- 1Dipartimento di Scienze e Tecnologie Chimiche, Università di Roma Tor Vergata, Rome, Italy
- 2MAtériaux Divisés, Interfaces, Réactivité, ELectrochimie (MADIREL – UMR 7246), Aix Marseille Université, Marseille, France
- 3Faculty of Material and Engineering, Kunming University of Science and Technology, Kunming, China
The cross-link reaction via sulfone bridges of sulfonated polyether ether ketone (SPEEK) by thermal treatment at 180°C in presence of dimethylsulfoxide is discussed. The modifications of properties subsequent to the cross-linking are presented. The mechanical strength as well as the hydrolytic stability increased with the thermal treatment time, i.e., with the degree of cross-linking. The proton conductivity was determined as function of temperature, IEC, degree of cross-linking, and hydration number. The memory effect, which is the membrane ability to “remember” the water uptake reached at high temperature also at lower temperature, is exploited in order to achieve high values of conductivity. Membranes swelled at 110°C can reach a conductivity of 0.14 S/cm at 80°C with a hydration number (λ) of 73.
Introduction
To work properly in electrochemical devices such as polymer electrolyte membrane fuel cells (PEMFCs), ionomer membranes must satisfy a whole list of requirements. The materials challenges include high ionic conductivity and long lifetime, high chemical and mechanical stability, low permeability, and low cost (Linkous et al., 1998; Devanathan, 2008; Peighambardoust et al., 2010; Bose et al., 2011; Chandan et al., 2013).
Sulfonated aromatic polymers (SAP) are proton-conducting solid electrolytes that can meet many of these properties, especially in terms of good thermal stability, appropriate mechanical strength, high proton conductivity, and they are inexpensive (Astill et al., 2009; Iulianelli and Basile, 2012; Yee et al., 2012; Zuo et al., 2012; Di Vona and Knauth, 2013). The conductivity of these ionomers is water assisted: the higher is the content of water inside the membrane, the higher is the proton transport (Kreuer, 2000; Paddison and Paul, 2002; Di Vona et al., 2013). Unfortunately, a too high water uptake (WU) causes a degradation of mechanical properties and the failure of the device (Iojoiu et al., 2005; Collier et al., 2006; Borup et al., 2007; Paik et al., 2009; Takamuku and Jannasch, 2012). Different solutions were explored in the last years: composite membranes, hybrid polymers, co-block polymer membranes, cross-linked polymers (Ghassemi et al., 2004; Hickner et al., 2004; Alberti et al., 2007; Su et al., 2007; Premchand et al., 2008; Di Vona et al., 2011; Subianto et al., 2013). The presence of cross-links (XL), especially by covalent bonds, induces some changes in the physico-chemical properties, such as good chemical and solvent resistance, low fuel permeability, a high dimensional stability and an excellent mechanical strength and it makes polymers more resistant to harsh environment (Kerres, 2005; Mikhailenko et al., 2006; Zhong et al., 2007; Feng et al., 2009; Han et al., 2010; Hou et al., 2012a). The extent of the effect depends mainly on the XL degree (DXL) or density. Recently, Di Vona et al. (Di Vona et al., 2009; Knauth et al., 2013) showed that an annealing treatment performed on cast SAP affords cross-linked membranes via sulfone bridges.
Among the various hydrocarbon polymers, polyether ether ketone (PEEK) based membranes are well known (Xing et al., 2004; Jiang et al., 2005; Fathima et al., 2007) due to their good thermal stability, appropriate mechanical strength, and when sulfonated, good proton conductivity, which increases with the degree of sulfonation (DS). However, these aromatic polymer electrolytes with high IEC, which show high proton conductivity, have the problem of weak mechanical behavior due to some water solubility, and this is one of the main obstacles for application in PEMFCs (Dyck et al., 2002; Roziere and Jones, 2003; Karlsson and Jannasch, 2005; Parcero et al., 2006; Di Vona et al., 2010).
This paper presents the advantages of thermally treated membranes based on sulfonated polyether ether ketone (SPEEK) in terms of hydrolytic stability and mechanical properties.
Experimental
Polyether ether ketone (Victrex 450P, M = 38300, and Goodfellow EK306010, abbreviated in the following SPEEK GF), was sulfonated following the procedure already reported (Kim et al., 2013; Maranesi et al., 2013). Depending on the reaction time, sulfonated PEEK (SPEEK) was obtained with different DS in the range 0.75–1. The DS was evaluated by titration and NMR analysis (Mikhailenko et al., 2000).
Preparation of Membranes
The polymers were dissolved in dimethylsulfoxide (DMSO) at 80–90°C. The ratio polymer/DMSO was about 1:10 (mg/mL). After evaporation to around 1/3 of the original volume, the solution was spread on a glass plate, using a doctor-blade type equipment, and then put in the oven for the casting treatment at 120°C for 24 h. After the casting, membranes were stored at ambient humidity and peeled off (called in the following “first treatment”).
Second treatment
Some membranes were placed on a Teflon substrate for the second treatment. The membranes were put in the oven at 180°C for a time in the range 2–33 h (180/h) depending on the desired cross-linking degree (DXL). After the preparation, the membranes were immersed at room temperature in a solution of H2O2 3% for 1 h, then in H2SO4 5 M for 1 h, and washed with water.
Characterization of SPEEK Membranes
Ion exchange capacity measurements
Ion exchange capacity (IEC in milliequivalent/gram) was measured as reported previously (Di Vona et al., 2009). In order to eliminate DMSO and its decomposition products, which can affect IEC, membranes were swelled in water at 100°C for 5 h. This procedure was insufficient to remove by-products; therefore, after swelling in water at 100°C for 5 h, the samples were treated with H2SO4 5 M at room temperature for 2 h and then washed until neutral pH before the titration.
Water uptake
Samples dried over P2O5 for 3 days were weighed (mdry) and were then immersed 24 h in liquid water in a closed Teflon vessel at a constant temperature. After the immersion, the membranes were equilibrated at 25°C for 24 h. The excess of water was carefully wiped off and the membrane mass was determined (mwet):
The hydration number was calculated as:
The uncertainty is about 0.5.
Mechanical properties
The stress–strain properties were investigated using an ADAMEL Lhomargy DY30 test machine at room temperature at a constant crosshead speed of 5 mm min-1 with aluminum sample holders. The clamping pressure was determined according to a preliminary tensile stress–strain test; it was about 40% of the apparent elasticity limit, corresponding to the linear part of the tensile curve.
Prior to the measurements, the polymer samples were stabilized at ambient temperature and humidity, which was (50 ± 10)% RH. The measurement time was below 5 min (Sgreccia et al., 2010).
Dynamic mechanical analysis (DMA) was performed from 30 to 250°C in air on a DMA 2980 dynamic analyzer (TA Instruments). Measurements were operated in air at a fixed frequency of 1 Hz with 0.05 N initial static force and oscillation amplitude of 10 μm. This last value was chosen to keep the linear viscoelastic response of samples during experiments. The storage (E′) and loss modulus (E″) spectra vs. temperature were obtained at 3 K/min between 30 and 250°C. DMA analysis allows deriving the glass transition temperature of the samples treated for different times at 180°C (Sgreccia et al., 2008).
Proton conductivity
The proton conductivity was measured by impedance spectroscopy (EG&G 6310 and Solartron 1260) in through-plane two-point configuration. The amplitude of the applied voltage was 20 mV and the frequency range was between 10 Hz and 1 MHz.
The measurements at full humidification were made in a Swagelok cell in presence of liquid water. Before the conductivity measurements, the membranes were immersed in water for 24 h at a fixed temperature (Di Vona et al., 2013). The conductivity measurements as function of temperature between 80 and 140°C or relative humidity (RH = 30–95%) were done in a home-made apparatus (Donnadio et al., 2012).
Results and Discussion
The cross-linking reaction occurs during the treatment process directly in situ on membranes cast from DMSO. The study of the mechanism (Maranesi et al., 2013) led to the following conclusions:
(a) Membranes cast from dimethylacetamide, N-methyl-2-pyrrolidone, acetone, and acetone/water did not undergo XL, while DMSO was essential to achieve the reaction.
(b) The combination of elemental analysis and FT-IR spectroscopy demonstrated that sulfone linkages, which form by transformation of sulfonic acid groups, were responsible for covalent cross-linking.
(c) The NMR analysis of the by-products showed that DMSO promotes the formation of the electrophilic −SO2+ intermediate either through the formation of a direct interaction with the solvent or by the formation of an anhydride moiety (Maranesi et al., 2013).
(d) A two-step thermal treatment was necessary for a successful XL process; the first step is an evaporation treatment that leaves the “optimal” quantity of residual DMSO inside the membrane. The second step is the effective treatment that allows running the XL reaction in the presence of a suitable amount of residual DMSO (Maranesi et al., 2013).
(e) The temperature selected for the second treatment was 180°C to achieve relatively short times. When the reaction was carried out at 160°C, a sufficiently large amount of XL is obtained only for times around 64 h, while a temperature higher than 180°C can lead to desulfonation reactions. Therefore, it is important to find the best compromise between time and temperature of treatment in order to facilitate the industrial scale-up.
Figure 1 shows the XL reaction. Table 1 reports the residue quantity of DMSO in the membrane after the treatment. A quantity around 5% at the beginning of the process is sufficient to achieve reticulated products; the DMSO percentage decreased, as expected, with the second treatment time.
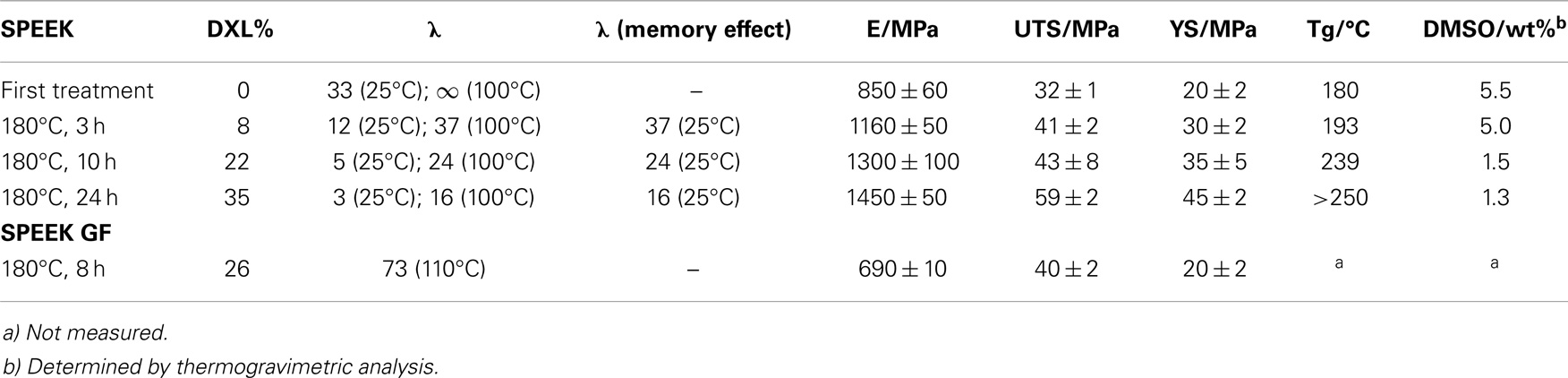
Table 1. Memory effect and mechanical properties (E, elastic modulus, UTS, ultimate tensile strength, YS, yield stress) for various membranes in acid form.
Implications on WU and thermal stability, which both depend on the degree of cross-linking, are of particular importance for the development of high performance proton-conducting membranes. Different DXL can be obtained varying the second treatment time. Figure 2 shows the behavior of WU as a function of DXL expressed as:
for a SPEEK sample with initial IECinit = 2.5 meq/g treated at 120/24–180/h. In Table 1, the corresponding times of treatment at 180°C are reported.
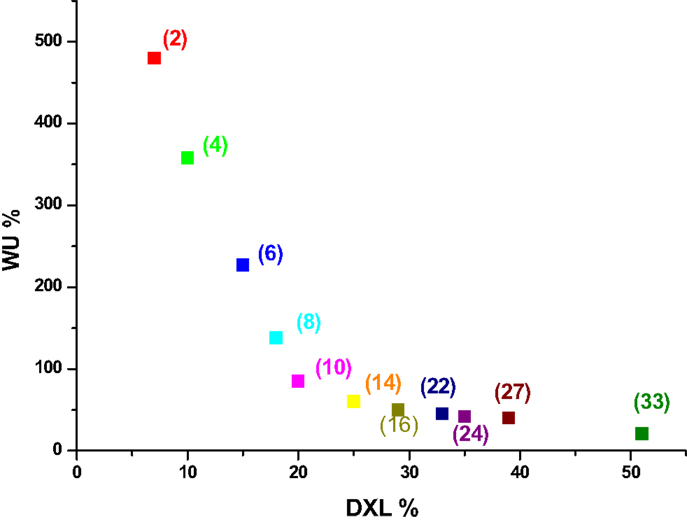
Figure 2. WU (%) after immersion in water at 100°C for 24 h as a function of DXL for a SPEEK sample with an initial IEC = 2.5 meq/g. In parenthesis, the time of the second treatment at 180°C is shown.
For this sample, the WU reaches a value around 50% after a time of treatment of 14 h with a final IECfin = 1.9 meq/g. A WU ≤50% for SAP membranes is a very significant improvement and it is important to note that the WU can be modulated very simply by varying the time.
Another important consequence of the formation of XL is the presence of a “memory effect”: the membrane ability to “remember” the WU reached at high temperature also at lower temperature. As shown in Table 1 for a sample with DXL = 0.22, the λ value is 24 after swelling in water for 24 h at 100°C, and this value remains identical when the membrane is hydrated at 25°C. However, in order to use the memory effect in practice, one must take care that the membrane is able to support a treatment in water at high temperature without dissolving under these conditions. A cross-link treatment is therefore mandatory before the memory effect can be used efficiently.
Table 1 shows mechanical properties for SPEEK samples with different DXL. In general, Young’s modulus (E, stiffness) characterizes the elastic domain of polymers, where weak inter-chain bonds are observed at the microscopic scale; it can be related to fundamental bond properties. Stiffness explores essentially weak bonds (low displacements) like: (a) Van der Waals bonds (change of distance between chains and of dipole-dipole interactions); (b) defects, such as entanglements; (c) the presence of solvents, such as water and DMSO.
Yield stress (YS) and tensile strength (TS) are instead related to strong bonds, including covalent cross-links between macromolecular chains, and are macroscopic scale properties. TS and YS explore large displacements (plasticity).
Table 2 shows the influence of the swelling in water for two samples. The effect of water that acts as plasticizer is evident with a distinct lowering of the mechanical properties. Water and DMSO, which are high dielectric constant solvents, act as plasticizer by reducing Van der Waals and ionic bond strength. The osmotic pressure of the acidic solutions is opposed by the stiffness of the polymers (Knauth et al., 2014).
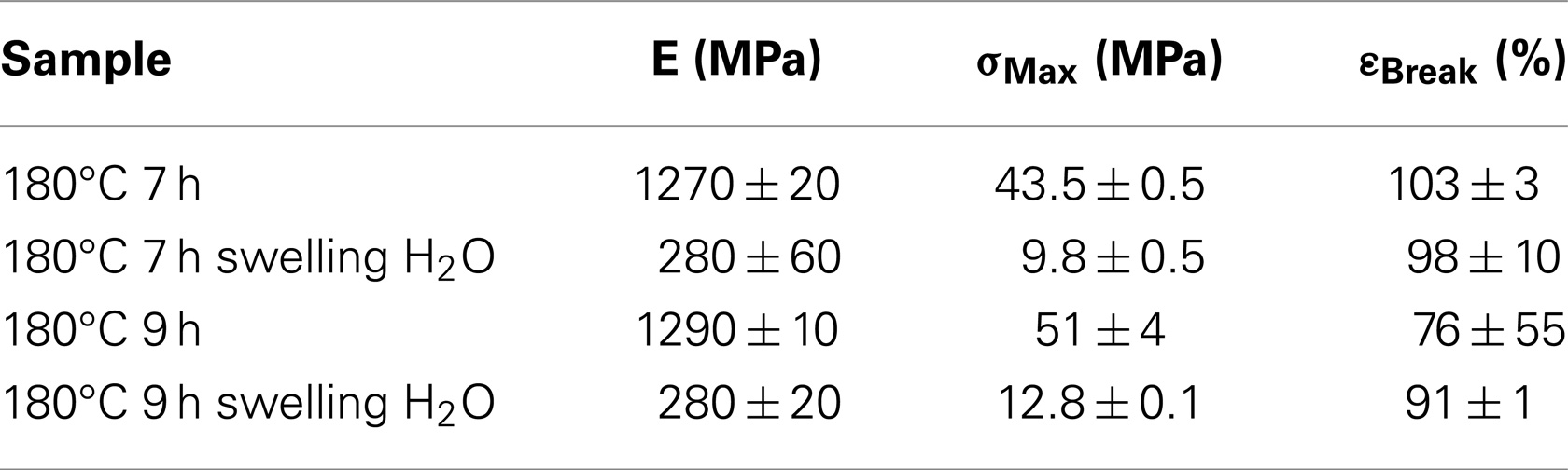
Table 2. SPEEK samples treated for 7 and 9 h at 180°C before and after swelling in water at 25°C for 24 h.
From the mechanical measurements, it is evident that the thermal treatment enhances significantly the mechanical properties of the membranes: the elastic modulus, ultimate TS, and YS all increase significantly by XL. If we assume that mechanical degradation of membranes is related to the existence of plastic deformation during fuel cell operation, it is clear that the enhancement of mechanical properties is of major importance for the improvement of membrane durability inside the fuel cell. It should also influence significantly the WU behavior in various relative humidity, and the membrane swelling at high RH.
The effect of the casting direction on membranes was also investigated. The measurements were made cutting the sample longitudinal or traversal to the direction of casting. It is possible to observe from Figure 3A a clear enhancement of TS and elastic modulus in longitudinal direction only after covalent cross-linking of chains.
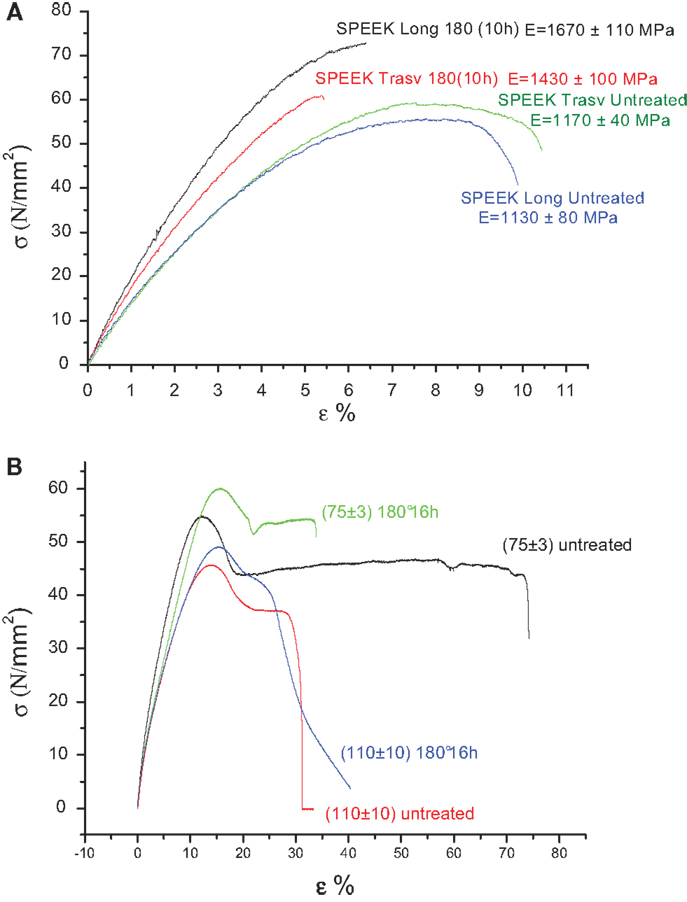
Figure 3. (A) Effect of the casting direction (longitudinal or transversal) and (B) effect of thickness (micrometer) on stress-strain curves of SPEEK membranes are shown.
The mechanical properties also depend on the membrane thickness. Thinner samples have higher E and UTS (Figure 3B), probably because the sliding of macromolecular chains is less easy.
The effect of regeneration after the second treatment of membranes in 5 M H2SO4 is evident in Figure 4. The elongation at break is considerably increased. The removal of ionic cross-links, probably by Ca2+ ions, by treatment in acidic media leads to a lower interaction between chains increasing the plasticity.
Typical dynamic mechanical analysis curves are shown in Figure 5. The left curve shows the storage modulus E′, which describes the elastic response of the polymer and should, in the ideal case, be equal to the elastic modulus. The right curve shows the phase angle (tan δ), which is defined as ratio of the loss modulus (related to the viscous response of the polymer) to the storage modulus. The phase angle is very sensitive to phase transitions, and especially to the glass transition of the polymer Tg (Hou et al., 2012b). One can notice a good overall agreement of elastic and storage moduli, which increase with treating time. The glass transition temperature increases clearly with the cross-linking time from about 180°C to over 250°C; this is correlated to a reduction of symmetry due to XL bonds.
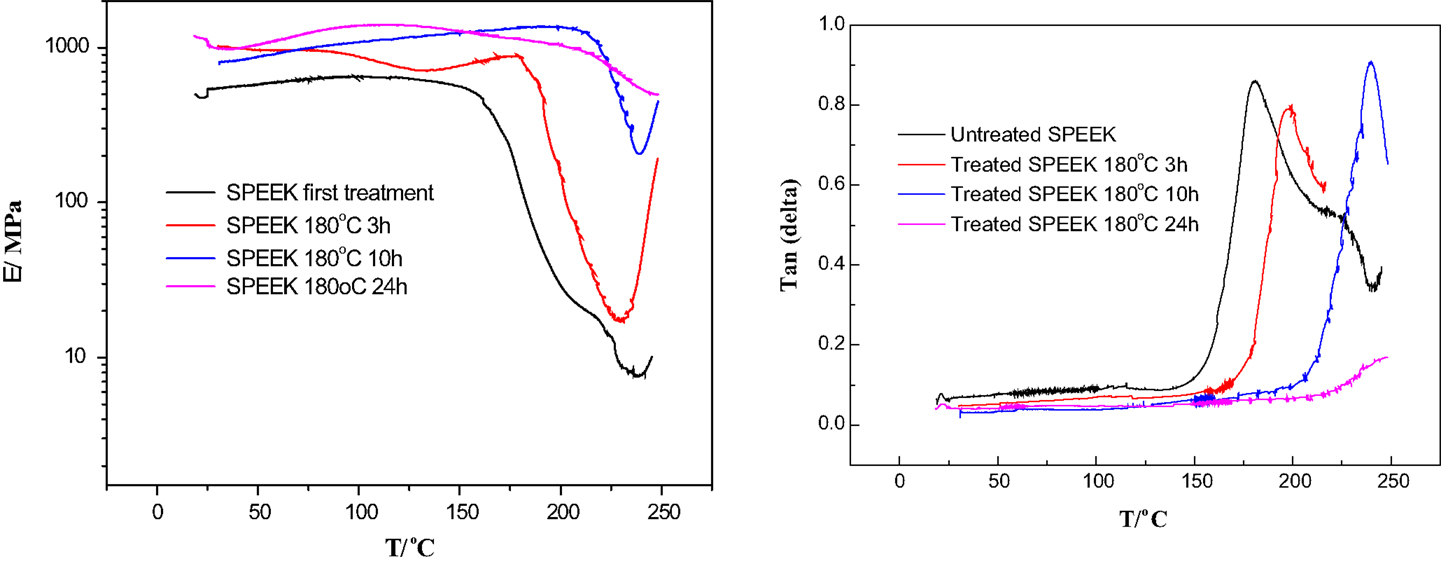
Figure 5. Dynamic mechanical analysis of SPEEK membranes is shown. Left: storage modulus vs. temperature, right: phase angle (tan δ) vs. temperature.
Table 3 shows the conductivity values at 140°C and RH = 90% for SPEEK samples with different treatment times; the values were checked over several weeks with consistent results. Although the IEC is relatively low, the conductivity is good and stable with time. Interesting is the comparison with a sample with the same IEC value, but without cross-linking. The conductivity is lower, probably the presence of the sulfone bridges straighten the pathways for conduction (Di Vona et al., 2013) and increase therefore the proton mobility.
To exploit the memory effect, membranes were swelled at high temperature (140°C) and then the conductivity was measured at 120°C as a function of the relativity humidity. For a sample with initial DS = 1 and treated 120/24–180/16, the conductivity at 30% RH increases by a factor 4 (from 3.2 × 10−5 to 1.4 × 10−4 S/cm) when the membranes were swelled at high temperature. Although the conductivity remains relatively low, this could be a method to improve the performances at low humidity. Correlated to the memory effect are the data presented in Table 4 for SPEEK GF. The values of conductivity are high and increase when the content of water in the membranes increases by the effect of temperature. In a previous paper, we predicted the proton conductivity that can be obtained for a certain hydration level (Di Vona et al., 2013). For example, in order to obtain conductivity above 10−2 S/cm at 25°C, a hydration number above 35 was foreseen. The data reported in Table 4 confirm this point. The proton conductivity of XL-SPEEK can be then enhanced by hydrating the cross-linked membranes at high temperature, under conditions that uncross-linked SPEEK does not support, achieving good electrical properties, compatible with fuel cell requirements.
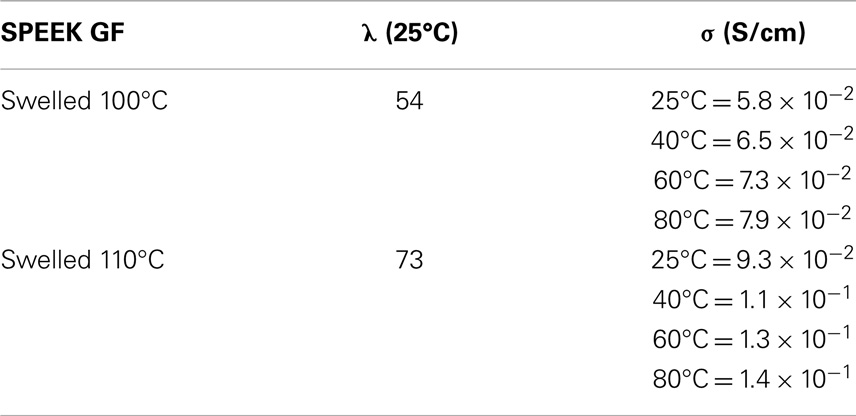
Table 4. Conductivity data in full humidify condition for SPEEK GF, treated at 180°C/8 h with a final IEC of 1.96 meq/g (DXL = 26) swelled at 100 and 110°C before measurements.
Conclusion
In this work, an overview of properties of cross-linked SPEEK is presented. The XL process is an electrophilic aromatic substitution reaction by sulfonium ions that occurs during heat treatments at 180°C in presence of small quantities of DMSO. Different properties of various cross-linked SPEEK membranes were determined. The cross-linked membranes present an improved hydrothermal stability and good mechanical properties that depend on the degree of cross-linking. Very interesting is the memory effect that can be exploited to achieve high values of proton conductivity also at low temperature. The modification of properties subsequent to cross-linking is of utmost importance for membrane development used in various electrochemical techniques, such as fuel cells.
Conflict of Interest Statement
The authors declare that the research was conducted in the absence of any commercial or financial relationships that could be construed as a potential conflict of interest.
Acknowledgments
The EU-FP7 (FCH-JU) project “LoLiPEM – Long-life PEM-FCH &CHP systems at temperatures higher than 100°C” (GA 245339), is gratefully acknowledged for co-funding this work.
References
Alberti, G., Casciola, M., Capitani, D., Donnadio, A., Narducci, R., Pica, M., et al. (2007). Novel Nafion-zirconium phosphate nanocomposite membranes with enhanced stability of proton conductivity at medium temperature and high relative humidity. Electrochim. Acta 52, 8125–8132. doi:10.1016/j.electacta.2007.07.019
Astill, T., Xie, Z., Shi, Z. Q., Navessin, T., and Holdcroft, S. (2009). Factors influencing electrochemical properties and performance of hydrocarbon-based electrolyte PEMFC catalyst layers. J. Electrochem. Soc. 156, B499–B508. doi:10.1149/1.3082119
Borup, R., Meyers, J., Pivovar, B., Kim, Y. S., Mukundan, R., Garland, N., et al. (2007). Scientific aspects of polymer electrolyte fuel cell durability and degradation. Chem. Rev. 107, 3904–3951. doi:10.1021/cr050182l
Bose, S., Kuila, T., Thi, X. L. N., Kim, N. H., Lau, K. T., and Lee, J. H. (2011). Polymer membranes for high temperature proton exchange membrane fuel cell: recent advances and challenges. Prog. Polym. Sci. 36, 813–843. doi:10.1016/j.progpolymsci.2011.01.003
Chandan, A., Hattenberger, M., El-Kharouf, A., Du, S. F., Dhir, A., Self, V., et al. (2013). High temperature (HT) polymer electrolyte membrane fuel cells (PEMFC) – a review. J. Power Sources 231, 264–278. doi:10.1016/j.jpowsour.2012.11.126
Collier, A., Wang, H. J., Yuan, X. Z., Zhang, J. J., and Wilkinson, D. P. (2006). Degradation of polymer electrolyte membranes. Int. J. Hydrogen Energy 31, 1838–1854. doi:10.1016/j.ijhydene.2006.05.006
Devanathan, R. (2008). Recent developments in proton exchange membranes for fuel cells. Energy Environ. Sci. 1, 101–119. doi:10.1039/b808149m
Di Vona, M. L., and Knauth, P. (2013). Sulfonated aromatic polymers as proton-conducting solid electrolytes for fuel cells: a short review. Z. Phys. Chem. 227, 595–614. doi:10.1524/zpch.2013.0337
Di Vona, M. L., Pasquini, L., Narducci, R., Pelzer, K., Donnadio, A., Casciola, M., et al. (2013). Cross-linked sulfonated aromatic ionomers via SO2 bridges: conductivity properties. J. Power Sources 243, 488–493. doi:10.1016/j.jpowsour.2013.05.127
Di Vona, M. L., Sgreccia, E., Donnadio, A., Casciola, M., Chailan, J. F., Auer, G., et al. (2011). Composite polymer electrolytes of sulfonated poly-ether-ether-ketone (SPEEK) with organically functionalized TiO(2). J. Memb. Sci. 369, 536–544. doi:10.1016/j.memsci.2010.12.044
Di Vona, M. L., Sgreccia, E., Licoccia, S., Alberti, G., Tortet, L., and Knauth, P. (2009). Analysis of temperature-promoted and solvent-assisted cross-linking in sulfonated poly-ether-ether-ketone (SPEEK) proton conducting membranes. J. Phys. Chem. B 113, 7505–7512. doi:10.1021/jp9006679
Di Vona, M. L., Sgreccia, E., Tamilvanan, M., Khadhraoui, M., Chassigneux, C., and Knauth, P. (2010). High ionic exchange capacity polyphenylsulfone (SPPSU) and polyethersulfone (SPES) cross-linked by annealing treatment: thermal stability, hydration level and mechanical properties. J. Memb. Sci. 354, 134–141. doi:10.1016/j.memsci.2010.02.058
Donnadio, A., Casciola, M., Di Vona, M. L., and Tamivanan, M. (2012). Conductivity and hydration of sulfonated polyethersulfone in the range 70-120 degrees C: effect of temperature and relative humidity cycling. J. Power Sources 205, 145–150. doi:10.1016/j.jpowsour.2011.12.060
Dyck, A., Fritsch, D., and Nunes, S. P. (2002). Proton-conductive membranes of sulfonated polyphenylsulfone. J. Appl. Polym. Sci. 86, 2820–2827. doi:10.1002/app.11264
Fathima, N. N., Aravindhan, R., Lawrence, D., Yugandhar, U., Moorthy, T. S. R., and Nair, B. U. (2007). SPEEK polymeric membranes for fuel cell application and their characterization: a review. J. Sci. Ind. Res. 66, 209–219. doi:10.1039/c0cs00144a
Feng, S. G., Shang, Y. M., Xie, X. F., Wang, Y. Z., and Xu, J. M. (2009). Synthesis and characterization of crosslinked sulfonated poly(arylene ether sulfone) membranes for DMFC applications. J. Memb. Sci. 335, 13–20. doi:10.1016/j.memsci.2009.02.034
Ghassemi, H., Ndip, G., and McGrath, J. E. (2004). New multiblock copolymers of sulfonated poly(4′-phenyl-2,5-benzophenone) and poly(arylene ether sulfone) for proton exchange membranes. Polymer 45, 5855–5862. doi:10.1016/j.polymer.2004.06.009
Han, M. M., Zhang, G., Li, M. Y., Wang, S. A., Zhang, Y., Li, H. T., et al. (2010). Considerations of the morphology in the design of proton exchange membranes: cross-linked sulfonated poly(ether ether ketone)s using a new carboxyl-terminated benzimidazole as the cross-linker for PEMFCs. Int. J. Hydrogen Energy 36, 2197–2206. doi:10.1016/j.ijhydene.2010.11.065
Hickner, M. A., Ghassemi, H., Kim, Y. S., Einsla, B. R., and McGrath, J. E. (2004). Alternative polymer systems for proton exchange membranes (PEMs). Chem. Rev. 104, 4587–4611. doi:10.1021/cr020711a
Hou, H. Y., Di Vona, M. L., and Knauth, P. (2012a). Building bridges: crosslinking of sulfonated aromatic polymers-A review. J. Memb. Sci. 423, 113–127. doi:10.1016/j.memsci.2012.07.038
Hou, H., Maranesi, B., Chailan, J.-F., Khadhraoui, M., Polini, R., Di Vona, M. L., et al. (2012b). Crosslinked SPEEK membranes: mechanical, thermal, and hydrothermal properties. J. Mater. Res. 27, 1950–1957. doi:10.1557/jmr.2012.151
Iojoiu, C., Marechal, M., Chabert, F., and Sanchez, J. Y. (2005). Mastering sulfonation of aromatic polysulfones: crucial for membranes for fuel cell application. Fuel Cells 5, 344–354. doi:10.1002/fuce.200400082
Iulianelli, A., and Basile, A. (2012). Sulfonated PEEK-based polymers in PEMFC and DMFC applications: a review. Int. J. Hydrogen Energy 37, 15241–15255. doi:10.1016/j.ijhydene.2012.07.063
Jiang, R. C., Kunz, H. R., and Fenton, J. M. (2005). Investigation of membrane property and fuel cell behavior with sulfonated poly(ether ether ketone) electrolyte: temperature and relative humidity effects. J. Power Sources 150, 120–128. doi:10.1016/j.jpowsour.2005.03.180
Karlsson, L. E., and Jannasch, P. (2005). Polysulfone ionomers for proton-conducting fuel cell membranes – 2. Sulfophenylated polysulfones and polyphenylsulfones. Electrochim. Acta 50, 1939–1946. doi:10.1016/j.electacta.2004.09.003
Kerres, J. A. (2005). Blended and cross-linked ionomer membranes for application in membrane fuel cells. Fuel Cells 5, 230–247. doi:10.1002/fuce.200400079
Kim, J.-D., Donnadio, A., Jun, M.-S., and Di Vona, M. L. (2013). Crosslinked SPES-SPPSU membranes for high temperature PEMFCs. Int. J. Hydrogen Energy 38, 1517–1523. doi:10.1016/j.ijhydene.2012.10.110
Knauth, P., Pasquini, L., Maranesi, B., Pelzer, K., Polini, R., and Di Vona, M. L. (2013). Proton mobility in sulfonated polyetheretherketone (SPEEK): influence of thermal crosslinking and annealing. Fuel Cells 13, 79–85. doi:10.1002/fuce.201200120
Knauth, P., Sgreccia, E., and Di Vona, M. L. (2014). Chemomechanics of acidic ionomers: hydration isotherms and physical model. J. Power Sources 267, 692–699. doi:10.1016/j.jpowsour.2014.05.127
Kreuer, K. D. (2000). On the complexity of proton conduction phenomena. Solid State Ion 136, 149–160. doi:10.1016/S0167-2738(00)00301-5
Linkous, C. A., Anderson, H. R., Kopitzke, R. W., and Nelson, G. L. (1998). Development of new proton exchange membrane electrolytes for water electrolysis at higher temperatures. Int. J. Hydrogen Energy 23, 525–529. doi:10.1016/S0360-3199(97)00113-4
Maranesi, B., Hou, H., Polini, R., Sgreccia, E., Alberti, G., Narducci, R., et al. (2013). Cross-linking of sulfonated poly(ether ether ketone) by thermal treatment: how does the reaction occur? Fuel Cells 13, 107–117. doi:10.1002/fuce.201200010
Mikhailenko, S. D., Robertson, G. P., Guiver, M. D., and Kaliaguine, S. (2006). Properties of PEMs based on cross-linked sulfonated poly(ether ether ketone). J. Memb. Sci. 285, 306–316. doi:10.1016/j.memsci.2006.08.036
Mikhailenko, S. D., Zaidi, S. M. J., and Kaliaguine, S. (2000). Electrical properties of sulfonated polyether ether ketone/polyetherimide blend membranes doped with inorganic acids. J. Polym. Sci. B Polym. Phys. 38, 1386–1395. doi:10.1002/(SICI)1099-0488(20000515)38:10<1386::AID-POLB160>3.3.CO;2-W
Paddison, S. J., and Paul, R. (2002). The nature of proton transport in fully hydrated Nafion (R). Phys. Chem. Chem. Phys. 4, 1158–1163. doi:10.1039/b109792j
Paik, Y., Chae, S. A., Han, O. H., Youp, H. S., and Yong, H. H. (2009). Influence of water and degree of sulfonation on the structure and dynamics of SPEEK studied by solid-state 13C and 1H NMR. Polymer 50, 2664–2673. doi:10.1016/j.polymer.2009.03.039
Parcero, E., Herrera, R., and Nunes, S. P. (2006). Phosphonated and sulfonated polyhphenylsulfone membranes for fuel cell application. J. Memb. Sci. 285, 206–213. doi:10.1016/j.memsci.2005.12.065
Peighambardoust, S. J., Rowshanzamir, S., and Amjadi, M. (2010). Review of the proton exchange membranes for fuel cell applications. Int. J. Hydrogen Energy 35, 9349–9384. doi:10.1016/j.ijhydene.2010.05.017
Premchand, Y. D., Di Vona, M. L., and Knauth, P. (2008). Proton Conducting Nanocomposite and Hybrid Polymers, in Nanocomposites: Ionic Conducting Materials and Structural Spectroscopies. New York: Springer.
Roziere, J., and Jones, D. J. (2003). Non-fluorinated polymer materials for proton exchange membrane fuel cells. Annu. Rev. Mater. Res. 33, 503–555. doi:10.1146/annurev.matsci.33.022702.154657
Sgreccia, E., Chailan, J. F., Khadhraoui, M., Di Vona, M. L., and Knauth, P. (2010). Mechanical properties of proton-conducting sulfonated aromatic polymer membranes: stress-strain tests and dynamical analysis. J. Power Sources 195, 7770–7775. doi:10.1016/j.jpowsour.2009.09.061
Sgreccia, E., Khadhraoui, M., de Bonis, C., Licoccia, S., Di Vona, M. L., and Knauth, P. (2008). Mechanical properties of hybrid proton conducting polymer blends based on sulfonated polyetheretherketones. J. Power Sources 178, 667–670. doi:10.1016/j.jpowsour.2007.09.099
Su, Y.-H., Liu, Y.-L., Sun, Y.-M., Lai, J.-Y., Wang, D.-M., Gao, Y., et al. (2007). Proton exchange membranes modified with sulfonated silica nanoparticles for direct methanol fuel cells. J. Memb. Sci. 296, 21–28. doi:10.1016/j.memsci.2007.03.007
Subianto, S., Pica, M., Casciola, M., Cojocaru, P., Merlo, L., Hards, G., et al. (2013). Physical and chemical modification routes leading to improved mechanical properties of perfluorosulfonic acid membranes for PEM fuel cells. J. Power Sources 233, 216–230. doi:10.1016/j.jpowsour.2012.12.121
Takamuku, S., and Jannasch, P. (2012). Properties and degradation of hydrocarbon fuel cell membranes: a comparative study of sulfonated poly(arylene ether sulfone)s with different positions of the acid groups. Polym. Chem. 3, 1202–1214. doi:10.1039/c2py00611a
Xing, P. X., Robertson, G. P., Guiver, M. D., Mikhailenko, S. D., Wang, K. P., and Kaliaguine, S. (2004). Synthesis and characterization of sulfonated poly(ether ether ketone) for proton exchange membranes. J. Memb. Sci. 229, 95–106. doi:10.1016/j.memsci.2003.09.019
Yee, R. S. L., Rozendal, R. A., Zhang, K., and Ladewig, B. P. (2012). Cost effective cation exchange membranes: a review. Chem. Eng. Res. Des. 90, 950–959. doi:10.1016/j.cherd.2011.10.015
Zhong, S. L., Cui, X. J., Cai, H. L., Fu, T. Z., Zhao, C., and Na, H. (2007). Crosslinked sulfonated poly(ether ether ketone) proton exchange membranes for direct methanol fuel cell applications. J. Power Sources 164, 65–72. doi:10.1016/j.jpowsour.2006.10.077
Keywords: SPEEK, reticulation reaction, mechanical properties, conductivity, memory effect
Citation: Di Vona ML, Sgreccia E, Narducci R, Pasquini L, Hou H and Knauth P (2014) Stabilized sulfonated aromatic polymers by in situ solvothermal cross-linking. Front. Energy Res. 2:39. doi: 10.3389/fenrg.2014.00039
Received: 02 July 2014; Accepted: 12 September 2014;
Published online: 10 October 2014.
Edited by:
Antonio Moran, Universidad de León, SpainReviewed by:
Enrica Fontananova, Institute on Membrane Technology of the National Research Council, ItalyAnna Donnadio, University of Perugia, Italy
Meng Ni, The Hong Kong Polytechnic University, Hong Kong
Copyright: © 2014 Di Vona, Sgreccia, Narducci, Pasquini, Hou and Knauth. This is an open-access article distributed under the terms of the Creative Commons Attribution License (CC BY). The use, distribution or reproduction in other forums is permitted, provided the original author(s) or licensor are credited and that the original publication in this journal is cited, in accordance with accepted academic practice. No use, distribution or reproduction is permitted which does not comply with these terms.
*Correspondence: Maria Luisa Di Vona, Dipartimento di Scienze e Tecnologie Chimiche, Università di Roma Tor Vergata, Via della Ricerca Scientifica 1, 00133 Roma, Italia e-mail: divona@uniroma2.it