- 1Department of Materials Science and Engineering, Zhejiang University, Hangzhou, China
- 2Ningbo Institute of Materials Technology and Engineering, Chinese Academy of Sciences, Ningbo, China
- 3School of Physical Science and Technology, ShanghaiTech University, Shanghai, China
The review presents an overview of the recent advances in inorganic solid lithium ion conductors, which are of great interest as solid electrolytes in all-solid-state lithium batteries. It is focused on two major categories: crystalline electrolytes and glass-based electrolytes. Important systems such as thio-LISICON Li10SnP2S12, garnet Li7La3Zr2O12, perovskite Li3xLa(2/3)−xTiO3, NASICON Li1.3Al0.3Ti1.7(PO4)3, and glass–ceramic xLi2S⋅(1 − x)P2S5 and their progress are described in great detail. Meanwhile, the review discusses different on-going strategies on enhancing conductivity, optimizing electrolyte/electrode interface, and improving cell performance.
Introduction
Lithium batteries are being scrutinized as the most promising electrical energy storage for electric vehicles (EVs), which hold great promise in resolving the jeopardy on future transportation. On the other hand, they have not yet been able to meet automobiles’ stringent requirement on high energy density, long cycle life, excellent safety, and wide operating temperature range (Goodenough and Kim, 2009; Kotobuki, 2012).
In order to get lithium batteries ready for their large-scale implementation in EVs, researchers extensively look at all aspects in a cell that would leapfrog the cell performance (e.g., novel electrolytes, high energy-density, and stable electrode materials, high-performance conductive additives/binders/current collectors, and efficient packaging). Among these approaches, the electrolyte holds the key to the success of EV batteries. The state-of-the-art electrolytes mainly consist of lithium salts and organic solvents. Therefore, they cause irreversible capacity losses resulted from the formation of stable solid electrolyte interphase (SEI), hinder the increase in cycle life, limit the temperature window, and, not to mention, pose severe safety concerns on lithium batteries.
In this respect, the replacement of currently used organic liquid electrolytes with inorganic solid electrolytes (SEs) is very appealing. For one thing, inorganic SEs are solid materials. Thus, they can address the above-mentioned concerns on capacity losses, cycle life, operation temperatures, safety and reliability (Hayashi et al., 2012; Sahu et al., 2014). In addition, they present advantages such as the simplicity of design, absence of leakage and pollution, better resistance to shocks and vibrations compared to organic liquid electrolytes (Thangadurai and Weppner, 2006b; Knauth, 2009; Fergus, 2010). For another thing, most of inorganic SEs are single ion conductors. Lithium single ion conductors can have a lithium transference number of unity. As a result, there is no concentration gradient inside the cell while it is operating. This is very beneficial to the lowering of cell overpotential (Quartarone and Mustarelli, 2011).
Also stemming from these two prominent features, great challenges remain for achieving high-performance SEs. One of them is how to create favorable solid–solid interface between electrode and electrolyte (Ohta et al., 2006, 2007; Sakuda et al., 2011). Another one is how to obtain high ionic conductivity at room temperature, e.g., 10−3 S cm−1.
In this review, the advantages, as well as efficient ways to addressing the aforementioned grant challenges, are discussed. The first part is about crystalline electrolytes, including LISICON and thio-LISICON-type, Garnet-type, Perovskite-type, and NASICON-type lithium ion conductors. The second part is about glass-based electrolytes, including glassy and glass-ceramic systems made of oxides and sulfides. Table 1 lists important materials and their conductivities and Figure 1 shows Arrhenius plots for ionic conductivities of some selected SEs.
Crystalline Inorganic Electrolytes
LISICON and Thio-LISICON-Type Electrolytes
LISICON-type SEs possess relatively low conductivity at room temperature (~10−7 S cm−1) and Li14ZnGe4O16 is its typical representative, which was first described by Hong (1978). Its one member of Li2+2xZn1−xGeO4 system and can be viewed as a Li4GeO4–Zn2GeO4 solid solution. Figure 2 shows the projection of the LISICON structure on the a-b plane, whose framework is related to the γ-Li3PO4 crystal structure. Li11ZnGe4O16 forms a three-dimensional (3D) skeleton structure and lithium ions in the skeleton distribute in two sites: 4c and 8d sites. These sites are occupied by four and seven Li+ ions, respectively. The three remaining Li+ ions are located in 4c and 4a interstitial sites and their temperature coefficients are abnormally high, indicating that they can be mobile. Each 4a site is connected to two 4c sites and vice versa. The bottlenecks to Li+ transport between these sites are parallelograms, which have a tilt angle with the a-b plane. On the basis of calculation, the average size of the bottlenecks (4.38 Å) is bigger than the minimum size required for Li+ transport (2rLi + 2ro = 4.0 Å), which promotes Li+ movement (Zheng et al., 2003).
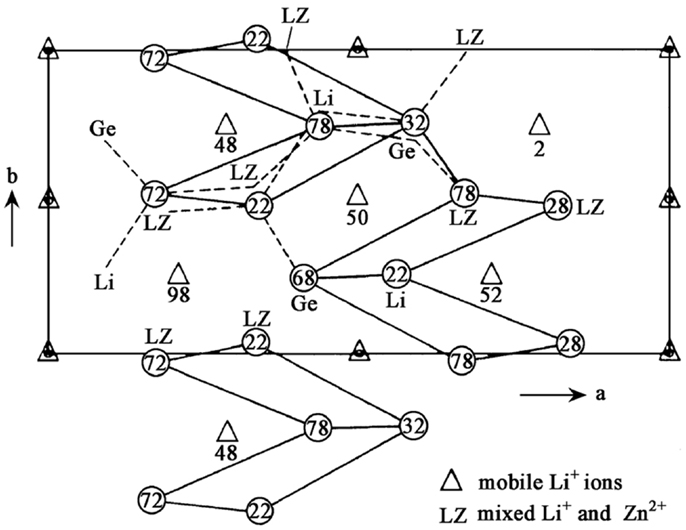
Figure 2. Projection of the LISICON structure on the a-b plane. Reproduced with permission of Hong (1978).
Although the ionic conductivity of Li14ZnGe4O16 is as high as 0.125 S cm−1 at 300°C, it is only 10−7 S cm−1 at room temperature. This is explained by trapping of the mobile Li+ ions by the immobile sublattice at lower temperatures via the formation of defect complexes (Robertson et al., 1997). Furthermore, Li14ZnGe4O16 is highly reactive with Li-metal and atmospheric CO2 and the conductivity decreases with time (Thangadurai and Weppner, 2006b).
Recent efforts to improve the ionic conductivity of LISICON-type SEs focus on the replacement of oxide by sulfur in the framework (Figure 3). These sulfide SEs are referred to as thio-LISICON, which was introduced by Kanno et al. (2000).
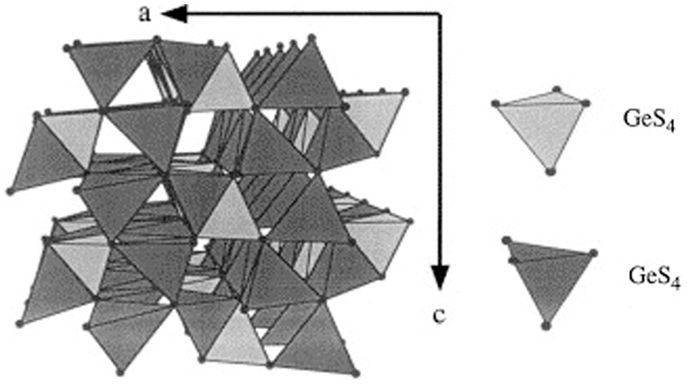
Figure 3. Structure of Li4GeS4-a parent structure of the new thio-LISICON family. Reproduced with permission of Kanno et al. (2000).
Material design of inorganic SEs are based on certain structural criteria: (i) mobile ions should have conduction pathways large enough in the lattice, (ii) there should be a disordered mobile ion sublattice, and (iii) highly polarizable mobile ions and anion sublattices are preferable (Kanno and Murayama, 2001). The ionic conduction properties are strongly dominated by the size and polarizability of constituent ions, or interstitial vacancy character caused by the substitutions.
Since the radius of S2− is higher than O2−, this substitution can significantly enlarge the size of Li+ transport bottlenecks. Besides, S2− has better polarization capability than O2−, thus weakens the interaction between skeleton and Li+ ions. Therefore, compared with LISICON systems, thio-LISICON materials can achieve really high ionic conductivity (over 10−4 S cm−1 at room temperature). Thio-LISICON SEs also have advantages, such as easy reduction of grain-boundary resistance by conventional cold-press of electrolyte powers and preferable application to all-solid-state batteries due to its mechanical property (Tatsumisago et al., 2013).
A series of thio-LISICON were firstly synthesized and Li3.25Ge0.25P0.75S4 showed a high conductivity of 2.2 × 10−3 S cm−1 at room temperature, negligible electronic conductivity, high electrochemical stability, and no phase transition up to 500°C (Kanno and Murayama, 2001). Most recently, a very high conductivity of 12 m S cm−1 (27°C) was achieved by Li10GeP2S12. Its crystal structure was different from typical thio-LISICON structures. As shown in Figure 4A, it had a 3D framework structure consisting of (Ge0.5P0.5)S4 tetrahedra, PS4 tetrahedra, LiS4 tetrahedra, and LiS6 octahedra. The high ionic conductivity benefited from the 3D diffusion pathways both along c axis and in a-b plane (Kamaya et al., 2011).
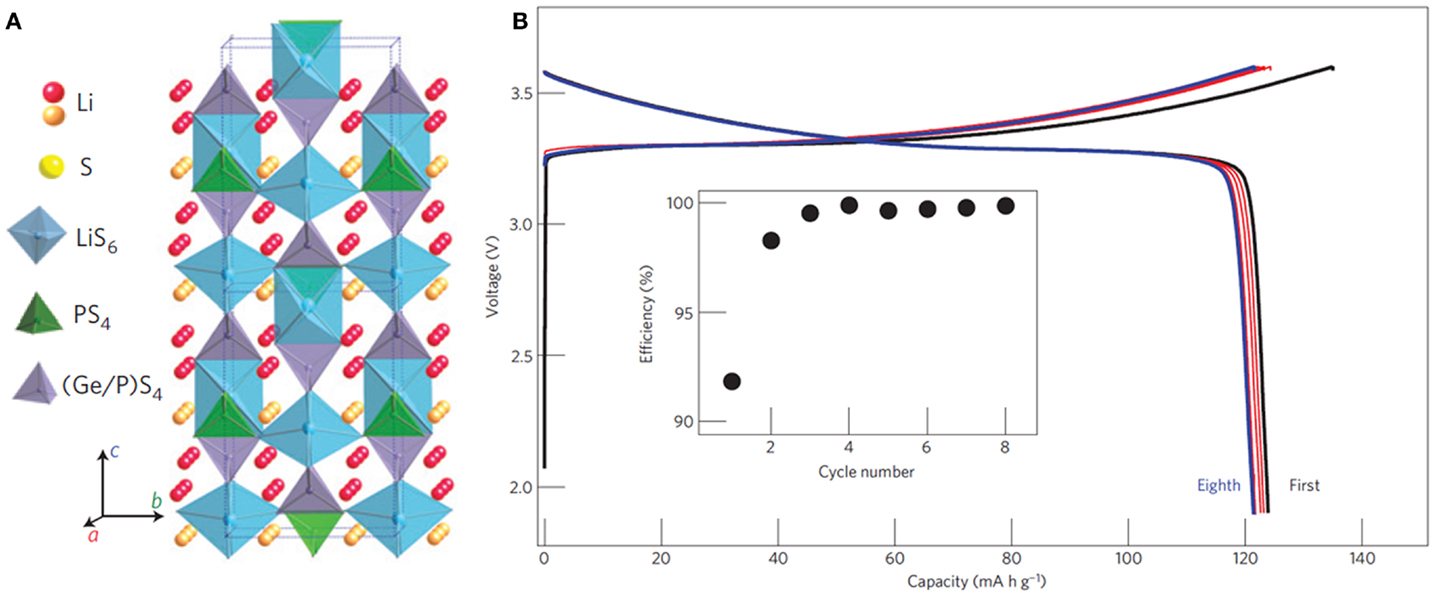
Figure 4. (A) Crystal structure of Li10GeP2S12. (B) Charge–discharge curves of an all-solid-state battery with this SE. Reproduced with permission of Kamaya et al. (2011).
Furthermore, Bron et al. (2013) reported the synthesis of Li10SnP2S12 by replacing Ge with Sn, whose total conductivity reached 4 m S cm−1 at room temperature. All-solid-state battery with Li10GeP2S12 (cathode: LiCoO2; anode: in metal) exhibited a discharge capacity of over 120 mA g−1 and an excellent coulombic efficiency of about 100% after the second cycle as well as a high decomposition potential of over 5 V (Figure 4B).
Garnet-Type Electrolytes
Garnet-type lithium single ion conductors have a general formula of Li5La3M2O12 (M = Ta, Nb). They were firstly reported by Thangadurai and Weppner (2005a) and recently have been intensively studied as SEs for all-solid-state lithium batteries. They have high ionic conductivity and excellent chemical stability in contact with lithium metal.
Figure 5 shows the crystal structure of Li5La3M2O12. La and M occupy eight- and six-coordinated sites, respectively, and Li occupies octahedral sites. The MO6 octahedra are surrounded by six lithium ions and two Li+ vacancies in the parent Li5La3M2O12 structure. Therefore, the structure facilitates lithium ion conduction (Thangadurai and Weppner, 2005b).
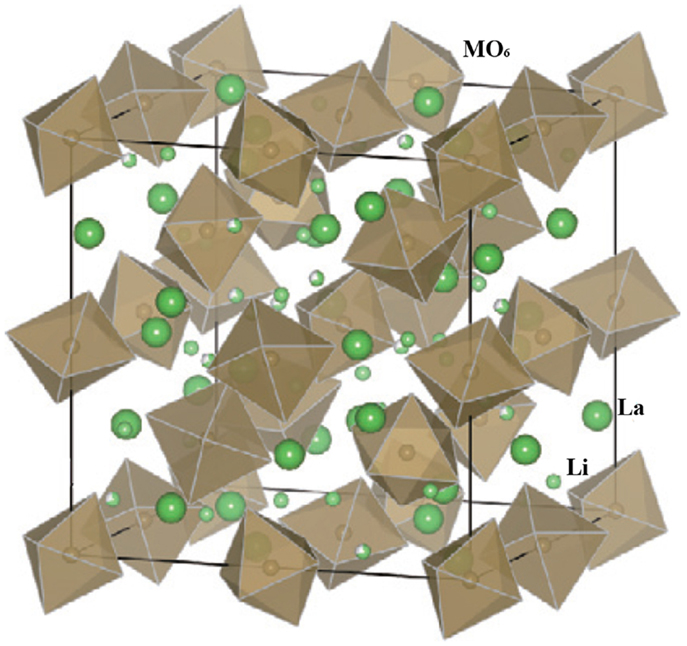
Figure 5. Crystal structure of parent garnet-like Li5La3M2O12. Reproduced with permission of Takada (2009).
Among the materials investigated, Li6BaLa2Ta2O12 exhibited a high ionic conductivity of 4 × 10−5 S cm−1 at 22°C with an activation energy of 38.5 kJ mol−1. It had low grain-boundary resistance, which meant that the total and bulk conductivities were nearly identical (Thangadurai and Weppner, 2005b).
Conductivity can be further improved through partially substitution of Y or In at the M site in Li5La3M2O12. For example, Li5.5La3Nb1.75In0.25O12 showed an enhanced conductivity (1.8 × 10−4 S cm−1 at 50°C) with low activation energy of 49.1 kJ mol−1 (Thangadurai and Weppner, 2006a). High conductivity of 2.7 × 10−4 S cm−1 at 25°C was obtained for Li5+2xLa3Nb2−xYxO12 with x = 0.75 (Narayanan et al., 2012). The high Li+ conductivity resulted from short Li+–Li+ distances in the edge-sharing LiO6 octahedra and a high concentration of Li on the octahedral sites.
Recently, garnet-type Li7La3Zr2O12 (LLZO) has attracted much attention since firstly reported (Murugan et al., 2007). In the structure, La is located at the center of a dodecahedron with eight coordinated oxygen atoms while Zr is at the center of an octahedron with six-coordinated oxygen atoms (Figure 6). The lithium ions could migrate within the garnet lattice framework with a 3D conduction mechanism (Dumon et al., 2013).
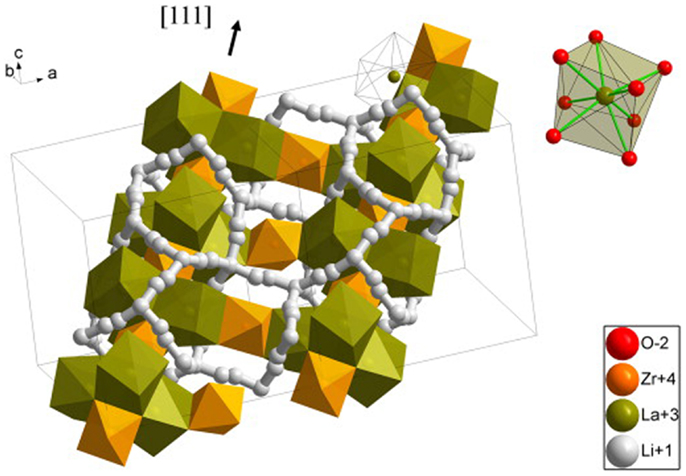
Figure 6. Crystallographic structure of cubic LLZO. Reproduced with permission of Dumon et al. (2013).
LLZO undergoes a phase change from tetragonal to cubic structure as the sintering temperature increases, which belong to the space group Iad and I41A/cd, respectively. Conductivity of the cubic phase (10−4 S cm−1, room temperature) is about two orders of magnitude higher than that of the tetragonal phase (Kokal et al., 2011; Tietz et al., 2013).
Therefore, the challenge has been to stabilize the cubic phase, which can be achieved by doping. Geiger et al. (2011) firstly suggested the important role Al could play to stabilize the cubic phase relative to the tetragonal phase. Then Düvel et al. (2012) described in depth the effects of Al incorporation on both structural and dynamic properties of LLZO. It was suggested that at low Al concentrations Al3+ ions acted as an aliovalent dopant by replacing three Li+ ions. However, with increasing Al content, La3+ and Zr4+ ions were progressively replaced by Al ions. The substitution of La3+ and Zr4+ with Al3+ ions stabilized the cubic phase and greatly affected the corresponding Li ion dynamics. Similar stabilization of the cubic phase was observed with Ga and Ta substitution. Allen et al. (2012) recently reported Li6.75La3Zr1.75Ta0.25O12 cubic garnet with relatively high total Li+ conductivity (8.7 × 10−4 S cm−1 at 25°C).
Owing to its high ionic conductivity, excellent stability with Li and wide electrochemical voltage window (Ishiguro et al., 2013; Jin and McGinn, 2013b), LLZO has been successfully used to fabricate all-solid-state lithium batteries. Jin and McGinn (2013a) reported a Cu0.1V2O5/LLZO/Li all-solid-state battery, which exhibited an initial discharging capacity of 93 mA h g−1 at 10 μA cm−2 (at 50°C). A full cell consisting of a LiCoO2 cathode, a Li6.75La3Zr1.75Nb0.25O12 electrolyte and Li-metal anode exhibited stable cycle performance (Figure 7). Its discharge capacities were 129 mA h g−1 at the 1st cycle and 127 mA h g−1 at the 100th cycle, respectively (Ohta et al., 2012).
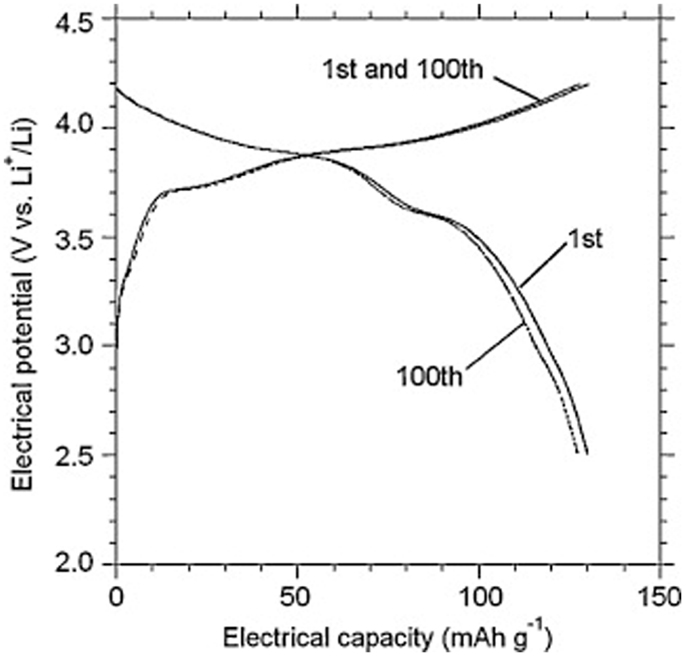
Figure 7. Charge-discharge curves for the LiCoO2/Li6.75La3Zr1.75Nb0.25 O12/Li cell. Reproduced with permission of Ohta et al. (2012).
Perovskite-Type Electrolytes
The lithium–lanthanum–titanates, Li3xLa(2/3)−x□(1/3)−2xTiO3 (LLTO, 0 < x < 0.16), with the perovskite structure (ABO3), are characteristic of high bulk conductivity, of the order of 10−3 S cm−1 at room temperature (Bohnke, 2008).
LLTO consists of a mixture of phases, i.e., a high-temperature phase with cubic Pm3m symmetry (α-LLTO) and a lower temperature β-LLTO phase having tetragonal P4/mmm symmetry. Figure 8 shows the crystal structure of LLTO. The A site cations, which were Li+ and La3+ in the cubic α-LLTO phase, were randomly distributed, while the A sites of the ordered β-LLTO had a doubled perovskite structure, with an alternating arrangement of La-rich and Li-vacancy-rich layers along the c axis (Gao et al., 2013; Teranishi et al., 2013). It is believed that the conductivity of LLTO electrolytes is mainly governed by two factors: bottleneck size and site percolation. The crystal structure of tetragonal LLTO explains the high Li+ conductivity by the large concentration of A site vacancies, allowing motion of lithium ions by a vacancy mechanism and through square planar bottleneck between A sites, formed by four O2- ions between two neighboring A sites (Alonso et al., 2000).
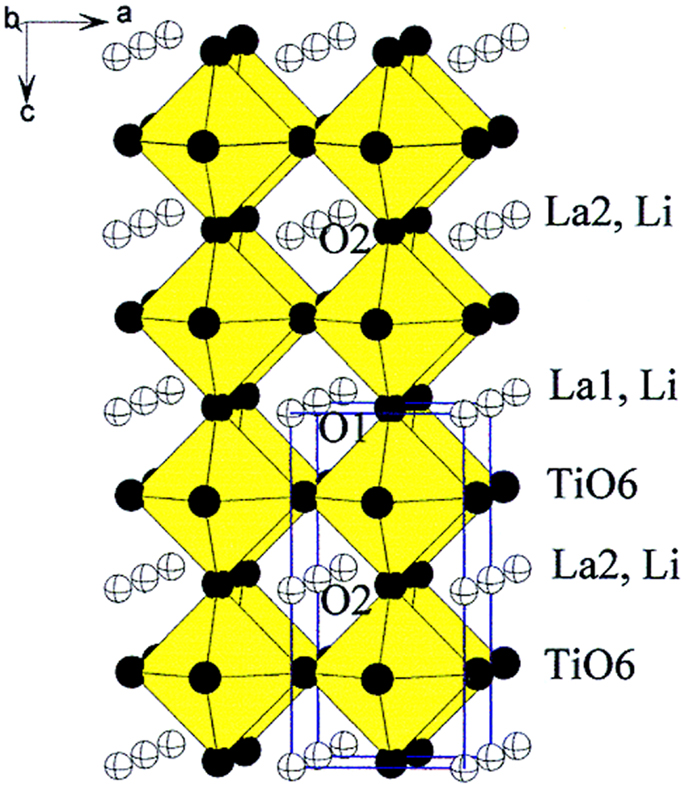
Figure 8. Crystal structure of tetragonal LLTO. Reproduced with permission of Stramare et al. (2003).
LLTO-based SEs have many advantages, such as lithium single ion conductors, negligible electronic conductivity, high electrochemical stability (>8 V), stability in dry and hydrated atmospheres, and stability over a wide temperature range from 4 K to 1600 K (Bohnke, 2008). However, there are two major challenges for LLTO electrolytes, i.e., relatively low grain-boundary conductivity (<10−5 S cm−1) and the instability against Li metal anode (Ban and Choi, 2001).
Thus, it is of utmost importance to increase the grain-boundary conductivity of LLTO electrolytes. It has been reported that the introduction of silica (Mei et al., 2010) and LLZO (Chen et al., 2012, 2013) could modify the grain-boundary layer of LLTO, and the total ionic conductivity could be over 1 × 10−4 and 1.2 × 10−4 S cm−1 at room temperature, respectively. A high conductivity was also achieved by doping Al (Morata-Orrantia et al., 2003) or Nb (Teranishi et al., 2013), whereas the addition of Ag (Abhilash et al., 2013) led to a decrease in conductivity. The replacement of some oxygen with fluorine did not significantly affect the conductivity (Fergus, 2010). Furthermore, effective sintering to decrease grain boundary is important to improve total conductivity (Vidal et al., 2014).
Another issue that lies with LLTO is its instability against Li metal anode. Lithium can be intercalated into LLTO at a potential below about 1.7–1.8 V vs. Li (Chen and Amine, 2001), which causes the reduction of Ti4+ to Ti3+ and induces high electronic conductivity. Nevertheless, chemical substitutional studies have been undergoing motivated by the discovery of new applications for LLTO compounds in future figurations of lithium ion batteries: as cathode coatings (Qian et al., 2012) or electrolyte separators (Inaguma and Nakashima, 2013). As shown in Figure 9, the stable discharge/charge behaviors of a rechargeable lithium/air cell with LLTO separator were confirmed.
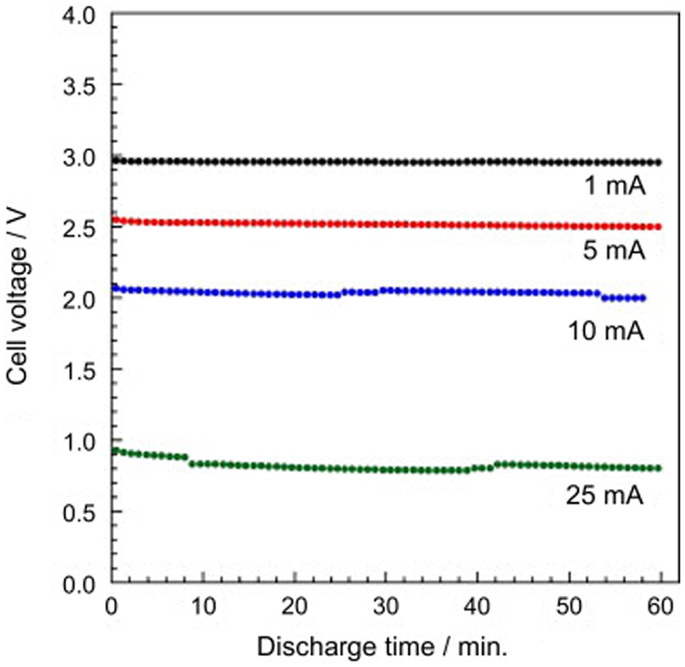
Figure 9. Discharge curve of the lithium-air battery using LLTO as a separator at various currents. Reproduced with permission of Inaguma and Nakashima (2013).
NASICON-Type Electrolytes
The term NASICON, which stands for Na+ super ionic conductors, was first given to the solid solution phase Na1+xZr2SixP3−xO12, x = 2.0, discovered by Hong (1976). The general formula of NASICON-type SE can be described as LiA2IV(PO4)3 (AIV = Ti, Zr, Ge, Hf).
In the structure, AO6 octahedra are linked by PO4 tetrahedra to form 3D interconnected channels and two types of interstitial positions (M′ and M″) where mobile cations are distributed, as shown in Figure 10. The mobile cations move from one site to another through bottlenecks, the size of which depends on the nature of the skeleton ions and on the carrier concentration in both type of sites (M′ and M″) (Cretin and Fabry, 1999).
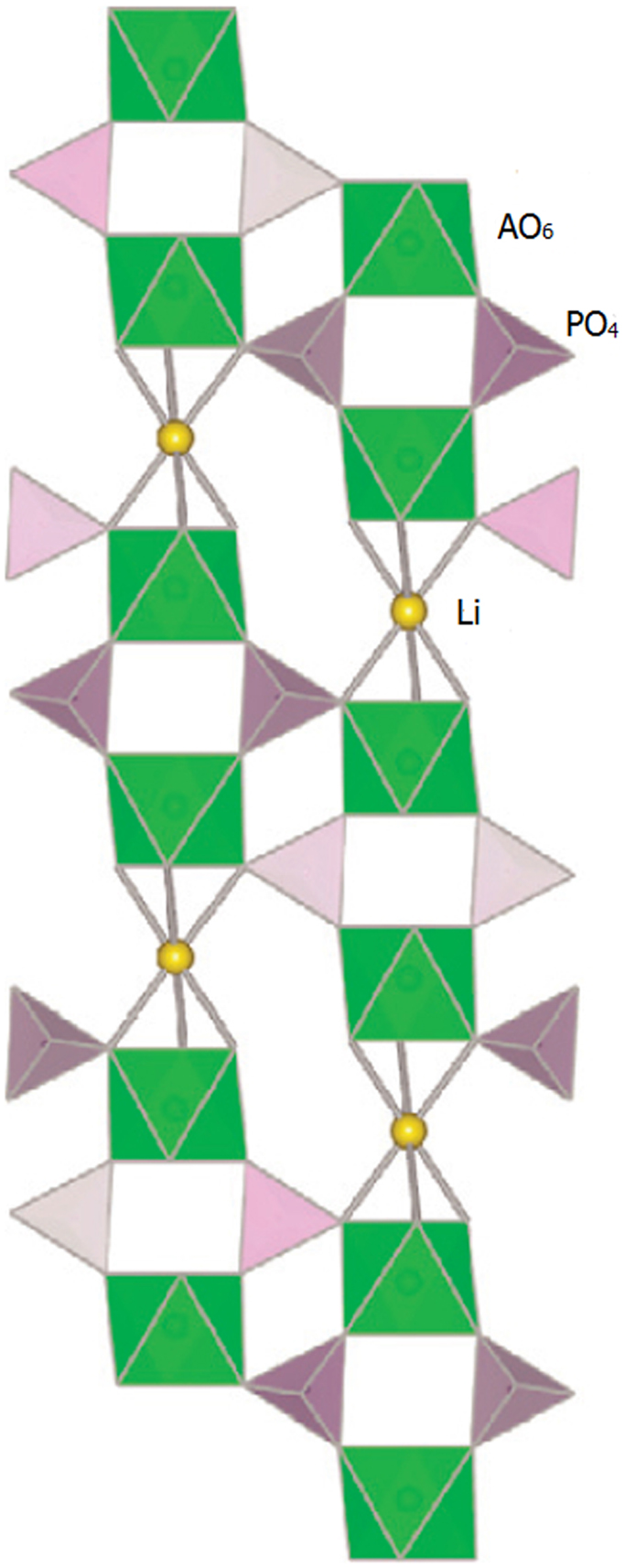
Figure 10. Crystal structure of NASICON. Reproduced with permission of Takada (2009).
Among the LiA2IV(PO4)3 NASICONs, the systems with Ti exhibited high Li+ conductivity (about 10−5 S cm−1 at room temperature) (Takada, 2009). This could be explained by the ionic radius of Li+ matching well with the size of skeleton framework, which consists of TiO6 octahedra. Great efforts have been made for maximizing the ionic conductivity of LiA2IV(PO4)3 systems, especially LiTi2(PO4)3. An increase in conductivity was observed when Ti4+ was partially substituted by Al3+ in Li1+xAlxTi2−x(PO4)3 (LATP) (Key et al., 2012; Duluard et al., 2013; Morimoto et al., 2013) or when P5+ was substituted by Si4+ in Li1+x+yAlxTi2−xSiyP3−yO12 (Fu, 1997; Tan et al., 2012). Conductivity was enhanced significantly to 3 × 10−3 S cm−1 for Li1.3Al0.3Ti1.7(PO4)3 at room temperature.
Due to their excellent Li+ conductivity and stability in air and water, LiTi2(PO4)3-based SEs have been applied to electrochemical energy devices, such as all-solid-state lithium batteries (Yada et al., 2009) and lithium/air secondary batteries (Shimonishi et al., 2011). Besides, NASICON-type SEs can have high electrochemical oxidative voltage. For example, LiGe2(PO4)3-based SEs were reported to show high electrochemical oxidative voltage of about 6 V (vs. Li/Li+) (Xu et al., 2007), as shown in Figure 11. However, similar to LLTO, LiTi2(PO4)3-based SEs are instable toward Li metal, with the reduction of Ti4+ to Ti3+ (Hartmann et al., 2013).
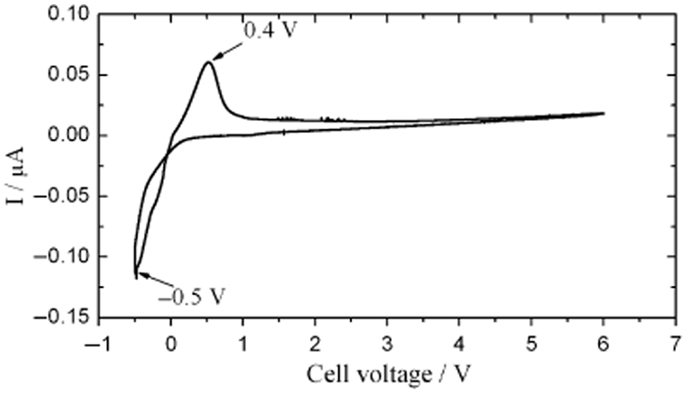
Figure 11. Cyclic voltammogram of LiGe2(PO4)3-based SEs. Reproduced with permission of Xu et al. (2007).
Glass-Based Inorganic Electrolytes
Glassy Electrolytes
The glassy electrolytes have attracted much attention mainly due to their several advantages over the crystalline materials: isotropic ionic conduction, no grain-boundary resistance, ease to be fabricated into film, a wide range of compositions, etc. (Ravaine, 1980; Minami, 1987). In addition, the ion conductivity of amorphous glasses is generally higher than that of the corresponding crystalline ones because of their so-called open structure (Tatsumisago, 2004), as shown in Figure 12.
Generally, lithium ion conducting glasses can be divided into two categories: oxide and sulfide. For most of the oxide glassy electrolytes, the lithium ion conductivity at room temperature is too low to be practical for high energy batteries, normally on the order of 10−6 ~ 10−8 S cm−1 (Tatsumisago et al., 1987; Lee et al., 2002). While in sulfide glasses, high lithium ion conductivities of 10−3 ~ 10−5 S cm−1 at room temperature can be achieved due to high polarizability of sulfur ions, such as Li2S–SiS2 and Li2S–P2S5 (Machida and Shigematsu, 2004; Tatsumisago, 2004; Ohtomo et al., 2013c).
However, these sulfide glass electrolytes can react with ambient moisture and generate H2S gas (Knauth, 2009). Therefore, handling of sulfide SEs must be done in an inert atmosphere. However, partial substitution of oxygen atoms for sulfur atoms in sulfide electrolytes can be effective in suppressing H2S gas generation (Ohtomo et al., 2013b). Very recently, Hayashi et al. (2014) reported a composite electrolyte with 90 mol% of 75Li2S·21P2S5·4P2O5 glass and 10 mol% ZnO via mechanical milling. In the work, partial substitution of P2O5 for P2S5, as well as the addition of ZnO, decreased the rate of H2S generation when exposed to air. The conductivity on the other hand decreased with the addition of P2O5.
In order to improve the conductivity of glassy electrolytes, several approaches have been proposed. One effective way is to mix two different anion species, so called “mixed anion effect” or “mixed former effect” (Tatsumisago et al., 1987; Raguenet et al., 2012). For instance, the addition of network former or modifier SeO2 into binary Li2O–B2O3 glassy electrolyte led to the increase in ionic conductivity at room temperature from 1.2 × 10−8 to 8 × 10−7 S cm−1 (Lee et al., 2002). The addition of lithium salts such as lithium halides (Ujiie et al., 2012) and lithium ortho-oxosalts (Aotani et al., 1994) is another effective way to enhance the conductivity of glassy electrolytes, because of the increase in lithium concentration and the decrease in activation energy for conduction. For example, the lithium ion conductivity of 67Li2S·33P2S5 glass at room temperature could increase from 10−4 S cm−1 to 10−3 S cm−1 by adding 45 mol% of LiI (Mercier et al., 1981).
However, increasing the amount of network modifiers with lithium ions facilitates the crystallization of glass. Thus the glasses with large amount of lithium ions are often prepared by twin-roller rapid quenching (Tatsumisago et al., 1981; Hayashi et al., 2002). This technique allows a cooling rate as high as 106 K S−1 (Tatsumisago and Hayashi, 2009) to prevent crystallization.
In all-solid-state batteries, the glasses need to be ground into fine powders by mechanical milling techniques (Morimoto et al., 1999) in order to make good contact with the electrodes. Mechanical milling also is a commonly used method to form amorphous materials (Hayashi et al., 2001; Ohtomo et al., 2013a,b). It has two major advantages: the process is very simple and the synthesis can be performed at room temperature.
Glass–Ceramic Electrolytes
Glass–ceramic electrolyte can be produced by the crystallization of a precursor glass. The crystallization usually would decrease the ionic conductivity, but the precipitation of a superionic conducting crystal from a precursor glass can enhance ionic conductivity. The grain-boundaries around crystal domains in glass–ceramics are filled with amorphous phases. Thus, glass–ceramic electrolytes usually have lower grain-boundary resistance than polycrystalline systems do (Tatsumisago et al., 2013).
Similar to the case of glassy electrolytes, the glass–ceramics also can be categorized into oxides and sulfides. For glass–ceramic oxides, NASICON-type systems have been studied the most, such as LATP (Fu, 1997b; Kotobuki and Koishi, 2013; Patil et al., 2013) and LAGP (Fu, 1997a; Nikolic et al., 2013; He et al., 2014) glass–ceramics. Their ionic conductivities at room temperature can reach 10−3 ~ 10−4 S cm−1.
Sulfide glass–ceramics can have higher ionic conductivity than that of oxides due to the large ionic radius and high polarizability of sulfur ions than those of oxide ions. For example, the conductivity of Li2S–P2S5 glass–ceramics can reach 10−3 S cm−1 at room temperature (Tatsumisago et al., 2002).
A superionic crystal with a structure analogous to that of the thio-LISICON phases can be precipitated by mechanical milling Li2S–P2S5 glasses (Hayashi et al., 2003). Very recently, Seino et al. (2014) reported a Li2S–P2S5 glass-ceramic conductor that had a very high ionic conductivity of 1.7 × 10−2 S cm−1 at room temperature by optimized heat treatment. The optimized conditions of heat treatment reduced the grain-boundary resistance and thus the total conductivity was five-time higher than that previously reported for the Li2S–P2S5 system (Mizuno et al., 2005). As shown in Figure 13, it suggested that the densification process increased overall conductivity, not by enhancing ionic conduction in the bulk, but reducing the grain-boundary resistance.
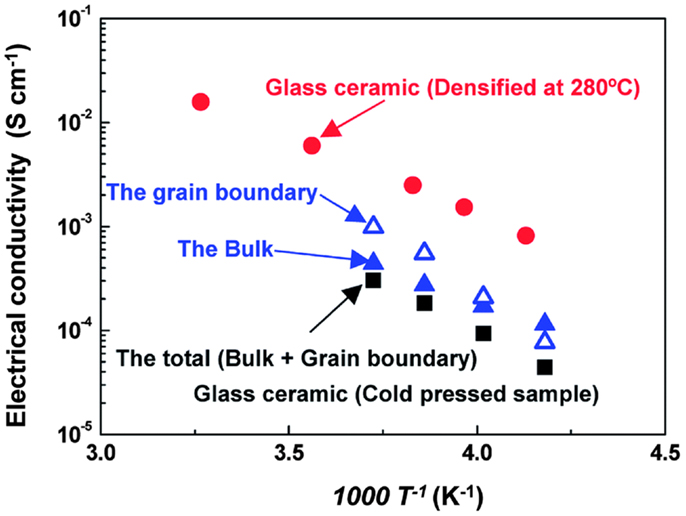
Figure 13. Temperature dependency of the bulk and grain-boundary resistances of the cold-pressed glass–ceramic material. Reproduced with permission of Seino et al. (2014).
Concluding Remarks
Solid electrolytes are being viewed as a necessary component for a safe and high-performance lithium battery in future, thereby drawing extensive attention in the field. Many efforts have been made to advance their performance.
Since the high resistance at the electrode/SE interface is one crucial issue for the development of high power all-solid-state lithium batteries, it is of utmost importance to form a favorable contact between electrodes and the electrolyte. Both the achievement of close contact and the increase in contact area are essential (Tatsumisago et al., 2013). Coating of SE thin films on electrode active materials is being explored as an effective route. For instance, Li2S–GeS2 sulfide electrolyte thin films with the conductivity of 1.8 × 10−4 S cm−1 on LiCoO2 particles were prepared by a pulsed laser deposition (PLD) technique (Ito et al., 2013). From a cross-sectional SEM image, this obtained thin film was dense and attached firmly to the Si substrate. Besides, other techniques, such as preparation of nanocomposites by a ball milling process (Nagao et al., 2012) or utilization of supercooled liquid of glass electrolyte (Kitaura et al., 2011), are documented to be effective in forming an ideal interface between electrodes and the electrolyte.
Regarding the important conductivity, the systems based on sulfur chemistries can show higher ionic conductivities than oxides. For example, in Table 1, high ionic conductivities on the order of 10−2 S cm−1 are achieved in sulfide systems, like thio-LISICON Li10GeP2S12 and Li2S–P2S5 glass–ceramics. On the other hand, sulfides usually are chemically instable and require extra attention to handle. In addition, several effective ways to increasing ionic conductivity have been developed, such as doping, hot isotactic pressing to reduce the grain-boundary resistance, the utilization of “mixed former effect,” and the precipitation of superionic crystals from glassy electrolytes.
Conflict of Interest Statement
The authors declare that the research was conducted in the absence of any commercial or financial relationships that could be construed as a potential conflict of interest.
Acknowledgments
This work is supported by the “Strategic Priority Research Program” of the Chinese Project Academy of Science, Grant No. XDA01020304, the National Natural Science Foundation of China (Grant No. 51371186), Ningbo 3315 International Team of Advanced Energy Storage Materials, Zhejiang Province Key Science and Technology Innovation Team.
References
Abhilash, K. P., Selvin, P. C., Nalini, B., Nithyadharseni, P., and Pillai, B. C. (2013). Investigations on pure and Ag doped lithium lanthanum titanate (LLTO) nanocrystalline ceramic electrolytes for rechargeable lithium-ion batteries. Ceram. Int. 39, 947–952. doi: 10.1016/j.ceramint.2012.07.011
Allen, J. L., Wolfenstine, J., Rangasamy, E., and Sakamoto, J. (2012). Effect of substitution (Ta, Al, Ga) on the conductivity of Li7La3Zr2O12. J. Power Sources 206, 315–319. doi:10.1016/j.jpowsour.2012.01.131
Alonso, J., Sanz, J., Santamaria, J., Leon, C., Varez, A., and Fernandez-Diaz, M. (2000). On the location of Li+ cations in the fast Li-cation conductor La0.5Li0.5TiO3 perovskite. Angew. Chem. Int. Ed. 39, 619–621. doi:10.1002/(SICI)1521-3773(20000204)39:3<619::AID-ANIE619>3.0.CO;2-O
Aono, H., Sugimoto, E., Sadaoka, Y., Imanaka, N., and Adachi, G. (1990). Ionic conductivity of solid electrolytes based on lithium titanium phosphate. J. Electrochem. Soc. 137, 1023–1027. doi:10.1149/1.2086597
Aotani, N., Iwamoto, K., Takada, K., and Kondo, S. (1994). Synthesis and electrochemical properties of lithium ion conductive glass, Li3PO4-Li2S-SiS2. Solid State Ionics 68, 35–39. doi:10.1016/0167-2738(94)90232-1
Ban, C. W., and Choi, G. M. (2001). The effect of sintering on the grain boundary conductivity of lithium lanthanum titanates. Solid State Ionics 140, 285–292. doi:10.1016/S0167-2738(01)00821-9
Bohnke, O. (2008). The fast lithium-ion conducting oxides Li3xLa2/3-xTiO3 from fundamentals to application. Solid State Ionics 179, 9–15. doi:10.1016/j.ssi.2007.12.022
Bron, P., Johansson, S., Zick, K., Schmedt Auf Der Gunne, J., Dehnen, S., and Roling, B. (2013). Li10SnP2S12: an affordable lithium superionic conductor. J. Am. Chem. Soc. 135, 15694–15697. doi:10.1021/ja407393y
Chen, C. H., and Amine, K. (2001). Ionic conductivity, lithium insertion and extraction of lanthanum lithium titanate. Solid State Ionics 144, 51–57. doi:10.1016/S0167-2738(01)00884-0
Chen, K., Huang, M., Shen, Y., Lin, Y., and Nan, C. W. (2012). Enhancing ionic conductivity of Li0.35La0.55TiO3 ceramics by introducing Li7La3Zr2O12. Electrochim. Acta 80, 133–139. doi:10.1016/j.electacta.2012.06.115
Chen, K., Huang, M., Shen, Y., Lin, Y. H., and Nan, C. W. (2013). Improving ionic conductivity of Li0.35La0.55TiO3 ceramics by introducing Li7La3Zr2O12 sol into the precursor powder. Solid State Ionics 235, 8–13. doi:10.1016/j.ssi.2013.01.007
Cretin, M., and Fabry, P. (1999). Comparative study of lithium ion conductors in the system Li1+xAlxA2-xIV(PO4)3 with AIV = Ti or Ge and 0 ≤x ≤0.7 for use as Li+ sensitive membranes. J. Eur. Ceram. Soc. 19, 2931–2940. doi:10.1016/S0955-2219(99)00055-2
Duluard, S., Paillassa, A., Puech, L., Vinatier, P., Turq, V., Rozier, P., et al. (2013). Lithium conducting solid electrolyte Li1.3Al0.3Ti1.7(PO4)3 obtained via solution chemistry. J. Eur. Ceram. Soc. 33, 1145–1153. doi:10.1016/j.jeurceramsoc.2012.08.005
Dumon, A., Huang, M., Shen, Y., and Nan, C. W. (2013). High Li ion conductivity in strontium doped Li7La3Zr2O12 garnet. Solid State Ionics 243, 36–41. doi:10.1016/j.ssi.2013.04.016
Düvel, A., Kuhn, A., Robben, L., Wilkening, M., and Heitjans, P. (2012). Mechanosynthesis of solid electrolytes: preparation, characterization, and Li ion transport properties of garnet-type Al-doped Li7La3Zr2O12 crystallizing with cubic symmetry. J. Phys. Chem. C 116, 15192–15202. doi:10.1021/jp301193r
Fergus, J. W. (2010). Ceramic and polymeric solid electrolytes for lithium-ion batteries. J. Power Sources 195, 4554–4569. doi:10.1016/j.jpowsour.2010.01.076
Fu, J. (1997). Fast Li+ ion conduction in Li2O-A12O3-TiO2-SiO2-P205 glass-ceramics. J. Am. Ceram. Soc. 80, 1901–1903. doi:10.1111/j.1151-2916.1997.tb03070.x
Fu, J. (1997a). Fast Li+ ion conducting glass-ceramics in the system Li2O-Al2 O3-GeO2-P2O5. Solid State Ionics 104, 191–194. doi:10.1016/S0167-2738(97)00434-7
Fu, J. (1997b). Superionic conductivity of glass-ceramics in the system Li2O-Al2O3-TiO2-P2O5. Solid State Ionics 96, 195–200. doi:10.1016/S0167-2738(97)00018-0
Gao, X., Fisher, C. A. J., Kimura, T., Ikuhara, Y. H., Moriwake, H., Kuwabara, A., et al. (2013). Lithium atom and A-site vacancy distributions in lanthanum lithium titanate. Chem. Mater. 25, 1607–1614. doi:10.1021/cm3041357
Geiger, C. A., Alekseev, E., Lazic, B., Fisch, M., Armbruster, T., Langner, R., et al. (2011). Crystal chemistry and stability of “Li7La3Zr2O12” garnet: a fast lithium-ion conductor. Inorg. Chem. 50, 1089–1097. doi:10.1021/ic101914e
Goodenough, J. B., and Kim, Y. (2009). Challenges for rechargeable Li batteries. Chem. Mater. 22, 587–603. doi:10.1021/cm901452z
Hartmann, P., Leichtweiss, T., Busche, M. R., Schneider, M., Reich, M., Sann, J., et al. (2013). Degradation of NASICON-type materials in contact with lithium metal: formation of mixed conducting interphases (MCI) on solid electrolytes. J. Phys. Chem. C 117, 21064–21074. doi:10.1021/jp4051275
Hayashi, A., Hama, S., Minami, T., and Tatsumisago, M. (2003). Formation of superionic crystals from mechanically milled Li2S-P2S5 glasses. Electrochem. Commun. 5, 111–114. doi:10.1016/S1388-2481(02)00555-6
Hayashi, A., Hama, S., Morimoto, H., Tatsumisago, M., and Minami, T. (2001). Preparation of Li2S-P2S5 amorphous solid electrolytes by mechanical milling. J. Am. Ceram. Soc. 84, 477–479. doi:10.1111/j.1151-2916.2001.tb00685.x
Hayashi, A., Komiya, R., Tatsumisago, M., and Minami, T. (2002). Characterization of Li2S-SiS2-Li3MO3 (M=B, Al, Ga and In) oxysulfide glasses and their application to solid state lithium secondary batteries. Solid State Ionics 15, 285–290. doi:10.1016/S0167-2738(02)00313-2
Hayashi, A., Muramatsu, H., Ohtomo, T., Hama, S., and Tatsumisago, M. (2014). Improved chemical stability and cyclability in Li2S-P2S5-P2O5-ZnO composite electrolytes for all-solid-state rechargeable lithium batteries. J. Alloy. Compd. 591, 247–250. doi:10.1016/j.jallcom.2013.12.191
Hayashi, A., Noi, K., Sakuda, A., and Tatsumisago, M. (2012). Superionic glass-ceramic electrolytes for room-temperature rechargeable sodium batteries. Nat. Commun. 3, 856–860. doi:10.1038/ncomms1843
He, K., Zu, C., Wang, Y., Han, B., Yin, X., Zhao, H., et al. (2014). Stability of lithium ion conductor NASICON structure glass ceramic in acid and alkaline aqueous solution. Solid State Ionics 254, 78–81. doi:10.1016/j.ssi.2013.11.011
Hong, H. Y. P. (1976). Crystal structures and crystal chemistry in the system Na1+xZr2SixP3-xO12. Mater. Res. Bull. 11, 173–182. doi:10.1016/0025-5408(76)90073-8
Hong, H. Y.-P. (1978). Crystal structure and ionic conductivity of Li14Zn(GeO4)4 and other new Li+ superionic conductors. Mater. Res. Bull. 13, 117–124. doi:10.1016/0025-5408(78)90075-2
Inaguma, Y., and Nakashima, M. (2013). A rechargeable lithium-air battery using a lithium ion-conducting lanthanum lithium titanate ceramics as an electrolyte separator. J. Power Sources 228, 250–255. doi:10.1016/j.jpowsour.2012.11.098
Ishiguro, K., Nakata, Y., Matsui, M., Uechi, I., Takeda, Y., Yamamoto, O., et al. (2013). Stability of Nb-doped cubic Li7La3Zr2O12 with lithium metal. J. Electrochem. Soc. 160, A1690–A1693. doi:10.1039/c2cp40634a
Ito, Y., Sakuda, A., Ohtomo, T., Hayashi, A., and Tatsumisago, M. (2013). Preparation of Li2S-GeS2 solid electrolyte thin films using pulsed laser deposition. Solid State Ionics 236, 1–4. doi:10.1016/j.ssi.2013.01.014
Jin, Y., and McGinn, P. J. (2013a). Bulk solid state rechargeable lithium ion battery fabrication with Al-doped Li7La3Zr2O12 electrolyte and Cu0.1V2O5 cathode. Electrochim. Acta 89, 407–412. doi:10.1016/j.electacta.2012.11.059
Jin, Y., and McGinn, P. J. (2013b). Li7La3Zr2O12 electrolyte stability in air and fabrication of a Li/Li7La3Zr2O12/Cu0.1V2O5 solid-state battery. J. Power Sources 239, 326–331. doi:10.1016/j.jpowsour.2013.03.155
Kamaya, N., Homma, K., Yamakawa, Y., Hirayama, M., Kanno, R., Yonemura, M., et al. (2011). A lithium superionic conductor. Nat. Mater. 10, 682–686. doi:10.1038/nmat3066
Kanno, R., Hata, T., Kawamoto, Y., and Irie, M. (2000). Synthesis of a new lithium ionic conductor, thio-LISICON-lithium germanium sulfide system. Solid State Ionics 130, 97–104. doi:10.1016/S0167-2738(00)00277-0
Kanno, R., and Murayama, M. (2001). Lithium ionic conductor thio-LISICON: the Li2S-GeS2-P2S5 system. J. Electrochem. Soc. 148, A742–A746. doi:10.1149/1.1379028
Key, B., Schroeder, D. J., Ingram, B. J., and Vaughey, J. T. (2012). Solution-based synthesis and characterization of lithium-ion conducting phosphate ceramics for lithium metal batteries. Chem. Mater. 24, 287–293. doi:10.1021/cm202773d
Kitaura, H., Hayashi, A., Ohtomo, T., Hama, S., and Tatsumisago, M. (2011). Fabrication of electrode-electrolyte interfaces in all-solid-state rechargeable lithium batteries by using a supercooled liquid state of the glassy electrolytes. J. Mater. Chem. 21, 118. doi:10.1039/c0jm01090a
Knauth, P. (2009). Inorganic solid Li ion conductors: an overview. Solid State Ionics 180, 911–916. doi:10.1016/j.ssi.2009.03.022
Kokal, I., Somer, M., Notten, P. H. L., and Hintzen, H. T. (2011). Sol-gel synthesis and lithium ion conductivity of Li7La3Zr2O12 with garnet-related type structure. Solid State Ionics 185, 42–46. doi:10.1016/j.ssi.2011.01.002
Kotobuki, M. (2012). The current situation and problems of rechargeable lithium ion batteries. Open Electrochem. J. 4, 28–35. doi:10.2174/1876505X01204010028
Kotobuki, M., and Koishi, M. (2013). Preparation of Li1.5 Al1.5(PO4)3 solid electrolyte via a sol-gel route using various Al sources. Ceram. Int. 39, 4645–4649. doi:10.1016/j.ceramint.2012.10.206
Lee, C. H., Joo, K. H., Kim, J. H., Woo, S. G., Sohn, H. J., Kang, T., et al. (2002). Characterizations of a new lithium ion conducting Li2O-SeO2-B2O3 glass electrolyte. Solid State Ionics 149, 59–65. doi:10.1016/S0167-2738(02)00137-6
Machida, N., and Shigematsu, T. (2004). An all-solid-state lithium battery with sulfur as positive electrode materials. Chem. Lett. 33, 376–377. doi:10.1246/cl.2004.376
Mei, A., Wang, X. L., Lan, J. L., Feng, Y. C., Geng, H. X., Lin, Y. H., et al. (2010). Role of amorphous boundary layer in enhancing ionic conductivity of lithium-lanthanum-titanate electrolyte. Electrochim. Acta 55, 2958–2963. doi:10.1016/j.electacta.2010.01.036
Mercier, R., Malugani, J. P., Fahys, B., and Robert, G. (1981). Superionic conduction in Li2S-P2S5-LiI-glasses. Solid State Ionics 5, 663–666. doi:10.1016/0167-2738(81)90341-6
Minami, T. (1987). Recent progress in superionic conducting glasses. J. Non Cryst. Solids 95–96(Part 1), 107–118. doi:10.1016/S0022-3093(87)80103-5
Mizuno, F., Hayashi, A., Tadanaga, K., and Tatsumisago, M. (2005). New, highly ion-conductive crystals precipitated from Li2S-P2S5 glasses. Adv. Mater. 17, 918–921. doi:10.1002/adma.200401286
Morata-Orrantia, A., García-Martí, G., and Alario-Franco, M. (2003). Optimization of lithium conductivity in La/Li titanates. Chem. Mater. 15, 3991–3995. doi:10.1021/cm0300563
Morimoto, H., Awano, H., Terashima, J., Shindo, Y., Nakanishi, S., Ito, N., et al. (2013). Preparation of lithium ion conducting solid electrolyte of NASICON-type Li1+xAlxTi2-x(PO4)3 (x 0.3) obtained by using the mechanochemical method and its application as surface modification materials of LiCoO2 cathode for lithium cell. J. Power Sources 240, 636–643. doi:10.1016/j.jpowsour.2013.05.039
Morimoto, H., Yamashita, H., Tatsumisago, M., and Minami, T. (1999). Mechanochemical synthesis of new amorphous materials of 60Li2S·40SiS2 with high lithium ion conductivity. J. Am. Ceram. Soc. 82, 1352–1354. doi:10.1111/j.1151-2916.1999.tb01923.x
Murugan, R., Thangadurai, V., and Weppner, W. (2007). Fast lithium ion conduction in garnet-type Li7La3Zr2O12. Angew. Chem. Int. Ed. 46, 7778–7781. doi:10.1002/anie.200701144
Nagao, M., Hayashi, A., and Tatsumisago, M. (2012). High-capacity Li2S-nanocarbon composite electrode for all-solid-state rechargeable lithium batteries. J. Mater. Chem. 22, 10015–10020. doi:10.1039/c2jm16802b
Narayanan, S., Ramezanipour, F., and Thangadurai, V. (2012). Enhancing Li ion conductivity of garnet-type Li5La3Nb2O12 by Y- and Li-codoping: synthesis, structure, chemical stability, and transport properties. J. Phys. Chem. C 116, 20154–20162. doi:10.1021/jp304737x
Nikolić, J. D., Smiljanjić, S. V., Matijašević, S. D., Živanović, V. D., Tošič, M. B., Grujić, S. R., et al. (2013). Preparation of glass-ceramic in Li2O-Al2O3-GeO2-P2O5 system. Process. Appl. Ceram. 7, 147–151. doi:10.2298/PAC1304147N
Ohta, N., Takada, K., Sakaguchi, I., Zhang, L., Ma, R., Fukuda, K., et al. (2007). LiNbO3-coated LiCoO2 as cathode material for all solid-state lithium secondary batteries. Electrochem. Commun. 9, 1486–1490. doi:10.1016/j.elecom.2007.02.008
Ohta, N., Takada, K., Zhang, L., Ma, R., Osada, M., and Sasaki, T. (2006). Enhancement of the high-rate capability of solid-state lithium batteries by nanoscale interfacial modification. Adv. Mater. 18, 2226–2229. doi:10.1002/adma.200502604
Ohta, S., Kobayashi, T., Seki, J., and Asaoka, T. (2012). Electrochemical performance of an all-solid-state lithium ion battery with garnet-type oxide electrolyte. J. Power Sources 202, 332–335. doi:10.1016/j.jpowsour.2011.10.064
Ohtomo, T., Hayashi, A., Tatsumisago, M., and Kawamoto, K. (2013a). All-solid-state batteries with Li2O-Li2S-P2S5 glass electrolytes synthesized by two-step mechanical milling. J. Solid State Electrochem. 17, 2551–2557. doi:10.1007/s10008-013-2149-5
Ohtomo, T., Hayashi, A., Tatsumisago, M., and Kawamoto, K. (2013b). Characteristics of the Li2O-Li2S-P2S5 glasses synthesized by the two-step mechanical milling. J. Non Cryst. Solids 364, 57–61. doi:10.1016/j.jnoncrysol.2012.12.044
Ohtomo, T., Hayashi, A., Tatsumisago, M., Tsuchida, Y., Hama, S., and Kawamoto, K. (2013c). All-solid-state lithium secondary batteries using the 75Li2S·25P2S5 glass and the 70Li2S·30P2S5 glass-ceramic as solid electrolytes. J. Power Sources 233, 231–235. doi:10.1016/j.jpowsour.2013.01.090
Patil, V., Patil, A., Yoon, S. J., and Choi, J. W. (2013). Structural and electrical properties of NASICON type solid electrolyte nanoscaled glass-ceramic powder by mechanical milling for thin film batteries. J. Nanosci. Nanotech. 13, 3665–3668. doi:10.1166/jnn.2013.7240
Qian, D., Xu, B., Cho, H. M., Hatsukade, T., Carroll, K. J., and Meng, Y. S. (2012). Lithium lanthanum titanium oxides: a fast ionic conductive coating for lithium-ion battery cathodes. Chem. Mater. 24, 2744–2751. doi:10.1021/cm300929r
Quartarone, E., and Mustarelli, P. (2011). Electrolytes for solid-state lithium rechargeable batteries: recent advances and perspectives. Chem. Soc. Rev. 40, 2525–2540. doi:10.1039/c0cs00081g
Raguenet, B., Tricot, G., Silly, G., Ribes, M., and Pradel, A. (2012). The mixed glass former effect in twin-roller quenched lithium borophosphate glasses. Solid State Ionics 208, 25–30. doi:10.1016/j.ssi.2011.11.034
Ravaine, D. (1980). Glasses as solid electrolytes. J. Non Cryst. Solids 38–39(Part 1), 353–358. doi:10.1016/0022-3093(80)90444-5
Robertson, A. D., West, A. R., and Ritchie, A. G. (1997). Review of crystalline lithium-ion conductors suitable for high temperature battery applications. Solid State Ionics 104, 1–11. doi:10.1016/S0167-2738(97)00429-3
Sahu, G., Lin, Z., Li, J. C., Liu, Z. C., Dudney, N., and Liang, C. D. (2014). Air-stable, high-conduction solid electrolytes of arsenic-substituted Li4SnS4. Energy Environ. Sci. 7, 1053–1058. doi:10.1039/c3ee43357a
Sakuda, A., Hayashi, A., Ohtomo, T., Hama, S., and Tatsumisago, M. (2011). All-solid-state lithium secondary batteries using LiCoO2 particles with pulsed laser deposition coatings of Li2S-P2S5 solid electrolytes. J. Power Sources 196, 6735–6741. doi:10.1021/am302164e
Seino, Y., Ota, T., Takada, K., Hayashi, A., and Tatsumisago, M. (2014). A sulphide lithium super ion conductor is superior to liquid ion conductors for use in rechargeable batteries. Energy Environ. Sci. 7, 627–631. doi:10.1039/c3ee41655k
Shimonishi, Y., Zhang, T., Imanishi, N., Im, D., Lee, D. J., Hirano, A., et al. (2011). A study on lithium/air secondary batteries – stability of the NASICON-type lithium ion conducting solid electrolyte in alkaline aqueous solutions. J. Power Sources 196, 5128–5132. doi:10.1016/j.jpowsour.2011.02.023
Stramare, S., Thangadurai, V., and Weppner, W. (2003). Lithium lanthanum titanates: a review. Chem. Mater. 15, 3974–3990. doi:10.1088/0953-8984/22/40/404203
Takada, K. (2009). Electrolytes: solid oxide. Enc. Electrochem. Power Sources 5, 328–336. doi:10.1016/B978-044452745-5.00211-2
Tan, G. Q., Wu, F., Li, L., Liu, Y. D., and Chen, R. J. (2012). Magnetron sputtering preparation of nitrogen-incorporated lithium-aluminum-titanium phosphate based thin film electrolytes for all-solid-state lithium ion batteries. J. Phys. Chem. C 116, 3817–3826. doi:10.1021/jp207120s
Tatsumisago, M. (2004). Glassy materials based on Li2S for all-solid-state lithium secondary batteries. Solid State Ionics 175, 13–18. doi:10.1016/j.ssi.2004.09.012
Tatsumisago, M., Hachida, N., and Minami, T. (1987). Mixed anion effect in conductivity of rapidly quenched Li4SiO4-Li3BO3 glasses. Yogyo Kyokaishi. 95, 197–201. doi:10.2109/jcersj1950.95.1098_197
Tatsumisago, M., Hama, S., Hayashi, A., Morimoto, H., and Minami, T. (2002). New lithium ion conducting glass-ceramics prepared from mechanochemical Li2S-P2S5 glasses. Solid State Ionics 15, 635–640. doi:10.1016/S0167-2738(02)00509-X
Tatsumisago, M., and Hayashi, A. (2009). “Secondary batteries-lithium rechargeable systems – electrolytes: Glass,” in Encyclopedia of Electrochemical Power Source, ed. J. Garche, et al. (Amsterdam: Elsevier B.V.), 138–144.
Tatsumisago, M., Minami, T., and Tanaka, M. (1981). Rapid thermal-image furnace for glass preparation. J. Am. Ceram. Soc. 64, C–97–C–98. doi:10.1111/j.1151-2916.1981.tb09886.x
Tatsumisago, M., Nagao, M., and Hayashi, A. (2013). Recent development of sulfide solid electrolytes and interfacial modification for all-solid-state rechargeable lithium batteries. J. Asian Ceram. Soc. 1, 17–25. doi:10.1016/j.jascer.2013.03.005
Teranishi, T., Yamamoto, M., Hayashi, H., and Kishimoto, A. (2013). Lithium ion conductivity of Nd-doped (Li, La)TiO3 ceramics. Solid State Ionics 243, 18–21. doi:10.1016/j.ssi.2013.04.014
Thangadurai, V., and Weppner, W. (2005a). Li6ALa2Nb2O12 (A=Ca, Sr, Ba): a new class of fast lithium ion conductors with garnet-like structure. J. Am. Ceram. Soc. 88, 411–418. doi:10.1111/j.1551-2916.2005.00060.x
Thangadurai, V., and Weppner, W. (2005b). Li6ALa2Ta2O12(A=Sr, Ba):novel garnet-like oxides for fast lithium ion conduction. Adv. Funct. Mater. 15, 107–112. doi:10.1039/c4cs00020j
Thangadurai, V., and Weppner, W. (2006a). Effect of sintering on the ionic conductivity of garnet-related structure Li5La3Nb2O12 and In- and K-doped Li5La3Nb2O12. J. Solid State Chem. 179, 974–984. doi:10.1016/j.jssc.2005.12.025
Thangadurai, V., and Weppner, W. (2006b). Recent progress in solid oxide and lithium ion conducting electrolytes research. Ionics 12, 81–92. doi:10.1007/s11581-006-0013-7
Tietz, F., Wegener, T., Gerhards, M. T., Giarola, M., and Mariotto, G. (2013). Synthesis and Raman micro-spectroscopy investigation of Li7La3Zr2O12. Solid State Ionics 230, 77–82. doi:10.1016/j.ssi.2012.10.021
Ujiie, S., Hayashi, A., and Tatsumisago, M. (2012). Structure, ionic conductivity and electrochemical stability of Li2S-P2S5-LiI glass and glass-ceramic electrolytes. Solid State Ionics 211, 42–45. doi:10.1016/j.ssi.2012.01.017
Vidal, K., Ortega-San-Martín, L., Larrañaga, A., Merino, R. I., Orera, A., and Arriortua, M. I. (2014). Effects of synthesis conditions on the structural, stability and ion conducting properties of Li0.30(La0.50Ln0.50)0.567TiO3 (Ln=La, Pr, Nd) solid electrolytes for rechargeable lithium batteries. Ceram. Int. 40, 8761–8768. doi:10.1016/j.ceramint.2014.01.097
Xu, X. X., Wen, Z. Y., Wu, X. W., Yang, X. L., and Gu, Z. H. (2007). Lithium ion-conducting glass-ceramics of Li1.5Al0.5Ge1.5(PO4)3-xLi2O (x=0.0-0.20) with good electrical and electrochemical properties. J. Am. Ceram. Soc. 90, 2802–2806. doi:10.1111/j.1551-2916.2007.01827.x
Yada, C., Iriyama, Y., Abe, T., Kikuchi, K., and Ogumi, Z. (2009). A novel all-solid-state thin-film-type lithium-ion battery with in situ prepared positive and negative electrode materials. Electrochem. Commun. 11, 413–416. doi:10.1016/j.elecom.2008.12.004
Yamauchi, A., Sakuda, A., Hayashi, A., and Tatsumisago, M. (2013). Preparation and ionic conductivities of (100-x)(0.75Li2S·0.25P2S5)·xLiBH4 glass electrolytes. J. Power Sources 244, 707–710. doi:10.1016/j.jpowsour.2012.12.001
Keywords: all-solid-state, lithium batteries, inorganic electrolytes, ion conductivity, interface
Citation: Cao C, Li Z-B, Wang X-L, Zhao X-B and Han W-Q (2014) Recent advances in inorganic solid electrolytes for lithium batteries. Front. Energy Res. 2:25. doi: 10.3389/fenrg.2014.00025
Received: 16 April 2014; Accepted: 11 June 2014;
Published online: 27 June 2014.
Edited by:
Mariusz Walkowiak, Institute of Non-Ferrous Metals, PolandReviewed by:
Lei Li, Shanghai Jiao Tong University, ChinaJie Shu, Ningbo University, China
Xiao-Guang Sun, Oak Ridge National Laboratory, USA
Copyright: © 2014 Cao, Li, Wang, Zhao and Han. This is an open-access article distributed under the terms of the Creative Commons Attribution License (CC BY). The use, distribution or reproduction in other forums is permitted, provided the original author(s) or licensor are credited and that the original publication in this journal is cited, in accordance with accepted academic practice. No use, distribution or reproduction is permitted which does not comply with these terms.
*Correspondence: Xin-Bing Zhao, Department of Materials Science and Engineering, Zhejiang University, 38#, Zheda Road, Hangzhou, Zhejiang Province, China e-mail:emhhb3hiQHpqdS5lZHUuY24=;
Wei-Qiang Han, 1219#, Zhongguan Road, Ningbo, Zhejiang Province, China e-mail:aGFud2VpcWlhbmdAbmltdGUuYWMuY24=
†Present address: Xiao-Liang Wang, Seeo, Inc., 3906 Trust Way, Hayward, CA 94545, USA