- 1Chemical and Environmental Laboratories (CEL), School of Applied Chemical and Environmental Sciences, Sheridan Institute of Technology, Brampton, ON, Canada
- 2Innovation Concepts B.V., Twello, Netherlands
- 3School of Engineering, University of Guelph, Guelph, ON, Canada
To overcome the challenges of mineral CO2 sequestration, Innovation Concepts B.V. is developing a unique proprietary gravity pressure vessel (GPV) reactor technology and has focussed on generating reaction products of high economic value. The GPV provides intense process conditions through hydrostatic pressurization and heat exchange integration that harvests exothermic reaction energy, thereby reducing energy demand of conventional reactor designs, in addition to offering other benefits. In this paper, a perspective on the status of this technology and outlook for the future is provided. To date, laboratory-scale tests of the envisioned process have been performed in a tubular “rocking autoclave” reactor. The mineral of choice has been olivine [~Mg1.6Fe2+0.4(SiO4) + ppm Ni/Cr], although asbestos, steel slags, and oil shale residues are also under investigation. The effect of several process parameters on reaction extent and product properties has been tested: CO2 pressure, temperature, residence time, additives (buffers, lixiviants, chelators, oxidizers), solids loading, and mixing rate. The products (carbonates, amorphous silica, and chromite) have been physically separated (based on size, density, and magnetic properties), characterized (for chemistry, mineralogy, and morphology), and tested in intended applications (as pozzolanic carbon-negative building material). Economically, it is found that product value is the main driver for mineral carbonation, rather than, or in addition to, the sequestered CO2. The approach of using a GPV and focusing on valuable reaction products could thus make CO2 mineralization a feasible and sustainable industrial process.
Motivation and Technology
CO2 Sequestration
Cumulative CO2 emissions have caused and continue to cause multiple environmental effects, but given the immense quantity of fossil fuels still economical to exploit, a drastic reduction is not likely to happen in the near future (Meinshausen et al., 2011). The emitted CO2 partly remains in the atmosphere as a gas, and partly is taken up by one of nature’s carbon cycles. There are three carbon cycles: the short organic cycle (the storage of CO2 in biomass), the long organic cycle (the storage of CO2 in fossil fuels and other organic forms), and the long inorganic cycle (the storage of CO2 in minerals, e.g., lime and dolomite) (Dunsmore, 1992). Carbon capture by mineral carbonates has the highest capacity and storage stability; hence, it is viewed as a potential route to permanent CO2 sequestration at large (industrial) scales (Lackner, 2003; Broecker, 2008).
The research of Innovation Concepts B.V. focuses on the inorganic carbon cycle, namely mineral carbonation. However, the goal is not simply CO2 sequestration but also to use CO2 as a feedstock to produce valuable materials. Plenty of materials are suitable as input minerals, such as wollastonite (calcium silicate) and olivine (magnesium silicate). In addition, residual products from mines and industrial processes could also be used, for example, asbestos, oilshale residue, nickel mining tailings, red mud, and steelmaking slags (Power et al., 2013; Sanna et al., 2014). The main challenge is that the geochemical reaction to capture CO2 is rather slow, making it unsuitable for large-scale industrial implementation, unless process intensification can be engineered. In addition, economic challenges exist, as associated operating costs can be high (Hitch and Dipple, 2012), while CO2 credit prices remain relatively low (Edenhofer, 2014).
The main research objective of this work is therefore to accelerate the kinetics of the reaction, in an economic manner. To this end, a novel carbonation process is developed, the “CO2 Energy Reactor™,” which makes use of a gravity pressure vessel (GPV). The acceleration takes place at high temperatures and pressures, by the use of additives and by optimization of other parameters. As for economics, the aim is to generate valuable products (minerals and reaction heat energy) that off-set processing costs, independent of CO2 credit prices. In this perspective paper, a novel laboratory-scale reactor that simulates the full-scale GPV is introduced (the “rocking autoclave”), and it is shown that reaction products produced in the unique mixing conditions of this reactor can be physically separated, to enable their further valorization into specialty applications.
Integrated Mineral Carbonation Reactor
The GPV (illustrated in Figure 1A) is a plug-flow autoclave that consists of three concentric tubes: the innermost is termed “downcomer,” the middle one is termed “upcomer,” and the outermost is termed “jacket.” The reactor is installed within a well drilled deep into the ground, with lengths that can reach up to 1200 m (2400 m two-way), resulting in hydrostatic-built pressures that can reach 120 bar. The reactor feed is a slurry of water and milled mineral. As the slurry flows down the downcomer tube, it is preheated by the counter-flowing hot slurry in upcomer. Once the stream temperature of the downcomer is sufficiently high, carbonation commences, generating exothermic heat that continues to drive the reaction. Carbon dioxide is injected at one or multiple levels along the downcomer. The reacting slurry flows back up and out, giving up its heat on the way to preheat new feed. To achieve a residence time of 90 min, nominal volumetric slurry flow rate would be ~57 m3/h. The jacket flow can be used during the reactor start-up to provide initial heat to ignite the reaction, or to recover excess heat during reactor steady-state operation. This recovered heat could be utilized in a preceding CO2 capture stage, for example, for solvent regeneration (Chakma, 1997).
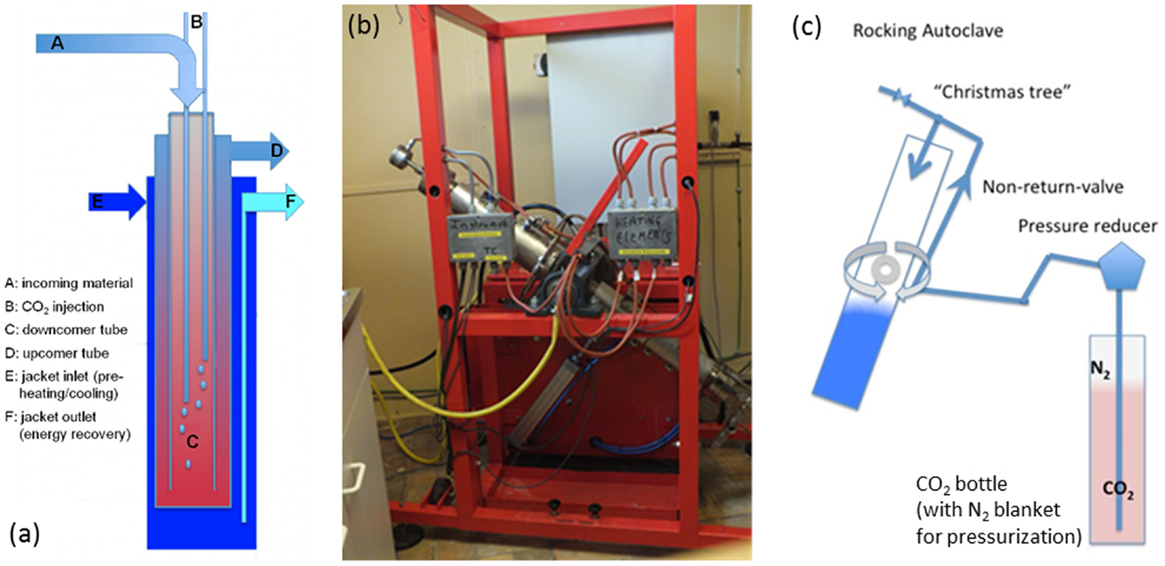
Figure 1. (A) Conceptual layout of “CO2 Energy Reactor™” (not to scale) (Santos et al., 2013, licensed under CC BY-NC-ND 3.0); (B) lab-scale “rocking autoclave” at ~45° rotation; (C) schematic of “rocking autoclave.”
The integrated configuration of the GPV leads to a few unique advantages: a built-in heat exchanger (U-tube in-tube design); pressure is built up by hydrostatic forces; mixing is turbulent and abrasive (autogenously) with no moving parts; small footprint; underground installation. The main design limitations of the GPV are residence time maximum of ~90 min (depending on depth and diameter), maximum pressure ~150 bar (depending on the gas/solid/water volume ratios in combination with the depth), maximum temperature ~300°C (due to mechanical considerations), and continuous liquid phase required (limits gas phase fraction).
The first patent of the GPV technique was granted for wet-air oxidation of sewage sludge (McGrew, 1981). As an extension of that design, and based on lessons learned from 12 years of operational experience in Apeldoorn (the Netherlands), Innovation Concepts B.V. patented the application of GPV to mineral carbonation (Rijnsburger and Knops, 2011).
Lab-Scale Investigation
Rocking Autoclave
Given the hydrostatic pressurization of a GVP and its dependence on length to achieve the desired residence time, it is not possible to faithfully scale it down. In order to test the mineralization process, a “rocking autoclave” (shown in Figure 1B and illustrated in Figure 1C) was built as a lab-scale representation of the foreseen full-scale process.
The rocking autoclave consists of a 1.8-l tube, which can be filled with the mineral, water, and additives, then sealed, pressurized with CO2, and heated. The tube is positioned vertically and periodically (within 1 s) rotated 180° in order to simulate the slurry flow in the GPV, and to promote mixing between the three phases (solids, aqueous solution, and supercritical CO2). Because there is no other mixing regime imposed (such as the use of high-speed impellers in CSTRs), the mixing is rather gentle. This reduces pressure fluctuations within the reactor, which occur around impeller blades (Yoshikawa et al., 2010), and may impact precipitated solids properties; this is a subject deserving more research.
The temperature profile within the rocking autoclave is controlled by electric heat supply and cooling water supply; in this way, the heating and cooling profiles of the GPV [modeled by Santos et al. (2013)] can be simulated. While rocking and under heat, CO2 (92% purity, balance N2) is added to carry out the carbonation; the nitrogen content of the gas bottle enables pressurizing the reactor beyond the vapor pressure of CO2 at ambient temperature without the need for a high-pressure CO2 pump.
Materials
Lab-scale investigation with the rocking autoclave has mainly focused on carbonation of olivine (the choice of mineral is detailed in Section S1 in Supplementary Material). Olivine was supplied by Sibelco and produced at their Norwegian mine located at Åheim (west coast of Norway, south of Ålesund). The material supplied (GL30) is a standard product of particle size 0.063–0.125 mm and consistent properties, typically used for sandblasting. The mineralogical composition of the olivine, determined by quantitative X-ray diffraction (QXRD), was forsterite 81.8 wt%; forsterite, ferrian 5.82 wt%; enstatite, ferrian 3.36 wt%; enstatite 2.94 wt%; clinochlore 2.40 wt%; chromite 1.60 wt%; phlogopite 1.44 wt%; actinolite 0.33 wt%; magnetite 0.28 wt%; antigorite 0.03 wt%. This composition is close to forsteritic olivine (Mg1.8Fe0.2SiO4), as usually reported in the literature about this deposit.
Tests have been conducted on the rocking autoclave using standard GL30, milled GL30 (obtained using a rotary mill and passing 80 μm sieve), and GL10 (a fines reject from the GL30 production process). The advantage found of using GL30 for lab-scale trials is that the unreacted olivine (coarse particles) can be rather easily separated from the reaction products by decantation. This facilitates analysis and testing of the isolated reaction products, as would be produced in a full-scale GPV (assuming complete conversion).
Process Optimization
The rocking autoclave has been utilized to better understand the mineral carbonation process of olivine, with the aim to achieve two objectives: (i) reach high carbonation conversion within residence times feasible for the GPV design and (ii) find valuable applications for the reaction products to make the process industrially feasible. These two objectives are not necessarily mutually beneficial: high conversion does not necessarily entail high profit, for two reasons. First, with CO2 credit prices at low levels (Edenhofer, 2014), the amount of CO2 sequestered is not the main economic driver (Tables S1 and S2 in Supplementary Material). Hence, increasing the processing costs to achieve maximal sequestration may not be advisable. Second, product value is intimately tied to product properties. If product properties (e.g., morphology, pozzolanic activity, etc.) deteriorate as a result of chasing highest conversions, this is again not advisable.
To study how processing conditions affect conversion and product quality, an extensive experimental program has been run using the rocking autoclave. The studied process parameters have included: solid-to-liquid ratio; olivine particle size; type and concentration of additives (salts, organic acids, inorganic acids, chelating agents, oxidizing agents); reaction time; reaction temperature (maximum and profile); and CO2 partial pressure. For brevity, selected results are herein discussed, and complementary data are presented in Figures S1–S3 in Supplementary Material. The investigated effects of these parameters were reaction kinetics and conversion, product composition and morphology, and ease of product separation (from the unreacted/passivated feed, and between different product phases).
Higher conversion is obtained with higher pressures; using GL30, conversion after 180 min approximately doubled (from 8 to 16%) when doubling the total pressure from 60 to 120 bar (Figure S1 in Supplementary Material). This supports the idea that higher carbonic acid concentration promotes mineral dissolution and simultaneously promotes precipitation of carbonates, if the pH of the system is buffered using additives. Continuous precipitation of carbonate during the reaction (not only upon de-pressurization) enables further leaching of magnesium, thereby overcoming any potential solubility limit. Seeing as higher pressures promote carbonation, it would be desirable to construct the GPV as long as technically possible to maximize conversion. These low conversions are consequence of GL30’s large particle size (average 110 μm); milling GL30 (to average 20 μm) further doubles conversion (Figure S2 in Supplementary Material), which affirms that rate of mineral dissolution controls conversion extent.
As for temperature, as reported in the literature, it was found that an optimum level exists (Figure S3 in Supplementary Material). Too high temperatures reduce the CO2 solubility too drastically, while lower temperatures slow mineral dissolution. At 100 bar total pressure, the optimum temperature was found to lie around 220°C. O’Connor et al. (2005) reported an optimal temperature of 185°C at 150 atm CO2 pressure. Gadikota et al. (2014a) found higher conversion at higher temperatures, but their maximum temperature was limited to 185°C. These findings indicate that heat generation and heat transfer within the GPV are critical to sustain a high rate of reaction. As reported by Santos et al. (2013), to sustain the autothermic reaction, a sufficiently small particle size and sufficiently high solids loading are required, thus ensuring sufficient rate of heat generation per unit volume of slurry. In addition, according to thermodynamic calculations (Prigiobbe and Marco, 2013), high temperatures favor the precipitation of magnesite over that of hydromagnesite or nesquehonite; this is preferred in view of generating maximal value from the reaction products.
Because the reaction proceeds by surface-controlled mineral dissolution mechanism (Gadikota et al., 2014b), it is imperative that a high surface-to-volume ratio is created by first crushing and milling the freshly mined mineral. However, mechanical grinding is energy consuming, thus diminishing net CO2 sequestration and increasing processing costs. Rocking autoclave tests have been performed with several particle size distributions: standard GL30 (average ~110 μm), milled GL30 (average 20 μm), and GL10 (average 18 μm) (Santos et al., 2015a). Experiments have shown that smaller average particle size results in higher carbonation conversion, more than double (from 18 to 40%) between GL30 and milled GL30 after 180 min (Figure S2 in Supplementary Material). These results are in agreement with similar results reported by Eikeland et al. (2015), who compared <10 and ~100 μm olivine samples. Because of incomplete conversion, product separation appears to remain a necessary postreaction step at full-scale implementation. It should be noted, however, that complete olivine carbonation conversion is possible, as long as the reaction time is sufficiently long; Santos et al. (2015b) reached full conversion over 72 h, at 55 bar total pressure and 200°C.
To improve the overall reaction rate further, one must speed up the rate limiting step: mineral dissolution. Increasing the leaching rate of magnesium can proceed by decreasing the pH, adding acids, or organic ligands (Gadikota et al., 2014b). Organic acids are typically used for removing scale from piping and reactor systems (Frenier, 2001). Noting that residual acids after descaling of the rocking autoclave appeared to improve carbonation conversion, tests were conducted on different acids at different concentrations. A proprietary mixture at a concentration of 0.0056M delivered optimal results, increasing carbonation conversion from 12 to 22% when using GL30 after 180 min (Santos et al., 2015a). Acids that did not deliver good results included lactic, citric, and formic acids. The combination of organic acids used possibly play a role in preventing the passivation of olivine particles or in aiding the transfer of magnesium from the solid phase to the aqueous phase; the latter mechanism is illustrated by Gadikota et al. (2014b), though further study is needed to experimentally detect the precise effect.
Reaction Products
Product Characterization
Due to the preferred heterogeneous precipitation as single particles within the rocking autoclave, relatively purer products are formed; Figure 2 presents evidence of this. Using energy dispersive X-rays (EDX), it was possible to differentiate during scanning electron microscopy (SEM) the magnesite-rich particles (agglomerated primary crystals) and the silica-rich particles (colloidal aggregates of primary spherical particles); this is seen in Figure 2A. Some particles are a combination of the two products. Likewise, chromite-rich particles and residual olivine particles were identified (Figure 2B). From previous literature (Gadikota et al., 2014a; Eikeland et al., 2015), it was also noted that both magnesium- and silica-rich phases did exist but not as separate particles as shown here. It is further notable that the surface of the partially reacted olivine particle is “clean,” with streaks of dissolution clearly visible.
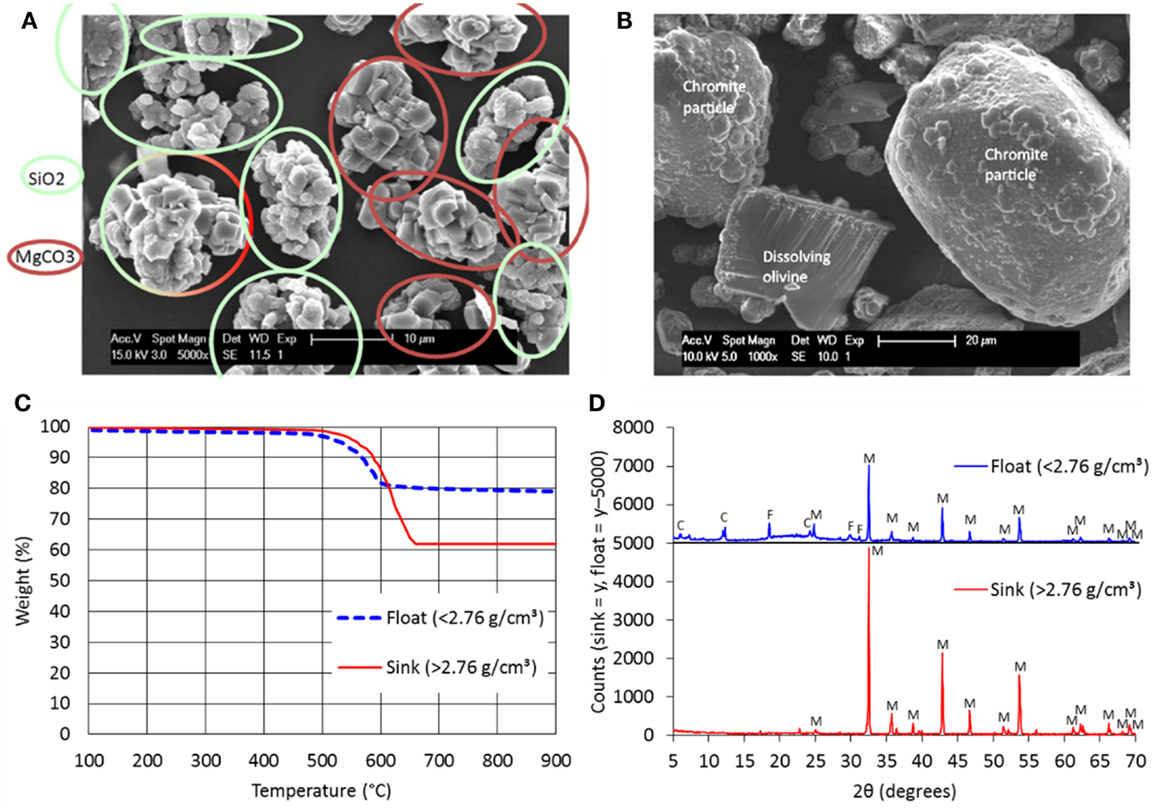
Figure 2. (A) SEM–EDX characterization of reaction products (carbonate- and silica-rich particles indicated); (B) SEM–EDX characterization of reaction products (residual olivine and chromite-rich particles indicated); (C) TGA analysis of dense liquid-separated reaction products; (D) XRD analysis of dense liquid-separated reaction products (C, clinochlore; F, forsterite; M, magnesite).
Valorization of the reaction product can be maximized by separating the two main phases: carbonate and silica. A trial was performed at the Mineral Separation Laboratory of the Vrije Universiteit Amsterdam using dense liquids, whereby lighter solids “float” and denser solids “sink” within a centrifuge. Figure 2C presents the TGA profile of two separated fractions, one of density <2.76 g/cm3 and another of density >2.76 g/cm3. It is evident from the TGA results that the denser fraction is richer in carbonate; this was verified by XRD (Figure 2D). The lighter fraction is richer in silica, as verified by its greater amorphous mineral content (“bump” seen in the “float” x-ray diffractogram). In this trial, the separation is not ideal (some carbonates still mixed with silica) but can be further optimized.
Product Application
The primary application of the reaction products that have been investigated is as substitute material in mortar and concrete applications (Santos et al., 2015a), as these are some of the few large-scale application domains that can absorb a large amount of carbonation products, reviewed by Sanna et al. (2012). The reaction products are sufficiently stable (i.e., no undesirable hydration swelling) to be used in bound applications as aggregate and possess some level of pozzolanic activity to partly replace cement in the building materials (Lazaro et al., 2013). The pozzolanic character imparts the highest value to the product (Table S1 in Supplementary Material) and presents the largest economic risk of the proposed process (Table S2 in Supplementary Material), and as such has been a main focus of research. It can be maximized through optimization of separation, specific surface area, and amorphous silica content. Trials have been performed applying the reaction products as fine aggregates in cement mortar (Santos et al., 2015a). It has been found that 2- and 90-day compressive strength reduces if cement alone is replaced (Figure S4 in Supplementary Material) but could possibly be preserved if fine sand, and to a lesser extent a smaller portion of cement, is replaced. This is a topic of ongoing research. In addition to building materials, the reaction products may find application in papermaking and polymers; these applications will be the subject of future research.
Another opportunity is the recovery of metallic components (primarily nickel and chromium) from carbonated olivine. Santos et al. (2015b) and Chiang et al. (2014) have reported on nickel extraction efforts from carbonated olivine using chemical and biological means, respectively. It has been found that at elevated acidity, more nickel can be extracted from carbonated olivine compared to fresh olivine. Trials have also been done on separating chromite-rich particles by gravity and magnetism, since they possess the highest density and magnetic properties, and positive preliminary results have been found. These chromite particles may be valorized as chromium ore or be used directly in chemical processes (e.g., as catalyst).
Conclusion and Outlook
The CO2 Energy Reactor can be an important component of the multi-array of carbon capture and sequestration (CCS) technologies needed to substantially reduce greenhouse gas emissions and stabilize, if not reduce, atmospheric levels. Compared to other CCS techniques, a major advantage of the present approach is the production of several valuable products (magnesite, colloidal silica, chromite particles, recoverable thermal energy), which can off-set processing costs. Given that the amount of Ni and Cr is not high, at “small scales” (~100 kton range) the production of the magnesium carbonate and silica remains as the main economic drivers. At large scales (megatonnes), the metal components, along with the sequestered CO2 and the exothermic re-usable heat energy, also become important economic drivers.
As mentioned throughout the article, several research questions are still under investigation. The main challenges are further intensification of the reaction, to achieve high product recoveries within the GPV limitations and better separation of the product fractions. In addition, testing of the products in potential applications, as an aggregate, filler, or pozzolan replacement, is under way. Finally, additional work is needed to accurately estimate processing costs (olivine milling, slurry pumping, gas compression, solids separation, additives consumption) to improve confidence in economic models and overall process feasibility.
Author Contributions
RS wrote and revised the paper, supervised Ph.D. student PK, conducted materials analyses, and interpreted the results. PK cowrote and revised the paper, conceptualized the technology, conducted materials analyses, organized industrial trials, and interpreted the results. KR conceptualized the technology and performed carbonation experiments, organized industrial trials, and interpreted the results. YC cowrote and revised the paper, performed leaching experiments, supervised Master’s student, and conducted materials analyses.
Conflict of Interest Statement
The CO2 Energy Reactor technology is patented by Pol C. M. Knops and Keesjan L. Rijnsburger of Innovation Concepts B.V. through patent number WO2011155830 A1.
Acknowledgments
The authors express their gratitude to Prof. Tom Van Gerven and Prof. Jan Elsen (KU Leuven) for their valued contribution and for permitting access to their analytical equipment. Sibelco is thanked for the supply of olivine. The work was partially supported by the Dutch Institute for Sustainable Process Technology (ISPT) (TKI-ISPT project DV-20-03).
Supplementary Material
The Supplementary Material for this article can be found online at http://journal.frontiersin.org/article/10.3389/fenrg.2016.00005
References
Broecker, W. S. (2008). CO2 capture and storage; possibilities and perspectives. Elements 4, 295–297.
Chakma, A. (1997). CO2 capture processes – opportunities for improved energy efficiencies. Energy Convers. Manag. 38, S51–S56. doi: 10.1016/S0196-8904(96)00245-2
Chiang, Y. W., Santos, R. M., Van Audenaerde, A., Monballiu, A., Van Gerven, T., and Meesschaert, B. (2014). Chemoorganotrophic bioleaching of olivine for nickel recovery. Minerals 4, 553–564. doi:10.3390/min4020553
Dunsmore, H. E. (1992). A geological perspective on global warming and the possibility of carbon dioxide removal as calcium carbonate mineral. Energy Convers. Manag. 33, 565–572. doi:10.1016/0196-8904(92)90057-4
Edenhofer, O. (2014). Climate policy: reforming emissions trading. Nat. Clim. Change 4, 663–664. doi:10.1038/nclimate2327
Eikeland, E., Blichfeld, A. B., Tyrsted, C., Jensen, A., and Iversen, B. B. (2015). Optimized carbonation of magnesium silicate mineral for CO2 storage. ACS Appl. Mater. Interfaces 7, 5258–5264. doi:10.1021/am508432w
Frenier, W. W. (2001). “Novel scale removers are developed for dissolving alkaline earth deposits,” in Proceedings – SPE International Symposium on Oilfield Chemistry (Richardson: Society of Petroleum Engineers).
Gadikota, G., Matter, J., Kelemen, P., and Park, A. A. (2014a). Chemical and morphological changes during olivine carbonation for CO2 storage in the presence of NaCl and NaHCO3. Phys. Chem. Chem. Phys. 16, 4679–4693. doi:10.1039/c3cp54903h
Gadikota, G., Swanson, E. J., Zhao, H., and Park, A. A. (2014b). Experimental design and data analysis for accurate estimation of reaction kinetics and conversion for carbon mineralization. Ind. Eng. Chem. Res. 53, 6664–6676. doi:10.1021/ie500393h
Hitch, M., and Dipple, G. M. (2012). Economic feasibility and sensitivity analysis of integrating industrial-scale mineral carbonation into mining operations. Miner. Eng. 39, 268–275. doi:10.1016/j.mineng.2012.07.007
Lazaro, A., Quercia, G., Brouwers, H. J. H., and Geus, J. W. (2013). Synthesis of a green nano-silica material using beneficiated waste dunites and its application in concrete. World J. Nano Sci. Eng. 3, 41–51. doi:10.4236/wjnse.2013.33006
McGrew, J. L. (1981). Method and Apparatus for Effecting Subsurface, Controlled, Accelerated Chemical Reactions. US4272383 A. USA: United States Patent and Trademark Office.
Meinshausen, M., Smith, S. J., Calvin, K., Daniel, J. S., Kainuma, M. L. T., Lamarque, J.-F., et al. (2011). The RCP greenhouse gas concentrations and their extensions from 1765 to 2300. Clim. Change 109, 213–241. doi:10.1007/s10584-011-0156-z
O’Connor, W. K., Dahlin, D. C., Rush, G. E., Gerdemann, S. J., Penner, L. R., and Nilsen, D. N. (2005). Aqueous Mineral Carbonation – Mineral Availability, Pretreatment, Reaction Parametrics, and Process Studies (Tech. Rep. No. DOE/ARC-TR-04-002). Albany, OR: US Department of Energy.
Power, I. M., Harrison, A. L., and Dipple, G. M. (2013). Carbon mineralization: from natural analogues to engineered systems. Rev. Mineral. Geochem. 77, 305–360. doi:10.2138/rmg.2013.77.9
Prigiobbe, V., and Marco, M. (2013). Precipitation of Mg-carbonates at elevated temperature and partial pressure of CO2. Chem. Eng. J. 223, 755–763. doi:10.1016/j.cej.2013.03.033
Rijnsburger, K. J. L., and Knops, P. C. M. (2011). Method for Converting Metal Comprising Silicate Minerals into Silicon Compounds and Metal Compounds. WO2011155830 A1. Switzerland: World Intellectual Property Organization.
Sanna, A., Hall, M. R., and Maroto-Valer, M. (2012). Post-processing pathways in carbon capture and storage by mineral carbonation (CCSM) towards the introduction of carbon neutral materials. Energy Environ. Sci. 5, 7781–7796. doi:10.1039/c2ee03455g
Sanna, A., Uibu, M., Caramanna, G., Kuusik, R., and Maroto-Valer, M. M. (2014). A review of mineral carbonation technologies to sequester CO2. Chem. Soc. Rev. 43, 8049–8080. doi:10.1039/c4cs00035h
Santos, R. M., Knops, P. C. M., and Rijnsburger, K. (2015a). “CO2 utilization via intensified and integrated mineral carbonation: process and products optimization,” in Proceedings of the 54th Annual Conference of Metallurgists (COM2015) (Westmount: The Canadian Institute of Mining, Metallurgy and Petroleum), 8734.
Santos, R. M., Van Audenaerde, A., Chiang, Y. W., Iacobescu, R. I., Knops, P., and Van Gerven, T. (2015b). Nickel extraction from olivine: effect of carbonation pre-treatment. Metals 5, 1620–1644. doi:10.3390/met5031620
Santos, R. M., Verbeeck, W., Knops, P., Rijnsburger, K., Pontikes, Y., and Van Gerven, T. (2013). Integrated mineral carbonation reactor technology for sustainable carbon dioxide sequestration: ‘CO2 Energy Reactor’. Energy Procedia 37, 5884–5891. doi:10.1016/j.egypro.2013.06.513
Keywords: gravity pressure vessel, mineral carbonation, olivine, magnesite, colloidal silica, chromite, mineral separation, building materials
Citation: Santos RM, Knops PCM, Rijnsburger KL and Chiang YW (2016) CO2 Energy Reactor – Integrated Mineral Carbonation: Perspectives on Lab-Scale Investigation and Products Valorization. Front. Energy Res. 4:5. doi: 10.3389/fenrg.2016.00005
Received: 15 September 2015; Accepted: 01 February 2016;
Published: 15 February 2016
Edited by:
Renato Baciocchi, University of Rome Tor Vergata, ItalyReviewed by:
Stefano Stendardo, Italian National Agency for New Technologies, Energy, ItalySebastian Teir, VTT Technical Research Centre of Finland Ltd., Finland
Copyright: © 2016 Santos, Knops, Rijnsburger and Chiang. This is an open-access article distributed under the terms of the Creative Commons Attribution License (CC BY). The use, distribution or reproduction in other forums is permitted, provided the original author(s) or licensor are credited and that the original publication in this journal is cited, in accordance with accepted academic practice. No use, distribution or reproduction is permitted which does not comply with these terms.
*Correspondence: Rafael M. Santos, cmFmYWVsLnNhbnRvcyYjeDAwMDQwO2FsdW1uaS51dG9yb250by5jYQ==