents of Switchgrass During Pyrolysis. Front. Energy Res. 6:96. doi: 10.3389/fenrg.2018.00096
Received: 10 April 2018; Accepted: 31 August 2018;
Published: 15 October 2018.
Edited by:
Allison E. Ray, Idaho National Laboratory (DOE), United StatesReviewed by:
Selhan Karagoz, Karabük University, TurkeyHalil Durak, Yüzüncü Yil University, Turkey
Tianju Chen, Qingdao Institute of Bioenergy and Bioprocess Technology (CAS), China
Copyright © 2018 Kim, Hamilton, Elder and Labbé. This is an open-access article distributed under the terms of the Creative Commons Attribution License (CC BY). The use, distribution or reproduction in other forums is permitted, provided the original author(s) and the copyright owner(s) are credited and that the original publication in this journal is cited, in accordance with accepted academic practice. No use, distribution or reproduction is permitted which does not comply with these terms.
*Correspondence: Pyoungchung Kim, cHlvdW5nY2h1bmdAZ21haWwuY29t
Nicole Labbé, bmxhYmJlQHV0ay5lZHU=
†Present Address: Pyoungchung Kim, TerraPower, LLC, Bellevue, WA, United States