- Center for Microbial Ecology and Technology (CMET), Ghent University, Ghent, Belgium
Gas fermentation has rapidly emerged as a commercial technology for the production of low-carbon fuels and chemicals from (industrial) CO and/or CO2-rich feedstock gas. Recent advances in using CO2 and H2 for acetic acid production demonstrated that high productivity and substrate utilization are achievable. However, the costly constant addition of base and the energy-intensive nature of conventional recovery options (e.g., distillation) need to be overcome to drive organic acid production forward. Recently, membrane electrolysis has been presented as a technology that enables for the direct extraction of carboxylates across an anion exchange membrane (AEM) into a clean and low pH concentrate stream. Continuous in-situ extraction of acetate directly from the catholyte of a microbial electrosynthesis reactor showed that membrane electrolysis allows pure product recovery while improving productivity. Here we demonstrate that the system can be further enhanced through additional input of electrolytic hydrogen, produced at higher energetic efficiency while improving the overall extraction efficiency. A gas-lift reactor was used to investigate the hydrogen uptake efficiency at high hydrogen loading rates. During stable operation acetate transport across the membrane accounted for 31% of the charge balancing, indicating that the use of external H2 can lead to a more efficient use of the extraction across the membrane. By coupling membrane electrolysis with the gas fermentation reactor the pH decrease associated with H2/CO2 fermentations could be prevented, resulting in a stable and zero-chemical input process (except for the CO2). This now enables us to produce more than 0.6 M of acetic acid, a more attractive starting point toward further processing.
Introduction
In recent years, microbial electrosynthesis (MES) has emerged as a promising bioreactor technology for the production of multi-carbon compounds from CO2 and renewable electricity (Rabaey and Rozendal, 2010; Logan and Rabaey, 2012). This electricity-driven CO2-conversion process uses the cathode of a so-called bio-electrochemical system to supply the reducing equivalents (in the form of electrons and/or H2) for reducing CO2 in the Wood-Ljungdahl pathway (May et al., 2016). Thus far acetic acid has been the main natural end-product of acetogenic metabolism in MES (Nevin et al., 2010, 2011; Marshall et al., 2012; Jourdin et al., 2015, 2016b; Patil et al., 2015; Bajracharya et al., 2016; Chen et al., 2016; Song et al., 2018), but recent reports have demonstrated the production of higher-value organics like isopropanol (C3) (Batlle-Vilanova et al., 2017), butyric acid (C4) (Arends et al., 2017), and caproic acid (Vassilev et al., 2018) from CO2 feed. Since its first description in 2010 (Nevin et al., 2010), considerable advancements in MES performance have been achieved, but today production rates, energy efficiencies and product titers are far too low to push MES forward as an industrial relevant platform for CO2-based bioproduction (Desloover et al., 2012). Since production rates are ultimately limited by the applied current, it is essential to engineer MES systems that have the ability to deal with high electron supply rates at a high conversion efficiency and low power input (Gildemyn, 2016).
Gildemyn and co-workers have already demonstrated the advantages of using membrane electrolysis (ME) for MES. This approach can uniquely couple the production and recovery of acetic acid through in-situ product extraction across an anion exchange membrane (AEM) using nothing but an electrical current (Andersen et al., 2014; Gildemyn et al., 2015). The use of an AEM for MES can simultaneously separate, concentrate and acidify the product as a single organic acid in a solid-free extraction liquid, while enhancing performance through the combined effect of product recovery and in-situ pH control (Gildemyn et al., 2017). To date, the integrated MES approach for production and extraction is limited in terms of: (i) production rate; (ii) efficiency for electrons used for acetic acid recovery; and (iii) energy input requirements for acetic acid production. At best 40% of the electrons ended up in residual H2 during MES experiments at 5 A m−2 applied current density, indicating that the transfer of reducing power to the homoacetogenic culture needs optimization (Gildemyn et al., 2015; Patil et al., 2015;). At 100% efficiency for production and extraction, acetate transport can at most account for 12.5% of the charge balance, as 8 moles electrons are required per mole of acetic acid produced, while extraction of the monovalent acetate ion (theoretically) only requires one electron. Since the extraction efficiency is limited by the production rate, acetate experimentally accounts for only 5–8% of the charge balancing (Gildemyn et al., 2015, 2017). Most of the charge is thus balanced by other anions, mainly HCO. It should be recognized that the full extraction capacity of the reactor can only be utilized if additional acetic acid is produced with externally supplied reducing equivalents (as hydrogen gas). We thus proposed an improved design where acetate production from an external H2 source is linked to an extraction reactor providing only 12.5% of the total load of reducing equivalents, aimed at enhancing extraction efficiency at a lower power input. An alternative embodiment for this would be the extraction of acetate from an organic sidestream in combination with additional acetate production using the cathodic hydrogen.
Considering the aforementioned aspects, the focus of the present study was to investigate the impact of additional H2 injection in an external fermenter on: (i) the current efficiency for acetate extraction; (ii) the final acetic acid concentration in the extraction liquid; (iii) the acetic acid production rate of the integrated MES-extraction approach; and (iv) the energy input for acetic acid production. Operation of the MES reactor was modified by coupling it to a bubble-column fermenter and adding externally produced H2 to the reactor system to increase both H2 retention time in the aqueous medium and productivity. Accordingly, this work reports on the development of a platform for CO2 conversion based on existing gas fermentation technology coupled to membrane electrolysis as a tool for product recovery and pH control. Use of CO2 as a raw material for large scale bioproduction will require proper integration of autotrophic biotechnology to fully exploit the intrinsic power of CO2-based bioproduction.
Materials and Methods
Reactor Setup and Operation
The experimental setup included a three-chambered electrochemical cell, a two-chambered water electrolyzer and a custom-made glass bubble-column reactor (Figure 1). The three-chambered reactor consisted of three identical Perspex frames with a working volume of 0.2 L per chamber (20 × 5 × 2 cm inner dimensions). The anode compartment contained a 50 mM Na2SO4 solution as electrolyte (adjusted to pH 2 with sulfuric acid) and a 20 × 5 cm MMO-coated titanium mesh electrode (Magneto Special Anodes BV, The Netherlands). The cathode compartment contained a modified homoacetogenic medium (pH 7.7) as described by Gildemyn et al. (2015) and a carbon felt electrode (100 cm2 projected surface area, thickness of 3.18 mm, Alfa Aesar, Germany) with a stainless steel frame current collector. The initial volume of the catholyte was 1 L with the bubble-column reactor positioned in the recirculation loop op the cathode chamber. The electrolyte in the extraction compartment consisted of a 4-fold concentrated salt solution containing the same salts as the catholyte, adjusted to pH 2 with H2SO4. The initial working volume of the acolyte and extraction medium was 0.35 L, including an external recirculation flask. The anode and extraction compartments were separated by a cation exchange membrane (Fumatech FKB, Fumasep, Germany), while an AEM (Fumatech FAB, Fumasep, Germany) was placed in between the cathode and extraction chambers. All compartments were operated in batch mode during the entire experimental period (86 days) and recirculated at approximately 50 mL min−1. A N2/CO2 mixture (90/10%, v/v) was continuously bubbled into the cathode compartment at a flow rate of 28.5 ± 12.4 L d−1. The reactor was operated as a three-electrode setup using the cathode as working electrode and placement of a reference electrode (Ag/AgCl, 3 M KCl, + 210 mV vs. SHE, BASi) in the catholyte. A fixed reductive current of −50 mA (corresponding to a current density of −5 A m−2) was used to facilitate electrosynthesis by means of potentiostatic control (VSP, BioLogic, France).
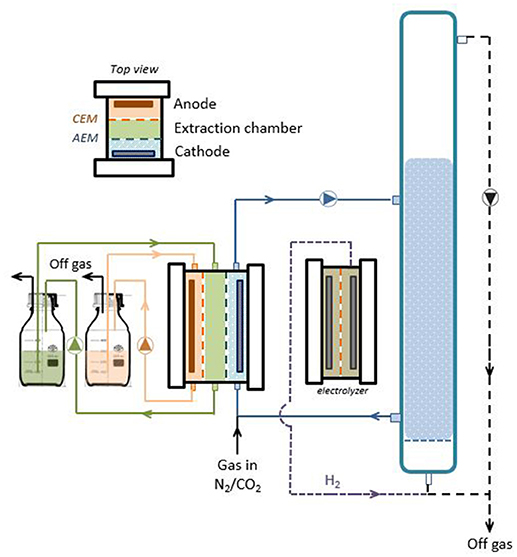
Figure 1. Bubble column reactor setup for simultaneous biological production and extraction of acetic acid from CO2 and electrical current through a hybrid microbial electrosynthesis-gas fermentation approach. An external fermenter is fed with additional hydrogen from a water electrolyzer to increase acetate production rates. Full black lines show liquid streams, dotted lines gas streams.
An additional two-chambered electrochemical cell was constructed using identical materials except that a stainless steel mesh was used as cathode material and a 0.5 M Na2SO4 solution was used as electrolyte in both reactor chambers. Both electrolytes were recirculated over a buffer vessel at high speed (~100 mL min−1) to ensure proper mixing. The cell was operated as a water electrolyzer (with CEM) at a fixed current density of −35 A m−2 (0.35 A), producing H2 gas that was sparged into the bubble column through anaerobic tubing.
The bubble-column was a cylindrical reactor with a volume of 2 L (1 m height, 5 cm internal diameter) and an integrated sintered glass to allow fine bubble dispersion. Connection to the MES cell was established through glass nipples on the side of the column. An ATEX gas pump (KNF Verder, Belgium) was installed to intensively recirculate the headspace gas through the fermentation medium (~ 15 L min−1). The same experimental procedures as described in Gildemyn et al. (2015) were used. Any liquid removed during sampling as well as liquid lost via electro-osmosis was replaced with an equal amount of sterile anaerobic stock solution. The experiments were conducted under anaerobic conditions, at room temperature (21 ± 2°C). The reactor setup (electrochemical cell + column) was inoculated at the start of the experiment up to a final cell density of ~ 105 viable cells mL with a pre-enriched autotrophic acetate-producing mixed microbial community that was used in previous MES experiments (Patil et al., 2015; Gildemyn et al., 2017). Gas and liquid samples were taken three times per week from each reactor compartment for monitoring gas composition, VFAs, alcohols, anions, cations, pH, conductivity and bicarbonate. Water transfer was estimated based on the volume changes in the different recirculation vessels. The flow rate of N2/CO2 was monitored by water displacement measurements prior to sampling. For abiotic control experiments (current but no bacteria as well as bacteria but no current) we refer to Gildemyn et al. (2015) and Patil et al. (2015) since these studies showed that in both control experiments no production of organic products or biomass was detected.
Analytical Procedures
Conductivity and pH were determined according to standard methods. VFAs, alcohols and inorganic anions were measured using ion chromatography as described in Gildemyn et al. (2015). Sodium, ammonium, potassium, magnesium, and calcium were determined on a 761 Compact Ion Chromatograph (Metrohm, Switzerland) using a conductivity detector. The device was equipped with a Metrosep C6 – 250/4 column and a Metrosep C4 Guard/4.0 guard column. The eluent was 1,7 mM HNO3, 1.7 mM dipicolinic acid. Gas samples were analyzed for the presence of N2, O2, H2, and CH4 by gas chromatography using a Compact GC (Global Analyser Solutions, Breda, The Netherlands) equipped with a thermal conductivity detector.
Data Representation
The calculations for the volumetric acetic acid production rate [based on the fermentation broth volume (g L−1 d−1)], electron recovery and energy efficiency are based on the methods described in (Patil et al., 2015). The calculation of electron recovery in unutilized H2 is based on the residual H2 concentration in the off-gas from the bubble column reactor. The extraction efficiency is defined as the ratio of extraction rate to production rate (of the whole system, electrolyzer + MES), while the charge balancing efficiency is defined as the ratio of the charge transported as a specific ion through a membrane and the total electrical charge of the extraction cell. Only the power input for electrochemical reactions (water splitting) is taken into account for specific energy input calculations.
In an abiotic test preceding the inoculation, the hydrogen production rate of both the MES cell (operated at a fixed current density of −5 A m−2) and the electrolyzer (operated at a fixed current density of −35 A m−2) was quantified. With a combined hydrogen gas flow rate of 3.5 ± 0.2 L day−1 leaving the reactor, the electron balance could be closed for 86.4 ± 0.1%, indicating some loss through tubing, connectors and sampling ports (provided 100% current efficiency for H2 production).
Results and Discussion
Additional Hydrogen Injection Enhances Acetic Acid Productivity
Production of acetic acid by the microbial community in a galvanostatic operated MES reactor started 10 days after inoculation. The longer lag-phase in this study (3–5 days in our previous studies) could potentially be attributed to the lower initial biomass concentration and the larger reactor volume. Once acetogenic activity started, gas recirculation was activated to improve the H2 mass transfer to the fermentation broth. A cathode potential of −1.21 ± 0.07 V vs. SHE was recorded during the experiment. Carbon fixation via homoacetogenesis allowed for a sustained increase in the concentration of acetic acid throughout the test. Acetic acid gradually accumulated in the extraction chamber, reaching 37.0 g L−1 (617 mM) on day 86 (Figure 2A). This is the highest titer of acetic acid reported so far for MES from CO2 feed. From day 56, acetate concentration in the catholyte remained fairly constant (4.1 ± 0.6 g L−1), while the concentration in the anolyte rose to reach 13.7 g L−1 by the end of the cycle. For the whole 86 days operation, the average acetic acid production rate was 0.76 gacetate L−1 d−1. Higher carbon fixation rates (1.48 g L−1 d−1) were observed during stable operation (from day 37 to 65), whereas a maximum value of 3.54 g L−1 d−1 can be reported. These results confirm that an 8 times higher H2 feeding rate and a higher H2 retention time (~ 1 h by continuous recirculation of the H2 headspace through the fermentation medium) resulted in 2.6–4.1 times higher acetic acid concentration and 2.1–2.7 times higher volumetric productivity compared to our previous studies (Gildemyn et al., 2015, 2017). Acetic acid accounted for 99.8% of all organic compounds present at the end of the experiment (as carbon, sum of products in all reactor compartments). Other carboxylates such as formate, propionate and butyrate were present but only in low concentrations (<50 mg L−1). Just as in our previous work, product diversification to alcohols was not observed and methane was not consistently detected in the off-gas. The batch cycle resulted in a total acetic acid production of 42.9 g acetic acid by day 86 (Figure 2B), resulting in an overall electron recovery in acetic acid of 21% (Figure 2C). When only taking into account the stable operation period, the coulombic efficiency (CE) was 41%. CE increased throughout the test, probably due to a higher biomass density in the fermentation broth, and reached a plateau from day 75 (Figure 2C). Unutilized H2 in the reactor off-gas resulted in an overall electron recovery in H2 of 45%. The electron balance can be further closed with the presence of other products (short-chain carboxylic acids and methane; <1%), losses of H2 gas through tubing, connectors and stoppers (13.6% based on abiotic quantification), and biomass production. The concentrations in the different reactor compartments did not reflect the real productivity of the system because water displacement between the compartments caused a change in the volumes throughout the reactor run. An average water flow across the AEM of 0.75 L m d−1 was observed (from cathode to extraction compartment), which was 11 times higher than the flow across the CEM (0.07 L m d−1). The water flux through the AEM is diluting the acetic acid stream, limiting the product titer in the extraction liquid so that a final concentration of 37 g L−1 (in 1.20 L) instead of 108 g L−1 (in 0.35 L) was achieved. It was observed that the water flux showed a linear dependency on current in the range of current densities tested (data not shown), and seems to be related to the hydration shell of the anions crossing the membrane (electro-osmosis) (Lakshminarayanaiah, 1969; Giorno et al., 2016).
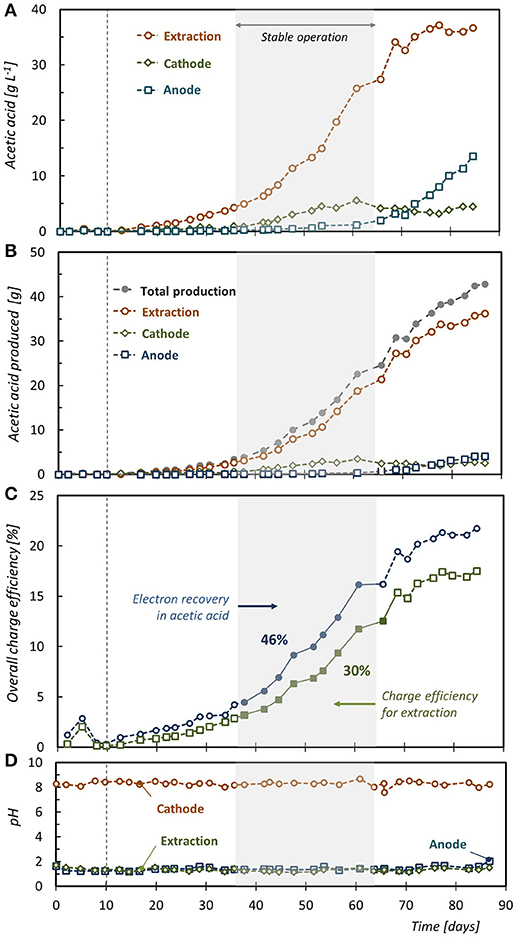
Figure 2. (A) Acetic acid concentration in the catholyte, middle compartment and anolyte. (B) Total mass of acetic acid produced, extracted across the AEM and present in the extraction liquid. (C) Overall charge efficiency for production and extration. (D) pH in the different reactor chambers. Dotted vertical lines represent the start of gas recirculation.
MES offers the intrinsic advantage to directly supply bacteria with electrons, however there is more and more evidence that production via MES is mainly driven by an indirect electron flow from the cathode to the acetogens, occurring via abiotically or biologically induced H2 production (Patil et al., 2015; Jourdin et al., 2016b). The crucial role of H2 in the conversion of CO2 to organics is thus creating the need for a MES reactor design that can work at high current density and consume high H2 fluxes. As discussed in previous reports, MES reactor design (often H-type, cylindrical or plate and frame type reactors) is not optimized for in-situ H2 conversion, leading to high losses of residual H2. Efforts to increase CEs have been focusing on 3D electrodes that supply H2 in the whole cathode chamber (Jourdin et al., 2016a; Song et al., 2018), but the scalability of these systems is questionable and channeling issues may arise when microbial growth completely fills the electrode pores (Klasson et al., 1991). Due to the fact that electrosynthesis is limited to the surface (and close surroundings) of the cathode reactor, scalability of this 2D system is more challenging compared to 3D gas fermentation systems. For the first time coupling MES to the gas fermentation platform is demonstrated as a strategy to achieve higher electron supply rates for CO2-based bioproduction. As a CO2-based bioproduction platform MES is still far behind H2/CO2 or syngas based fermentation in terms of production rates, energy efficiencies, scalability, and maturity, so integration within the gas fermentation platform could push MES forward as an elegant way to control/steer fermentation and achieve in-situ product recovery (see further). The coupling of MES to a bubble column reactor is also a promising strategy to increase the H2 retention in the reactor, thereby increasing the H2 conversion efficiency. However, more optimization will be needed to boost production and achieve high H2 uptake efficiencies typically obtained in optimized gas fermentation reactors (El-Gammal et al., 2017; Steger et al., 2017). The continuous supply of N2:CO2 gas mixture resulted in a relatively low H2 partial pressure of 0.07 ± 0.03 bar, limiting the driving force for H2 mass transfer from gas to liquid. The low gas-to-liquid mass transfer of H2 has been identified previously as the rate-limiting factor in gas fermentation processes (De Tissera et al., 2017). It could be expected that production rates will increase when H2 is not flushed out permanently, but accumulates in the headspace of a pressurized reactor system, increasing the pH2. The use of pure CO2 (limiting the dilution of H2/CO2 with N2) or intermittent sparging of CO2 (for example pH or [CO2]dissolved controlled) could be exploited as gas feeding strategies to increase pH2. Efforts to increase the volumetric mass transfer coefficient (kLa) mainly focus on increasing the interfacial surface area for mass transfer via mixing, microbubble sparging, or the use of packing material (Orgill et al., 2013). Bioreactor designs such as bubble, immobilized cell and trickle bed columns are proposed as low-cost reactor platforms for gas fermentation (De Tissera et al., 2017). Although the design and operation of gas fermentation reactors has reached industrial scale, energy efficient recovery of the water-soluble products from the aqueous broth still presents an engineering challenge. For the first time interlinking of different autotrophic bioprocesses is proposed as a way to overcome the limitations of separate technologies. Furthermore, additional value could be created by coupling different production platforms to upgrade the low-value products typically produced in MES as well as gas fermentations and produce higher value chemicals. The further conversion of acetic acid to caproic and caprylic acid through chain elongation has been proposed as an efficient way to increase the product value of primary fermentation products (Gildemyn, 2016).
Membrane Electrolysis as a Tool to Assist Gas Fermentation: in-situ Product Recovery and pH Control
As the result of charge balancing, an electrochemical reactor with an AEM has the intrinsic ability to extract in-situ the produced acetic acid as the negatively charged acetate ion, into the acidic extraction compartment (termed membrane electrolysis, ME) (Andersen et al., 2014; Gildemyn et al., 2015). Since acetate synthesis from CO2 requires 8 electrons per mole acetate (at 100% current efficiency), and since the electricity-driven extraction of one mole of acetate theoretically requires only one electron, the current use for acetate extraction (limited to a maximum of 12.5%) can only be improved by linking an external H2 source to the MES reactor (increasing the theoretical production rate and thus the membrane availability for extraction). Acetate transport from the cathode to the extraction compartment accounted for 17.5% of the charge balancing through the AEM (Figure 2C), while in a MES cell without external H2 injection, acetate accounted for only 8.1 ± 0.8% of the charge passing through the AEM (Gildemyn et al., 2015). This result clearly indicates that through external H2 supply, a more efficient use of the intrinsic extraction capacity of the reactor can be achieved, but also that the charge efficiency for extraction is limited by the efficiency for production. During stable operation, a charge balancing efficiency for acetate production of 31% was achieved. Calculated on a mass balance, 94% of the produced acetic acid was extracted and 85% of the product was present in the extraction solution (Figure 2B). Diffusion of uncharged acetic acid molecules through the CEM resulted in an acetic acid loss of 9% toward the anode compartment although principally in time this should stabilize. From day 75, the extraction efficiency was 100%, as no product build-up in the catholyte took place. Membrane electrolysis can avoid product build-up in the fermentation broth, and thus allows a batch mode operation without the occurrence of product inhibition or product diversification. Separation of the fermentation product from the broth in a cost- and energy- efficient recovery process is envisaged as a crucial feature for scaling up gas fermentation processes to commercial-scale production plants. Distillation has been the traditional recovery technology for low boiling point fermentation products (Liew et al., 2013), but its energy-intensive nature has led to the development of alternative and potentially less expensive separation techniques (Ezeji et al., 2010), of which membrane electrolysis is of particular interest for charged metabolites that have the tendency to lower broth pH. ME stabilized the pH of the fermentation medium throughout the operation at a pH value of 8.30 ± 0.19 (Figure 2D), while typically a pH drop in the broth of MES and gas fermentation reactors is observed unless a chemical pH control mechanism is applied (Liew et al., 2013; Arends et al., 2017). pH control is an effective strategy to achieve long-term stable acetate production and high product concentrations (Drake et al., 2006; De Tissera et al., 2017), but the addition of large amounts of base is costly and adds salts to the broth (Gildemyn et al., 2017). Base (to prevent product inhibition) and acid dosage (to acidify the product stream) are fully replaced by OH− and H+ production at the cathode and anode of the ME reactor, respectively, highlighting that integration of ME in gas fermentation technology enables operation of a bioproduction reactor without addition of chemicals. This confirms earlier observations that an AEM can stabilize MES operation (Gildemyn et al., 2017). The results suggest that the in-situ extraction of the acetic acid produced in a gas fermenter can enhance productivity through the combined effect of product removal and in-situ pH control. The ME technology would be more efficient as “secondary” microbial electrochemical technology (MET), assisting H2/CO2 fermentation, rather than as a electrosynthesis approach itself. In this way a larger fraction of the intrinsic extraction capacity of the system can be used, and the power input of this cell can be lowered as only part of the reducing equivalents will be supplied by this reactor. As secondary MET, the extraction through ME supports H2/CO2 fermentation by: (i) extracting the produced acetic acid (avoiding product build-up and inhibition); (ii) balancing the pH of the fermentation broth (avoiding caustic addition); and (iii) providing additional reducing equivalents in the form of in-situ electrochemically produced hydrogen (generating high pH2 close to the electrode surface) (Figure 3). Periodic (ON-OFF) extraction could be exploited as a way to make fully use of the capabilities of ME during gas fermentation as it allows to recover the product more efficiently at higher product concentrations in the broth. It could thus be implemented as a recovery approach that intermittently extracts the product when pH stabilization is needed, or when acetic acid accumulates above a set concentration. Fine-tuning of this ON-OFF strategy could results in an optimized energy investment and reduction of water displacement across the membrane. By lowering the current density applied to the three-chambered reactor (and increasing the availability of acetate ions at the membrane surface) the electro-osmotic water transport per kilogram product can be reduced (compared a system where all H2 is produced in-situ). HCO transport over the AEM is of high importance for charge balancing and is a major contributor of the (electro-osmotic) water transport (Gildemyn et al., 2017). Intermittent extraction could result in short periods of very efficient extraction with limited water drag.
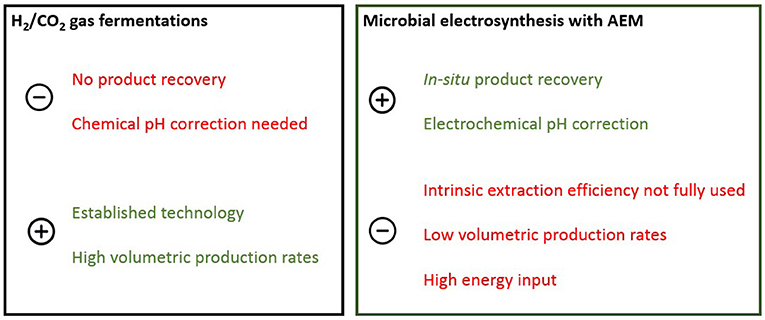
Figure 3. Benefits and drawbacks of gas fermentation and microbial electrosynthesis as CO2-based production platforms. The coupling of both technologies results in a hybrid reactor configuration that combines the product recovery and pH stabilizing ability with the maturity and productivity of gas fermentation.
Membrane Electrolysis Assisted Gas Fermentation as Future Scenario to Reduce Power Input
Increasing productivity at a lower power input is crucial for the economics of both MES and H2/CO2 fermentation processes. The power input for the system presented here required 15 kWh kg−1 for the production of 3.7% acetic acid (only taking into account the electricity input of the electrochemical cells), which is 19–43% lower compared to the energy input in MES-extraction reactors without additional H2 (max. 1.35% acetic acid), but still, undeniably, too high to compete with current production standards (98% acetic acid production via methanol carbonylation at 4 kWh kg−1) (Althaus et al., 2007). The power consumption per kilogram product can be decreased by: (i) increasing the H2 conversion efficiency (getting more product with the same power input), or (ii) reducing the cell voltage of the system (getting the same amount of product at a lower power input). For an industrial process it is critically important to operate a production process at high volumetric production rates, so for MES this means that current densities will need to increase drastically. It is however highly debatable whether H2 can be produced at high energy efficiencies in a MES cell at high current densities when using the conventional (rather unconducive) bacterial growth media as electrolyte, while alkaline or polymer electrolyte membrane (PEM) electrolyzers are optimized for efficient H2 generation. Due to the fact that biological compatibility needs to be guaranteed during electrolyte selection, the low conductivity will make these systems not competitive with abiotic electrolyzers considering only H2 production. During stable reactor operation, cell voltages of 3.91 ± 0.10 V were recorded for the MES cell (5 A m−2), while conventional water electrolyzers are operated under current densities ranging from 1,000 to 3,000 A m−2 and stable cell voltages of 1.7–1.9 V (Zeng and Zhang, 2010). With an energy efficiency ranging from 65 to 82%, current industrial PEM electrolyzers are much more efficient in producing H2 than MES systems currently do (35% energy efficiency at only 5 A m−2). Projecting forward to a fully realized system, the power input of acetic acid production via the ME-assisted gas fermentation pipeline should be calculated based on realistic rather than experimental and non-optimized cell voltages. The economics of the proposed concept is briefly demonstrated for a 10,000 L gas fermentation reactor. At 2,000 A m−2 and 1.8 V, the power cost of water electrolysis is calculated at 4.3 kWh per Nm3 of H2 produced, which corresponds to € 4.8 per kg H2 (at an energy cost of € 0.1 per kWh). Considering a gas fermentation reactor fixing CO2 into acetic acid at a volumetric productivity of 148 g L d−1 (experimentally achieved by Kantzow et al., 2015) and 90% electron recovery, H2 gas should be supplied at a flow rate of 106 Nm3 per hour. If coupled to continuous extraction in a ME unit operated at 1000 A m−2, 42 m2 membrane surface is needed to allow for a stable broth concentration. At 5 V and a charge balancing efficiency by acetate of 65%, 17% of the total H2 load is produced in the ME cell and ME is able to recover 1.48 ton acetic acid per day (extraction rate is set equal to production rate). Based on these assumptions a power input for acetic acid production and extraction of 9.56 kWh per kg acetic acid is calculated, of which 36% can be attributed to the electrochemical extraction. Assuming 40 m2 membrane electrode assembly per cubic meter reactor (Desloover et al., 2015), a ME setup of 1.06 m2 would be sufficient to control fermentation. Potentially the power input can be further decreased if the system is operated with an intermittent rather than continuous extraction (for example 10% of the time ON, 90% OFF), since a higher molecular availability for flux results in a higher charge efficiency for carboxylate extraction and thus a potentially lower current use by the ME system. Assuming a charge balancing efficiency by acetate of 80% when acetate concentration in the broth is 20 g L−1, the power input can be lowered to 8.76 kWh per kg product. This demonstrates that the ME extraction would fit ideally with a high concentration fermentation to obtain an effective and cost-optimized ME step. To lower the energy input per kilogram acetic acid extracted, it is clear that a maximal use of the “expensive” charge for target ion recovery in the ME cell should be targeted. Furthermore, off-gases from industrial processes, such as steel production and reformed biogas, as well as syngas from biomass gasification can serve as substrate gas in the flexible/hybrid MES-gas fermentation approach.
Conclusion
Since its first demonstration, MES has been intensively studied in terms of microbiology (Nevin et al., 2011), electron transfer (Marshall et al., 2012; Jourdin et al., 2016b), electrode materials (Zhang et al., 2013; Jourdin et al., 2016a), CO2 supply (Bajracharya et al., 2016), media modification (Ammam et al., 2016), and product outcome (Ganigué et al., 2015; Vassilev et al., 2018), but engineering of the system has only been studied in terms of product recovery. This study presents an reactor setup that allows operation of MES reactors at higher current densities, thereby increasing the availability of reducing equivalents and, thus, increasing the (theoretical) production rates (provided that the kinetics of the acetogens are not the rate-limiting factor). Coupling direct extraction to an H2/CO2 gas fermentation column allows for recovery of the pure product in an acid and clean extraction liquid while simultaneously stabilizing the pH in the fermentation broth. The external hydrogen injection allows acetic acid production from CO2 at a lower power input and cost. We therefore believe that ME-assisted gas fermentation offers opportunities for the scalability of acetic acid production from CO2.
Author Contributions
All authors listed have made a substantial, direct and intellectual contribution to the work, and approved it for publication.
Funding
KV is supported by FWO Vlaanderen through a Ph.D. scholarship. KR acknowledges support by the European Research Council (StG ELECTROTALK) and Interreg grant EnOP.
Conflict of Interest Statement
The authors declare that the research was conducted in the absence of any commercial or financial relationships that could be construed as a potential conflict of interest.
Acknowledgments
The authors thank Amanda K. Luther for critically reading the manuscript.
References
Althaus, H.-J., Doka, G., Dones, R., Heck, T., Hellweg, S., Hischier, R., et al. (2007). “The Ecoinvent reference is the Swiss center for life cycle inventories,” in Overview and Methodology. Ecoinvent Report No. 1, eds R. Frischknecht and N. Jungbluth (Dübendorf). Available online at: https://www.ecoinvent.org/files/200712_frischknecht_jungbluth_overview_methodology_ecoinvent2.pdf.
Ammam, F., Tremblay, P.-L., Lizak, D. M., and Zhang, T. (2016). Effect of tungstate on acetate and ethanol production by the electrosynthetic bacterium Sporomusa ovata. Biotechnol. Biofuels 9:163. doi: 10.1186/s13068-016-0576-0
Andersen, S. J., Hennebel, T., Gildemyn, S., Coma, M., Desloover, J., Berton, J., et al. (2014). Electrolytic membrane extraction enables production of fine chemicals from biorefinery sidestreams. Environ. Sci. Technol. 48, 7135–7142. doi: 10.1021/es500483w
Arends, J. B. A., Patil, S. A., Roume, H., and Rabaey, K. (2017). Continuous long-term electricity-driven bioproduction of carboxylates and isopropanol from Co2 with a mixed microbial community. J. CO2 Util. 20, 141–149. doi: 10.1016/j.jcou.2017.04.014
Bajracharya, S., Vanbroekhoven, K., Buisman, C. J. N., Pant, D., and Strik, D. P. (2016). Application of gas diffusion biocathode in microbial electrosynthesis from carbon dioxide. Environ. Sci. Pollut.Res. 23, 22292–22308. doi: 10.1007/s11356-016-7196-x
Batlle-Vilanova, P., Ganigué, R., Ramió-Pujol, S., Bañeras, L., Jiménez, G., Hidalgo, M., et al. (2017). Microbial electrosynthesis of butyrate from carbon dioxide: production and extraction. Bioelectrochemistry 117, 57–64. doi: 10.1016/j.bioelechem.2017.06.004
Chen, L., Tremblay, P.-L., Mohanty, S., Xu, K., and Zhang, T. (2016). Electrosynthesis of acetate from CO2 by a highly structured biofilm assembled with reduced graphene oxide-tetraethylene pentamine. J. Mater. Chem. A 4, 8395–8401. doi: 10.1039/C6TA02036D
De Tissera, S., Köpke, M., Simpson, S. D., Humphreys, C., Minton, N. P., and Dürre, P. (2017). Syngas Biorefinery and Syngas Utilization. Berlin; Heidelberg: Springer Berlin Heidelberg.
Desloover, J., Arends, J. B. A., Hennebel, T., and Rabaey, K. (2012). Operational and technical considerations for microbial electrosynthesis. Biochem. Soc. Trans. 40, 1233–1238. doi: 10.1042/BST20120111
Desloover, J., De Vrieze, J., Van de Vijver, M., Mortelmans, J., Rozendal, R., and Rabaey, K. (2015). Electrochemical nutrient recovery enables ammonia toxicity control and biogas desulfurization in anaerobic digestion. Environ. Sci. Technol. 49, 948–955. doi: 10.1021/es504811a
Drake, H. L., Küsel, K., Matthies, C., Dworkin, M., Falkow, S., Rosenberg, E., et al. (Eds). (2006). “Acetogenic prokaryotes,” in The Prokaryotes, Vol. 2, Ecophysiology and Biochemistry, eds M. Dworkin, S. Falkow, E. Rosenberg, K.-H. Schleifer, and E. Stackebrandt (New York, NY: Springer), 354–420.
El-Gammal, M., Abou-Shanab, R., Angelidaki, I., Omar, B., Sveding, P. V., Karakashev, D. B., et al. (2017). High efficient ethanol and vfa production from gas fermentation: effect of acetate, gas and inoculum microbial composition. Biomass Bioenergy 105, 32–40. doi: 10.1016/j.biombioe.2017.06.020
Ezeji, T. C., Li, Y. A. A., Vertès, N., Qureshi, Blaschek, H. P., and Yukawa H (Eds). (2010). “Advanced product recovery technologies,” in Biomass to Biofuels, eds A. A. Vertès, N. Qureshi, H. P. Blaschek, and H. Yukawa (Chichester: John Wiley & Sons Ltd), 331–346.
Ganigué, R., Puig, S., Batlle-Vilanova, P., Balaguer, M., and Colprim, J. (2015). Microbial electrosynthesis of butyrate from carbon dioxide. Chem. Commun. 51, 3235–3238. doi: 10.1039/c4cc10121a
Gildemyn, S. (2016). Technology and Tools for Bioelectrochemical Production of Short- and Medium-Chain Carboxylic Acids from CO2. Ghent: Ghent University; Faculty of Bioscience Engineering.
Gildemyn, S., Verbeeck, K., Jansen, R., and Rabaey, K. (2017). The type of ion selective membrane determines stability and production levels of microbial electrosynthesis. Bioresour. Technol. 224, 358–364. doi: 10.1016/j.biortech.2016.11.088
Gildemyn, S., Verbeeck, K., Slabbinck, R., Andersen, S. J., Prevoteau, A., and Rabaey, K. (2015). Integrated production, extraction, and concentration of acetic acid from CO2 through microbial electrosynthesis. Environ. Sci. Tech. Let. 2, 325–328. doi: 10.1021/acs.estlett.5b00212
Giorno, L., Drioli, E., Strathmann, H., Drioli, E., and Giorno, L. (Eds). (2016). “Water transport in ion-exchange membranes,” in Encyclopedia of Membranes, eds E. Drioli and L. Giorno (Berlin; Heidelberg: Springer), 968–989.
Jourdin, L., Freguia, S., Flexer, V., and Keller, J. (2016a). Bringing high-rate, CO2-based microbial electrosynthesis closer to practical implementation through improved electrode design and operating conditions. Environ. Sci. Technol. 50, 1982–1989. doi: 10.1021/acs.est.5b04431
Jourdin, L., Grieger, T., Monetti, J., Flexer, V., Freguia, S., Lu, Y., et al. (2015). High acetic acid production rate obtained by microbial electrosynthesis from carbon dioxide. Environ. Sci. Technol. 49, 13566–13574. doi: 10.1021/acs.est.5b03821
Jourdin, L., Lu, Y., Flexer, V., Keller, J., and Freguia, S. (2016b). Biologically induced hydrogen production drives high rate/high efficiency microbial electrosynthesis of acetate from carbon dioxide. Chem. Electro. Chem. 3, 581–591. doi: 10.1002/celc.201500530
Kantzow, C., Mayer, A., and Weuster-Botz, D. (2015). Continuous gas fermentation by Acetobacterium woodii in a submerged membrane reactor with full cell retention. J. Biotechnol. 212, 11–18. doi: 10.1016/j.jbiotec.2015.07.020
Klasson, K. T., Ackerson, M. D., Clausen, E. C., and Gaddy, J. L. (1991). Bioreactor design for synthesis gas fermentations. Fuel 70, 605–614. doi: 10.1016/0016-2361(91)90174-9
Lakshminarayanaiah, N. (1969). Electroosmosis in ion-exchange membranes. J. Electrochem. Soc. 116, 338–342. doi: 10.1006/jcis.2000.7063
Liew, F. M., Köpke, M., Simpson Sa, D., and Fang, Z. (ed). (2013). “Gas fermentation for commercial biofuels production,” in Liquid, Gaseous and Solid Biofuels - Conversion Techniques, ed Z. Fang (Rijeka: InTech), 125–173.
Logan, B. E., and Rabaey, K. (2012). Conversion of wastes into bioelectricity and chemicals by using microbial electrochemical technologies Science 337, 686–690. doi: 10.1126/science.1217412
Marshall, C. W., Ross, D. E., Fichot, E. B., Norman, R. S., and May, H. D. (2012). Electrosynthesis of commodity chemicals by an autotrophic microbial community. Appl. Environ. Microbiol. 78, 8412–8420. doi: 10.1128/AEM.02401-12
May, H. D., Evans, P. J., and LaBelle, E. V. (2016). The bioelectrosynthesis of acetate. Curr. Opin. Biotechnol. 42, 225–233. doi: 10.1016/j.copbio.2016.09.004
Nevin, K. P., Hensley, S. A., Franks, A. E., Summers, Z. M., Ou, J., Woodard, T. L., et al. (2011). Electrosynthesis of organic compounds from carbon dioxide is catalyzed by a diversity of acetogenic microorganisms. Appl. Environ. Microbiol. 77, 2882–2886. doi: 10.1128/AEM.02642-10
Nevin, K. P., Woodard, T. L., Franks, A. E., Summers, Z. M., and Lovley, D. R. (2010). Microbial electrosynthesis: feeding microbes electricity to convert carbon dioxide and water to multicarbon extracellular organic compounds. MBio 1:e00103-10. doi: 10.1128/mBio.00103-10
Orgill, J. J., Atiyeh, H. K., Devarapalli, M., Phillips, J. R., Lewis, R. S., and Huhnke, R. L. (2013). A comparison of mass transfer coefficients between trickle-bed, hollow fiber membrane and stirred tank reactors. Bioresour. Technol. 133, 340–346. doi: 10.1016/j.biortech.2013.01.124
Patil, S. A., Arends, J. B., Vanwonterghem, I., Van Meerbergen, J., Guo, K., Tyson, G. W., et al. (2015). Selective enrichment establishes a stable performing community for microbial electrosynthesis of acetate from CO2. Environ. Sci. Technol. 49, 8833–8843. doi: 10.1021/es506149d
Rabaey, K., and Rozendal, R. A. (2010). Microbial electrosynthesis—revisiting the electrical route for microbial production. Nat. Rev. Microbiol. 8, 706–716. doi: 10.1038/nrmicro2422
Song, T. S., Fei, K. Q., Zhang, H. K., Yuan, H., Yang, Y., Ouyang, P. K., et al. (2018). High efficiency microbial electrosynthesis of acetate from carbon dioxide using a novel graphene-nickel foam as cathode. J. Chem. Technol. Biotechnol. 93, 457–466. doi: 10.1002/jctb.5376
Steger, F., Rachbauer, L., Windhagauer, M., Montgomery, L. F. R., and Bochmann, G. (2017). Optimisation of continuous gas fermentation by immobilisation of acetate-producing acetobacterium woodii. Anaerobe 46, 96–103. doi: 10.1016/j.anaerobe.2017.06.010
Vassilev, I., Hernandez, P. A., Batlle-Vilanova, P., Freguia, S., Krömer, J. O., Keller, J., et al. (2018). Microbial electrosynthesis of isobutyric, butyric, caproic acids, and corresponding alcohols from carbon dioxide ACS Sustainable. Chem. Eng. 6, 8485–8493. doi: 10.1021/acssuschemeng.8b00739
Zeng, K., and Zhang, D. (2010). Recent progress in alkaline water electrolysis for hydrogen production and applications. Progr. Energy. Combust. Sci. 36, 307–326. doi: 10.1016/j.pecs.2009.11.002
Keywords: microbial electrosynthesis, anion exchange membrane, bioproduction, CO2 utilization, bioelectrochemical systems
Citation: Verbeeck K, Gildemyn S and Rabaey K (2018) Membrane Electrolysis Assisted Gas Fermentation for Enhanced Acetic Acid Production. Front. Energy Res. 6:88. doi: 10.3389/fenrg.2018.00088
Received: 01 June 2018; Accepted: 15 August 2018;
Published: 04 September 2018.
Edited by:
Sebastià Puig, University of Girona, SpainReviewed by:
Juan Antonio Baeza, Autonomous University of Barcelona, SpainNabin Aryal, Aarhus University, Denmark
Copyright © 2018 Verbeeck, Gildemyn and Rabaey. This is an open-access article distributed under the terms of the Creative Commons Attribution License (CC BY). The use, distribution or reproduction in other forums is permitted, provided the original author(s) and the copyright owner(s) are credited and that the original publication in this journal is cited, in accordance with accepted academic practice. No use, distribution or reproduction is permitted which does not comply with these terms.
*Correspondence: Korneel Rabaey, a29ybmVlbC5yYWJhZXlAdWdlbnQuYmU=
†Present Address: Sylvia Gildemyn, OWS nv, Ghent, Belgium