- 1Chinese Academy of Sciences Key Laboratory of Low-Carbon Conversion Science and Engineering, Shanghai Advanced Research Institute, Chinese Academy of Sciences, Shanghai, China
- 2Department of Chemical and Environmental Engineering, Faculty of Engineering, University of Nottingham, Nottingham, UK
CO2 capture represents the key technology for CO2 reduction within the framework of CO2 capture, utilization, and storage (CCUS). In fact, the implementation of CO2 capture extends far beyond CCUS since it will link the CO2 emission and recycling sectors, and when renewables are used to provide necessary energy input, CO2 capture would enable a profitable zero- or even negative-emitting and integrated energy–chemical solution. To this end, highly efficient CO2 capture technologies are needed, and adsorption using solid adsorbents has the potential to be one of the ideal options. Currently, the greatest challenge in this area is the development of adsorbents with high performance that balances a range of optimization-needed factors, those including costs, efficiency, and engineering feasibility. In this review, recent advances on the development of carbon-based and immobilized organic amines-based CO2 adsorbents are summarized, the selection of these particular categories of materials is because they are among the most developed low-temperature (<100°C) CO2 adsorbents up to date, which showed important potential for practical deployment at pilot-scale in the near future. Preparation protocols, adsorption behaviors as well as pros and cons of each type of the adsorbents are presented, it was concluded that encouraging results have been achieved already, however, the development of more effective adsorbents for CO2 capture remains challenging and further innovations in the design and synthesis of adsorbents are needed.
Introduction
CO2 Capture and an Integrated Energy–Chemical Solution
The atmospheric CO2 concentration now exceeds 400 ppm (CO2Now.org, 2014), and its continuous increase is believed to be the major factor leading to global warming and climatic change (Crowley, 2000; Held and Soden, 2000; Patz et al., 2005). Kaya et al. and Hoffert et al. have quantified net CO2 emissions in terms of population, economic activity, and energy-related factors using Eq. 1 (Kaya, 1995; Hoffert et al., 1998).
where C is the net CO2 emission, P is population, GDP is the gross domestic product that is used here as an indicator of economy-related factor, and E is energy production. Therefore, GDP/P is the Per capita GDP, and E/GDP can be regarded as the energy intensity during production that is closely related to the efficiency of energy utilization. The last term, C/E, represents the carbon intensity during energy production. As for the development of the human society and life quality, it is improper to limit population and production, which means there is no solution to CO2 reduction from the first and second term in Eq. 1. On the other hand, increase in the energy production/utilization efficiency and control of the carbon footprint during energy processes are the major strategies for CO2 sequestration (third and fourth term in Eq. 1).
The complete decarbonization of energy is believed to be the ultimate solution for the long-term sustainability, and thus over the past decades, considerable researches have been devoted to the development of low-carbon technologies covering nearly all forms of energy (Song, 2006; Li and Fan, 2008; Kumar et al., 2009; Panwar et al., 2011; Swain et al., 2011; Vasireddy et al., 2011; Chu and Majumdar, 2012; Shi et al., 2013; Sangeeta et al., 2014). Novel concepts and ideas for fossil, bio-, and renewable energies have emerged as depicted in Figure 1. Indeed, for the continued using of fossil energy and fully decarbonized electricity supply, CO2 capture, utilization, and storage (CCUS) has been recognized as one of the key technologies that needs to be demonstrated and commercialized (Mikkelsen et al., 2010; Boot-Handford et al., 2014).
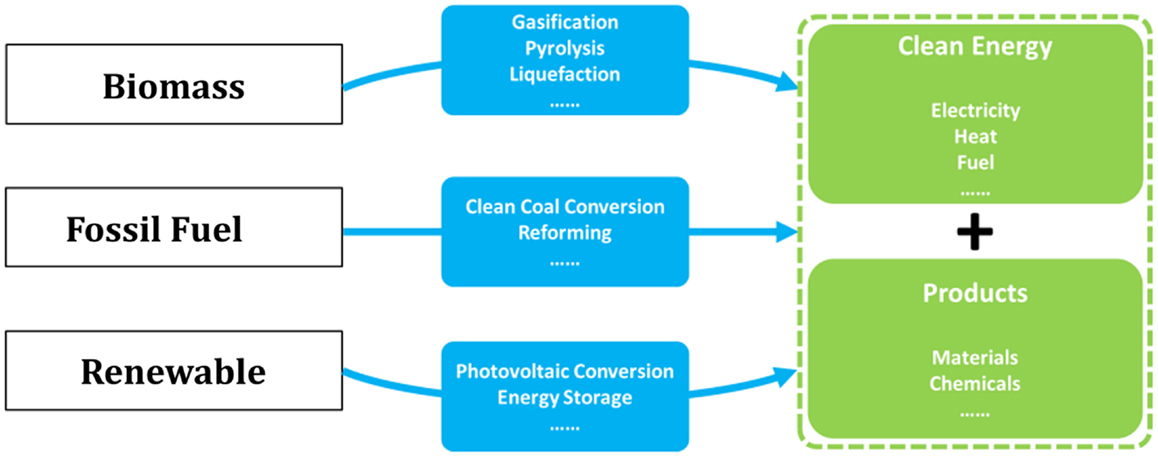
Figure 1. Overall solutions for low-carbon energy and chemical production from fossil, biomass, and renewable sources.
As the most energy- and cost-intensive phase of CCUS, it is well-demonstrated that CO2 capture is of vital importance to the technical and economical availability of the technology, and many investigations have been carried out (MacDowell et al., 2010; Markewitz et al., 2012). However, fewer researchers have recognized that CO2 capture, when linked to CO2 utilization and chemical production processes, will act as an enabling technology for more effective integrated energy solutions, for example, as those depicted in Figure 2 where all the energies needed for fuel upgrading and chemical production are provided by renewables, and CO2 capture represents a linker between the CO2 emitting and recycling processes (red and green arrows in Figure 2, respectively; Graves et al., 2011; Budzianowski, 2012; Siglinda and Centi, 2014). Consequently, carbon is fully converted to fuels and/or chemicals in this integrated energy–chemical scenario so that near zero emissions can be achieved. Furthermore, if CO2 is captured from power plants running on biomass, then an integrated energy and chemical production scenario with negative carbon emissions can be achieved that would potentially be profitable when carbon tax is applied.
Based on these future energy scenarios, it is obvious that CO2 capture is even more important to the integrated energy–chemical solution as compared to CCUS. This is not to say that development of CCUS is unnecessary, but to emphasize that CO2 capture may be involved in our combat toward climate change and energy solution much longer than we currently recognize.
Technical Options for CO2 Capture and the Potential of Solid Adsorbents
Currently, the state-of-the-art technology for CO2 separation is amine scrubbing. In this process, CO2 in a mixture gas is absorbed by an aqueous solution of alkanolamine via the acid–base reaction, and the amines can be regenerated by stripping with water vapor at elevated temperatures, concentrated CO2 can be obtained after water is condensed from the stripper vapor (Rochelle, 2009). However, although amine scrubbing technology has been deployed in a range of applications such as natural gas sweetening, it suffers from a number of shortcomings such as high energy penalty, thermal/oxidative degradation of the solvents, and corrosion (Sunil and Merched, 2014). Furthermore, when amine scrubbing is applied, the overall energy penalty at power plants reduces efficiency typically by 10%, representing a reduction in electricity generation of 25% for a modern supercritical coal power plant. On the other hand, some emerging technologies, such as membranes, are still in their infancy, therefore, more energy-efficient processes are needed to be developed for CO2 capture, where adsorption is one such alternative (Choi et al., 2009; Wang et al., 2011a).
The greatest challenge for adsorption is the development of appropriate materials that can be effectively used under processing conditions (Choi et al., 2009; Bollini et al., 2011; Jones, 2011; Sayari et al., 2011; Wang et al., 2011a,b). In many papers, adsorption capacity is the only criterion used to evaluate adsorbents, for practical CO2 capture applications however, there are more requirements. This is illustrated in Figure 3, where process cost, process efficiency, and engineering feasibility are represented as the corners of a triangle (elements, hereafter), and factors (circles inside the triangle) that determine the quality of an adsorbents can be located in the triangle according to their influence on each of the elements. For example, the factor of adsorption/desorption kinetics (factor 6 in Figure 3) is located at the bottom left of the triangle since adsorbents with fast kinetics can increase the efficiency of a process and lowering the related costs by accelerating the adsorption–desorption cycles. Meanwhile, faster cycling requires better designed and integrated adsorption/desorption sub-units, leading to further difficulties in process engineering and limitations in adsorbents selection. The comprehensive requirements for CO2 adsorbents are also discussed in a recent review by Sayari et al. (2011). In fact, it is arbitrary to gain any quantitative conclusions from Figure 3 as the influence of the different factors on the three key elements may vary according to different CO2 capture environments, but it is obvious that development of suitable materials for CO2 capture is indeed the key challenge.
Aims and Scope of This Review
In this review, recent advances on researches of solid adsorbents for CO2 capture is summarized, readers who are interested in other CO2 capture technologies such as absorption, oxy-fuel, and chemical looping are directed to references (Bara et al., 2010; Blamey et al., 2010; Karadas et al., 2010; Toftegaard et al., 2010; Du et al., 2012; Zhang et al., 2012; Sunil and Merched, 2014). We will focus on the low-temperature adsorbents because they can (i) potentially match the requirements in Figure 3 and (ii) be suitable for using in the integrated energy–chemical solutions (Figure 2). Readers who are interested in mid-high-temperature CO2 adsorbents (which are very important materials for CO2 treatment as well) are directed to references (Choi et al., 2009; Wang et al., 2011a,b; Donat et al., 2012; Ridha et al., 2013; Zhao et al., 2013, 2014). At the same time, some of the emerging adsorbents such as metal organic frameworks (MOFs), covalent organic frameworks (COFs) where material cannot yet be prepared at large-scale for the moment will not be covered. Readers who are interested in these adsorbents are directed to several excellent reviews published recently (Choi et al., 2009; Wang et al., 2011a,b; Dawson et al., 2012; Sumida et al., 2012).
Carbon
General Information
Carbon-based materials such as activated carbons (ACs), carbon nano-tubes (CNTs) have been used in gas separation for a long time (Bradley, 2011; Ren et al., 2011; Ma et al., 2013; Sevilla and Mokaya, 2014), and therefore, it is not surprising that various carbons are prepared and tested as adsorbents for CO2 capture. Basically, carbon-based materials can be prepared by high-temperature treatment of carbon-containing substance in an inert atmosphere, (carbonization, pre-treatments may be applied before carbonization in some cases), the obtained carbons can be further treated by activation, where the carbons are partially gasified by reaction with an activating agent (H2O steam, CO2, and KOH), leading to enhanced porosity (microporosity, particularly). Functionalization of carbon materials may be performed at any stage of their preparation to further modify the surface chemistry toward desired tasks.
Activated carbons are characterized by their high surface areas (typically >1000 m2/g) and low chemical activity, therefore, they have been typically characterized as physical adsorbents (Sayari et al., 2011; Wang et al., 2011a). Consequently, adsorption of CO2 on carbon surface was believed to rely on the highly developed porous structure. It was reported that narrow microporosity (<0.7 nm) is vitally important for CO2 adsorption at low pressures (~1 bar), while at elevated pressures, larger pores are gradually occupied, which means the adsorption occurs via a “pore-filling” mechanism. Such a theory has been verified by some authors via the linear relationship between the measured CO2 adsorption capacities and the volumes of pores with different sizes as illustrated in Figures 4 and 5 (Martin et al., 2011; Sun et al., 2013). More recently, Hu et al. used a more sophisticated method (Quenched-Solid Density Functional Theory) to analyze the low-temperature N2 adsorption data, by which high-resolution pore distributions of a series of petroleum coke-derived carbons could be obtained. This enabled conclusions to be drawn that at atmospheric pressure, high-density CO2 filled the pores smaller than 1 nm, upon increasing pressure, larger pores are filled (up to about 4 nm at 10 bar; Hu et al., 2011). Similar conclusions have also been achieved by using Grand Canonical Monte Carlo simulation and Non-Local Density Functional Theory (NLDFT) (Martin-Martinez et al., 1995; Cazorla-Amoros et al., 1996; Vishnyakov et al., 1999; Wei et al., 2012).
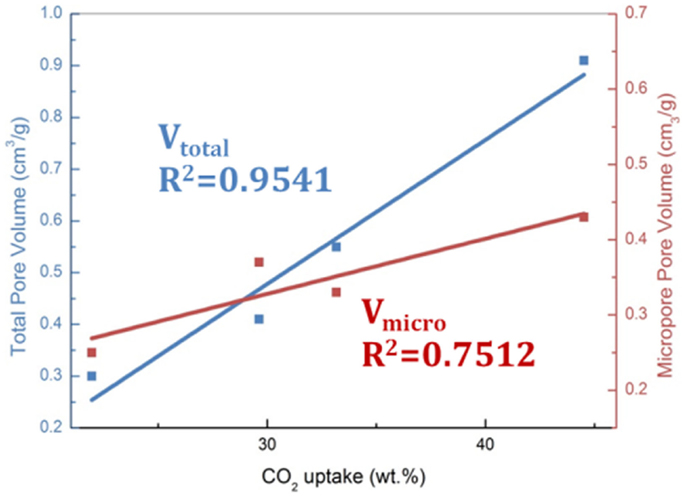
Figure 4. The linearship between high-pressure CO2 adsorption capacities (30°C, 40 bar) and porosity, reproduced from Sun et al. (2013).
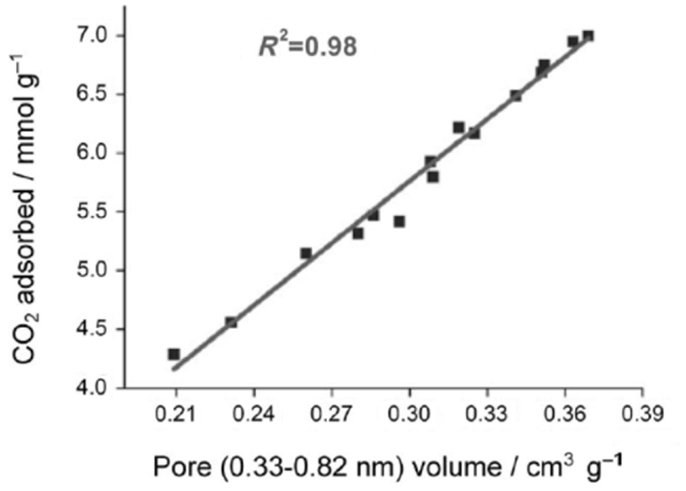
Figure 5. The linearship between CO2 adsorption capacities (25°C, 1 bar) and microporosity, reproduced from Wei et al. (2012).
Merits for carbon adsorbents include low cost, fast kinetics (equilibrium adsorption can be achieved within less than 10 min), ultra-high stability, easy-to-regenerate, hydrophobicity, and most importantly, high adsorption capacities at elevated (partial) pressures, these features make them well suited to IGCC applications (Choi et al., 2009; Shafeeyan et al., 2010). Their main disadvantage currently is the low capacities at low (partial) pressures due to the inert surface chemistry. To circumvent this issue, some attempts with encouraging results have been published on the modification and/or activation of carbons to strengthen the surface affinity toward CO2, by which the adsorption occurs to be more “chemical.” All these will be overviewed in the following sections, some of the key parameters for carbon adsorbents reported in the literature are summarized in Table 1.
Pure Carbons from Different Precursors
As mentioned above, carbon materials can be prepared by carbonization of almost all carbon-containing substances. In this section, pure carbons without any hetero-atom modification for CO2 capture that have been developed are summarized.
Carbons from polymers
Polymers have been frequently used as precursors for preparing carbon-based CO2 adsorbents since the purity, porous structure, macroscopic morphologies, and surface chemistries can be easily tuned by optimization and appropriate pre-treatment of the polymer precursors. The most used polymer precursors were phenolic resins due to their relatively low costs (An et al., 2009; Martin et al., 2011; de Souza et al., 2013; Garcia et al., 2013; Sun et al., 2013; Wickramaratne and Jaroniec, 2013). By varying the formaldehyde-to-phenol ratio and catalysts during synthesis, resol and novolac phenolic resins were prepared by Pevida et al., after carbonization of the resulted resins and subsequent activation in a CO2 atmosphere, microporous carbons were obtained with CO2 adsorption capacities of around 10.8 wt. % (25°C and 1 bar), which further increased to 44.7 wt. % at a pressure of 25 bar on the best sample (Martin et al., 2011; Garcia et al., 2013). The adsorption capacities of the phenolic resin-based adsorbents can be effectively improved by KOH activation (20.2 wt. % at 23°C and 1 bar) or alternatively, by inducing a second carbon source during preparation (ca. 13.0 wt. % at 25°C and 1 bar; An et al., 2009; de Souza et al., 2013; Wickramaratne and Jaroniec, 2013).
In addition to phenolic resins, Qiu and co-workers carbonized porous aromatic frameworks PAF-1 (Ben et al., 2009), and an all-carbon-scaffold microporous carbon was thus prepared, which adsorbs 19.8 wt. % CO2 at 0°C and 1 bar, and the CO2/N2 adsorption selectivity is as high as 209 in a 15/85 CO2/N2 mixture as calculated by the Ideal Adsorption Solution Theory (IAST) (Ben et al., 2012). Similar to the strategy of Feng et al. (An et al., 2009), adsorbents were prepared by embedding an extra carbon source into the PAF-1 framework and, following carbonization, the resulted sample gave a CO2 adsorption capacity of 18.2 wt. % as compared to 15.5 wt. % over the bare PAF-1-derived carbon owing to the introduction of ultra-narrow pores (<0.8 nm) that are very effective for CO2 adsorption at low (partial) pressure as previously mentioned (Zhang et al., 2013).
Carbons from biomass
Biomass represents a cost-effective and widely available option for the preparation of carbon materials (Mohan and Pittman, 2006; White et al., 2009; Kalyani and Anitha, 2013). For example, CO2 could be substantially adsorbed on olive stone or almond shell (both are low-cost biomass residuals) derived carbon materials (Plaza et al., 2009). In Fuertes et al.’s work, hydrochar that was obtained by hydrothermal treatment of selected biomass was submitted to KOH activation, a high CO2 adsorption capacity of 21.2 wt. % was achieved at 25°C and 1 bar (Sevilla and Fuertes, 2011). Similarly, carbonization of bamboo followed by KOH activation led to ACs with almost the same CO2 adsorption capacity at identical conditions (Wei et al., 2012).
Carbons from pitches
Carbons with superior adsorption capacity toward CO2 were prepared from KOH activation of petroleum pitch by Silvestre-Albero et al., the nature of the petroleum residue, the KOH/pitch ratio, the mesophase content, and the preparation conditions are optimized, the best sample achieved a CO2 adsorption capacity of 20.2 wt. % at 25°C and 1 bar with excellent selectivity (~100% for CO2/CH4 separation and 14% for CO2/N2 separation), the performance was claimed to be highly exceeding that for MOFs (Wahby et al., 2010; Silvestre-Albero et al., 2011). Employing similar fabrication protocol (carbonization-KOH activation), Cao et al. prepared ACs with high surface areas (~3000 m2/g) from coal tar pitches (Shao et al., 2011), the samples showed relatively weak CO2 adsorption at low pressures (<10 wt. % at 25°C and 1 bar), surprisingly, with the increasing of pressure, significant enhancing of adsorption was observed with an extremely high CO2 uptake of nearly 90 wt. % at 18 bar.
Carbons from other precursors
In contrast to the traditional precursors described above, some alternative materials are emerging and have been tested by several researchers. For example, deep eutectic solvents composed of resorcinol, 4-hexylresorcinol, and tetraethylammonium bromide were used by Monte et al. for the preparation of carbons with good capacities for CO2 adsorption (up to 10.0 wt. % at 25°C and 1 bar; Patino et al., 2012). Gogotsi et al. treated titanium carbide powders in chlorine and hydrogen, after activation, one of the samples exhibited a high CO2 adsorption capacity of 31.2 wt. % at 0°C and 1 bar, a linearship between CO2 uptake and the pore volume of narrow micropores was observed confirming their importance to the adsorption of CO2 at low pressures (Presser et al., 2011). More recently, Srinivas prepared a novel carbon materials by direct carbonization of MOFs, thanks to the releasing of CO, CO2, and gaseous Zn (which is formed by the reduction of ZnO during carbonization), a hierarchically porous structure with simultaneous high surface area and pore volume can be obtained, and thus the obtained samples adsorbed ~15.0 and 119.0 wt. % CO2 at 1 and 30 bar, respectively (27°C) (Srinivas et al., 2014).
Hetero-Atom Modified Carbons
One of the challenges for the development of carbon-based CO2 adsorbents is to enhance their adsorption capacities at relatively low pressures by strengthening their surface affinity toward the CO2 molecule. The most studied strategy to address this issue is modification of the carbon framework with hetero-atoms, such as nitrogen, sulfur, and metallic atoms.
Nitrogen-modified carbons
Nitrogen-bearing functionalities are basic in nature, they interact more strongly with the acidic CO2 molecule as compared with the neutral carbon framework. Therefore, introduction of these groups into carbons has been investigated extensively as a means to increase the CO2 adsorption capability. There are basically two approaches to involve nitrogen into the carbon frameworks, namely post-synthesis treatment and using nitrogen-bearing precursors.
To introduce nitrogen by post-synthesis treatment, thermal treatment in ammonia gas is normally used since it decomposes at elevated temperatures to nitrogen-bearing radicals such as NH2 and NH that are particularly active and can attack the carbon framework leading to the modification of the carbon surface by anchoring of nitrogen-bearing functionalities. By thermal treatment of commercially available ACs and biomass-derived carbons in ammonia, it was confirmed that nitrogen functional groups were successfully introduced into the carbon framework, and an increase of CO2 adsorption was observed on the modified samples as compared with the pristine one (Przepiorski et al., 2004). A similar effect of amination in enhancing the adsorption of CO2 has also been reported based on the modification of ordered mesoporous carbons, which showed an adsorption capacity of 14.5 wt. % at 25°C and 1 bar (Liu et al., 2011). In fact, the amount of nitrogen that can be introduced into the carbon framework by post-synthesis treatment is low (<5 wt. %), which led to limited increases in CO2 uptakes, although a pre-oxidation step would benefit the introduction of nitrogen (Shafeeyan et al., 2011), more effective methodologies are yet to be developed.
Direct carbonization of nitrogen-bearing precursors will lead to the in situ retention of nitrogen as various functionalities in the produced carbons. This has been demonstrated to be a more promising means for the synthesis of carbon-based CO2 adsorbents as compared with the post-synthesis method in terms of the amount of nitrogen that can be introduced into the carbon framework. Nitrogen-bearing polymers are excellent candidates as precursors for the preparation of CO2 adsorbents owing to their wide availability. To such a purpose, polyacrylonitrile (PAN), polyacrylonitrile-block-poly(n-butyl acrylate) (PAN-b-PBA), melamine modified phenolic resins, and polypyrrole have been carbonized and activated by several authors. Evaluation of these materials indicated high CO2 adsorption capacities up to 22.6 wt. % (25°C, 1 bar) with excellent kinetics, selectivity, and regenerability (Drage et al., 2007; Sevilla et al., 2011; Shen et al., 2011; Chen et al., 2012; Nandi et al., 2012; Zhong et al., 2012). In Kim’s work, polypyrrole was combined with graphene sheets by in situ polymerization of pyrrole in graphene oxide using ammonium persulfate and subsequent reduction using hydrazine. Such a combination offered a solution to the small surface area and high cost of polypyrrole when used for CO2 capture, and the resulted composite adsorbent possessed a high CO2 adsorption capacity of 18.9 wt. % at 25°C and 1 bar (Chandra et al., 2012).
Hard templating represents an alternative route for the preparation of nitrogen-modified carbons by using wet chemistry and/or chemical vapor deposition. Nitrogen-bearing precursors with a “pre-defined porous structure” can be obtained by introducing precursors into the matrix of the sacrificial template, which facilitates the efficacy of subsequent carbonization and activation. Following this strategy, Han et al. developed porous carbon materials by using a tri-continuous mesoporous silica IBN-9 as a hard template, the resulted samples after KOH activation exhibited very high CO2 uptakes of 19.8 and 46.3 wt. % at 1 and 8 bar, respectively (25°C) (Zhao et al., 2012a). Similar results by using other templates and carbon precursors are reported by a number of groups (Pevida et al., 2008; Li et al., 2010; Xia et al., 2011; Wang and Yang, 2012).
Non-nitrogen atom modified carbons
Park and co-workers employed a copper/nickel-electroplating method to prepare copper oxide and nickel oxide-decorated carbons, it was found that the introduction of these metal oxides into the carbon framework enhanced the adsorption of CO2 substantially due to the electron donating effect of copper and nickel oxides. However, the adsorption capacity of these metal oxide modified carbons are relatively low (<10 wt. % at 25°C and 1 bar) as compared with those of nitrogen-modified carbons (Jang and Park, 2012; Kim et al., 2012).
Sulfur-doped carbons were prepared and evaluated for CO2 capture by Kim et al., the microporosity, surface area, and oxidized sulfur content of the material were found to be the determining factors for CO2 adsorption, with the highest CO2 uptake reported being 19.8 wt. % at 25°C and 1° bar (Seema et al., 2014). Xia et al. used zeolite EMC-2 as a hard template to fabricate sulfur modified carbons, a much lower adsorption capacity of 11.0 wt. % was reported, most probably because activation was not carried out. However, an ultra-high CO2 adsorption heat of 59 kJ/mol was calculated at low CO2 coverage, this result indicates the promise of the sulfur modified carbons to be used in low-pressure CO2 adsorption applications, and therefore further studies are needed (Xia et al., 2012).
It is worth mentioning here that in a study by Han et al., extra-framework potassium cation was introduced into a nitrogen containing carbon material attempting to combine the strong affinity from both basic sites, a high CO2 uptake at 25°C of 7.13 wt. % at only 0.1 bar (more relevant to CO2 capture from flue gas) was reached with excellent adsorption selectivity, which can be related to the enhanced surface electrostatic interactions (Zhao et al., 2012b).
Carbons with Ordered Macroscopic Morphologies
As illustrated in Figure 3, adsorbents that can be used in practical CO2 capture applications will have to be fabricated in certain physical forms, however, most of the reported carbon-based CO2 adsorbents are powders with very low densities. Therefore, they need to be further fabricated via pelletization or granulation process with the aid of binders or additives, which can often lead to significant loss in their adsorption performance (Williams, 2001; Davis, 2002; Kadib et al., 2009; Lohe et al., 2009; Wen et al., 2010; Hao et al., 2011). Correspondingly, it is of great promise to directly prepare carbon adsorbents with ordered macroscopic morphologies. To this end, using polymers as precursors, advantage of their ability to be easily fabricated to desired forms can be taken, which can potentially be retained in the produced carbons. By carbonization of monolithic polymer precursors, Lu’s group developed a nitrogen-modified carbon monolith with a high mechanical strength, which exhibited CO2 adsorption capacities up to 14.5 wt. % at 25°C and 1 bar (Hao et al., 2010, 2011). Uyama et al. dissolved PAN in a mixture of hot DMSO and H2O, where cooling of the solution led to the phase separation and resulted in a PAN monolith, and CO2 uptakes up to 22.6 wt. % (25°C and 1° bar) were obtained after carbonization (Nandi et al., 2012). Sun et al. developed a hydrothermal process to prepare phenolic resin beads, after carbonization and subsequent modification, carbon beads with a diameter of ca. 0.7 mm were obtained. Nitrogen-bearing functionalities can be introduced by either post-synthesis treatment of the beads or directly preparing melamine modified resin beads, the samples exhibited CO2 uptakes of ca. 10.0 and 40.0 wt. % at 1 and 40 bar, respectively (30°C) (Sun et al., 2013).
In summary, the development of carbon-based CO2 adsorbents with high performance has been extensively investigated recently with some encouraging results emerging. However, important challenges remain unsolved such as enhancement of adsorption capacities at low (partial) pressures by surface modification, lowering the cost of preparation by using biomass or wastes as precursors, and so on.
Immobilized Organic Amines
General Information
Immobilization of organic amines into the pores of supporting materials (normally SiO2) is one of the most studied adsorbent systems for CO2 capture after Song and co-workers described the concept of “molecular basket” (Xu et al., 2002, 2003). The basic idea is dispersing organic amines, which are normally liquids of high viscosity, in a porous support to best expose the basic amine groups as adsorption sites, and thus the mass transfer difficulties in pure amines can be circumvented (Figure 6).
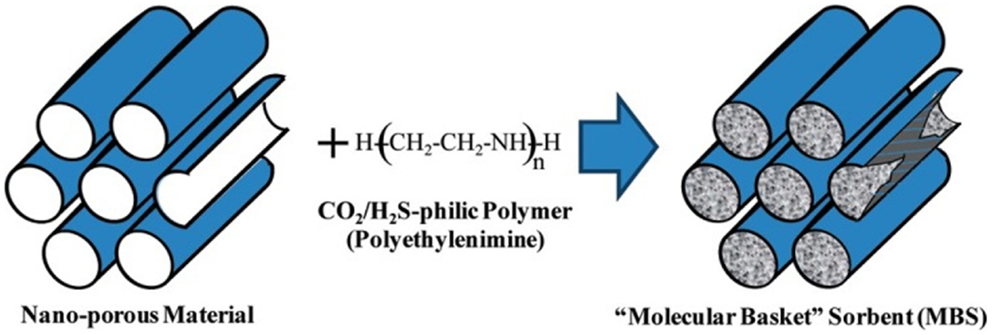
Figure 6. Concept of “molecular basket,” reproduced from Ma et al. (2009).
The basic adsorption mechanism of these materials is the chemical reaction between amine groups and CO2 that is similar to aqueous amine scrubbing as shown by Eqs 2 and 3 (Sayari et al., 2011).
Primary and secondary amines react with CO2 following a zwitterion mechanism where water or another amine group is needed. Consequently, two amine groups are required to capture one CO2 molecule in dry conditions (Eq. 2), while in the presence of water, bicarbonate can be formed to potentially double the amine efficiency as indicated in Eq. 3. For tertiary amines, H2O is necessary for CO2 adsorption via the formation of bicarbonate. It should be noted that when taking the surface characteristics of the immobilized amine adsorbents into consideration, the adsorption mechanism becomes more complicated. For example, amine groups may react with the surface silanol groups leading to amine efficiency lower than expected, and some of the initially formed carbamic acid may remain unchanged due to the lack of amine groups at close vicinity (Knofel et al., 2009; Bollini et al., 2011). Even in the presence of H2O, the formation of carbamates is kinetically favored over bicarbonate although the latter is more stable. The co-existence of the two competing reactions as well as the potential mass transfer barriers from the surface to bulk amine groups suggests that the CO2 adsorption by immobilized amines will depend at least partially on contact time (Vaidya and Kenig, 2007; Serna-Guerrero et al., 2008; Wang et al., 2009).
According to Jones et al., the immobilized amine adsorbents can be classified into three categories (Choi et al., 2009; Bollini et al., 2011), namely (i) those prepared from physical deposition/combination of pre-synthesized amines onto SiO2 (Class 1), (ii) those materials with covalent bonds between the amines and the SiO2 support (Class 2), and (iii) those prepared by the in situ polymerization of reactive amine monomers on and in the silica support. Figure 7 reproduced from Bollini et al. (2011) illustrates the difference between these materials, and this section will be organized according to this classification, key parameters for immobilized amine adsorbents can be found in Tables 2–4.
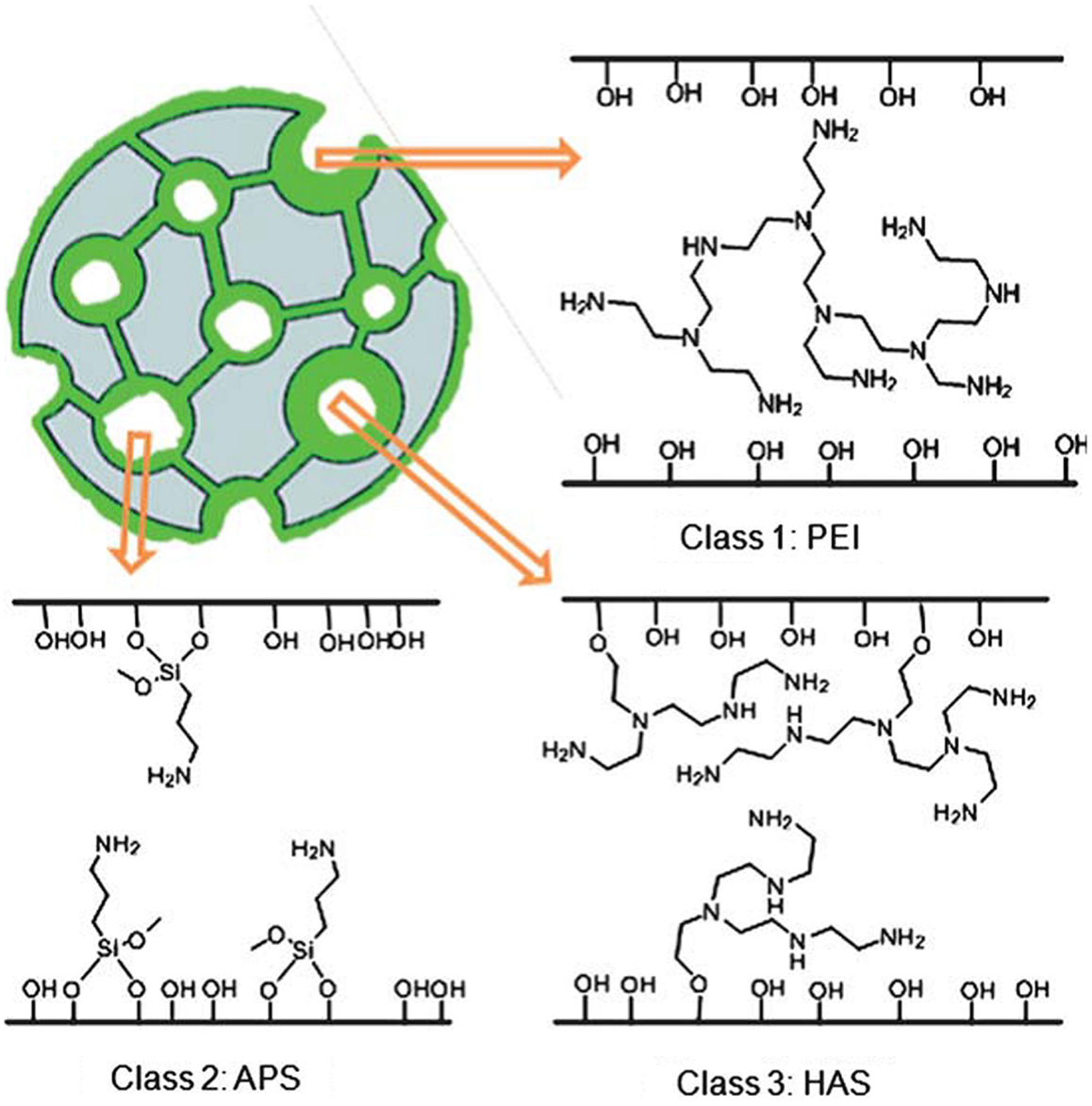
Figure 7. Classification of immobilized amine adsorbents, reproduced from Bollini et al. (2011).
Introduction of Amine by Physical Deposition/Combination (Class 1)
Effect of support
For the Class 1 immobilized amine adsorbents, pre-synthesized organic amines are firstly dissolved in methanol or H2O, porous SiO2 is then added and the formed slurry is stirred and dried to remove the solvent, leading to the formation of amine–SiO2 hybrid materials that amines are dispersed and filled into the pores of SiO2. Therefore, the nature of support is of great importance to the performance of the adsorbents by providing enough dispersing spaces (Bollini et al., 2011).
The most widely investigated supports are ordered mesoporous SiO2 such as MCM-41 and SBA-15. It was reported that upon loading of polyethylenimine (PEI) up to a certain level, the porous structure of the original supports can be roughly reserved although the surface area and pore volume decreased drastically. Accordingly, the adsorption capacities of the resulted adsorbents increased with the loading of PEI before a maximum value (ca. 14.0 and 18.0 wt. % for MCM-41 and SBA-15 supports, respectively at 75°C and 15/85% CO2/N2) is reached, after which over-loading of the amines led to the decreasing of capacities as well as the adsorption kinetics. It is believed that under extreme high amine loadings, all the pores are filled and the outer surface of SiO2 will be coated by the sticky amines, leading to limited accessibility of the bulk amine molecules toward CO2 as illustrated in Figure 8 (Xu et al., 2003). According to this model, the maximum amount of amines that a SiO2 support can accommodate depends upon its pore volume, and this hypothesis has been verified by experimental observations that when the amount of the used amine is larger than that calculated according to the pore volume of the support and the density of amine, the resultant adsorbent appears to be sticky and gel-like. The high viscosity of the organic amines will also cause difficulties in CO2 diffusion from the surface to the bulk amines, therefore a two-stage adsorption kinetics was observed (a fast surface reaction stage followed by a slower diffusion-controlling stage; Wang and Song, 2012; Chen et al., 2013), and when adsorption temperature was considered, it appears that rather than lower temperatures, the highest capacities were typically obtained at 75°C so as to get rid of the diffusion barrier.
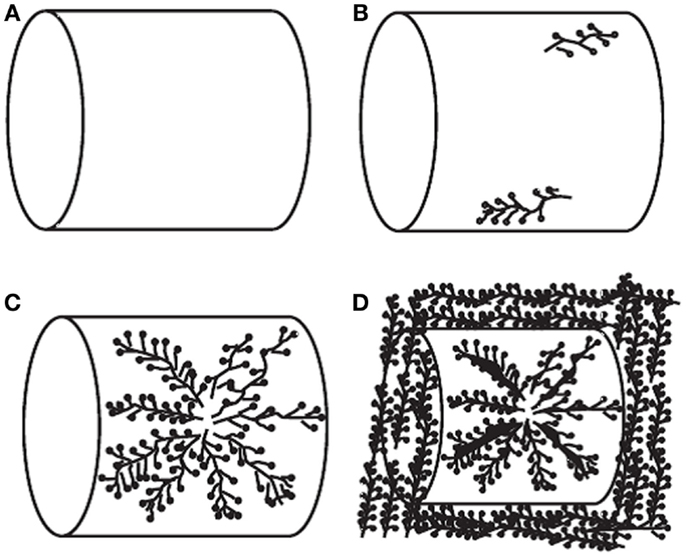
Figure 8. Schematic diagram of PEI loaded in the mesoporous molecular sieve of MCM-41. (A) MCM-41 support; (B) low PEI loading; (C) high PEI loading; (D) extremely high PEI loading, reproduced from Ma et al. (2009).
The effectiveness of larger pore volumes in promoting the performance of the adsorbents was further evidenced by comparing materials including MCM-41, MCM-48, SBA-15, SBA-16, KIT-6, and two hexagonal mesoporous silicas by Ahn et al. (Son et al., 2008; Chen et al., 2010). Based on similar principle, commercialized fumed silica with large pore volumes (>1 cm3/g) was also tested to support PEI as CO2 adsorbents (Goeppert et al., 2010; Zhang et al., 2014a,b). Recently, Giannelis and co-workers prepared a silica foam with ultra-large mesopores (1.46 cm3/g) so that 80 wt. % PEI can be loaded, a high uptake of ca. 25.5 wt. % (75°C and 1 bar) with fast adsorption kinetics was observed (Qi et al., 2012). Similar capacities were achieved from the same group by using mesoporous SiO2 capsules as a support (Qi et al., 2011), the measured pore volume of the capsules was not high, however, the hollow cores provided abundant space for the accommodation of amines, leading to the exceptionally high adsorption capacities.
Effect of amines
Amine groups are the main adsorption sites and, therefore, the type of the amine is another decisive factor in determining the performance of the adsorbents. The most studied amines for impregnating SiO2 support are tetraethylenepentamine (TEPA) and PEIs with different molecular weights. Basically, owing to the higher concentration of amine groups, larger amounts of primary amine, and lower viscosity, higher capacities can be obtained by using TEPA as compared with that of PEIs. However, the cyclic stability of using TEPA is questionable due to its lower thermal stability which led to amine loss during desorption at elevated temperatures (Chen et al., 2009; Liu et al., 2010; Qi et al., 2011). A similar theory can be applied to linear PEI as well. In contrast, in spite of the relatively lower adsorption capacity, PEIs with higher molecular mass or a branched structure possessed better thermal stability leading to a stable performance during multi-adsorption/desorption cycles (Goeppert et al., 2010).
Non-SiO2 support
Comparable adsorption capacities have also been reported using supports other than SiO2 (Wang et al., 2011c, 2012; Chen et al., 2013). For example, low-cost carbons were used by Song et al. to prepare the “molecular basket” adsorbents, and similar performance could be achieved as compared with the use of SBA-15 (Wang et al., 2011c). More importantly, when the volume-based adsorption capacity was compared, those adsorbents using carbon as support are even better (Wang et al., 2012). When synthesized mesoporous alumina was used as a support for PEI, an enhanced stability was observed under direct contact with steam, indicating the feasibility of this support for the use in practical CO2 capture applications (Chaikittisilp et al., 2011a).
Introduction of Amines by Grafting (Class 2)
Class 2 immobilized amine adsorbents are those materials where the amines are covalently bonded with the framework of a “supporting” material. They are typically prepared by surface grafting based on the reaction between silanol groups on the surface of SiO2 and the alkoxy groups in an aminosilane molecule.
A number of papers on Class 2 adsorbents are contributed from Sayari’s group based on a pore-expended MCM-41 support, by grafting either a monoamine silane or triamine silane, the materials achieved CO2 capacities of 9.9 and 12.3 wt. % at 0.15 and 1 bar (25°C), respectively (Serna-Guerrero et al., 2008, 2010), and the materials can be used in a variety of environments (Belmabkhout et al., 2010). Other mesoporous silicas that have been tested for the preparation of Class 2 adsorbents include SBA-12, SBA-15, MCM-41, MCM-22, MCM-36, ITQ-2, ITQ-6, and silica microspheres (Zelenak et al., 2008; Zukal et al., 2009; Belmabkhout et al., 2010; Serna-Guerrero et al., 2010; Yang et al., 2012). In contrast to Class 1 adsorbents, pore volumes of the supports for Class 2 are less important, and it was reported that the effectiveness of these materials is influenced by the surface area, pore diameter, pore connectivity, and most importantly, the density of the surface silanol groups. This is expectable since the maximum amount of aminosilane that can be grafted onto SiO2 is determined by the amount of silanol groups existing on the surface. A good demonstration of this relationship was presented by Yang et al., where the density of surface silanol groups was tuned by using different template removal procedures, and higher amine loadings can be achieved on the sample with more silanol groups, resulting in a higher CO2 adsorption capacity and selectivity (Wang and Yang, 2011).
It is necessary to mention here that, although several authors have tried to enhance the grafting process by surface pre-treatment (Harlick and Sayari, 2007; Zhao et al., 2010), the amount of amine groups that can be introduced is still low as compared with Class 1 adsorbents, leading to a substantially lower adsorption capacities which represents a major disadvantage of this type of adsorbents. Merits of Class 2 adsorbents are obvious such as the better-preserved textural properties of the supporting framework, and more importantly, since the amines are covalently bonded with SiO2, their interactions with the support are stronger, leading to a better stability as compared with Class 1 adsorbents.
Class 3
Class 3 immobilized amine adsorbents are characterized by the in situ polymerization of amines inside the pores of a silica material. Chaffee and co-workers used a stepwise growth strategy to induce melamine-based dendrimers into the pores of SiO2, with up to ca. 55 wt. % organic loadings could be achieved (fourth generation) leading to a substantially higher CO2 adsorption (4.0 wt. % at 20°C and 90% CO2–10% Ar) as compared with the bare support (Liang et al., 2008, 2009). Jones et al. reported a hyperbranched aminosilica (HAS) material for CO2 capture that was synthesized by surface polymerization of aziridine initiated by the silanols on the surface of SBA-15, the resultant adsorbent exhibited a CO2 uptake of ca. 8.8 wt. % at 75°C in a wet 10% CO2–90% Ar stream (Hicks et al., 2008; Drese et al., 2009). Later, an investigation into the effect of support on the polymerization of aziridine was carried out by the same group, but higher loadings could not be achieved by using pore-expanded SiO2 materials, revealing that fabricating and controlling of the in situ polymerization may be more complicated than expected (Drese et al., 2012). Another example of the Class 3 adsorbents was synthesized by in situ ring-opening polymerization of Z-protected lysine N-carboxyanhydride also by Jones’ group (Chaikittisilp et al., 2011b).
Compared to Class 1 and 2 adsorbents, it was claimed that the Class 3 counterpart combined the advantages of the first two types, namely increased amount of amine groups with covalent bonding with the support, leading to a thermal stable adsorbents with higher adsorption capacities (Hicks et al., 2008; Drese et al., 2009, 2012; Liang et al., 2009). However, it seems that the actual CO2 uptakes of Class 3 adsorbents are relatively low and, therefore, further increasing the loading and/or the efficiency of the in situ formed polymers should be addressed in future works.
Degradation and Rejuvenation of Immobilized Amine Adsorbents
In most of the literatures, a gradual decreasing of adsorption capacity of the immobilized amine adsorbents has been observed owing to thermal and/or oxidative degradation (Heydari-Gorji and Sayari, 2012). According to Sayari et al., formation of urea-like compounds in dry capturing conditions caused irreversible adsorption and finally leading to the deactivation of the adsorbents, the deactivated adsorbents can be fully recovered by treatment in steam-containing gases, and thus using wet CO2 as a purge gas could provide opportunities to stabilized separation of CO2 (Sayari and Belmabkhout, 2010; Sayari et al., 2012). More recently, an in-depth investigation on the oxidative degradation was reported by the same group, C = O and –CH = N– species were conclusively identified by 1D and 2D NMR techniques (Ahmadalinezhad and Sayari, 2014). Nevertheless, the mechanism of amine degradation induced by different factors remains unclarified, and it is of particular importance to move to a further step, namely how the deactivated amines can be rejuvenated, this is also important to liquid amine scrubbing technologies since the degradation of solvents represents a major contributor to the entire costs of the process.
To conclude, immobilization of organic amines results in a series of adsorbents particularly suited to low (partial) pressure CO2 capture due to the chemical adsorption that occurs, which makes these materials the most suitable candidates for flue gas treatment. Therefore, significant research efforts have been devoted to this area. Currently, all three classes of adsorbents have their own pros and cons, and further innovations and solutions are still needed. Furthermore, the degradation of amines in practical CO2 capture conditions was an universal observation for all the three classes of adsorbents and understanding the involved mechanisms together with further treatments to mitigate degradation are important.
Concluding Remarks and Overall Perspectives
CO2 capture is an indispensable component for CCUS. Based on our analysis however, the deployment of CO2 capture goes far beyond CCUS and, in the future, it will connect the CO2 emission and recycling sectors, leading to a profitable near carbon-zero or even carbon-negative energy–chemical production and consumption networks. Thus, highly efficient CO2 capture processes, such as using solid adsorbents and others (such as chemical looping, oxy-fuel), are the enablers for integrated energy–chemical solutions with higher efficiencies. In this review, the recent advances for adsorbents for low-temperature (<100°C) CO2 capture have been covered, namely carbon-based adsorbents and amine immobilized adsorbents (Table 5).
• Carbons are one of the most widely used adsorbents, which also occurred to be one of the options for CO2 capture. Many researches have been reported focusing on the investigations of (i) selecting proper precursors for the preparation of carbons and (ii) involving hetero-atoms to enhance the surface affinity toward CO2. Encouraging results have emerged and these have established the competence for scaling-up the production of carbons for practical capture applications, and further works on improving the capacity at low partial pressures and preparation of carbons from low-cost precursors are necessary.
• Immobilized amines are characterized by their high capacities at low (partial) pressures, this feature makes them a promising candidate for CO2 capture from flue gas. Based on the preparation strategies, these adsorbents can be categorized into three classes, namely (i) those prepared from the physical deposition/combination of pre-synthesized amine onto SiO2 (Class 1), (ii) those materials with covalent bonds between the amines and the SiO2 support (Class 2), and (iii) those prepared by the in situ polymerization of reactive amine monomers on and in the silica support. All the three classes of adsorbents have their own pros and cons, further promotion of the capacities (for Class 2 and 3), kinetics (for Class 1), and stabilities (for all three classes) are needed. To this end, understanding the mechanisms of surface adsorption reaction and pathways for the thermal/oxidative degradation is of vital importance.
Overall, the development of effective adsorbents for CO2 capture remains challenging despite the significant R&D activities already happened. By taking practical conditions under different circumstances for CO2 capture into consideration, further innovations in the design and synthesis of adsorbents are needed to identify more efficient materials to balance the costs and engineering feasibilities of different capture processes. Further, the importance of CO2 capture to enable future integrated energy and chemical production processes needs to be re-assessed to drive forward the development of solid adsorbent technology.
Conflict of Interest Statement
The authors declare that the research was conducted in the absence of any commercial or financial relationships that could be construed as a potential conflict of interest.
Acknowledgments
The authors wish to acknowledge the financial support from the National Natural Science Foundation of China (21203230).
References
Ahmadalinezhad, A., and Sayari, A. (2014). Oxidative degradation of silica-supported polyethylenimine for CO2 adsorption: insights into the nature of deactivated species. Phys. Chem. Chem. Phys. 16, 1529–1535. doi:10.1039/c3cp53928h
Pubmed Abstract | Pubmed Full Text | CrossRef Full Text | Google Scholar
An, H., Feng, B., and Su, S. (2009). CO2 capture capacities of activated carbon fibre-phenolic resin composites. Carbon N. Y. 47, 2396–2405. doi:10.1016/j.carbon.2009.04.029
Bara, J. E., Camper, D. E., Gin, D. L., and Noble, R. D. (2010). Room-temperature ionic liquids and composite materials: platform technologies for CO2 capture. Acc. Chem. Res. 43, 152–159. doi:10.1021/ar9001747
Pubmed Abstract | Pubmed Full Text | CrossRef Full Text | Google Scholar
Belmabkhout, Y., Serna-Guerrero, R., and Sayari, A. (2010). Adsorption of CO2-containing gas mixtures over amine-bearing pore-expanded MCM-41 silica: application for gas purification. Ind. Eng. Chem. Res. 49, 359–365. doi:10.1021/ie900837t
Ben, T., Li, Y. Q., Zhu, L. K., Zhang, D. L., Cao, D. P., Xiang, Z. H., et al. (2012). Selective adsorption of carbon dioxide by carbonized porous aromatic framework (PAF). Energy Environ. Sci. 5, 8370–8376. doi:10.1039/c2ee21935b
Ben, T., Ren, H., Ma, S., Cao, D., Lan, J., Jing, X., et al. (2009). Targeted synthesis of a porous aromatic framework with high stability and exceptionally high surface area. Angew. Chem. Int. Ed. 48, 9457–9460. doi:10.1002/anie.200904637
Blamey, J., Anthony, E. J., Wang, J., and Fennell, P. S. (2010). The calcium looping cycle for large-scale CO2 capture. Prog. Energy Combust. Sci. 36, 260–279. doi:10.1016/j.pecs.2009.10.001
Bollini, P., Didas, S. A., and Jones, C. W. (2011). Amine-oxide hybrid materials for acid gas separations. J. Mater. Chem. 21, 15100–15120. doi:10.1039/c1jm12522b
Boot-Handford, M. E., Abanades, J. C., Anthony, E. J., Blunt, M. J., Brandani, S., Mac Dowell, N., et al. (2014). Carbon capture and storage update. Energy Environ. Sci. 7, 130–189. doi:10.1039/c3ee42350f
Pubmed Abstract | Pubmed Full Text | CrossRef Full Text | Google Scholar
Bradley, R. H. (2011). Recent developments in the physical adsorption of toxic organic vapours by activated carbons. Adsorpt. Sci. Technol. 29, 1–28. doi:10.1260/0263-6174.29.1.1
Budzianowski, W. M. (2012). Negative carbon intensity of renewable energy technologies involving biomass or carbon dioxide as inputs. Renew. Sustain. Energ. Rev. 16, 6507–6521. doi:10.1016/j.rser.2012.08.016
Cazorla-Amoros, D., Alcaniz-Monge, J., and Linares-Solano, A. (1996). Characterization of activated carbon fibers by CO2 adsorption. Langmuir 12, 2820–2824. doi:10.1021/la960022s
Chaikittisilp, W., Kim, H. J., and Jones, C. W. (2011a). Mesoporous alumina-supported amines as potential steam-stable adsorbents for capturing CO2 from simulated flue gas and ambient air. Energy Fuel 25, 5528–5537. doi:10.1021/ef201224v
Chaikittisilp, W., Lunn, J. D., Shantz, D. F., and Jones, C. W. (2011b). Poly(L-lysine) brush-mesoporous silica hybrid material as a biomolecule-based adsorbent for CO2 capture from simulated flue gas and air. Chem. Eur. J. 17, 10556–10561. doi:10.1002/chem.201101480
Chandra, V., Yu, S. U., Kim, S. H., Yoon, Y. S., Kim, D. Y., Kwon, A. H., et al. (2012). Highly selective CO2 capture on N-doped carbon produced by chemical activation of polypyrrole functionalized graphene sheets. Chem. Commun. 48, 735–737. doi:10.1039/c1cc15599g
Pubmed Abstract | Pubmed Full Text | CrossRef Full Text | Google Scholar
Chen, C., Kim, J., and Ahn, W. S. (2012). Efficient carbon dioxide capture over a nitrogen-rich carbon having a hierarchical micro-mesopore structure. Fuel 95, 360–364. doi:10.1016/j.fuel.2011.10.072
Chen, C., Son, W. J., You, K. S., Ahn, J. W., and Ahn, W. S. (2010). Carbon dioxide capture using amine-impregnated HMS having textural mesoporosity. Chem. Eng. J. 161, 46–52. doi:10.1016/j.cej.2010.04.019
Chen, C., Yang, S. T., Ahn, W. S., and Ryoo, R. (2009). Amine-impregnated silica monolith with a hierarchical pore structure: enhancement of CO2 capture capacity. Chem. Commun. 24, 3627–3629. doi:10.1039/b905589d
Pubmed Abstract | Pubmed Full Text | CrossRef Full Text | Google Scholar
Chen, Z. H., Deng, S. B., Wei, H. R., Wang, B., Huang, J., and Yu, G. (2013). Polyethylenimine-impregnated resin for high CO2 adsorption: an efficient adsorbent for CO2 capture from simulated flue gas and ambient air. ACS Appl. Mater. Interfaces 5, 6937–6945. doi:10.1021/am400661b
Pubmed Abstract | Pubmed Full Text | CrossRef Full Text | Google Scholar
Choi, S., Drese, J. H., and Jones, C. W. (2009). Adsorbent materials for carbon dioxide capture from large anthropogenic point sources. ChemSusChem 2, 796–854. doi:10.1002/cssc.200900036
Pubmed Abstract | Pubmed Full Text | CrossRef Full Text | Google Scholar
Chu, S., and Majumdar, A. (2012). Opportunities and challenges for a sustainable energy future. Nature 488, 294–303. doi:10.1038/nature11475
Pubmed Abstract | Pubmed Full Text | CrossRef Full Text | Google Scholar
CO2Now.org. (2014). Available at: http://co2now.org/
Crowley, T. J. (2000). Causes of climate change over the past 1000 years. Science 289, 270–277. doi:10.1126/science.289.5477.270
Pubmed Abstract | Pubmed Full Text | CrossRef Full Text | Google Scholar
Davis, M. E. (2002). Ordered porous materials for emerging applications. Nature 417, 813–821. doi:10.1038/nature00785
Pubmed Abstract | Pubmed Full Text | CrossRef Full Text | Google Scholar
Dawson, R., Cooper, A. I., and Adams, D. J. (2012). Nanoporous organic polymer networks. Prog. Polym. Sci. 37, 530–563. doi:10.1016/j.progpolymsci.2011.09.002
de Souza, L. K. C., Wickramaratne, N. P., Ello, A. S., Costa, M. J. F., da Costa, C. E. F., and Jaroniec, M. (2013). Enhancement of CO2 adsorption on phenolic resin-based mesoporous carbons by KOH activation. Carbon N. Y. 65, 334–340. doi:10.1016/j.carbon.2013.08.034
Donat, F., Florin, N. H., Anthony, E. J., and Fennell, P. S. (2012). Influence of high-temperature steam on the reactivity of CaO sorbent for CO2 capture. Environ. Sci. Technol. 46, 1262–1269. doi:10.1021/es202679w
Pubmed Abstract | Pubmed Full Text | CrossRef Full Text | Google Scholar
Drage, T. C., Arenillas, A., Smith, K. M., Pevida, C., Piippo, S., and Snape, C. E. (2007). Preparation of carbon dioxide adsorbents from the chemical activation of urea-formaldehyde and melamine-formaldehyde resins. Fuel 86, 22–31. doi:10.1016/j.fuel.2006.07.003
Drese, J. H., Choi, S., Didas, S. A., Bollini, P., Gray, M. L., and Jones, C. W. (2012). Effect of support structure on CO2 adsorption properties of pore-expanded hyperbranched aminosilicas. Microporous Mesoporous Mater. 151, 231–240. doi:10.1016/j.micromeso.2011.10.031
Drese, J. H., Choi, S., Lively, R. P., Koros, W. J., Fauth, D. J., Gray, M. L., et al. (2009). Synthesis-structure-property relationships for hyperbranched aminosilica CO2 adsorbents. Adv. Funct. Mater. 19, 3821–3832. doi:10.1002/adfm.200901461
Du, N. Y., Park, H. B., Dal-Cin, M. M., and Guiver, M. D. (2012). Advances in high permeability polymeric membrane materials for CO2 separations. Energy Environ. Sci. 5, 7306–7322. doi:10.1039/c1ee02668b
Garcia, S., Pis, J. J., Rubiera, F., and Pevida, C. (2013). Predicting mixed-gas adsorption equilibria on activated carbon for precombustion CO2 capture. Langmuir 29, 6042–6052. doi:10.1021/la4004998
Pubmed Abstract | Pubmed Full Text | CrossRef Full Text | Google Scholar
Goeppert, A., Meth, S., Prakash, G. K. S., and Olah, G. A. (2010). Nanostructured silica as a support for regenerable high-capacity organoamine-based CO2 sorbents. Energy Environ. Sci. 3, 1949–1960. doi:10.1039/c0ee00136h
Graves, C., Ebbesen, S. D., Mogensen, M., and Lackner, K. S. (2011). Sustainable hydrocarbon fuels by recycling CO2 and H2O with renewable or nuclear energy. Renew. Sustain. Energ. Rev. 15, 1–23. doi:10.1016/j.rser.2010.07.014
Hao, G. P., Li, W. C., Qian, D., and Lu, A. H. (2010). Rapid synthesis of nitrogen-doped porous carbon monolith for CO2 capture. Adv. Mater. 22, 853–857. doi:10.1002/adma.200903765
Hao, G. P., Li, W. C., Qian, D., Wang, G. H., Zhang, W. P., Zhang, T., et al. (2011). Poly(benzoxazine-co-resol)-based porous carbon monoliths and their application as high-performance CO2 capture sorbents. J. Am. Chem. Soc. 133, 11378–11388. doi:10.1021/ja203857g
Pubmed Abstract | Pubmed Full Text | CrossRef Full Text | Google Scholar
Harlick, P. J. E., and Sayari, A. (2007). Applications of pore-expanded mesoporous silica. 5. Triamine grafted material with exceptional CO2 dynamic and equilibrium adsorption performance. Ind. Eng. Chem. Res. 46, 446–458. doi:10.1021/ie060774+
Held, I. M., and Soden, B. J. (2000). Water vapor feedback and global warming. Annu. Rev. Energy Environ. 25, 441–475. doi:10.1146/annurev.energy.25.1.441
Heydari-Gorji, A., and Sayari, A. (2012). Thermal, oxidative, and CO2-induced degradation of supported polyethylenimine adsorbents. Ind. Eng. Chem. Res. 51, 6887–6894. doi:10.1021/ie3003446
Hicks, J. C., Drese, J. H., Fauth, D. J., Gray, M. L., Qi, G. G., and Jones, C. W. (2008). Designing adsorbents for CO2 capture from flue gas-hyperbranched aminosilicas capable of capturing CO2 reversibly. J. Am. Chem. Soc. 130, 2902–2903. doi:10.1021/ja077795v
Hoffert, M. I., Caldeira, K., Jain, A. K., Haites, E. F., Harvey, L. D. D., Potter, S. D., et al. (1998). Energy implications of future stabilization of atmospheric CO2 content. Nature 395, 881–884. doi:10.1038/27638
Hu, X., Radosz, M., Cychosz, K. A., and Thommes, M. (2011). CO2-filling capacity and selectivity of carbon nanopores: synthesis, texture, and pore-size distribution from quenched-solid density functional theory (QSDFT). Environ. Sci. Technol. 45, 7068–7074. doi:10.1021/es200782s
Pubmed Abstract | Pubmed Full Text | CrossRef Full Text | Google Scholar
Jang, D. I., and Park, S. J. (2012). Influence of nickel oxide on carbon dioxide adsorption behaviors of activated carbons. Fuel 102, 439–444. doi:10.1016/j.fuel.2012.03.052
Jones, C. W. (2011). CO2 capture from dilute gases as a component of modern global carbon management. Annu. Rev. Chem. Biomol. Eng. 2, 31–52. doi:10.1146/annurev-chembioeng-061010-114252
Pubmed Abstract | Pubmed Full Text | CrossRef Full Text | Google Scholar
Kadib, A. E., Chimenton, R., Sachse, A., Fajula, F., Galarneau, A., and Coq, B. (2009). Functionalized inorganic monolithic microreactors for high productivity in fine chemicals catalytic synthesis. Angew. Chem. Int. Ed. 48, 4969–4972. doi:10.1002/anie.200805580
Pubmed Abstract | Pubmed Full Text | CrossRef Full Text | Google Scholar
Kalyani, P., and Anitha, A. (2013). Biomass carbon & its prospects in electrochemical energy systems. Int. J. Hydrogen Energy 38, 4034–4045. doi:10.1016/j.ijhydene.2013.01.048
Karadas, F., Atilhan, M., and Aparicio, S. (2010). Review on the use of ionic liquids (ILs) as alternative fluids for CO2 capture and natural gas sweetening. Energy Fuel 24, 5817–5828. doi:10.1021/ef1011337
Kaya, Y. (1995). The role of CO2 removal and disposal. Energy Convers. Manag. 36, 375–380. doi:10.1016/0196-8904(95)00025-9
Kim, B. J., Cho, K. S., and Park, S. J. (2012). Copper oxide-decorated porous carbons for carbon dioxide adsorption behaviors. J. Colloid Interface Sci. 342, 575–578. doi:10.1016/j.jcis.2009.10.045
Pubmed Abstract | Pubmed Full Text | CrossRef Full Text | Google Scholar
Knofel, C., Martin, C., Hornebecq, V., and Llewellyn, P. L. (2009). Study of carbon dioxide adsorption on mesoporous aminopropylsilane-functionalized silica and titania combining microcalorimetry and in situ infrared spectroscopy. J. Phys. Chem. C 113, 21726–21734. doi:10.1021/jp907054h
Kumar, P., Barrett, D. M., Delwiche, M. J., and Stroeve, P. (2009). Methods for pretreatment of lignocellulosic biomass for efficient hydrolysis and biofuel production. Ind. Eng. Chem. Res. 48, 3713–3729. doi:10.1021/ie801542g
Li, F. X., and Fan, L. S. (2008). Clean coal conversion processes – progress and challenges. Energy Environ. Sci. 1, 248–267. doi:10.1039/b809218b
Li, Q., Yang, J. P., Feng, D., Wu, Z. X., Wu, Q. L., Park, S. S., et al. (2010). Facile synthesis of porous carbon nitride spheres with hierarchical three-dimensional mesostructures for CO2 capture. Nano Res. 3, 632–642. doi:10.1007/s12274-010-0023-7
Liang, Z., Fadhel, B., Schneider, C. J., and Chaffee, A. L. (2008). Stepwise growth of melamine-based dendrimers into mesopores and their CO2 adsorption properties. Microporous Mesoporous Mater. 111, 536–543. doi:10.1016/j.micromeso.2007.08.030
Liang, Z. J., Fadhel, B., Schneider, C. J., and Chaffee, A. L. (2009). Adsorption of CO2 on mesocellular siliceous foam iteratively functionalized with dendrimers. Adsorption 15, 429–437. doi:10.1007/s10450-009-9192-7
Liu, L., Deng, Q. F., Ma, T. Y., Lin, X. Z., Hou, X. X., Liu, Y. P., et al. (2011). Ordered mesoporous carbons: citric acid-catalyzed synthesis, nitrogen doping and CO2 capture. J. Mater. Chem. 21, 16001–16009. doi:10.1039/c1jm12887f
Liu, Y. M., Shi, J. J., Chen, J., Ye, Q., Pan, H., Shao, Z. H., et al. (2010). Dynamic performance of CO2 adsorption with tetraethylenepentamine-loaded KIT-6. Microporous Mesoporous Mater. 134, 16–21. doi:10.1016/j.micromeso.2010.05.002
Lohe, M. R., Rose, M., and Kaskel, S. (2009). Metal-organic framework (MOF) aerogels with high micro- and macroporosity. Chem. Commun. 40, 6056–6058. doi:10.1039/b910175f
Pubmed Abstract | Pubmed Full Text | CrossRef Full Text | Google Scholar
Ma, T. Y., Liu, L., and Yuan, Z. Y. (2013). Direct synthesis of ordered mesoporous carbons. Chem. Soc. Rev. 42, 3977–4003. doi:10.1039/c2cs35301f
Pubmed Abstract | Pubmed Full Text | CrossRef Full Text | Google Scholar
Ma, X. L., Wang, X. X., and Song, C. S. (2009). “Molecular basket” sorbents for separation of CO2 and H2S from various gas streams. J. Am. Chem. Soc. 131, 5777–5783. doi:10.1021/ja8074105
Pubmed Abstract | Pubmed Full Text | CrossRef Full Text | Google Scholar
MacDowell, N., Florin, N., Buchard, A., Hallett, J., Galindo, A., Jackson, G., et al. (2010). An overview of CO2 capture technologies. Energy Environ. Sci. 3, 1645–1669. doi:10.1039/c004106h
Markewitz, P., Kuckshinrichs, W., Leitner, W., Linssen, J., Zapp, P., Bongartz, R., et al. (2012). Worldwide innovations in the development of carbon capture technologies and the utilization of CO2. Energy Environ. Sci. 5, 7281–7305. doi:10.1039/c2ee03403d
Martin, C. F., Plaza, M. G., Garcia, S., Pis, J. J., Rubiera, F., and Pevida, C. (2011). Microporous phenol-formaldehyde resin-based adsorbents for pre-combustion CO2 capture. Fuel 90, 2064–2072. doi:10.1016/j.fuel.2011.01.019
Martin-Martinez, J. M., Torregrosa-Macia, R., and Mittelmeijer-Hazeleger, M. C. (1995). Mechanisms of adsorption of CO2 in the micropores of activated anthracite. Fuel 74, 111–114. doi:10.1016/0016-2361(94)P4340-8
Mikkelsen, M., Jorgensen, M., and Krebs, F. C. (2010). The teraton challenge. A review of fixation and transformation of carbon dioxide. Energy Environ. Sci. 3, 43–81. doi:10.1039/b912904a
Mohan, D., and Pittman, C. U. (2006). Activated carbons and low cost adsorbents for remediation of tri- and hexavalent chromium from water. J. Hazard. Mater. 137, 762–811. doi:10.1016/j.jhazmat.2006.06.060
Pubmed Abstract | Pubmed Full Text | CrossRef Full Text | Google Scholar
Nandi, M., Okada, K., Dutta, A., Bhaumik, A., Maruyama, J., Derks, D., et al. (2012). Unprecedented CO2 uptake over highly porous N-doped activated carbon monoliths prepared by physical activation. Chem. Commun. 48, 10283–10285. doi:10.1039/c2cc35334b
Pubmed Abstract | Pubmed Full Text | CrossRef Full Text | Google Scholar
Panwar, N. L., Kaushik, S. C., and Kothari, S. (2011). Role of renewable energy sources in environmental protection: a review. Renew. Sustain. Energ. Rev. 15, 1513–1524. doi:10.1016/j.rser.2010.11.037
Patino, J., Gutierrez, M. C., Carriazo, D., Ania, C. O., Parra, J. B., Ferrer, M. L., et al. (2012). Deep eutectic assisted synthesis of carbon adsorbents highly suitable for low-pressure separation of CO2-CH4 gas mixtures. Energy Environ. Sci. 5, 8699–8707. doi:10.1039/c2ee22029f
Patz, J. A., Campbell-Lendrum, D., Holloway, T., and Foley, J. A. (2005). Impact of regional climate change on human health. Nature 438, 310–317. doi:10.1038/nature04188
Pubmed Abstract | Pubmed Full Text | CrossRef Full Text | Google Scholar
Pevida, C., Drage, T. C., and Snape, C. E. (2008). Silica-templated melamine-formaldehyde resin derived adsorbents for CO2 capture. Carbon N. Y. 46, 1464–1474. doi:10.1016/j.carbon.2008.06.026
Plaza, M. G., Pevida, C., Arias, B., Fermoso, J., Casal, M. D., Martin, C. F., et al. (2009). Development of low-cost biomass-based adsorbents for postcombustion CO2 capture. Fuel 88, 2442–2447. doi:10.1016/j.fuel.2009.02.025
Presser, V., McDonough, J., Yeon, S. H., and Gogotsi, Y. (2011). Effect of pore size on carbon dioxide sorption by carbide derived carbon. Energy Environ. Sci. 4, 3059–3066. doi:10.1039/c1ee01176f
Przepiorski, J., Skrodzewicz, M., and Morawski, A. W. (2004). High temperature ammonia treatment of activated carbon for enhancement of CO2 adsorption. Appl. Surf. Sci. 225, 235–242. doi:10.1016/j.apsusc.2003.10.006
Qi, G. G., Fu, L. L., Choi, B. H., and Giannelis, E. P. (2012). Efficient CO2 sorbents based on silica foam with ultra-large mesopores. Energy Environ. Sci. 5, 7368–7375. doi:10.1039/c2ee21394j
Qi, G. G., Wang, Y. B., Estevez, L., Duan, X. N., Anako, N., Park, A. H. A., et al. (2011). High efficiency nanocomposite sorbents for CO2 capture based on amine-functionalized mesoporous capsules. Energy Environ. Sci. 4, 444–452. doi:10.1039/c0ee00213e
Ren, X. M., Chen, C. L., Nagatsu, M., and Wang, X. K. (2011). Carbon nanotubes as adsorbents in environmental pollution management: a review. Chem. Eng. J. 170, 395–410. doi:10.1016/j.cej.2010.08.045
Ridha, F. N., Manovic, V., Wu, Y. H., Macchi, A., and Anthony, E. J. (2013). Pelletized CaO-based sorbents treated with organic acids for enhanced CO2 capture in Ca-looping cycles. Int. J. Greenhouse Gas Control 17, 357–365. doi:10.1016/j.ijggc.2013.05.009
Rochelle, G. T. (2009). Amine scrubbing for CO2 capture. Science 325, 1652–1654. doi:10.1126/science.1176731
Pubmed Abstract | Pubmed Full Text | CrossRef Full Text | Google Scholar
Sangeeta, Moka, S., Pande, M., Rani, M., Gakhar, R., Sharma, M., et al. (2014). Alternative fuels: an overview of current trends and scope for future. Renew. Sustain. Energ. Rev. 32, 697–712. doi:10.1016/j.rser.2014.01.023
Sayari, A., and Belmabkhout, Y. (2010). Stabilization of amine-containing CO2 adsorbents: dramatic effect of water vapor. J. Am. Chem. Soc. 132, 6312–6314. doi:10.1021/ja1013773
Pubmed Abstract | Pubmed Full Text | CrossRef Full Text | Google Scholar
Sayari, A., Belmabkhout, Y., and Serna-Guerrero, R. (2011). Flue gas treatment via CO2 adsorption. Chem. Eng. J. 171, 760–774. doi:10.1016/j.cej.2011.02.007
Sayari, A., Heydari-Gorji, A., and Yang, Y. (2012). CO2-induced degradation of amine-containing adsorbents: reaction products and pathways. J. Am. Chem. Soc. 134, 13834–13842. doi:10.1021/ja304888a
Pubmed Abstract | Pubmed Full Text | CrossRef Full Text | Google Scholar
Seema, H., Kemp, K. C., Le, N. H., Park, S. W., Chandra, V., Lee, J. W., et al. (2014). Highly selective CO2 capture by S-doped microporous carbon materials. Carbon N. Y. 66, 320–326. doi:10.1016/j.carbon.2013.09.006
Serna-Guerrero, R., Belmabkhout, Y., and Sayari, A. (2010). Further investigations of CO2 capture using triamine-grafted pore-expanded mesoporous silica. Chem. Eng. J. 158, 513–519. doi:10.1016/j.cej.2010.01.041
Serna-Guerrero, R., Da’na, E., and Sayari, A. (2008). New insights into the interactions of CO2 with amine-functionalized silica. Ind. Eng. Chem. Res. 47, 9406–9412. doi:10.1021/ie801186g
Sevilla, M., and Fuertes, A. B. (2011). Sustainable porous carbons with a superior performance for CO2 capture. Energy Environ. Sci. 4, 1765–1771. doi:10.1039/c0ee00784f
Sevilla, M., and Mokaya, R. (2014). Energy storage applications of activated carbons: supercapacitors and hydrogen storage. Energy Environ. Sci. 7, 1250–1280. doi:10.1002/cssc.201300295
Pubmed Abstract | Pubmed Full Text | CrossRef Full Text | Google Scholar
Sevilla, M., Valle-Vigon, P., and Fuertes, A. B. (2011). N-doped polypyrrole-based porous carbons for CO2 capture. Adv. Funct. Mater. 21, 2781–2787. doi:10.1002/adfm.201100291
Shafeeyan, M. S., Daud, W. M. A. W., Houshmand, A., and Arami-Niya, A. (2011). Ammonia modification of activated carbon to enhance carbon dioxide adsorption: effect of pre-oxidation. Appl. Surf. Sci. 257, 3936–3942. doi:10.1016/j.apsusc.2010.11.127
Shafeeyan, M. S., Daud, W. M. A. W., Houshmand, A., and Shamiri, A. (2010). A review on surface modification of activated carbon for carbon dioxide adsorption. J. Anal. Appl. Pyrolysis 89, 143–151. doi:10.1016/j.jaap.2010.07.006
Shao, X. H., Feng, Z. H., Xue, R. S., Ma, C. C., Wang, W. C., Peng, X., et al. (2011). Adsorption of CO2, CH4, CO2/N2 and CO2/CH4 in novel activated carbon beads: preparation, measurements and simulation. AIChE J. 57, 3042–3051. doi:10.1002/aic.12515
Shen, W. Z., Zhang, S. C., He, Y., Li, J. F., and Fan, W. B. (2011). Hierarchical porous polyacrylonitrile-based activated carbon fibers for CO2 capture. J. Mater. Chem. 21, 14036–14040. doi:10.1039/c1jm12585k
Shi, L., Yang, G. H., Tao, K., Yoneyama, Y., Tan, Y. S., and Tsubaki, N. (2013). An introduction of CO2 conversion by dry reforming with methane and new route of low-temperature methanol synthesis. Acc. Chem. Res. 46, 1838–1847. doi:10.1021/ar300217j
Pubmed Abstract | Pubmed Full Text | CrossRef Full Text | Google Scholar
Siglinda, P., and Centi, G. (2014). CO2 Recycling: a key strategy to introduce green energy in the chemical production chain. ChemSusChem 7, 1274–1282. doi:10.1002/cssc.201300926
Pubmed Abstract | Pubmed Full Text | CrossRef Full Text | Google Scholar
Silvestre-Albero, J., Wahby, A., Sepulveda-Escribano, A., Martinez-Escandell, M., Kaneko, K., and Rodriguez-Reinoso, F. (2011). Ultrahigh CO2 adsorption capacity on carbon molecular sieves at room temperature. Chem. Commun. 47, 6840–6842. doi:10.1039/c1cc11618e
Pubmed Abstract | Pubmed Full Text | CrossRef Full Text | Google Scholar
Son, W. J., Choi, J. S., and Ahn, W. S. (2008). Adsorptive removal of carbon dioxide using polyethyleneimine-loaded mesoporous silica materials. Microporous Mesoporous Mater. 113, 31–40. doi:10.1016/j.jhazmat.2010.11.125
Pubmed Abstract | Pubmed Full Text | CrossRef Full Text | Google Scholar
Song, C. (2006). Global challenges and strategies for control, conversion and utilization of CO2 for sustainable development involving energy, catalysis, adsorption and chemical processing. Catal. Today 115, 2–32. doi:10.1016/j.cattod.2006.02.029
Srinivas, G., Krungleviciute, V., Guo, Z. X., and Yildirim, T. (2014). Exceptional CO2 capture in a hierarchically porous carbon with simultaneous high surface area and pore volume. Energy Environ. Sci. 7, 335–342. doi:10.1039/c3ee42918k
Sumida, K., Rogow, D. L., Mason, J. A., McDonald, T. M., Bloch, E. D., Herm, Z. R., et al. (2012). Carbon dioxide capture in metal-organic frameworks. Chem. Rev. 112, 724–781. doi:10.1021/cr2003272
Sun, N. N., Sun, C. G., Liu, H., Liu, J. J., Stevens, L., Drage, T., et al. (2013). Synthesis, characterization and evaluation of activated spherical carbon materials for CO2 capture. Fuel 113, 854–862. doi:10.1016/j.fuel.2013.03.047
Sunil, D. S., and Merched, A. (2014). A critical review of existing strategies for emission control in the monoethanolamine-based carbon capture process and some recommendations for improved strategies. Fuel 121, 178–188. doi:10.1016/j.fuel.2013.12.023
Swain, P. K., Das, L. M., and Naik, S. N. (2011). Biomass to liquid: a prospective challenge to research and development in 21st century. Renew. Sustain. Energ. Rev. 15, 4917–4933. doi:10.1016/j.rser.2011.07.061
Toftegaard, M. B., Brix, J., Jensen, P. A., Glarborg, P., and Jensen, A. D. (2010). Oxy-fuel combustion of solid fuels. Prog. Energy Combust. Sci. 36, 581–625. doi:10.1016/j.pecs.2010.02.001
Vaidya, P. D., and Kenig, E. Y. (2007). CO2-alkanolamine reaction kinetics: a review of recent studies. Chem. Eng. Technol. 30, 1467–1474. doi:10.1002/ceat.200700268
Vasireddy, S., Morreale, B., Cugini, A., Song, C., and Spivey, J. J. (2011). Clean liquid fuels from direct coal liquefaction: chemistry, catalysis, technological status and challenges. Energy Environ. Sci. 4, 311–345. doi:10.1039/c0ee00097c
Vishnyakov, A., Ravikovitch, P. I., and Neimark, A. V. (1999). Molecular level models for CO2 sorption in nanopores. Langmuir 15, 8736–8742. doi:10.1021/la990726c
Wahby, A., Ramos-Fernandez, J. M., Martinez-Escandell, M., Sepulveda-Escribano, A., Silvestre-Albero, J., and Rodriguez-Reinoso, F. (2010). High-surface-area carbon molecular sieves for selective CO2 adsorption. ChemSusChem 3, 974–981. doi:10.1002/cssc.201000083
Pubmed Abstract | Pubmed Full Text | CrossRef Full Text | Google Scholar
Wang, D. X., Ma, X. L., Sentorun-Shalaby, C., and Song, C. S. (2012). Development of carbon-based “molecular basket” sorbent for CO2 capture. Ind. Eng. Chem. Res. 51, 3048–3057. doi:10.1021/ie2022543
Wang, L. F., and Yang, R. T. (2011). Increasing selective CO2 adsorption on amine-grafted SBA-15 by increasing silanol density. J. Phys. Chem. C 115, 21264–21272. doi:10.1021/jp206976d
Wang, L. F., and Yang, R. T. (2012). Significantly increased CO2 adsorption performance of nanostructured templated carbon by tuning surface area and nitrogen doping. J. Phys. Chem. C 116, 1099–1106. doi:10.1021/jp2100446
Wang, Q., Luo, J., Zhong, Z., and Borgna, A. (2011a). CO2 capture by solid adsorbents and their applications current status and new trends. Energy Environ. Sci. 4, 42–55. doi:10.1039/c0ee00064g
Wang, S. P., Yan, S. L., Ma, X. B., and Gong, J. L. (2011b). Recent advances in capture of carbon dioxide using alkali-metal-based oxides. Energy Environ. Sci. 4, 3805–3819. doi:10.1039/c1ee01116b
Wang, D. X., Sentorun-Shalaby, C., Ma, X. L., and Song, C. S. (2011c). High-capacity and low-cost carbon-based “molecular basket” sorbent for CO2 capture from flue gas. Energy Fuel 25, 456–458. doi:10.1021/ef101364c
Wang, X. X., Schwartz, V., Clark, J. C., Ma, X. L., Overbury, S. H., Xu, X. C., et al. (2009). Infrared study of CO2 sorption over “molecular basket” sorbent consisting of polyethylenimine-modified mesoporous molecular sieve. J. Phys. Chem. C 113, 7260–7268. doi:10.1021/jp809946y
Wang, X. X., and Song, C. S. (2012). Temperature-programmed desorption of CO2 from polyethylenimine-loaded SBA-15 as molecular basket sorbents. Catal. Today 194, 44–52. doi:10.1016/j.cattod.2012.08.008
Wei, H. R., Deng, S. B., Hu, B. Y., Chen, Z. H., Wang, B., Huang, J., et al. (2012). Granular bamboo-derived activated carbon for high CO2 adsorption: the dominant role of narrow micropores. ChemSusChem 5, 2354–2360. doi:10.1002/cssc.201200570
Pubmed Abstract | Pubmed Full Text | CrossRef Full Text | Google Scholar
Wen, J. J., Gu, F. N., Wei, F., Zhou, Y., Lin, W. G., Yang, J., et al. (2010). One-pot synthesis of the amine-modified meso-structured monolith CO2 adsorbent. J. Mater. Chem. 20, 2840–2846. doi:10.1039/b920027d
White, R. J., Budarin, V., Luque, R., Clark, J. H., and Macquarrie, D. J. (2009). Tuneable porous carbonaceous materials from renewable resources. Chem. Soc. Rev. 38, 3401–3418. doi:10.1039/b822668g
Pubmed Abstract | Pubmed Full Text | CrossRef Full Text | Google Scholar
Wickramaratne, N. P., and Jaroniec, M. (2013). Importance of small micropores in CO2 capture by phenolic resin-based activated carbon spheres. J. Mater. Chem. A 1, 112–116. doi:10.1039/c2ta00388k
Williams, J. L. (2001). Monolith structures, materials, properties and uses. Catal. Today 69, 3–9. doi:10.1016/S0920-5861(01)00348-0
Xia, Y. D., Mokaya, R., Walker, G. S., and Zhu, Y. Q. (2011). Superior CO2 adsorption capacity on N-doped, high-surface-area, microporous carbons templated from zeolite. Adv. Energy Mater. 1, 678–683. doi:10.1002/aenm.201100061
Xia, Y. D., Zhu, Y. Q., and Tang, Y. (2012). Preparation of sulfur-doped microporous carbons for the storage of hydrogen and carbon dioxide. Carbon N. Y. 50, 5543–5553. doi:10.1016/j.carbon.2012.07.044
Xu, X. C., Song, C. S., Andresen, J. M., Miller, B. G., and Scaroni, A. W. (2002). Novel polyethylenimine-modified mesoporous molecular sieve of MCM-41 type as high-capacity adsorbent for CO2 capture. Energy Fuel 16, 1463–1469. doi:10.1021/ef020058u
Xu, X. C., Song, C. S., Andresen, J. M., Miller, B. G., and Scaroni, A. W. (2003). Preparation and characterization of novel CO2 “molecular basket” adsorbents based on polymer-modified mesoporous molecular sieve MCM-41. Microporous Mesoporous Mater. 62, 29–45. doi:10.1016/S1387-1811(03)00388-3
Yang, S. T., Kim, J. Y., Kim, J., and Ahn, W. S. (2012). CO2 capture over amine-functionalized MCM-22, MCM-36 and ITQ-2. Fuel 97, 435–442. doi:10.1016/j.fuel.2012.03.034
Zelenak, V., Badanicova, M., Halamova, D., Cejka, J., Zukal, A., Murafa, N., et al. (2008). Amine-modified ordered mesoporous silica: effect of pore size on carbon dioxide capture. Chem. Eng. J. 144, 336–342. doi:10.1016/j.cej.2008.07.025
Zhang, W. B., Liu, H., Sun, C., Drage, T. C., and Snape, C. E. (2014a). Performance of polyethyleneimine-silica adsorbent for post-combustion CO2 capture in a bubbling fluidized bed. Chem. Eng. J. 251, 293–303. doi:10.1016/j.cej.2014.04.063
Zhang, W. B., Liu, H., Sun, C., Drage, T. C., and Snape, C. E. (2014b). Capturing CO2 from ambient air using a polyethyleneimine-silica adsorbent in fluidized beds. Chem. Eng. Sci. 116, 306–316. doi:10.1016/j.ces.2014.05.018
Zhang, X. P., Zhang, X. C., Dong, H. F., Zhao, Z. J., Zhang, S. J., and Huang, Y. (2012). Carbon capture with ionic liquids: overview and progress. Energy Environ. Sci. 5, 6668–6681. doi:10.1039/c2ee21152a
Zhang, Y. M., Li, B. Y., Williams, K., Gao, W. Y., and Ma, S. Q. (2013). A new microporous carbon material synthesized via thermolysis of a porous aromatic framework embedded with an extra carbon source for low-pressure CO2 uptake. Chem. Commun. 49, 10269–10271. doi:10.1039/c3cc45252b
Pubmed Abstract | Pubmed Full Text | CrossRef Full Text | Google Scholar
Zhao, C. W., Chen, X. P., Anthony, E. J., Jiang, X., Duan, L. B., Wu, Y., et al. (2013). Capturing CO2 in flue gas from fossil fuel-fired power plants using dry regenerable alkali metal-based sorbent. Prog. Energy Combust. Sci. 39, 515–534. doi:10.1016/j.pecs.2013.05.001
Zhao, G. Y., Aziz, B., and Hedin, N. (2010). Carbon dioxide adsorption on mesoporous silica surfaces containing amine-like motifs. Appl. Energy 87, 2907–2913. doi:10.1016/j.apenergy.2009.06.008
Zhao, M., Shi, J., Zhong, X., Tian, S., Blamey, J., Jiang, J., et al. (2014). A novel calcium looping absorbent incorporated with polymorphic spacers for hydrogen production and CO2 capture. Energy Environ. Sci. 7, 3291–3295. doi:10.1039/C4EE01281J
Zhao, Y. F., Zhao, L., Yao, K. X., Yang, Y., Zhang, Q., and Han, Y. (2012a). Novel porous carbon materials with ultrahigh nitrogen contents for selective CO2 capture. J. Mater. Chem. 22, 19726–19731. doi:10.1039/c2jm33091a
Zhao, Y. F., Liu, X., Yao, K. X., Zhao, L., and Han, Y. (2012b). Superior capture of CO2 achieved by introducing extra-framework cations into n-doped microporous carbon. Chem. Mater. 24, 4725–4734. doi:10.1021/cm303072n
Zhong, M. J., Natesakhawat, S., Baltrus, J. P., Luebke, D., Nulwala, H., Matyjaszewski, K., et al. (2012). Copolymer-templated nitrogen-enriched porous nanocarbons for CO2 capture. Chem. Commun. 48, 11516–11518. doi:10.1039/c2cc36652e
Pubmed Abstract | Pubmed Full Text | CrossRef Full Text | Google Scholar
Zukal, A., Dominguez, I., Mayerova, J., and Cejka, J. (2009). Functionalization of delaminated zeolite ITQ-6 for the adsorption of carbon dioxide. Langmuir 25, 10314–10321. doi:10.1021/la901156z
Pubmed Abstract | Pubmed Full Text | CrossRef Full Text | Google Scholar
Keywords: CCUS, CO2 capture, adsorption, energy, material science
Citation: Sun N, Tang Z, Wei W, Snape CE and Sun Y (2015) Solid adsorbents for low-temperature CO2 capture with low-energy penalties leading to more effective integrated solutions for power generation and industrial processes. Front. Energy Res. 3:9. doi: 10.3389/fenrg.2015.00009
Received: 27 October 2014; Accepted: 12 February 2015;
Published online: 09 March 2015.
Edited by:
Peter Styring, The University of Sheffield, UKCopyright: © 2015 Sun, Tang, Wei, Snape and Sun. This is an open-access article distributed under the terms of the Creative Commons Attribution License (CC BY). The use, distribution or reproduction in other forums is permitted, provided the original author(s) or licensor are credited and that the original publication in this journal is cited, in accordance with accepted academic practice. No use, distribution or reproduction is permitted which does not comply with these terms.
*Correspondence: Yuhan Sun, Shanghai Advanced Research Institute, Chinese Academy of Sciences, 100 Haike Road, Zhangjiang, Pudong, Shanghai 201203, China e-mail:c3VueWhAc2FyaS5hYy5jbg==