- Electrochemistry Branch, RDRL-SED-C, Sensors and Electron Devices Directorate, U.S. Army Research Laboratory, Adelphi, MD, USA
Gas generation (namely, the volume swelling of battery, or called the gassing) is a common phenomenon of the degradation of battery performance, which is generally a result of the electrolyte decomposition occurring during the entire lifespan of Li-ion batteries no matter whether the battery is in service or not. Abuse conditions such as overcharging and overheating make the gassing worse or even result in disastrous accidents. In overcharging, the gassing occurs mainly through the electrochemical oxidation of electrolyte solvents on the cathode with the Li+ ions from the electrolyte being reduced into metallic Li on the anode. In overheating, the gassing takes place through not only the redox decomposition but also the chemical decomposition of the electrolyte solvents on both the anode and cathode besides the vapor expansion of volatile electrolyte solvents. In this opinion article, only the gas generation occurring under the normal operation and storage conditions will be addressed.
Assuming that the Li-ion battery is well formed in manufacture and properly operated in service, the gas generation can be attributed to the chemical decomposition and redox decomposition of the electrolyte solvents on the anode and cathode. The chemical decomposition of dialkyl carbonate solvents produces ether and CO2, as described by Eq. 1, which can take place on both the anode and cathode. Resulting CO2 can be reduced into CO in accompany with the consumption of Li+ ions that are eventually originated from the cathode either by the chemical reduction (Eq. 2) or by the electrochemical reduction (Eq. 3) on the anode.
Therefore, CO2 and CO are often coexistent inside the battery. In particular, the chemical decomposition is increased with the temperature, and the redox decomposition is increased with the state-of-charge (SOC) of battery. Chemical decomposition of the carbonate solvents is catalyzed by the anode, cathode, conducting carbon, and impurity particles, and lasts the entire lifespan of the Li-ion battery. Since a catalyst can be effectively deactivated by very small amounts of poisoning species, electrolyte additives appear to be very effective in suppressing the gas generation.
For the gas generation caused by the redox decomposition of electrolyte solvents on two electrodes, Figure 1 shows that the swelling ratio of a graphite/LiCoO2 cell remains nearly constant when the SOC is lower than 80%, however, dramatically increases as the SOC exceeds 80% (Lee et al., 2003). Potential-capacity profiles of the charging process indicate that the potential of the graphite anode is very flat at ~0.25 V vs. Li/Li+, whereas that of the LiCoO2 cathode linearly increases with the SOC (Zhang et al., 2006). This observation suggests that the gassing below 80% SOC can be attributed to the reduction of electrolyte solvents on the anode, and the increased gassing above 80% SOC to the oxidization of electrolyte solvents on the cathode. The redox-relative gas generation is closely associated with the anode and cathode materials, which are discussed below.
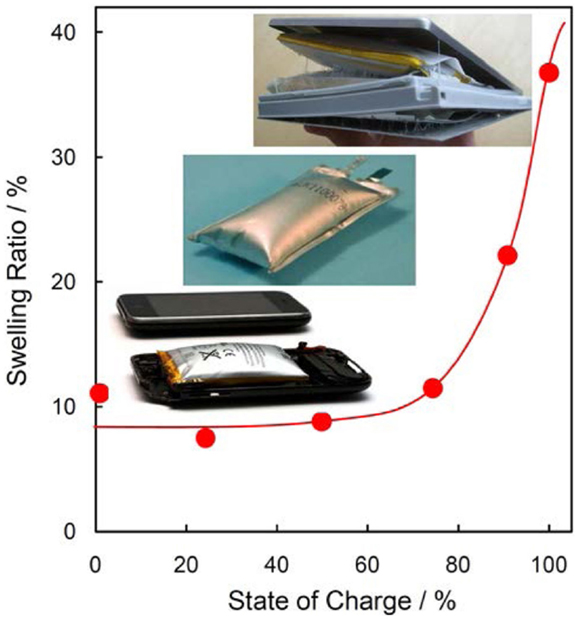
Figure 1. A trend of swelling ratio with SOC for graphite/LiCoO2 prismatic cells stored at 90°C for 4 h. Drawn from the data of Lee et al. (2003).
On Anode
The gases from the graphite anode are dominated by the reductive gases such as H2, CO, olefins, and alkanes. The mildly oxidative CO2 is typically a product of the catalytic decomposition of carbonate solvents. The reduction of electrolyte solvents is considerably affected by the solid electrolyte interface (SEI) on the graphite surface, which is formed by the electrochemical reduction of the electrolyte solvents or additives at higher potentials than those of the intercalation of Li+ ions into graphite. The gases generated during the formation of SEI have been degassed before the battery is sealed. Further gas generation is accompanied by the growth of SEI due to the parasitic solvent reduction or the failure of the pre-formed SEI. Therefore, forming a robust SEI is the key to the suppression of gas generation on the graphite based anode. It is in particular importance to note that the gassing from Li4Ti5O12 (LTO) anode is due to the intrinsic redox reaction between the LTO and carbonate solvents at the LTO–electrolyte interface (He et al., 2012). The redox reaction on one hand produces H2, CO, and CO2, and on the other hand transforms the lattice structure of the LTO surface from (111) plane to (222) plane, resulting in the formation of a new TiO2 phase. Surface coating with nanoscale carbon particles shows very effective in suppressing the interfacial reaction and resultant gas generation from the LTO anode.
On Cathode
The gases from the cathode are dominated by the mildly oxidative CO2, which can be produced by both the oxidative and chemical decompositions of the electrolyte solvents. Lithiated transition metal oxides are of very strong alkaline. Even a short period exposure to air, they will rapidly absorb CO2 and H2O to form Li2CO3 and LiOH, resulting in the removal of Li+ ions from the cathode particle surface, which changes not only the chemical composition but also the lattice structure of the cathode particle surface. Alkali metal carbonates (M2CO3, M = K, Na, and Li) are a known catalyst for the direct synthesis of dialkyl carbonates from CO2 and alcohol (Yang et al., 2013). In the same principle, Li2CO3 on the cathode particle surface could catalyze and participate in the chemical decomposition of dialkyl carbonate solvents to generate CO2, as indicated by Eq. 4. Additionally, the Li2CO3 may react with LiPF6 from the electrolyte to produce CO2, as described by Eq. 5.
As evidence for the above reactions, it is shown that the simple removal of Li2CO3 from the surface of LiNi0.83Co0.15Al0.02O2 cathode particles by washing with water can dramatically reduce the gassing of the cathode (Kim et al., 2006). In order to mitigate the gassing caused by the Li2CO3, the exposure to air should be maximally avoided in the storage of cathode materials as well as the coating and drying processes of the cathode sheets.
The other nature of the cathode materials is the dissolution of transition metal ions into the electrolyte solution (hereafter referred as to “metal dissolution” for simplicity) with the cycling and storage of Li-ion batteries, which was earliest ascribed to the HF-assisted disproportionation of Mn3+ ions for spinel LiMn2O4 cathode. In addition to those staying in the electrolyte solution, the dissolved metal ions are also incorporated into the SEI of two electrodes by combining with the solvent decomposition molecular moieties on the electrodes (both the cathode and the anode) or being reduced into the metal on the anode, leading to a rise in the SEI resistance (Xu, 2014). It is interesting to note that the metal dissolution is highly dependent of the SOC, showing a dramatic increase as the SOC approaches the end of charging (Terada et al., 2001; Pieczonka et al., 2013). This finding reveals that the metal dissolution is also associated with the direct redox between the delithiated cathode material (for example, MnO2 for LiMn2O4) and electrolyte solvents. Comparison of the previous results (Terada et al., 2001; Lee et al., 2003) indicates an excellent corresponding correlation between the swelling-SOC chart and the metal dissolution-SOC chart, suggesting that the metal dissolution must be accompanied by the gas generation. Therefore, the strategies for suppressing the metal dissolution are also applicable to the reduction of gas generation. The most effective suppression would be to coat the cathode with more stable compounds, such as, a metal oxide, a halide, a phosphate, and preferably a solid electrolyte (Li et al., 2013). Another benefit of the surface coating is to reduce the basicity of the cathode particle surface, which favors reducing CO2 absorption of the cathode and increasing chemical stability of the PVdF binder that is otherwise subject to dehydrofluorinate in strong alkaline environments (Dias and McCarthy, 1985). Besides the gassing caused by the Li2CO3 impurity and the metal dissolution, each type of cathode materials affects the gas generation in their specific manners, which are discussed, respectively, as below.
Layered Cathode Materials
The gassing from the layered cathode materials is mainly due to O2 evolution and metal dissolution. The layered cathode materials, such as LiCoO2, nickel-cobalt-aluminum (NCA), nickel-manganese-cobalt (NMC), and a family of Li-rich transition metal oxide solid solutions with a general formula of xLi2MnO3.(1 − x)LiMO2 (M = Co, Mn, Ni), have no distinct potential rise that can be used to detect the fully charged state (i.e., the end of charging) by the battery charger. With the service going on, the impedance of the battery is progressively increased due to the growth of the SEI on the anode and cathode, which meanwhile consumes the Li+ ions from the cathode. As a result, the capacity ratio of the cathode to anode is decreased so that the cathode can be readily overcharged if the charging protocol is not adjusted according to the real-time health of battery. Overcharging results in the evolution of O2, during which the oxygen radical anion intermediate can nucleophilically attack dialkyl carbonate solvents to produce CO2 and other insoluble products, as occurred in the Li-air batteries (Freunberger et al., 2011), with the insoluble products further contributing to the growth of resistive SEI on the cathode. The O2 evolution results in a net loss of Li2O, and consequently changes the lattice structure of the cathode materials, as described by Eq. 6:
Since “4δLi+ + δO2” in the product side of Eq. 6 is equivalent to “2δLi2O – 4δe−,” the net result of the O2 evolution is a loss of Li2O. In accompany with the O2 evolution, the released Li+ ions are incorporated into the SEI of the cathode, which results in the growth of the cathode SEI and contributes to the irreversible overcharging capacity. Therefore, the O2 evolution caused by overcharging is the source of not only the gas generation but also the SEI growth on the cathode. In particular, charging potentials of the Li-rich xLi2MnO3.(1 − x)LiMO2 (M = Co, Mn, Ni) solid solutions are up to above 4.6 V vs. Li/Li+. Even in normal operation potential range (2.0–4.6 V), the O2 evolution is inevitable, leading to an irreversible lattice structural transformation of the cathode materials (Armstrong et al., 2006; Gu et al., 2013). The O2 evolution and resulting reactions with the carbonate solvents and even with the conducting carbon agent (i.e., oxidation in the latter case) have been recognized to be the major cause for the gas generation and capacity degradation of the Li-rich cathode materials. Therefore, timely adjusting of the charging protocol to strictly avoid the overcharging and structural stabilization by doping with more stable metal ions such as Al3+ would be very effective to suppress the gas generation from the layered cathode materials.
Spinel Cathode Materials
The gassing from the spinel cathode materials, such as 4 V Li2Mn2O4 and 4.7 V LiNi0.5Mn1.5O4, is dominated by the oxidation of the electrolyte solvents. The ultimate products of the oxidation of carbonate solvents are CO2 and H2O, and organic acids (H+) are the possible intermediate of the solvent oxidization (Armstrong et al., 2005). Therefore, the oxidation very likely initiates other problems, such as the hydrolysis of LiPF6 salt and H2 generation as the H2O and organic acid intermediate are diffused onto the anode and reduced there. The electrochemical oxidation occurs only in the charging process, whereas the chemical oxidation lasts the entire lifespan and it is often accompanied by the metal dissolution. Surface coating with more robust compounds such as AlPO4, AlF3, Al2O3, ZnO, Bi2O3 (Liu and Manthiram, 2009), and solid electrolyte (Li et al., 2013) shows very effective in suppressing the metal dissolution, this strategy is certainly applicable to the gassing reduction. Lithium bis(oxalato)borate (LiBOB) is very effective in suppressing metal dissolution, however, it is oxidized to generate CO2 at high potentials. Some electrolyte additives, such as fluorinated carbonates (Zhang et al., 2013) and phosphates (Cresce and Xu, 2011), have the ability to form robust SEI on the cathode surface by the chemical reaction, and hence could offer an alternative approach for the in situ surface coating to protect the 4.7 V LiNi0.5Mn1.5O4 cathode from direct contact with the liquid electrolyte. The above approaches are also applicable to the other high-voltage cathode materials such as Li-rich layered oxides and LiCoPO4. For the LiNi0.5Mn1.5O4 cathode, the LixNi1 − xO impurity is also responsible for the gas generation because above 4.5 V vs. Li/Li+ it evolves O2, during which the oxygen radical anion intermediate reacts with the carbonate solvents to produce CO2. A combination of the surface coating, using electrolyte additive and purifying cathode material likely leads to a synergistic effect for the gassing suppression of the high-voltage LiNi0.5Mn1.5O4 cathode.
In summary, gas generation in the Li-ion batteries involves many complicated reactions in relation to the chemical and redox decompositions of the electrolyte solvents. The chemical decomposition is catalyzed by the active electrode materials, conducting carbon, and Li2CO3 impurity. The redox decomposition may be an electrochemical process or/and a chemical process, in which the former occurs only in the charging process, whereas the latter lasts the entire lifespan of the battery and is often accompanied by the metal dissolution. The dissolved metal ions participate in the progressive formation of SEI on the cathode and anode, resulting in the growth of the resistive SEI and adversely affecting the performance of the Li-ion batteries. Given that all the materials are in high purity and are strictly dried, the combination of the surface coating and electrolyte additive would offer the most effective solution to the gassing problem of Li-ion batteries.
Conflict of Interest Statement
The author declares that the research was conducted in the absence of any commercial or financial relationships that could be construed as a potential conflict of interest.
Acknowledgments
The author thanks Dr. C. Lundgren for her critical reading of the manuscript and valuable suggestions.
References
Armstrong, A. R., Holzapfel, M., Novak, P., Johnson, C. S., Kang, S. H., Thackeray, M. M., et al. (2006). Demonstrating oxygen loss and associated structural reorganization in the lithium battery cathode Li[Ni0.2Li0.2Mn0.6]O2. J. Am. Chem. Soc. 128, 8694–8698. doi:10.1021/ja062027+
Pubmed Abstract | Pubmed Full Text | CrossRef Full Text | Google Scholar
Armstrong, A. R., Robertson, A. D., and Bruce, P. G. (2005). Overcharging manganese oxides: extracting lithium beyond Mn4+. J. Power Sources 146, 275–280. doi:10.1016/j.jpowsour.2005.03.104
Cresce, A. V., and Xu, K. (2011). Electrolyte additive in support of 5 V Li ion chemistry. J. Electrochem. Soc. 158, A337–A342. doi:10.1149/1.3532047
Dias, A. J., and McCarthy, T. J. (1985). Dehydrofluorination of poly(vinylidene fluoride) in dimethylformamide solution: synthesis of an operationally soluble semiconducting polymer. J. Polym. Sci. A Polym. Chem. 23, 1057–1061. doi:10.1002/pol.1985.170230410
Freunberger, S. A., Chen, Y., Peng, Z., Griffin, J. M., Hardwick, L. J., Barde, F., et al. (2011). Reactions in the rechargeable lithium–O2 battery with alkyl carbonate electrolytes. J. Am. Chem. Soc. 133, 8040–8047. doi:10.1021/ja2021747
Pubmed Abstract | Pubmed Full Text | CrossRef Full Text | Google Scholar
Gu, M., Belharouak, I., Zheng, J., Wu, H., Xiao, J., Genc, A., et al. (2013). Formation of the spinel phase in the layered composite cathode used in Li-ion batteries. ACS Nano 7, 760–767. doi:10.1021/nn305065u
Pubmed Abstract | Pubmed Full Text | CrossRef Full Text | Google Scholar
He, Y. B., Li, B., Liu, M., Zhang, C., Lv, W., Yang, C., et al. (2012). Gassing in Li4Ti5O12-based batteries and its remedy. Sci. Rep. 2, 913. doi:10.1038/srep00913
Pubmed Abstract | Pubmed Full Text | CrossRef Full Text | Google Scholar
Kim, J., Hong, Y., Ryu, K. S., Kim, M. G., and Cho, J. (2006). Washing effect of a LiNi0.83Co0.15Al0.02O2 cathode in water. Electrochem. Solid State Lett. 9, A19–A23. doi:10.1149/1.2135427
Lee, K. H., Song, E., and Lim, H. S. (2003). “Swelling mechanism of the lithium ion battery at high temperature,” in 203rd ECS Meeting, Abs. #110 (Paris). Available at: https://www.electrochem.org/dl/ma/203/pdfs/0110.pdf
Li, J., Baggetto, L., Martha, S. K., Veith, G. M., Nanda, J., Liang, C., et al. (2013). An artificial solid electrolyte interphase enables the use of a LiNi0.5Mn1.5O4 5 V cathode with conventional electrolytes. Adv. Energy Mater. 3, 1275–1278. doi:10.1002/aenm201300378
Liu, J., and Manthiram, A. (2009). Understanding the improvement in the electrochemical properties of surface modified 5 V LiMn1.42Ni0.42Co0.16O4 spinel cathodes in lithium-ion cells. Chem. Mater. 21, 1695–1707. doi:10.1021/cm9000043
Pieczonka, N. P. W., Liu, Z., Lu, P., Olson, K. L., Moote, J., Powell, B. R., et al. (2013). Understanding transition-metal dissolution behavior in LiNi0.5Mn1.5O4 high-voltage spinel for lithium ion batteries. J. Phys. Chem. C 117, 15947–15957. doi:10.1021/jp405158m
Terada, Y., Nishiwaki, Y., Nakai, I., and Nishikawa, F. (2001). Study of Mn dissolution from LiMn2O4 spinel electrodes using in situ total reflection X-ray fluorescence analysis and fluorescence XAFS technique. J. Power Sources 9, 420–422. doi:10.1016/S0378-7753(01)00741-8
Xu, K. (2014). Electrolytes and interphases in Li-ion batteries and beyond. Chem. Rev. doi:10.1021/cr500003w
Yang, Q., Wang, H., Ding, X., Yang, X., and Wang, Y. (2013). One-pot synthesis of dimethyl carbonate from carbon dioxide, cyclohexene oxide, and methanol. Res. Chem. Intermed. 1–11. doi:10.1007/s11164-013-1514-4
Zhang, S. S., Xu, K., and Jow, T. R. (2006). Study of the charging process of a LiCoO2-based Li-ion battery. J. Power Sources 160, 1349–1354. doi:10.1016/j.jpowsour.2006.02.087
Pubmed Abstract | Pubmed Full Text | CrossRef Full Text | Google Scholar
Keywords: gas generation, battery swelling, performance degradation, high-voltage cathode, electrolyte additive, solid electrolyte interface
Citation: Zhang SS (2014) Insight into the gassing problem of Li-ion battery. Front. Energy Res. 2:59. doi: 10.3389/fenrg.2014.00059
Received: 03 November 2014; Accepted: 18 November 2014;
Published online: 05 December 2014.
Edited by:
Jie Xiao, Pacific Northwest National Laboratory, USACopyright: © 2014 Zhang. This is an open-access article distributed under the terms of the Creative Commons Attribution License (CC BY). The use, distribution or reproduction in other forums is permitted, provided the original author(s) or licensor are credited and that the original publication in this journal is cited, in accordance with accepted academic practice. No use, distribution or reproduction is permitted which does not comply with these terms.
*Correspondence:c2hlbmdzaHVpLnpoYW5nLmNpdkBtYWlsLm1pbA==;c2hlbmdzaHVpQGdtYWlsLmNvbQ==