Corrigendum: Toward an Energy Efficient Wastewater Treatment: Combining a Microbial Fuel Cell/Electrolysis Cell Anode With an Anaerobic Membrane Bioreactor
- 1Center for Environmental Research and Sustainable Technology (UFT), University of Bremen, Bremen, Germany
- 2Laboratory for MEMS Applications, IMTEK – Department of Microsystems Engineering, University of Freiburg, Freiburg, Germany
Recently, it has been shown that combining a bioelectrochemical system (BES) with an anaerobic membrane bioreactor (AnMBR) to produce electricity can reduce the overall energy consumption of wastewater treatment. In this study, we tested the recently proposed concept that integrates a microbial anode into an AnMBR, under application relevant conditions, for the treatment of synthetic brewery wastewater. We developed two system configurations: a filtering anode with stainless steel filter plate; and a hybrid anode, in which a polymeric membrane is combined with stainless steel mesh. As fouling is problematic in AnMBRs, we investigated the effect of two fouling mitigation methods, namely electrochemical cleaning and application of a turbulence promotor, on the permeate fluxes and current densities. We also investigated the effect of cathode (counter electrode) position on the permeate fluxes and current densities in filtering and hybrid anode. Our results revealed that permeate fluxes were influenced by the membrane pore size; and dropped below 5 L m−2 hr−1 on day 3 with filter grade 0.5 μm; whereas similar values of permeate flux were observed after 5 days of operation with the membrane with filter grade 0.1 μm. COD removal across the membrane reached up to 644 mg L−1 indicating improvement in energy efficiency and effluent quality of the AnMBR. The location of cathode did not influence permeate fluxes and current densities, but permeate pH was largely affected. Electrochemical cleaning improved permeate fluxes more than 2-fold (18.9 L m−2 hr−1 after 7 days of operation) compared to the operation of the 0.1 μm membrane without a cleaning procedure. Application of a turbulence promotor increased permeate fluxes and current densities in filtering anode. The hybrid anode resulted in similar current densities, but higher permeate fluxes as compared to the filtering anode, which dropped below 20 L m−2 hr−1 only after 8 days of operation. The hybrid anode configuration is an attractive option that combines high permeate fluxes on conventional non-conductive filters with current generation on an inexpensive conductive material. In summary, our results demonstrate that combining BES with AnMBR is a promising approach toward an energy efficient wastewater treatment.
Introduction
Wastewater treatment is energy intensive, and with an increasing awareness for sustainability research toward the development of energy efficient treatment technologies has gained importance within the last decades. In this context, the Anaerobic membrane bioreactor (AnMBR) is a technology that can be applied for the treatment of both low and high-strength wastewater (Skouteris et al., 2012; Shin and Bae, 2018). Since it enables energy recovery by biogas production, it is a cost-effective alternative to aerobic treatment processes. In AnMBRs, the advantages of anaerobic digestion, such as high organic matter removal, low sludge production, and energy recovery, are combined with membrane separation that results in high effluent quality (Dvorák et al., 2016).
Bioelectrochemical systems (BESs) are emerging technologies proposed for the direct conversion of soluble organic matter in wastewater into electrical energy (MFC; microbial fuel cell) or to hydrogen (MEC; microbial electrolysis cell). Conversion of soluble organic matter into electricity in MFCs occurs via use of electroactive bacteria. These microorganisms are capable of transferring metabolic electrons across their cell membrane to an anode, which acts as terminal electron acceptor (Liu et al., 2005; Logan, 2009). Similarly, MECs depend on electroactive bacteria at the anode, but reduce protons into molecular hydrogen at the cathode. So far, BES technologies have mainly been applied at the laboratory scale; but they have been suggested to have a potential for industrial applications (He et al., 2017).
Recently it has been shown that a porous microbial anode operated with Geobacter sulfurreducens in synthetic acetate medium can at the same time serve as AnMBR filter operated in cross-flow configuration (Madjarov et al., 2018). The combination of the two technologies patented as filtration active fuel cell (Danzer and Kerzenmacher, 2014a,b) aims not only at electricity generation to reduce the energy demand of membrane bioreactors, but also at eliminating soluble COD in the effluent of AnMBRs (Yuan and He, 2015). In general, the organic acids (e.g., acetate, propionate) that are reported to constitute the majority of remaining COD in the permeate of AnMBRs (Smith et al., 2015), can be utilized by the electroactive bacteria and, therefore, improve the removal of organic matter. However, so far this new concept has only been demonstrated under idealized conditions with a synthetic acetate medium and the electroactive model organism G. sulfurreducens.
The overall objective of this study is to test the recently proposed concept for the integration of a microbial anode into an AnMBR by Madjarov et al. (2018) under application relevant conditions, using a mixed anaerobic microbial consortium treating synthetic brewery wastewater. To this end, we operated an AnMBR inoculated with a mixture of aerobic and anaerobic sludge from a wastewater treatment plant and Geobacter sulfurreducens. In this context, we explored the applicability of two different system configurations: a ‘filtering anode’ consisting of a flat plate stainless steel membrane filter; and a ‘hybrid anode’, in which an off-the-shelf polymeric filter membrane is combined with a stainless steel mesh acting as microbial anode. The filtering anode allows for simultaneous filtration and current production on the filter, whereas with a hybrid anode filtration occurs on the polymeric membrane and current is produced on a conductive metal mesh situated in front of it. The latter concept is particularly promising, since it would allow for an easy integration of a microbial anode with the variety of polymeric membrane materials already available for MBRs. We evaluated and compared the different configurations in terms of wastewater treatment characteristics; permeate fluxes and current densities in the filtering and hybrid anode with various cathode (counter electrode) positions.
A major concern with MBRs is the occurrence of membrane fouling and scaling and the resulting reduced permeate flux (Dereli et al., 2012), thus membrane cleaning and scaling control strategies constitute importance for application. This is of particular relevance in case of the filtering anode, where biofilm formation is important for current production but at the same time may lead to lower permeate flux. Furthermore, previous work has demonstrated that high permeate flux through the porous anode structure leads to increased current densities (Madjarov et al., 2018). We therefore also investigated the effect of two fouling mitigation methods on the permeate fluxes. The first method, electrochemical cleaning, has been shown as an effective fouling mitigation method in literature (Liu et al., 2013; Tian et al., 2014; Formoso et al., 2017). We investigated electrochemical cleaning through in-situ hydrogen generation, which can be implemented with any electrically conductive filtration membrane. Another promising approach to mitigate fouling is altering the hydrodynamics toward more turbulent conditions by introducing a turbulence promoter (Popović and Wessling, 2015; Armbruster et al., 2018). In this context, the stainless steel mesh used in the hybrid anode approach may be advantageous and double-function as anode and turbulence promoter.
Materials and Methods
Experimental Setup
For the experiments with filtering anode and hybrid anode, we used a commercial cross-flow filtration setup (Sartoflow Study, Sartorius, Germany) as described by Madjarov et al. (2018). The filtration device consisted of two main parts: a 5 L glass reactor and a custom-made polypropylene membrane cassette. The membranes with 10 × 100 mm size were fitted in a filter cassette, which accommodated either a stainless steel membrane used as anode or a PVDF membrane combined with a stainless steel mesh as anode, and a platinum mesh counter electrode operating as hydrogen evolution cathode (Figure 1).
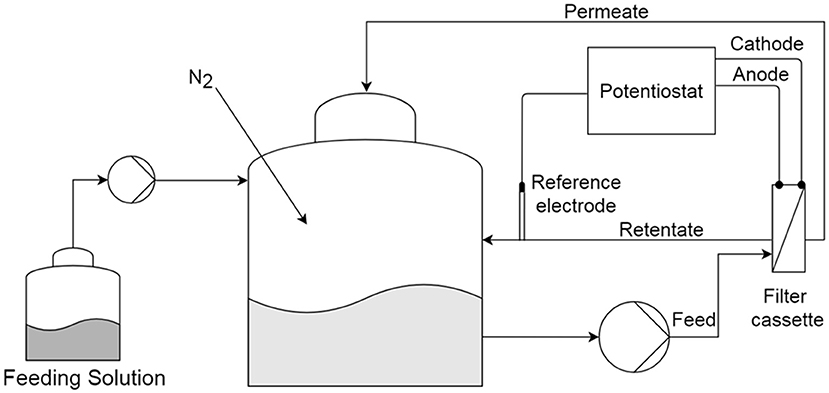
Figure 1. Experimental setup for the microbial anode integrated into an anaerobic membrane bioreactor.
A Gamry PCI4/300 potentiostat (Gamry Instruments, Warminster, USA) was used for analyzing electrochemical activities of the anode in a 3 electrode setup with a saturated calomel electrode (SCE) as reference electrode (KE11, Sensortechnik Meinsberg, Germany) in all experiments. All chronoamperometry experiments were carried out at an anode potential of −0.241V vs. SCE, except during the cleaning step in the electrochemical cleaning experiments. The potentials given in V vs. SHE were calculated by adding 0.241V to the potential measured vs. the SCE reference electrode. Counter electrodes were made from a platinum mesh (No. 900338, Chempur GmbH, Germany). All electrode positions are depicted in Figure 2. The default three-electrode positions are chosen as to minimize losses caused by uncompensated resistance (iR-drop).
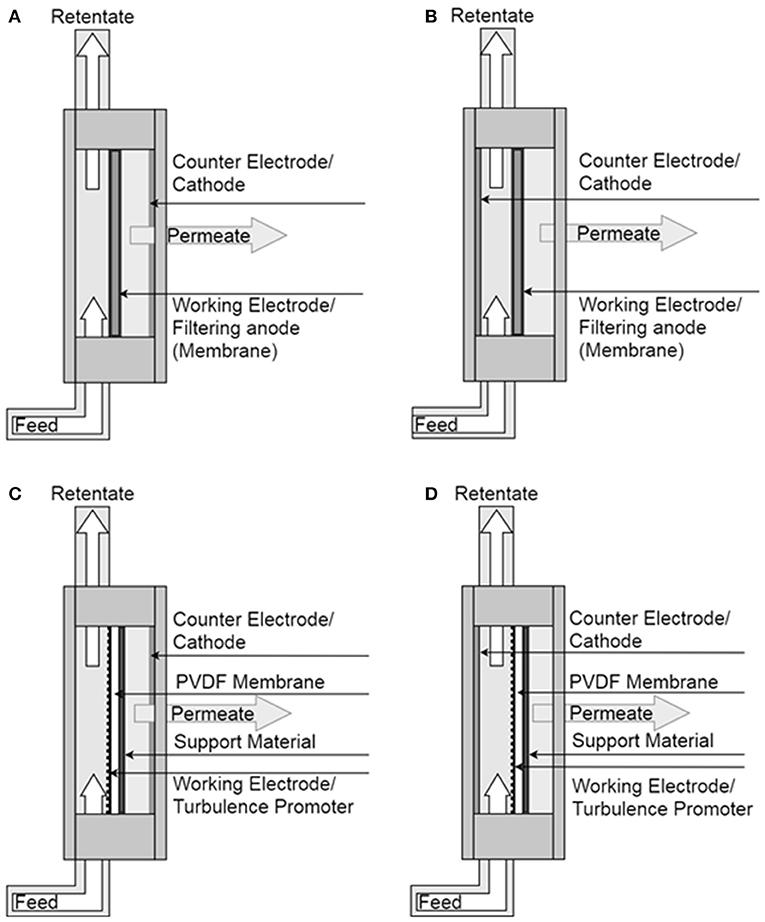
Figure 2. Illustration of filter cassette for various experimental configurations; filtering anode consists of stainless steel filter plate and cathode located on the permeate side (A) and on the retentate side (B); hybrid anode, in which a PVDF membrane combined with stainless steel mesh and cathode located on the permeate side (C) and on the retentate side (D).
Operation of Anaerobic Membrane Bioreactor
The experiments were performed in two sets, using two sludge contents in the reactor that were inoculated at different times. The first set of experiments were conducted with Sludge I, which was inoculated with Geobacter sulfurreducens, and sewage sludge containing anaerobic and aerobic sludge from a municipal sewage treatment plant with a volume ratio of 80% and 20%, respectively, as described by Madjarov and Kerzenmacher (2017). The second reactor content, Sludge II, was inoculated by a mixture of sludge, which contained 5, 15, and 80% of supernatant from aerobic sludge, anaerobic sludge and deionized water volume ratio, respectively, and filled up to 3 L after. To obtain supernatants, the aerobic and anerobic sludge were left for 4 h in airtight containers for settling.
The reactor head space was constantly purged with N2 to achieve anaerobic conditions. Reactor pH was controlled at 7 using 1M NaHCO3 or 2M HCl solutions. The temperature inside the reactor was held constant at 30°C by using a heat exchanger in combination with a thermostat (FP50-ME, Julabo, Germany).
The reactor content was pumped with a 4-piston diaphragm pump. Retentate and permeate were recirculated to the reactor. The transmembrane pressure (TMP; difference between the pressure on the feed and the permeate side) and cross-flow velocity were constantly held at 1 bar and 0.9 m s−1, respectively. The chosen cross-flow velocity was comparable to state-of-the-art AnMBRs (Dereli et al., 2012). The reactor was fed with synthetic brewery wastewater in batch during the week days during the first set of experiments, and daily in continuous feeding mode during the second set of experiments (as specified in Table 1) with 1 g COD L−1 day−1 organic loading rate. The feeding solution was provided with a peristaltic pump (Ismatec REGLO Digital MS-4/6, Cole-Parmer GmbH, Germany). The synthetic brewery wastewater was prepared by diluting Rothaus Tannenzäpfle beer (Badische Staatsbrauerei Rothaus AG, Germany) with deionized water (80% v:v). The chemical characteristics of the beer can be found in Supplementary Table 1.
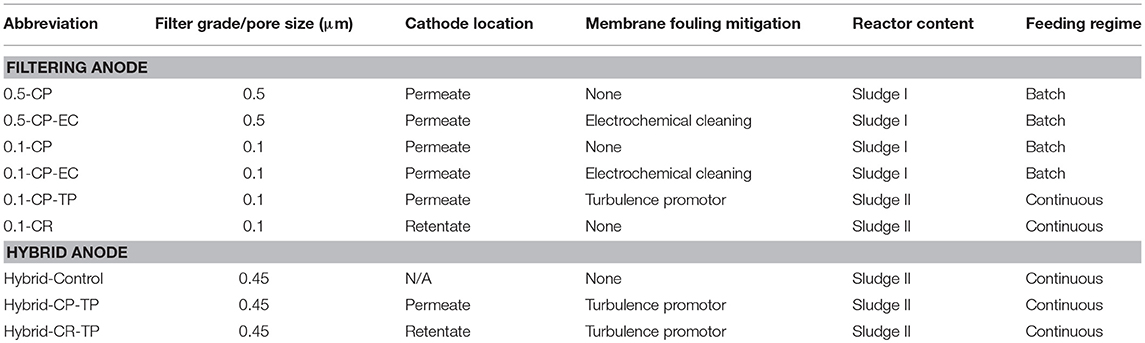
Table 1. Overview of the experiments performed in two system configurations using stainless steel membranes of filter grades 0.1 and 0.5 μm filtering anode; with electrochemical cleaning (EC) and turbulence promotor (TP) application as fouling mitigations methods, and the cathode located on the permeate side (CP) and retentate side (CR); and using polymeric membrane alone (Hybrid-Control) and polymeric membrane combined with a stainless steel mesh for hybrid anode configuration; with turbulence promotor (TP) application as fouling mitigation method, and the cathode located on the permeate side (CP) and retentate side (CR).
Aerobic and aneorobic sludge were taken from a municipal wastewater treatment plant in Teningen-Köndringen (Abwasserzweckverband Untere Elz), in Germany. The added electroactive strain in the reactor, Geobacter sulfurreducens PCA, was obtained from Johannes Gescher (Institute of Applied Biosciences, KIT, Karlsruhe, Germany). Geobacter sulfurreducens was prepared from a cryogenic culture in an anaerobic medium according to Pham et al. (2008) and 10 mL of the culture with an optical density of (OD600) of 0.86 was added into the reactor after washing the cells.
Filtering Anode Experiments
Simultaneous use of filtration membranes as microbial anodes was demonstrated by using commercially available electrically conductive filters of different filter grades made from sintered stainless steel (CrNi-steel grade 1.4404; GKN, Radevormwald, Germany), as suggested by Madjarov et al. (2018). To test the effect of membrane pore size, 2.5 mm thick membranes with filter grade of 0.5 μm (SIKA-R 0.5 AX) and 0.1 μm (SIKA-R 0.1 AS) were used. The 0.1 μm filter grade was asymmetric, with only a 200 μm thick layer of filter grade 0.1 μm on top of a macroporous carrier. All filters were cleaned in acetone, isopropanol and deionized water in an ultrasonic bath for 10 min each prior to experiments.
Hybrid Anode Experiments
For the hybrid anode experiments, PVDF membranes with a pore size of 0.45 μm (Millipore, Germany) were used as filtering membrane. The membranes were attached on the membrane cassette with the help of a stainless steel membrane with filter grade 1 μm as support material. As PVDF is not electrically conductive, a woven stainless steel mesh (DIN ISO 9044, DIN ISO 4783, Haver und Boecker, Oelde, Germany) with 0.224 mm wire diameter and aperture of 1 mm was used as anode. The same material was also tested as a turbulence promotor as described in the fouling mitigation experiments subsection. The stainless steel mesh was installed diagonally (45°) on top of the membrane. One experiment, namely Hybrid-Control, was conducted only with a PVDF membrane and without a stainless steel mesh application, to test the effect of turbulence promotor on permeate fluxes of the polymeric filter. An overview of the experiments conducted with the hybrid anode configuration is provided in Table 1.
Fouling Mitigation Experiments
We applied two fouling mitigation methods, electrochemical cleaning and introduction of a turbulence promotor. Electrochemical cleaning experiments were conducted by producing H2 on the membrane by water splitting. The current density was controlled by a potentiostat set to −0.1 Am−2. At this current density, gas evolution could be observed. The experimental procedure for electrochemical cleaning by the potentiostat consisted of four sequential steps; (i) open circuit potential for 5 min, (ii) chronoamperometry for 180 min at −0.241 V vs. SCE, (iii) open circuit potential for 5 min, (iv) electrochemical cleaning for 30 s (chronopotentiometry) at −0.1 Am−2.
Open circuit potential step was applied before and after each electrochemical cleaning step to reduce the sudden change in polarization of the electrodes between chronoamperometry steps and cleaning steps. Chronoamperometry experiment was run to observe the current generation as a result of the anode respiration of electroactive bacteria. This sequence was repeated throughout the entire duration of each electrochemical cleaning experiment (for 14 days).
The second fouling mitigation method is the introduction of a turbulence promotor. As described in hybrid anode experiments, a woven stainless steel with 0.224 mm wire diameter and aperture of 1 mm was installed diagonally (45°) on top of the membrane as a turbulence promotor. The mesh was fixed using adhesive tape (3M™ 851 polyester tape, 3M Germany,) and a silicone rubber gasket to have the same geometric surface area as the membrane.
Cathode Placement in Different Locations
The cathode (counter electrode) was placed in the filter cassette either on the permeate side (CP) or in the retentate chamber (CR) to investigate the effect of cathode position on the permeate fluxes and current densities in filtering and hybrid anode configurations. Cathode locations in the filter cassette are depicted in Figure 2.
Sampling and Analyses
Permeate flow was measured by collecting permeate over a defined period of time. Permeate was sampled directly from the permeate chamber through a three-way valve and was analyzed for turbidity, pH, and soluble COD. Turbidity and pH were measured immediately after sample collection using a turbidity meter (2100Q, Hach Lange, Germany) and pH meter (inoLab pH 720, WTW, Germany). Soluble COD was measured using Hach Lange test kit (LCK514) and DR3900 spectrophotometer (Hach Lange, Germany).
Mean current densities were calculated based on the current data over a period of 24 h after the last feeding event for batch fed experiments, and for the last 24 h of the continuously fed experiments (as shown in Supplementary Figure 1).
Results and Discussion
Wastewater Treatment Characteristics
Effect of Pore Size in Filtering Anodes
The results revealed that permeate fluxes are influenced by the membrane pore size. We observed initially higher permeate fluxes with 0.5 μm membranes compared to 0.1 μm membranes on the first day of the experiments, under similar operating conditions (Figure 3A). However, the permeate fluxes of 0.5 μm membranes decreased after day 1, resulting in lower permeate fluxes compared to 0.1 μm membranes (Figure 3B). In the experiments, where the membrane with filter grade 0.5 μm (0.5-CP) was used, the permeate flux went below 5 L m−2 hr−1 on day 3; whereas similar values of permeate flux were observed after 5 days of operation with the membrane with filter grade 0.1 μm (0.1-CP). The observed difference between the pore sizes was even more pronounced with electrochemical cleaning (EC), where 0.5-CP-EC and 0.1-CP-EC had permeate flux values below 5 L m−2 hr−1 on day 4 and day 14, respectively.
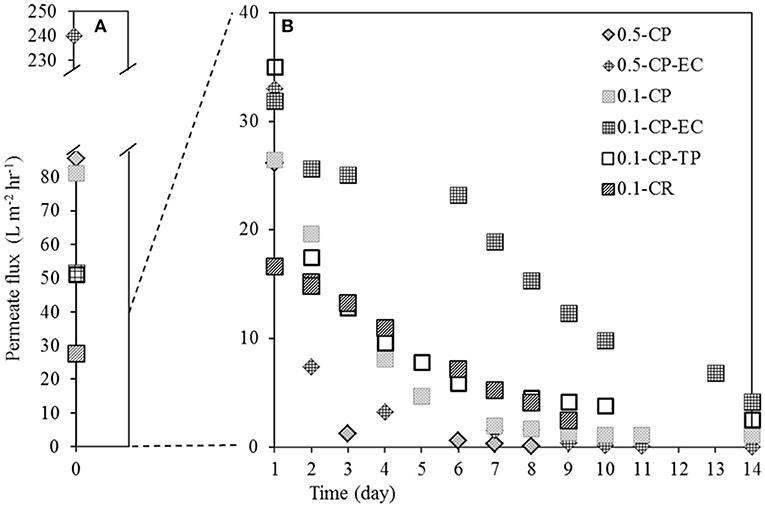
Figure 3. Permeate fluxes on (A) Day 0 and (B) Day 1-14 as a result of operating BES integrated with AnMBR with crossflow filtration using filtering anode of filter grade 0.1 and 0.5 μm stainless steel membranes; with electrochemical cleaning (EC) and turbulence promotor (TP) application as fouling mitigations methods, and the cathode located on the permeate side (CP) and retentate side (CR).
In literature, the effect of membrane pore size on the permeate flux in cross-flow filtration for wastewater treatment applications has previously been shown (Al-Malack and Anderson, 1997; Bendick et al., 2004). In a cross-flow filtration study with anaerobic bacteria, Elmaleh and Abdelmoumni (1997) have shown that increasing pore size led to lower permeate fluxes, which is in line with our findings. The differences in permeate fluxes with different pore sizes might be attributed to the infiltration of bacteria inside the pores of membrane with filter grade 0.5 μm; whereas filter grade 0.1 μm is too small for the size of bacteria to colonize. Bendick et al. (2004) reported the 0.2 μm membrane performing with a greater permeate flux rate than the 0.8 μm membrane as a result of a severe internal fouling of the larger pore size membrane. Similarly, Madjarov et al. (2018) studied cross-flow filtration with stainless steel membrane with filter grades 0.5 and 1 μm, and reported the presence of bacteria inside the pores throughout the membrane, which can clog the pores, and therefore lead to a decrease in permeate fluxes.
Together with the permeate flux rates, effluent quality constitutes an importance in membrane filtration processes. High concentrations of soluble COD in the effluent of AnMBRs are problematic in terms of effluent quality (Smith et al., 2013; Shoener et al., 2016). We observed a decrease of up to 644 mg L−1 (mean value for the experimental period) in soluble COD concentration in the permeate (307 mg L−1) compared to soluble COD concentration inside the reactor (951 mg L−1) for 0.1 μm membrane with a cathode located in the retentate side (0.1-CR) as shown in Supplementary Table 2. The difference in COD concentrations inside the reactor and in the permeate sample proves soluble COD removal across the membrane and a substantial improvement in effluent quality of AnMBR. We also observed turbidities as low as 2.3 NTU in the effluent of 0.1 μm membranes, which demonstrates a high particle and bacteria retention. However, the turbidity was higher for the effluent of membranes with filter grade 0.5 μm and could only drop to 27 NTU, which indicates a poor retention as compared to 0.1 μm membranes.
One important observation regarding permeate quality with the filtering anodes was the increasing pH in permeate samples (Figure 4). In a microbial fuel cell, as a result of the respiration of electroactive bacteria on the anode, electrical current generation is coupled with proton release on the anode, and hydroxyl ion release on the cathode. Once the decrease in permeate fluxes is substantial, the accumulation of OH− occurs in the permeate chamber, where the cathode is located. Accumulation of OH− ions leads to an increase in permeate pH, since OH− ions are continuously generated in the permeate chamber throughout the experiment, despite lower permeate fluxes. In such a system, high permeate pH is not desired as it might trigger mineral precipitation (scaling) at the back side of the membrane, which might further decrease permeate fluxes due to the clogged pores. Since placement of cathode on the permeate side resulted in increasing permeate pH, we conducted follow-up experiments with different cathode locations, as shown in the next subsection.
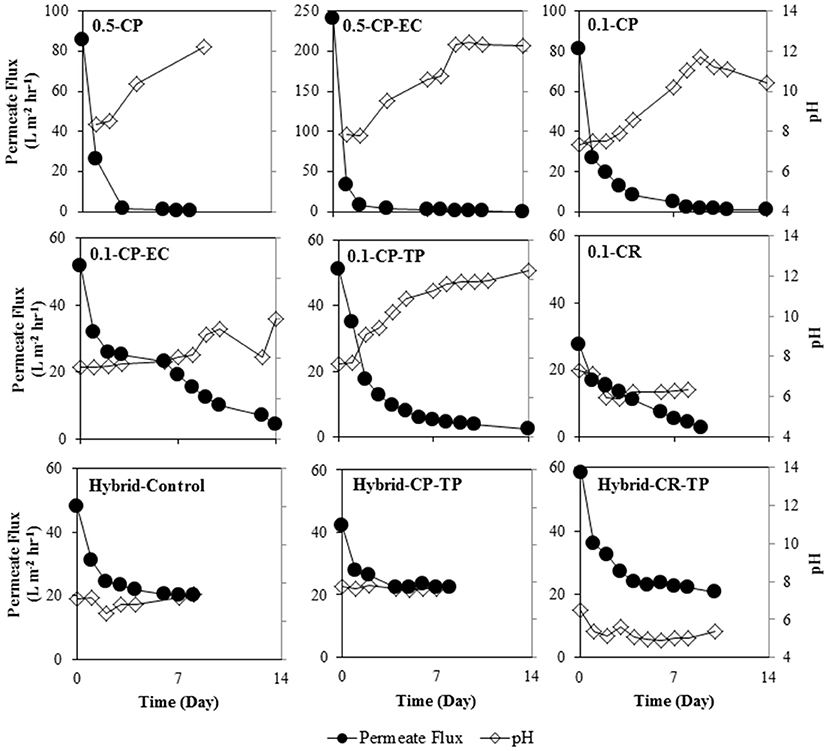
Figure 4. Permeate fluxes and permeate pH (note the two y-axes for permeate fluxes and pH on the left and right in each plot, respectively) over time as a result of operating BES integrated with AnMBR with crossflow filtration using filtering anode of filter grade 0.1 and 0.5 μm stainless steel membranes; with electrochemical cleaning (EC) and turbulence promotor (TP) application as fouling mitigations methods, and the cathode located on the permeate side (CP) and retentate side (CR); and using polymeric membrane alone (Hybrid-Control) and polymeric membrane combined with a stainless steel mesh for hybrid anode configuration; with turbulence promotor (TP) application as fouling mitigation method, and the cathode located on the permeate side (CP) and retentate side (CR).
Effect of Cathode Location
Permeate fluxes were not largely affected by the cathode location as similar permeate fluxes were observed for 0.1 μm membranes, in which the cathode was located in the permeate side (0.1-CP) and in the retentate side (0.1-CR). However, the permeate water quality, in terms of permeate pH, was clearly influenced by the cathode location, as Figure 4 depicts. Accumulation of OH− ions led to an increase in permeate pH for all filtering anode experiments, except for 0.1-CR experiment, as discussed earlier. In case of 0.1-CR experiment, the cathode was located in the retentate side, and therefore OH− ions were carried with the retentate back into the reactor, thus the permeate pH was not influenced.
As mentioned previously, mineral precipitation might be triggered due to high pH, which might contribute to a further decrease in permeate fluxes due to the clogged pores. However, our results do not indicate a pH-induced scaling problem as a major fouling mechanism, since the permeate fluxes remained at similar levels with different cathode locations, which influences permeate pH. Nevertheless, our results suggest that the cathode location is an important parameter affecting permeate quality in terms of permeate pH, and should be taken into consideration when designing a system, where a microbial anode is integrated into an AnMBR.
An interesting result is that, in case of the hybrid anode configuration with the cathode placed in the retentate chamber (Hybrid-CR-TP, Figure 4) at a value below 6, the pH of the permeate is lower than the pH inside the reactor. This indicates proton transfer from the microbial anode to the permeate chamber, and also that CO2 produced at the anode (through microbial COD removal) is transported to the cathode chamber, leaving the system. Such a transport of CO2 would constitute an additional feature of our system: if running the system as microbial electrolysis cell, the hydrogen produced at the cathode (placed in the retentate chamber) could stay inside the reactor. The leaving CO2 would thus not dilute the biogas, while the produced hydrogen can contribute to biogas upgrading via hydrogenotrophic methanation of CO2 inside the reactor. This biogas upgrading with increased methane concentration in the AnMBR would lead to a more energy efficient wastewater treatment. Further research is required to quantify and optimize this beneficial effect.
Operation of Hybrid Anode
Application of the hybrid anode resulted in somewhat lower permeate fluxes at the beginning of the experiments (Figure 5A), but dramatically higher permeate fluxes (Figure 5B) compared to the filtering anode (Figures 3A,B) over the operational period, which can be related to the different membrane materials and characteristics. The membrane material used for hybrid anode experiments was PVDF, whereas it was stainless steel for filtering anode experiments. Membrane properties have an important impact on fouling and therefore permeate fluxes, as membrane fouling results from the interaction between membrane surface and sludge suspension (Lin et al., 2013).
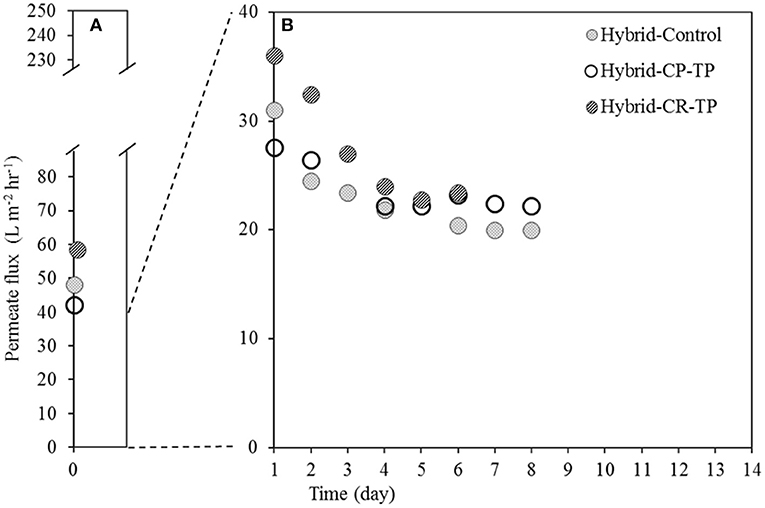
Figure 5. Permeate fluxes on (A) Day 0 and (B) Day 1–14 as a result of operating BES integrated with AnMBR with crossflow filtration using polymeric membrane alone (Hybrid-Control) and polymeric membrane combined with a stainless steel mesh for hybrid anode configuration; with turbulence promotor (TP) application as fouling mitigation method, and the cathode located on the permeate side (CP) and retentate side (CR).
Permeate pH was also influenced by the hybrid anode operation, as shown in Figure 4. As explained previously, hydroxyl ion release on the cathode is coupled with proton release on the anode during the electrical current generation. In filtering anode experiments (cathode on the permeate side), permeate pH usually increases up to 12, whereas in the hybrid anode experiments it remains around 8. This can be related to the higher permeate fluxes of the hybrid anode experiment (Figures 3B, 5B), which flush and dilute the cathodic compartment preventing the accumulation of hydroxyl ions. The relation between the permeate fluxes and pH is confirmed by the electrochemical cleaning experiment with the filtering anode (0.1-CP-EC), as the pH increases only after 8 days of operation, when fluxes drop below 20 L m−2 hr−1 (Figure 4).
Effect of Fouling Mitigation Methods
Electrochemical cleaning of the filtering anode
The application of regular electrochemical cleaning led to increased permeate fluxes for 0.1 μm membrane (0.1-CP-EC) over a longer operational period, compared to the use of 0.1 μm membrane without an electrochemical cleaning (0.1-CP). The permeate flux after 7 days of operation was approximately 19 L m−2 hr−1, which is more than a 2-fold increase compared to the control experiment (Figure 3B). The cleaning mechanism during the electrochemical cleaning process is cathodic hydrogen evolution that occurs via the applied current on the stainless steel membrane. By producing gas bubbles on the filter, foulants are pushed away. Hashaikeh et al. (2014) also studied the periodic electrolysis using an electrically conductive membrane to mitigate membrane fouling, and compared filtration cycles of 30, 40, and 60 min. They reported that shorter filtration intervals have a higher flux recovery after cleaning.
The permeate fluxes decreased below 5 m−2 hr−1 only after 3 and 4 days of operation for 0.5-CP and 0.5-CP-EC, respectively. Our results show that electrochemical cleaning was effective only for 0.1 μm membrane and only showed a slight improvement for permeate fluxes with 0.5 μm membranes. This could be due to the biofilm developed inside the 0.5 μm membrane's pores, which might impede the liquid contact inside the pores, thus limit hydrogen gas generation. Even if hydrogen evolution happens throughout the filter, it is very likely that the locally detached biofilm inside the pores cannot be taken away. This is different for biofilm or foulants at the surface of the 0.1 μm filter which are additionally impinged by the shear forces of the cross-flow.
Our results indicate that electrochemical cleaning based on hydrogen evolution extends the operation period of a filtering anode with high permeate fluxes, which may have potential for applications. The advantage of electrochemical cleaning compared to other fouling mitigation methods, is that membrane operation does not have to be interrupted by the cleaning procedure, if the membrane is used as a filtering anode. The optimization of the electrochemical cleaning process by testing various current densities and cleaning periods should be studied for further improvement of permeate fluxes and the extension of membrane operation periods. In this paper, electrochemical cleaning was studied as a fouling mitigation method, and the principles behind the mechanism were not investigated in depth as it was not the focus here. However, further research should be conducted for understanding the different mechanisms of electrochemical cleaning for different membranes.
Turbulence promotor
Introducing a turbulence promotor on top of a 0.1 μm stainless steel filtering anode (0.1-CP-TP) and on a hybrid anode (Hybrid-CP-TP) resulted in a noticeable increase in permeate fluxes over the operational period, though the absolute values remained at a low level. After 8 days of operating a 0.1 μm membrane with a turbulence promotor, the permeate flux was 4.5 L m−2 hr−1, which was more than 2-fold increase compared to the control experiment.
In the hybrid anode system, the influence of a turbulence promotor was smaller. With a turbulence promoter in place, the permeate flux was around 22 L m−2 hr−1, whereas the control experiment (Hybrid-Control) yielded up to 20 L m−2 hr−1 on Day 8, which amounted to a 10% increase. The smaller effect of the turbulence promotor might be due to the fouling inside the pores, as the pore size of the membranes was 0.45 μm. The turbulence promotor is expected to mitigate fouling on the membrane surface and not inside the pores.
Turbulence promotors mitigate membrane fouling by enhancing particle back-transport and increasing the shear rate at the membrane surface (Armbruster et al., 2018). Therefore, they increase fluxes and energy efficiency compared to the conventional operation of membrane filtration (Popović and Wessling, 2015). However, the geometric characteristics: angle, mesh size, thickness, etc. constitute an importance in the efficiency of turbulence promotors (Da Costa et al., 1994). In this study, the results imply that introducing a turbulence promotor can improve permeate fluxes. The optimum turbulence promotor geometry should be investigated in a future research for further enhanced permeate fluxes, thus extension of membrane operation.
Current Densities
Effect of Pore Size
With 0.1 and 0.5 μm membranes, the mean current densities obtained with filtering anodes were comparable and less than 1 A m−2 (Figure 6). This indicates that the pore size does not influence current densities. Our findings are in line with the results from our previous study (Madjarov et al., 2018), in which we reported similar current densities with different pore sizes. However, in our previous study the current densities reached up to 5 A m−2 with 0.5 μm membranes operating with a defined culture of Geobacter sulfurreducens. In contrast, the current density observed in this present is almost five times lower. Nevertheless, lower current densities are to be expected, as the experiments of this study are conducted with a feed solution (beer) with lower organic acid (acetate) concentrations and lower buffer capacity compared to the previous one.
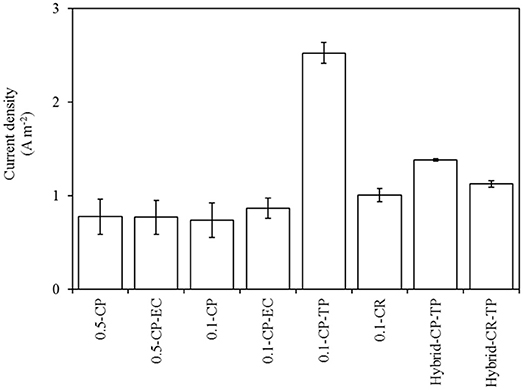
Figure 6. Mean current densities for 24 h after the last feeding event for batch fed experiments, and for the last 24 h of the continuously fed experiments (as shown in Supplementary Figure 1). Error bars show the standard deviations of current densities.
Current densities depend on the material type of the microbial anode, operational conditions and composition of the feed solution (substrate). In literature, the current densities achieved with the use of real wastewater range between 1 and 10 Am−2 (Kerzenmacher, 2017), which indicates that our results are within the range (see Supplementary Figure 1), but require further optimization.
Effect of Cathode Location
Changing the cathode location from permeate side (CP) to retentate side (CR) did not influence the current densities both for 0.1 μm filtering anode and the hybrid anode (Figure 6). In a microbial anode integrated AnMBR system, it might be more favorable to keep the cathode in the retentate side, rather than in the permeate side. By doing so, the permeate pH can be kept at a neutral level, which is an important parameter from the effluent quality point of view, as previously discussed in Wastewater treatment characteristics section.
As the applied potential on the anode is not iR corrected, changing the counter electrode position can affect the chronoamperometry experiment (Zhang et al., 2014; Madjarov et al., 2017) influencing the potential on the anode. From an electrochemical view, iR drop is negligible in case the counter electrode is placed on the permeate side (Madjarov et al., 2018). If there was a significantly different uncompensated resistance when placing the counter electrode in the retentate line, the anode potential would be lower in CR experiments compared to CP experiments. Consequentially current densities would always be underestimated in CR.
Operation of Hybrid Anode
The overall performance of the hybrid anode in terms of current densities was comparable to the filtering anode (Figure 6) and reached up to 2.48 A m−2 (Supplementary Figure 1). In a configuration with hybrid anode, the turbulence promotor acts as the anode for current generation. This result is noteworthy, as the turbulence promotor is fully exposed to the high shear forces of the cross-flow. The advantage of operating a hybrid anode, together with high permeate quality, is obtaining high permeate fluxes over longer operational periods, as discussed previously in Wastewater treatment characteristics section. Combining these advantages with current densities that are comparable to those of the filtering anode would allow for a decoupled operational mode for filtration and current generation. Yet another advantage of using polymer membranes in a hybrid anode configuration is the economic feasibility and easy availability of the material, especially due to wide application of polymer membranes in wastewater treatment industry, as compared to stainless steel membranes, which also requires higher material costs.
Effect of Fouling Mitigation Methods
Electrochemical cleaning of the filtering anode
Initially, the electrochemical cleaning procedure had a negative influence on the current generation, as the mean current density during day 0-7 was 0.11 A m−2 with electrochemical cleaning (0.1-CP-EC), which was lower compared to 0.98 A m−2 for the control experiment (Figure 7). However, during day 7–14 the current densities between the two experiments were more comparable. This indicates that the electrochemical cleaning step prevented the biofilm formation for the first 7 days of operation, which would be necessary for current generation on the filtering anode. As Figure 3 depicts, the permeate fluxes drop after 7 days of operation, which indicates fouling due to biofilm formation on the membrane surface.
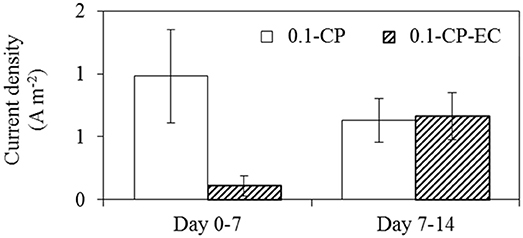
Figure 7. Mean current density of 0.1 μm membranes experiments; control experiment (0.1-CP) and electrochemical cleaning experiment (0.1-CP-EC) during day 0–7 and day 7–14, the error bars show the standard deviation of current densities.
Our results indicate that electrochemical cleaning improves permeate fluxes, but at the same time the current densities are negatively influenced. In the filtering anode configuration, an optimized biofilm formation through an optimized electrochemical cleaning would be desirable, which still allows for high permeate fluxes, while generating high currents.
Turbulence promotor
The application of a turbulence promotor together with the filtering stainless steel anode resulted in a higher current density (0.1-CP-TP) compared to the experiments without a turbulence promotor (0.1-CP), as depicted in Figure 6. We observed current densities reaching up to 5.40 A m−2 with 0.1 μm membrane (0.1-CP-TP), whereas only up to 3.90 A m−2 could be reached without a turbulence promotor (0.1-CP) throughout the operational period, as depicted in Supplementary Figure 1. This clear increase in current generation can be explained by the increase in specific anode surface area. The added electrically conductive turbulence promotor increases the anode area available for microbial colonization, while the projected surface area does not change. Similar results were obtained by Chen et al. (2012), who have reported increasing current densities as a result of increased anode surface area by using multiple layer of anode materials.
The role of a turbulence promotor on current generation in a hybrid anode system is prominent, since it acts as the anode for current generation. As for the filtering anode, introducing a turbulence promotor is a promising fouling mitigation method, which prompts the combined advantages of improved permeate fluxes and current densities, due to the increased specific anode surface area. Though, the turbulence promotor geometry remains as an important topic to be investigated in a future research for understanding its effects on both permeate fluxes and current densities.
Conclusions
In this paper, we demonstrated the applicability of a recently proposed technology to integrate a microbial anode into an AnMBR for the treatment of synthetic brewery wastewater, using a mixed anaerobic microbial consortium.
One of the most important observations in the present study is that the operation of a hybrid anode allows for high permeate quality and high permeate fluxes over longer operational periods than those obtained with the filtering anode. Combining these advantages with comparable current densities make hybrid anode an attractive option. The use of tried-and-tested polymer membranes in hybrid anode systems by placing a conductive mesh in front is easy to implement, economically feasible and significantly advanced in terms of experience and research on fouling mitigation compared to conductive membranes. Our results furthermore demonstrate substantial COD removal across the membrane, which contributes to a more energy efficient wastewater treatment and improved effluent quality in an AnMBR. In general, high effluent COD can be problematic in AnMBR systems, however, our results show that the integration of a microbial anode can reduce this problem. One interesting indication from our study was that the CO2 produced at the anode leaves the reactor with the permeate flow. As a result, biogas upgrading with increased methane concentration in the AnMBR becomes possible. This would lead to a more energy efficient wastewater treatment.
Cathode location does not influence permeate fluxes and current generation, but it is an important parameter affecting permeate quality that should be taken into consideration when designing a system, in which a microbial anode is integrated into an AnMBR. Membrane pore sizes in filtering anodes influence permeate fluxes, and lower pore sizes result in longer operational periods. On the other hand, we observed similar current densities in filtering anodes with different pore sizes. Electrochemical cleaning based on hydrogen generation is an effective method for fouling mitigation that extends the operation period of a filtering anode with high permeate fluxes. Introducing a turbulence promotor can improve permeate fluxes for both filtering and hybrid anodes. The optimization of both mitigation methods for further improvement of permeate fluxes and the extension of membrane operation periods should be investigated in future studies. As a general note, one limitation of our study is that the sequential experiments were conducted using the same two sludge (reactor content). The repeatability of the experiments might be an issue in reactors, where sludge consists of mixed cultures. The reactor content in our study consisted of a mixed culture, which might change characteristics over time and, therefore the permeate quality may be influenced by altering reactor characteristics. In a future research, the experiments should be repeated and the differences between replicates should be investigated, ideally by operating several reactors simultaneously. Another point is that our results are achieved using a synthetic brewery wastewater, and one should be aware that other wastewater types might yield different results. Therefore, we find it important to conduct further research using different type of wastewater with various organic strengths.
Overall, our study demonstrated promising strategies for energy efficient wastewater treatment. COD is converted directly into electricity using a combination of bioelectrochemical system combined with AnMBR as a novel technology for real applications. However, further research is needed to assess the complete energy balances by measuring methane production to obtain a broader evaluation of energy efficiency of the proposed technology.
Author Contributions
JM, SK, and NPK-S conceived and planned the experiments. SK and NPK-S supervised the project. PV and NPK-S carried out the experiments. All authors discussed and contributed to the interpretation of the results. NPK-S took the lead in writing the manuscript. JM and SK provided critical feedback. All authors contributed to the final manuscript.
Conflict of Interest Statement
The authors declare that the research was conducted in the absence of any commercial or financial relationships that could be construed as a potential conflict of interest.
Acknowledgments
We gratefully acknowledge financial support from the Baden-Württemberg Foundation for funding of the project F-MFC2, and Federal Ministry of Education and Research of Germany (BMBF) for funding of the project RECICL (Fkz.: 031B0365C).
Supplementary Material
The Supplementary Material for this article can be found online at: https://www.frontiersin.org/articles/10.3389/fenrg.2018.00095/full#supplementary-material
References
Al-Malack, M. H., and Anderson, G. K. (1997). Use of crossflow microfiltration in wastewater treatment. Water Res. 31, 3064–3072. doi: 10.1016/S0043-1354(96)00084-X
Armbruster, S., Cheong, O., Lölsberg, J., Popovic, S., Yüce, S., and Wessling, M. (2018). Fouling mitigation in tubular membranes by 3D-printed turbulence promoters. J. Memb. Sci. 554, 156–163. doi: 10.1016/j.memsci.2018.02.015
Bendick, J. A., Modise, C. M., Miller, C. J., Neufeld, R. D., and Vidic, R. D. (2004). Application of cross-flow microfiltration for the treatment of combined sewer overflow wastewater. J. Environ. Eng. 130, 1442–1449. doi: 10.1061/(ASCE)0733-9372(2004)130:12(1442)
Chen, S., He, G., Liu, Q., Harnisch, F., Zhou, Y., Chen, Y., et al. (2012). Layered corrugated electrode macrostructures boost microbial bioelectrocatalysis. Energy Environ. Sci. 5:9769–9772. doi: 10.1039/c2ee23344d
Da Costa, A. R., Fane, A. G., and Wiley, D. E. (1994). Spacer characterization and pressure drop modeling in spacer-filled channels.pdf. J. Memb. Sci. 87, 79–98. doi: 10.1016/0376-7388(93)E0076-P
Danzer, J., and Kerzenmacher, S. (2014a). Brennstoffzelle zur Filtration von Flüssigkeiten sowie Verwendung. Patent No DE 102013012663.
Dereli, R. K., Ersahin, M. E., Ozgun, H., Ozturk, I., Jeison, D., van der Zee, F., et al. (2012). Potentials of anaerobic membrane bioreactors to overcome treatment limitations induced by industrial wastewaters. Bioresour. Technol. 122, 160–170. doi: 10.1016/j.biortech.2012.05.139
Dvorák, L., Gómez, M., Dolina, J., and Cernín, A. (2016). Anaerobic membrane bioreactors—a mini review with emphasis on industrial wastewater treatment: applications, limitations and perspectives. Desalin. Water Treat. 57, 19062–19076. doi: 10.1080/19443994.2015.1100879
Elmaleh, S., and Abdelmoumni, L. (1997). Cross-flow filtration of an anaerobic methanogenic suspension. J. Memb. Sci. 131, 261–274. doi: 10.1016/S0376-7388(97)00049-5
Formoso, P., Pantuso, E., De Filpo, G., and Nicoletta, F. P. (2017). Electro-conductive membranes for permeation enhancement and fouling mitigation: a short review. Membranes (Basel). 7:E39. doi: 10.3390/membranes7030039
Hashaikeh, R., Lalia, B. S., Kochkodan, V., and Hilal, N. (2014). A novel in situ membrane cleaning method using periodic electrolysis. J. Memb. Sci. 471, 149–154. doi: 10.1016/j.memsci.2014.08.017
He, L., Du, P., Chen, Y., Lu, H., Cheng, X., Chang, B., et al. (2017). Advances in microbial fuel cells for wastewater treatment. Renew. Sustain. Energy Rev. 71, 388–403. doi: 10.1016/j.rser.2016.12.069
Kerzenmacher, S. (2017). “Engineering of microbial electrodes,” in Advances in Biochemical Engineering/Biotechnology, eds F. Harnisch and D. Holtmann (Berlin; Heidelberg: Springer).
Lin, H., Peng, W., Zhang, M., Chen, J., Hong, H., and Zhang, Y. (2013). A review on anaerobic membrane bioreactors: applications, membrane fouling and future perspectives. Desalination 314, 169–188. doi: 10.1016/j.desal.2013.01.019
Liu, H., Grot, S., and Logan, B. E. (2005). Electrochemically assisted microbial production of hydrogen from acetate. Environ. Sci. Technol. 39, 4317–4320. doi: 10.1021/es050244p
Liu, J., Liu, L., Gao, B., and Yang, F. (2013). Integration of bio-electrochemical cell in membrane bioreactor for membrane cathode fouling reduction through electricity generation. J. Memb. Sci. 430, 196–202. doi: 10.1016/j.memsci.2012.11.046
Logan, B. E. (2009). Exoelectrogenic bacteria that power microbial fuel cells. Nat. Rev. Microbiol. 7, 375–381. doi: 10.1038/nrmicro2113
Madjarov, J., Götze, A., Zengerle, R., and Kerzenmacher, S. (2018). Simultaneous use of a crossflow filtration membrane as microbial fuel cell anode – Permeate flow leads to 4-fold increased current densities. Bioresour. Technol. 257, 274–280. doi: 10.1016/j.biortech.2018.02.032
Madjarov, J., and Kerzenmacher, S. (2017). “Increasing COD removal and energy efficiency of an AnMBR by using the membrane as microbial anode,” in Increasing COD Removal and Energy Efficiency of an AnMBR by Using the Membrane as Microbial Anode (Florianópolis: The 14th IWA Leading Edge Conference on Water and Wastewater Technologies).
Madjarov, J., Popat, S. C., Erben, J., Götze, A., Zengerle, R., and Kerzenmacher, S. (2017). Revisiting methods to characterize bioelectrochemical systems: The influence of uncompensated resistance (iRu-drop), double layer capacitance, and junction potential. J. Power Sour. 356, 408–418. doi: 10.1016/j.jpowsour.2017.03.033
Pham, H. T., Boon, N., Aelterman, P., Clauwaert, P., De Schamphelaire, L., Van Oostveldt, P., et al. (2008). High shear enrichment improves the performance of the anodophilic microbial consortium in a microbial fuel cell. Microb. Biotechnol. 1, 487–496. doi: 10.1111/j.1751-7915.2008.00049.x
Popović, S., and Wessling, M. (2015). Turbulence promoters in membrane processes. J. Membr. Sci. 1–14. Available online at: http://media.journals.elsevier.com/content/files/turbulence-promoters-03180925.pdf
Shin, C., and Bae, J. (2018). Current status of the pilot-scale anaerobic membrane bioreactor treatments of domestic wastewaters: a critical review. Bioresour. Technol. 247, 1038–1046. doi: 10.1016/j.biortech.2017.09.002
Shoener, B. D., Zhong, C., Greiner, A. D. O., Khunjar, W., Hong, P.-Y., and Guest, J. S. (2016). Design of anaerobic membrane bioreactors for the valorization of dilute organic carbon waste streams. Energy Environ. Sci. 9, 1102–1112. doi: 10.1039/C5EE03715H
Skouteris, G., Hermosilla, D., López, P., Negro, C., and Blanco, Á. (2012). Anaerobic membrane bioreactors for wastewater treatment: a review. Chem. Eng. J. 198–199, 138–148. doi: 10.1016/j.cej.2012.05.070
Smith, A. L., Skerlos, S. J., and Raskin, L. (2013). Psychrophilic anaerobic membrane bioreactor treatment of domestic wastewater. Water Res. 47, 1655–1665. doi: 10.1016/j.watres.2012.12.028
Smith, A. L., Skerlos, S. J., and Raskin, L. (2015). Membrane biofilm development improves COD removal in anaerobic membrane bioreactor wastewater treatment. Microb. Biotechnol. 8, 883–894. doi: 10.1111/1751-7915.12311
Tian, Y., Li, H., Li, L., Su, X., Lu, Y., Zuo, W., et al. (2014). In-situ integration of microbial fuel cell with hollow-fiber membrane bioreactor for wastewater treatment and membrane fouling mitigation. Biosens. Bioelectron. 64, 189–195. doi: 10.1016/j.bios.2014.08.070
Yuan, H., and He, Z. (2015). Integrating membrane filtration into bioelectrochemical systems as next generation energy-efficient wastewater treatment technologies for water reclamation: a review. Bioresour. Technol. 195, 202–209. doi: 10.1016/j.biortech.2015.05.058
Keywords: bioelectrochemical systems, AnMBR, filtering anode, microbial anode, fouling mitigation, electrochemical cleaning, turbulence promotor
Citation: Kocatürk-Schumacher NP, Madjarov J, Viwatthanasittiphong P and Kerzenmacher S (2018) Toward an Energy Efficient Wastewater Treatment: Combining a Microbial Fuel Cell/Electrolysis Cell Anode With an Anaerobic Membrane Bioreactor. Front. Energy Res. 6:95. doi: 10.3389/fenrg.2018.00095
Received: 01 June 2018; Accepted: 30 August 2018;
Published: 19 September 2018.
Edited by:
Vânia Oliveira, Faculdade de Engenharia, Universidade do Porto, PortugalReviewed by:
Diogo M. F. Santos, Instituto Superior Técnico, Universidade de Lisboa, PortugalEnrico Negro, Università degli Studi di Padova, Italy
Copyright © 2018 Kocatürk-Schumacher, Madjarov, Viwatthanasittiphong and Kerzenmacher. This is an open-access article distributed under the terms of the Creative Commons Attribution License (CC BY). The use, distribution or reproduction in other forums is permitted, provided the original author(s) and the copyright owner(s) are credited and that the original publication in this journal is cited, in accordance with accepted academic practice. No use, distribution or reproduction is permitted which does not comply with these terms.
*Correspondence: Sven Kerzenmacher, kerzenmacher@uni-bremen.de