- 1School of Chemistry, Chemical Engineering and Life Science, Wuhan University of Technology, Wuhan, China
- 2The Novo Nordisk Foundation Center for Biosustainability, Technical University of Denmark, Kongens Lyngby, Denmark
- 3Department of Chemical and Biochemical Engineering, Danish Polymer Centre, Kongens Lyngby, Denmark
Microbial electrosynthesis (MES) is a bioelectrochemical technology developed for the conversion of carbon dioxide and electric energy into multicarbon chemicals of interest. As with other biotechnologies, achieving high production rate is a prerequisite for scaling up. In this study, we report the development of a novel cathode for MES, which was fabricated by coating carbon cloth with conductive poly(3,4-ethylenedioxythiophene):polystyrene sulfonate (PEDOT:PSS) polymer. Sporomusa ovata-driven MES reactors equipped with PEDOT:PSS-carbon cloth cathodes produced 252.5 ± 23.6 mmol d−1 acetate per m2 of electrode over a period of 14 days, which was 9.3 fold higher than the production rate observed with uncoated carbon cloth cathodes. Concomitantly, current density was increased to −3.2 ± 0.8 A m−2, which was 10.7-fold higher than the untreated cathode. The coulombic efficiency with the PEDOT: PSS-carbon cloth cathodes was 78.6 ± 5.6%. Confocal laser scanning microscopy and scanning electron microscopy showed denser bacterial population on the PEDOT:PSS-carbon cloth cathodes. This suggested that PEDOT:PSS is more suitable for colonization by S. ovata during the bioelectrochemical process. The results demonstrated that PEDOT: PSS is a promising cathode material for MES.
Introduction
Reductive bioelectrochemical reactions rely on the transfer of electrons from a cathode to a microbial catalyst for the reduction of a substrate with protons coming from an anodic reaction (Rabaey and Rozendal, 2010; Tremblay and Zhang, 2015). The substrate can be inorganic carbon molecules such as CO2 that will be reduced to multicarbon compounds or CH4 via microbial electrosynthesis (MES) (Cheng et al., 2009; Nevin et al., 2010; Lovley, 2012; Ganigué et al., 2015; Bajracharya et al., 2016, 2017; Tremblay et al., 2017). The MES process requires electric energy that could be generated by a combination of renewable sources such as wind and solar with biological oxidation reactions at the anode (Villano et al., 2010, 2013; Tremblay and Zhang, 2015). In the actual energetic and environmental context, MES could become a flexible approach for the capture of the greenhouse gas CO2 and the storage of intermittent clean energy into compounds of interest such as biofuels and commodity chemicals (Zhang and Tremblay, 2017).
Since the beginning of the decade, multiple strategies have been deployed to increase the productivity of MES reactors. For instance, better microbial catalysts capable of synthesizing a wider range of products have been developed and electrolyte solution have been optimized (Ganigué et al., 2015; Tremblay et al., 2015; Ammam et al., 2016; May et al., 2016; Aryal et al., 2017b; Lehtinen et al., 2017; Krieg et al., 2018). Additionally, several research groups have focused their effort on the development of optimal cathode materials to establish highly efficient electron transfer to microbes (Aryal et al., 2017c). Most commonly-used materials for MES cathodes are carbon base such as graphite, carbon cloth and carbon felt as well as metals including stainless steel and nickel (Nie et al., 2013; Soussan et al., 2013; Tremblay and Zhang, 2015; Cui et al., 2017). More recently, a highly performant MES cathode made of multiwalled carbon nanotube coated onto reticulated vitreous carbon was developed (Jourdin et al., 2014, 2015, 2016). The potential of graphene, a robust, cheap and highly conductive material, was also explored for MES (Aryal et al., 2016, 2017a; Chen et al., 2016; Song et al., 2017).
The ideal cathode material for MES application should have some critical properties such as high conductivity, excellent chemical stability, high mechanical strength, good biocompatibility, high surface area and low cost (Aryal et al., 2017c). The poly(3,4-ethylenedioxythiophene):polystyrene sulfonate (PEDOT:PSS) polymer and its derivatives, which have high conductivity at room temperature, are electrochemically stable, flexible and easy to process as well as relatively inexpensive could be interesting materials for the fabrication of performant MES electrodes (Groenendaal et al., 2000; Kirchmeyer and Reuter, 2005; Song et al., 2014). PEDOT:PSS consists of polycationic PEDOT chains incorporated into a polyanionic PSS matrix (Nardes et al., 2008). Combining PEDOT with PSS enables the formation of aqueous dispersion that can be cast into thin, optically transparent, conductive films. PEDOT:PSS films have been used for many applications including photovoltaics, circuits, field-effect transistors and light-emitting diodes (Chen et al., 2003; Kok et al., 2004; Ko et al., 2007; Yoo and Dodabalapur, 2007). PEDOT:PSS alone or in combination with other materials has also been employed in the fabrication of anodes to improve the performance of microbial fuel cell (MFC), a technology developed for the production of electric energy from the microbial oxidation of organic carbon (Wang et al., 2013; Chou et al., 2014; Antolini, 2015; Jiang et al., 2015; Webb et al., 2015; Pang et al., 2018).
This work reports the fabrication of a cathode made of carbon cloth coated with PEDOT:PSS. The PEDOT:PSS-carbon cloth cathode was tested in MES reactors with the well-characterized electroautotrophic bacteria Sporomusa ovata as microbial catalyst (Nevin et al., 2010). The composite cathode covered with the conductive polymer was shown to be suitable for bacterial colonization resulting in microbial acetate production from CO2 and current density nearly one order of magnitude higher than the unmodified electrode.
Experimental Procedure
Bacterial Strain and Growth Condition
S. ovata DSM 2662 was ordered from the Deutsche Sammlung Mikroorganismen und Zellkulturen (DSMZ) and grown in 311 medium with 40 mM betaine as substrate under a N2:CO2 (80:20) atmosphere (Möller et al., 1984). Bacteria were successively transferred four times in autotrophic condition with H2 as the electron source and CO2 as the carbon source omitting yeast extract, betaine, casitone, sodium sulfide, and resazurin from 311 medium. Cultures from the fourth transfer were used to inoculate the cathode chamber of MES reactors. Cysteine was omitted from 311 medium during MES experiments (Aryal et al., 2017b).
PEDOT:PSS-Carbon Cloth Electrode Fabrication
Carbon cloth (Jiangsu Tongkang Special Activated Carbon Fiber & Fabric Co., Ltd, China) was first pretreated by immersion into 3M HNO3 during 12 h before being extensively washed with ultrapure water (Aryal et al., 2016). The conductivity of carbon cloth is ca. 110 S cm−1 (Stolten et al., 2016). The acid-treated electrode was then dried under nitrogen gas. The dip and dry method described by Hou et al. (2012) was used to coat carbon cloth with PEDOT:PSS. Briefly, the carbon cloth material was dipped into an aqueous solution of commercially available PEDOT:PSS (3.0–4.0% in H2O, high-conductivity grade above 200 S cm−1, Sigma-Aldrich, USA) for 2 h to ensure that the porous carbon substrate was completely soaked. Then, the electrode was taken out of the solution and left at room temperature in air for 15 min to remove excess PEDOT:PSS. The process was repeated a second time before drying the electrode in an oven at 105°C for 5 h. In the last step, the PEDOT:PSS carbon cloth electrode was cleaned continuously for 15 min with running ultrapure water (Hou et al., 2012).
Operation of MES Reactor
Dual-chambered three-electrode system bioreactors were operated at room temperature with S. ovata grown in the cathode chamber as described previously (Nevin et al., 2010; Tremblay et al., 2015). Unmodified or PEDOT:PSS-coated carbon cloth cathodes (20.25 cm2) and the graphite stick anode (36 cm2) were both immerged into 250 ml of 311 medium and were separated by a Nafion 115 ion-exchange membrane (Ion Power, Inc., New Castle, DE, USA). A platinum wire and a marine wire were used as current collector for the cathode and the anode, respectively. Platinum wires were not in contact with the electrolyte solution to avoid unwanted H2 evolution reaction. An Ag/AgCl electrode model ET072 (eDAQ, Denmark) was used as reference electrode and the cathode potential was set at −690 mV versus Standard Hydrogen Electrode (SHE) during MES with a CH Instrument potentiostat (CH Instruments, Inc, USA). The cathode potential was chosen because it works well for S. ovata-driven MES and it enables performance comparison with other electrodes tested under the same MES operational conditions (Tremblay et al., 2015; Ammam et al., 2016; Aryal et al., 2017a,b; Lehtinen et al., 2017). After the inoculation of MES reactors with S. ovata, bacterial population were established with a N2:CO2:H2 (83:10:7) gas mix. After three fresh medium swaps, the gas mix was switched to N2:CO2 (80:20) and acetate production as well as current data started being collected (Aryal et al., 2017a). All MES experiments were done in triplicate.
Cyclic Voltammetry
The cyclic voltammetry (CV) experiments were performed with a Gamry potentiostat (Gamry Instruments, Warminster, PA). Electrochemical data for both MES and CV experiments were analyzed with the EC-Lab v.10.2 software (BioLogic, France) as described previously (Sharma et al., 2013; Lepage et al., 2014) CV of tested electrodes was performed at a scan rate of 1 mV s−1 within a potential range of 0 to −1000mV vs. Ag/AgCl.
High Performance Liquid Chromatography and Gas Chromatography
High Performance Liquid Chromatography (HPLC) was used for the quantification of acetate as previously described (Tremblay et al., 2015). Gas chromatography (GC) was used for the quantification of H2 in the MES reactor headspace. Briefly, gas samples were collected in N2-flushed serum bottles and H2 was measured with a Trace 1300 gas chromatograph (ThermoFisher Scientific, Denmark). Argon was the carrier gas with a HP-PLOT Molesieve column (Agilent). The GC oven temperature was 130°C. H2 was detected with a thermal conductivity detector (TCD).
Microscopy
Confocal Laser Scanning Microscopy (CLSM) images and Scanning Electron Microscopy (SEM) images were taken after 14 days of MES operation to study bacterial population at the surface of the different cathodes. For CLSM, S. ovata cells present on cathodes were stained with the LIVE/DEAD® BacLight™ Bacterial Viability Kit (ThermoFisher Scientific). Images were taken with a Zeiss LSM 5 Pascal microscope and analyzed with the ZEN imaging software (Zeiss, Germany) (Aryal et al., 2016). For SEM images, cathodes from MES reactors were fixed with 0.1M buffer solution at pH 7.0 containing 2.5% glutaraldehyde for 5 h at room temperature. Fixed samples were washed with the same buffer without glutaraldehyde and then immersed successively in acetonitrile and ethanol before being dried under nitrogen as described previously (Zhang et al., 2013). SEM images were taken with a Quanta 200 FEG scanning electron microscope (FEI) at an accelerating voltage of 10 V under high vacuum condition (Aryal et al., 2016).
Analytical Methods
Specific surface area of carbon cloth and PEDOT:PSS-carbon cloth cathodes was measured with the Brunauer–Emmett–Teller (BET) method as previously described (Poreddy et al., 2015). X-ray photoelectron spectroscopy (XPS) was performed with an ESCALAB 250Xi XPS system (ThermoFisher Scientific) with an aluminum K-alpha (1486.6 eV) source. X-ray spot area measurement was set at 500 μM.
Results and Discussion
The PEDOT:PSS-Carbon Cloth Electrode
The novel PEDOT:PSS-carbon cloth electrode tested here for MES activities was fabricated via the dip dry method (Hou et al., 2012). SEM image showed that the coating of the carbon cloth cathode electrode with the PEDOT:PSS polymer was successful (Figure 1). After treatment, a PEDOT:PSS layer significantly increasing surface roughness could be observed on the carbon fibers comprised in the carbon cloth electrode (Figure 1B inset). This layer was not present on the untreated electrode (Figure 1A inset). XPS also confirmed the presence of PEDOT:PSS at the surface of carbon cloth. Characteristic S(2p) peaks were observed corresponding to the sulfur signal from PEDOT at a binding energy of 164.1 and 163.1 eV and to the sulfur signal from PSS at 167.2 eV (Figure 2A) (Crispin et al., 2006). Electrochemical performance of the PEDOT:PSS-modified carbon cloth electrode was investigated by CV with sterile 311 medium as the electrolyte (Figure 2B). Interestingly, the reductive current response with the sterile medium was improved from −0.988 to −5.506 mA after coating with PEDOT:PSS. Furthermore, PEDOT:PSS increased the specific surface area of the electrode from 0.5 to 1.1 m2 g−1. This is consistent with results published before by Guzman et al. also showing a doubled specific surface area when carbon cloth material is coated with PEDOT (Guzman et al., 2017).
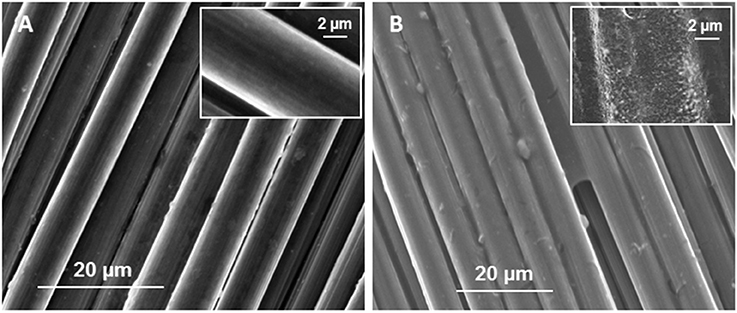
Figure 1. SEM images of (A) sterile carbon cloth and sterile (B) carbon cloth coated with PEDOT:PSS.
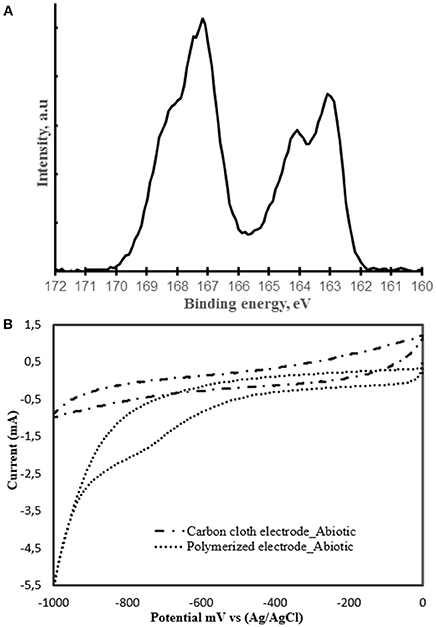
Figure 2. (A) S(2p) XPS spectrum of PEDOT:PSS-carbon cloth electrode. (B) Cyclic voltammograms of carbon cloth and PEDOT:PSS-carbon cloth electrode (polymerized) in sterile 311 medium. Scan rate of 1 mV s−1.
MES With PEDOT:PSS-Carbon Cloth Cathode
MES systems equipped with a PEDOT:PSS-carbon cloth cathode poised at −690 mV vs SHE had an acetate production rate of 252.3 ± 17.7 mmol m−2 d−1 (n = 3) over a period of 14 days with a current density of −3.2 ± 0.8 A m−2 and a coulombic efficiency of 78.6 ± 5.6% (Figure 3,Table 1). Acetate production rate was 9.2-fold faster and current density was 10.7-fold higher with the modified electrode compared to the untreated electrode. No H2 was detected in the headspace of MES reactors equipped with the PEDOT:PSS-carbon cloth cathode. In combination with the good coulombic efficiency, this suggested that most electrons coming from the cathode were harvested by the microbial catalyst.
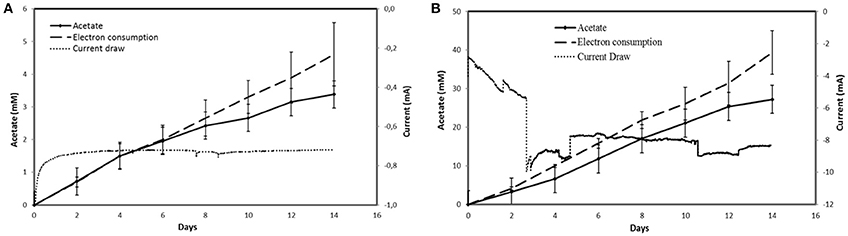
Figure 3. Acetate production, electron consumption and current draw with (A) unmodified carbon cloth cathode and (B) PEDOT:PSS-carbon cloth cathode. Electron consumption curves correspond to the acetate concentration in mM if all the electrons transferred were converted to acetate. Acetate production curves in mM correspond to the real progression of acetate concentration in the MES reactor detected by HPLC. Results shown for acetate production and electron consumption are in triplicate. Current draw curves are from a representative example of three replicate MES reactors.
To understand further the impact of PEDOT:PSS on MES productivity, SEM and CLSM images were taken after 14 days of reactor operation. The bacterial population found on the modified cathode was significantly denser than on the untreated electrode with the presence of numerous characteristic rod-shaped S. ovata cells (Figure 4). The higher number of bacteria attached to the PEDOT:PSS-carbon cloth cathode electrode suggested that this material is more suitable for colonization by electroautotrophic bacteria than carbon cloth. As with other MES systems, this increase in the bacterial population density may correspond to a higher number of electric contacts between microbial catalysts and the modified cathode, which is probably one of the main reasons for the observed higher rates of current consumption and acetate production (Chen et al., 2016; Aryal et al., 2017a).
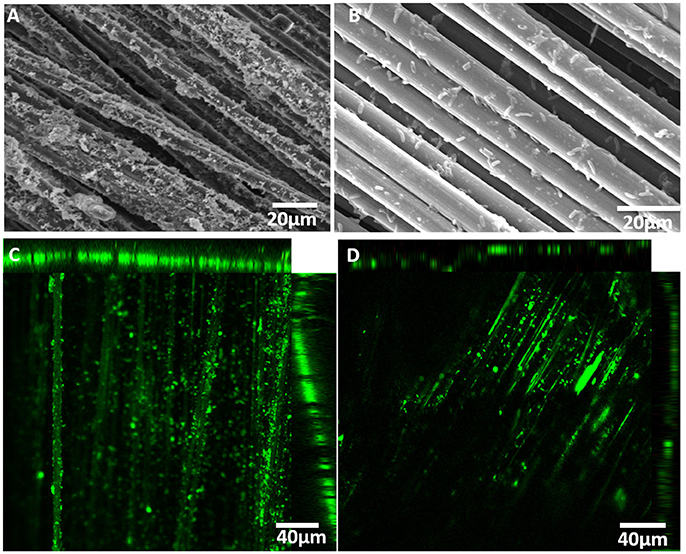
Figure 4. SEM and CLSM images of (A, C) a PEDOT:PSS-carbon cloth and (B, D) of a carbon cloth cathode in S. ovata-driven MES reactors.
Until now, the three-dimensional graphene carbon felt electrode (3D-G-CF) was the best reported cathode material for MES with wild type S. ovata as microbial catalyst and a cathode potential set at −690 mV versus SHE. The PEDOT:PSS-carbon cloth cathode performance was similar to the 3D-G-CF, which had an acetate production rate of 231.4 ± 7.4 mmol d−1 per m2 of electrode (Aryal et al., 2016). The PEDOT:PSS-carbon cloth electrode is more performant than other carbon-based cathodes tested at the same potential with S. ovata wild type including carbon cloth, carbon paper, carbon felt and reduced graphene oxide (RGO) paper (Aryal et al., 2017a). Within this group, RGO paper cathode achieved the highest acetate production rate of 168.5 ± 22.4 mmol d−1 per m2 of electrode, which was ca. 1.37 times lower than with PEDOT:PSS-carbon cloth electrode. These comparisons showed the potential of PEDOT:PSS as an electrode material for MES.
Electrochemical Performance of the PEDOT:PSS-Carbon Cloth Biocathode
The PEDOT:PSS-modified biocathode was further characterized via CV (Figure 5). The cathodic current of the PEDOT:PSS-carbon cloth biocathode was significantly improved compared to the unmodified biocathode, showing that the PEDOT:PSS modification increased bioelectrocatalytic activity. No reversible redox peaks were detected on the current–potential curves before and after colonization by S. ovata during MES (Figures 2, 5). This suggested that no electroactive species were present in the medium or excreted by S. ovata, acting as electron shuttles between the bacterial cells and the cathodes.
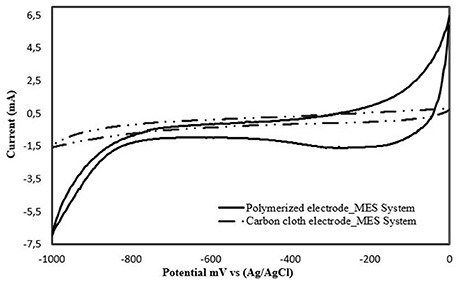
Figure 5. Cyclic voltammograms obtained at a carbon cloth and a PEDOT:PSS-carbon cloth electrode (polymerized) in S. ovata-driven MES reactors. Scan rate of 1 mV s−1.
Conclusion
The results presented here demonstrate that PEDOT:PSS is a suitable conductive polymer for the development of performant MES biocathode. The results of acetate production as well as current consumption were improved significantly in the presence of PEDOT:PSS. S. ovata population at the surface of PEDOT:PSS was more substantial when compared with the uncoated carbon cloth electrode, which demonstrated that PEDOT:PSS is biocompatible and suitable for the development of robust bioelectrocatalyst. Beside its good conductivity, PEDOT:PSS has other interesting properties such as low cost, good mechanical flexibility and high environmental stability that could be harnessed in the future for the fabrication of more performant and robust MES reactor (Groenendaal et al., 2000; Wang, 2009).
Author Contributions
NA, TZ, and AD designed the study. MX and NA fabricated the cathodes and did the microscopy characterization. NA performed the bioelectrochemical experiments and did the analytical chemistry. MX did the XPS analysis. NA, TZ, and P-LT analyzed the data and wrote the manuscript.
Conflict of Interest Statement
The authors declare that the research was conducted in the absence of any commercial or financial relationships that could be construed as a potential conflict of interest.
Acknowledgments
TZ acknowledges the financial support of the Chinese Thousand Talents Plan Program and the Novo Nordisk Foundation.
References
Ammam, F., Tremblay, P. -L., Lizak, D. M., and Zhang, T. (2016). Effect of tungstate on acetate and ethanol production by the electrosynthetic bacterium Sporomusa ovata. Biotechnol. Biofuels 9:163. doi: 10.1186/s13068-016-0576-0
Antolini, E. (2015). Composite mateials for polymer electrolyte membrane microbial fuel cells. Biosens. Bioelectron. 69, 54–70. doi: 10.1016/j.bios.2015.02.013
Aryal, N., Ammam, F., Patil, S. A., and Pant, D. (2017c). An overview of cathode materials for microbial electrosynthesis of chemicals from carbon dioxide. Green Chem. 19, 5748–5760. doi: 10.1039/C7GC01801K
Aryal, N., Halder, A., Tremblay, P. -L., Chi, Q., and Zhang, T. (2016). Enhanced microbial electrosynthesis with three-dimensional graphene functionalized cathodes fabricated via solvothermal synthesis. Electrochim. Acta 217, 117–122. doi: 10.1016/j.electacta.2016.09.063
Aryal, N., Halder, A., Zhang, M., Whelan, P. R., Tremblay, P. -L., Chi, Q., et al. (2017a). Freestanding and flexible graphene papers as bioelectrochemical cathode for selective and efficient CO2 conversion. Sci. Rep. 7:9107. doi: 10.1038/s41598-017-09841-7
Aryal, N., Tremblay, P. -L., Lizak, D. M., and Zhang, T. (2017b). Performance of different Sporomusa species for the microbial electrosynthesis of acetate from carbon dioxide. Bioresour. Technol. 233, 184–190. doi: 10.1016/j.biortech.2017.02.128
Bajracharya, S., Vanbroekhoven, K., Buisman, C. J. N., Pant, D., and Strik, D. P. (2016). Application of gas diffusion biocathode in microbial electrosynthesis from carbon dioxide. Enviro. Sci. Pollut. Res. 23, 22292–22308. doi: 10.1007/s11356-016-7196-x
Bajracharya, S., Yuliasni, R., Vanbroekhoven, K., Buisman, C. J. N., Strik, D. P., and Pant, D. (2017). Long-term operation of microbial electrosynthesis cell reducing CO2 to multi-carbon chemicals with a mixed culture avoiding methanogenesis. Bioelectrochemistry 113, 26–34. doi: 10.1016/j.bioelechem.2016.09.001
Chen, B., Cui, T., Liu, Y., and Varahramyan, K. (2003). All-polymer RC filter circuits fabricated with inkjet printing technology. Solid State Electron. 47, 841–847. doi: 10.1016/S0038-1101(02)00443-4
Chen, L., Tremblay, P. -L., Mohanty, S., Xu, K., and Zhang, T. (2016). Electrosynthesis of acetate from CO2 by a highly structured biofilm assembled with reduced graphene oxide–tetraethylene pentamine. J. Mater. Chem. A 4, 8395–8401. doi: 10.1039/C6TA02036D
Cheng, S., Xing, D., Call, D. F., and Logan, B. E. (2009). Direct biological conversion of electrical current into methane by electromethanogenesis. Environ. Sci. Technol. 43, 3953–3958. doi: 10.1021/es803531g
Chou, H. T., Lee, H. J., Lee, C. Y., Tai, N. H., and Chang, H. Y. (2014). Highly durable anodes of microbial fuel cells using a reduced graphene oxide/carbon nanotube-coated scaffold. Bioresour. Technol. 169, 532–536. doi: 10.1016/j.biortech.2014.07.027
Crispin, X., Jakobsson, F. L. E., Crispin, A., Grim, P. C. M., Andersson, P., Volodin, A., et al. (2006). The origin of the high conductivity of poly(3,4-ethylenedioxythiophene)–poly(styrenesulfonate) (PEDOT–PSS) plastic electrodes. Chem. Mater. 18, 4354–4360. doi: 10.1021/cm061032+
Cui, M., Nie, H., Zhang, T., Lovley, D., and Russell, T. P. (2017). Three-dimensional hierarchical metal oxide-carbon electrode material for high efficient microbial electrosynthesis. Sustain. Energ. Fuels 1, 1171–1176. doi: 10.1039/C7SE00073A
Ganigué, R., Puig, S., Batlle-Vilanova, P., Dolors Balaguer, M., and Colprim, J. (2015). Microbial electrosynthesis of butyrate from carbon dioxide. Chem. Comm. 51, 3235–3238. doi: 10.1039/C4CC10121A
Groenendaal, L., Jonas, F., Freitag, D., Pielartzik, H., and Reynolds, J. R. (2000). Poly(3,4-ethylenedioxythiophene) and its derivatives: past, present, and future. Adv. Mater. Weinheim. 12, 481–494. doi: 10.1002/(SICI)1521-4095(200004)12:7<481::AID-ADMA481>3.0.CO;2-C
Guzman, J. J. L., Pehlivaner, M. O., Frey, M. W., and Angenent, L. T. (2017). Performance of electro-spun carbon nanofiber electrodes with conductive poly(3,4-ethylenedioxythiophene) coatings in bioelectrochemical systems. J. Power Sources 356, 331–337. doi: 10.1016/j.jpowsour.2017.03.133
Hou, S., Cai, X., Wu, H., Lv, Z., Wang, D., Fu, Y., et al. (2012). Flexible, metal-free composite counter electrodes for efficient fiber-shaped dye-sensitized solar cells. J. Power Sources 215, 164–169. doi: 10.1016/j.jpowsour.2012.05.002
Jiang, H., Halverson, J. L., and Dong, L. (2015). A miniature microbial fuel cell with conducting nanofibers-based 3D porous biofilm Manuscript version. J. Micromech. Microeng. 25:125017. doi: 10.1088/0960-1317/25/12/125017
Jourdin, L., Freguia, S., Donose, B. C., Chen, J., Wallace, G. G., Keller, J., et al. (2014). A novel carbon nanotube modified scaffold as an efficient biocathode material for improved microbial electrosynthesis. J. Mater Chem. A 2:13093. doi: 10.1039/C4TA03101F
Jourdin, L., Freguia, S., Flexer, V., and Keller, J. (2016). Bringing High-Rate, CO2-Based microbial electrosynthesis closer to practical implementation through improved electrode design and operating conditions. Enviro. Sci. Technol. 50, 1982–1989. doi: 10.1021/acs.est.5b04431
Jourdin, L., Grieger, T., Monetti, J., Flexer, V., Freguia, S., Lu, Y., et al. (2015). High acetic acid production rate obtained by microbial electrosynthesis from carbon dioxide. Environ. Sci.Technol. 49, 13566–13574. doi: 10.1021/acs.est.5b03821
Kirchmeyer, S., and Reuter, K. (2005). Scientific importance, properties and growing applications of poly(3,4-ethylenedioxythiophene). J. Mater. Chem. 15, 2077–2088. doi: 10.1039/b417803n
Ko, C., Lin, Y., Chen, F., Chu, C., and Ko, C. (2007). Modified buffer layers for polymer photovoltaic devices. Appl. Phys. Lett. 90:063509. doi: 10.1063/1.2437703
Kok, M. M., De Buechel, M., Vulto, S. I. E., Weijer, P., Van De Meulenkamp, E. A., Winter, S. H. P. M., et al. (2004). Modification of PEDOT:PSS as hole injection layer in polymer LEDs. Physica Status Solidi A 201, 1342–1359. doi: 10.1002/pssa.200404338
Krieg, T., Sydow, A., Faust, S., Huth, I., and Holtmann, D. (2018). CO2 to terpenes: autotrophic and electroautotrophic a-humulene production with Cupriavidus necator. Angew. Chem. Int. Ed Engl. 57, 1879–1882. doi: 10.1002/anie.201711302
Lehtinen, T., Efimova, E., Tremblay, P., Santala, S., Zhang, T., and Santala, V. (2017). Bioresource technology production of long chain alkyl esters from carbon dioxide and electricity by a two-stage bacterial process. Bioresour. Technol. 243, 30–36. doi: 10.1016/j.biortech.2017.06.073
Lepage, G., Perrier, G., Merlin, G., Aryal, N., and Dominguez-Benetton, X. (2014). Multifactorial evaluation of the electrochemical response of a microbial fuel cell. RSC Adv. 4, 23815–23825. doi: 10.1039/C4RA03879G
Lovley, D. R. (2012). Electromicrobiology. Annu. Rev. Microbiol. 66, 391–409. doi: 10.1146/annurev-micro-092611-150104
May, H. D., Evans, P. J., and LaBelle, E. V. (2016). The bioelectrosynthesis of acetate. Curr. Opin. Biotechnol. 42, 225–233. doi: 10.1016/j.copbio.2016.09.004
Möller, B., Rolf, O., Howard, B. H., Gottsehalk, G., and Hippe, H. (1984). Sporomusa, a new genus of gram-negative anaerobic bacteria including Sporomusa sphaeroides spec. nov. and Sporomusa ovata spec. nov. Arch Mierobiol. 139, 388–396. doi: 10.1007/BF00408385
Nardes, A. M., Kemerink, M., de Kok, M. M., Vinken, E., Maturova, K., and Janssen, R. A. J. (2008). Conductivity, work function, and environmental stability of PEDOT:PSS thin films treated with sorbitol. Org. Electron. 9, 727–734. doi: 10.1016/j.orgel.2008.05.006
Nevin, K. P., Woodard, T. L., Franks, A. E., Summers, Z. M., and Lovley, D. R. (2010). Microbial electrosynthesis: feeding microbes electricity to convert carbon dioxide and water to multicarbon extracellular organic compounds. MBio 1, e00103–e00110. doi: 10.1128/mBio.00103-10
Nie, H., Zhang, T., Cui, M., Lu, H., Lovley, D. R., and Russell, T. P. (2013). Improved cathode for high efficient microbial-catalyzed reduction in microbial electrosynthesis cells. Phys. Chem. Chem. Phys. 15, 14290–14294. doi: 10.1039/c3cp52697f
Pang, S., Gao, Y., and Choi, S. (2018). Flexible and stretchable microbial fuel cells with modified conductive and hydrophilic textile. Biosens. Bioelectron. 100, 504–511. doi: 10.1016/j.bios.2017.09.044
Poreddy, R., Engelbrekt, C., and Riisager, A. (2015). Copper oxide as efficient catalyst for oxidative dehydrogenation of alcohols with air. Catal. Sci. Technol. 5, 2467–2477.
Rabaey, K., and Rozendal, R. A. (2010). Microbial electrosynthesis - revisiting the electrical route for microbial production. Nat. Rev. Microbiol. 8, 706–716. doi: 10.1038/nrmicro2422
Sharma, M., Aryal, N., Sarma, P. M., Vanbroekhoven, K., Lal, B., Benetton, X. D., et al. (2013). Bioelectrocatalyzed reduction of acetic and butyric acids via direct electron transfer using a mixed culture of sulfate-reducers drives electrosynthesis of alcohols and acetone. Chem. Comm. 49, 6495–6497. doi: 10.1039/c3cc42570c
Song, D., Li, M., Jiang, Y., Chen, Z., Bai, F., Li, Y., et al. (2014). Facile fabrication of MoS2 / PEDOT–PSS composites as low-cost and efficient counter electrodes for dye-sensitized solar cells. J. Photochem. Photobiol. A Chem. 279, 47–51. doi: 10.1016/j.jphotochem.2014.01.009
Song, T., Fei, K., Zhang, H., and Yuan, H. (2017). High efficiency microbial electrosynthesis of acetate from carbon dioxide using a novel graphene–nickel foam as cathode. J. Chem. Technol. Biotechnol. 93, 457–466. doi: 10.1002/jctb.5376
Soussan, L., Riess, J., Erable, B., Delia, M. L., and Bergel, A. (2013). Electrochemical reduction of CO2 catalysed by geobacter sulfurreducens grown on polarized stainless steel cathodes. Electrochem. commun. 28, 27–30. doi: 10.1016/j.elecom.2012.11.033
Stolten, D., Samsun, R. C., and Garland, N. (2016). Fuel Cells: Data, Facts, and Figures. New york, NY: Wiley.
Tremblay, P. L., and Zhang, T. (2015). Electrifying microbes for the production of chemicals. Front. Microbiol. 6:201. doi: 10.3389/fmicb.2015.00201
Tremblay, P. L., Angenent, L. T., and Zhang, T. (2017). Extracellular electron uptake: among autotrophs and mediated by surfaces. Trends Biotechnol. 35, 360–371. doi: 10.1016/j.tibtech.2016.10.004
Tremblay, P. L., Höglund, D., Koza, A., Bonde, I., and Zhang, T. (2015). Adaptation of the autotrophic acetogen Sporomusa ovata to methanol accelerates the conversion of CO2 to organic products. Sci. Rep. 5:16168. doi: 10.1038/srep16168
Villano, M., Aulenta, F., Ciucci, C., Ferri, T., Giuliano, A., and Majone, M. (2010). Bioelectrochemical reduction of CO2 to CH4 via direct and indirect extracellular electron transfer by a hydrogenophilic methanogenic culture. Bioresour. Technol. 101, 3085–3090. doi: 10.1016/j.biortech.2009.12.077
Villano, M., Scardala, S., Aulenta, F., and Majone, M. (2013). Bioresource Technology Carbon and nitrogen removal and enhanced methane production in a microbial electrolysis cell. Bioresour. Technol. 130, 366–371. doi: 10.1016/j.biortech.2012.11.080
Wang, Y. (2009). Research progress on a novel conductive Research progress on a novel conductive polymer — poly (3,4- ethylenedioxythiophene) (PEDOT). J. Phys. Conf. Ser. 152:012023. doi: 10.1088/1742-6596/152/1/012023
Wang, Y., Zhao, C. E., Sun, D., Zhang, J. R., and Zhu, J. J. (2013). A graphene/poly(3,4-ethylenedioxythiophene) hybrid as an anode for high-performance microbial fuel cells. Chempluschem 78, 823–829. doi: 10.1002/cplu.201300102
Webb, H. K., Notley, S. M., and Evans, D. R. (2015). Observation of electron transfer between bacteria and high conductivity graphene–PEDOT composites. RSC Adv. 5, 45642–45645. doi: 10.1039/C5RA08720A
Yoo, B., and Dodabalapur, A. (2007). Germanium nanowire transistors with ethylene glycol treated poly (3,4- ethylenedioxythiophene):poly(styrene sulfonate) contacts. Appl. Phys. Lett. 90:072106. doi: 10.1063/1.2535710
Zhang, T., and Tremblay, P. L. (2017). Hybrid photosynthesis - powering biocatalysts with solar energy captured by inorganic devices. Biotechnol. Biofuels 10:249. doi: 10.1186/s13068-017-0943-5
Keywords: microbial electrosynthesis, carbon dioxide, PEDOT:PSS, acetogens, acetate
Citation: Aryal N, Tremblay P-L, Xu M, Daugaard AE and Zhang T (2018) Highly Conductive Poly(3,4-ethylenedioxythiophene) Polystyrene Sulfonate Polymer Coated Cathode for the Microbial Electrosynthesis of Acetate From Carbon Dioxide. Front. Energy Res. 6:72. doi: 10.3389/fenrg.2018.00072
Received: 14 April 2018; Accepted: 03 July 2018;
Published: 20 July 2018.
Edited by:
Andrea Schievano, Università degli Studi di Milano, ItalyReviewed by:
Pascal E. Saikaly, King Abdullah University of Science and Technology, Saudi ArabiaMatteo Grattieri, University of Utah, United States
Copyright © 2018 Aryal, Tremblay, Xu, Daugaard and Zhang. This is an open-access article distributed under the terms of the Creative Commons Attribution License (CC BY). The use, distribution or reproduction in other forums is permitted, provided the original author(s) and the copyright owner(s) are credited and that the original publication in this journal is cited, in accordance with accepted academic practice. No use, distribution or reproduction is permitted which does not comply with these terms.
*Correspondence: Tian Zhang, tzhang@whut.edu.cn
†Present Address: Nabin Aryal, Biological and Chemical Engineering, Anaerobic Digestion Technologies, Aarhus University, Aarhus N, Denmark