- Department of Gastroenterology, The First Hospital of Jilin University, Changchun, China
In contemporary microbial research, the exploration of interactions between microorganisms and multicellular hosts constitutes a burgeoning field. The gut microbiota is increasingly acknowledged as a pivotal contributor to various disorders within the endocrine system, encompassing conditions such as diabetes and thyroid diseases. A surge in research activities has been witnessed in recent years, elucidating the intricate interplay between the gut microbiota and disorders of the endocrine system. Simultaneously, fecal microbiota transplantation (FMT) has emerged as a focal point, garnering substantial attention in both biomedical and clinical spheres. Research endeavors have uncovered the remarkable therapeutic efficacy of FMT across diverse diseases, with particular emphasis on its application in addressing type 2 diabetes mellitus (T2DM) and associated com-plications. Consequently, this manuscript accentuates the intimate connection between the gut microbiota and disorders within the endocrine system, with a specific focus on exploring the potential of FMT as an intervention in the therapeutic landscape of T2DM and its complications. Furthermore, the article scrutinizes concerns inherent in treatment modalities centered around the gut microbiota, proposing viable solutions to address these issues.
1 Introduction
Type 2 diabetes mellitus (T2DM) is a prevalent metabolic chronic disease characterized by insulin resistance and abnormally elevated blood glucose levels (1). Currently, the global number of adults with T2DM exceeds 400 million, and this figure continues to rise (2). This has led to a substantial burden of mortality and disability worldwide, contributing to a sustained increase in healthcare expenditures across nations (3). Notably, diabetic patients with complications incur significantly higher healthcare costs and rates of mortality and disability compared to those without complications (4). Diabetes has numerous associated complications, and all current medications targeting these complications come with certain side effects. The introduction of new treatment strategies is highly necessary and imminent.
Scientific investigations have revealed that the structural resemblance between human cells and bacteria is noteworthy (5). Specifically, the gut microbiota, constituting the most intricate and diverse microbial community within the human body, is commonly denoted as the “second genome” of humans within the bacterial system. Despite undergoing alterations in composition and structure over time, the human gut microbiota maintains a certain level of dynamic stability (5, 6). Mounting evidence underscores the pivotal role of the gut microbiota in host metabolism, immunity, and even behavior, achieved through the breakdown of food or host components and the subsequent generation of novel compounds or metabolites (7, 8). This reciprocal influence between the host and the microbial community establishes a bidirectional interplay characterized by mutual interactions between bacteria and the host, collectively shaping host performance (9). Fecal microbiota transplantation (FMT), a recent focal point of research, has exhibited therapeutic promise in addressing a spectrum of conditions, including metabolic syndrome, autoimmune disorders, and neurologic diseases (10, 11).
2 Association between gut microbiota and T2DM
Type 2 diabetes mellitus (T2DM) is characterized by both tissue-specific insulin resistance and dysfunction of pancreatic β-cells. The intricate local mechanisms contributing to T2DM involve metabolic signaling, alterations in mitochondrial metabolism, oxidative stress, endoplasmic reticulum stress, and localized inflammation (12, 13). Furthermore, the gut microbiota plays a pivotal role in the manifestation of T2DM.
2.1 Mechanisms of altered gut microbiota in T2DM
Microbial communities constitute the largest “ecosystem” within the human body, evolving concomitantly with human development (14). In healthy adults, the gut microbiota comprises approximately ten times more microbial cells than human cells, with genomes that are a hundred times more abundant (15). The microbiota is fundamentally involved in the development of the immune system, defense against pathogenic microorganisms, digestion of exogenous substances, and the regulation of metabolism (16).
From the esophagus to the rectum, variations in bacterial diversity, abundance, and quantity occur due to genetic predisposition, dietary factors, antibiotic use, and challenging Clostridioides difficile infections, resulting in alterations to the microbial community and dysbiosis (17, 18). Changes in the gut microbiota are associated with host oxidative stress and inflammatory responses. Elevated blood glucose levels can lead to intestinal damage characterized by tight junction protein degradation, compromising barrier integrity (19). Hyperglycemia damages tight and adherens junctions, leading to barrier disruption. This disruption contributes to dysregulation of the gut microbiota, weakening the intestinal clearance of toxic metabolites and increasing the abundance of pathogenic bacteria (20). Intestinal bacteria, entering the bloodstream through the compromised intestinal barrier, stimulate the immune system (21).
Intestinal barrier, comprising immune, biological, mechanical, and mucous barriers, segregates the host from microorganisms in the intestinal lumen, limiting their movement (22). An intact mucosal barrier prevents the migration of microorganisms and their products into the bloodstream (23). Compromised barriers result in heightened intestinal permeability, facilitating the entry of bacteria and their byproducts into the bloodstream. This process activates the mononuclear phagocyte system, triggering the release of inflammatory factors like IL-6 and TNF-α, culminating in chronic low-grade inflammation (24). Furthermore, lipopolysaccharide (LPS) can traverse the intestinal epithelium through compromised tight junctions or chylomicrons (25). In diabetic patients, increased intestinal permeability may be associated with inflammatory responses, where LPS, an influential endotoxin from Gram-negative bacterial cell walls, initiates inflammatory mechanisms by binding to CD14 and Toll-like receptor 4 (TLR4) on macrophage surfaces (26). Molecular proteins such as c-Jun N-terminal kinase (JNK) and p38 mitogen-activated protein kinase (MAPK) regulate the impact of inflammation on insulin signaling (27). Consequently, compromised intestinal barrier integrity and immune imbalance contribute to the development of T2DM (Figure 1).
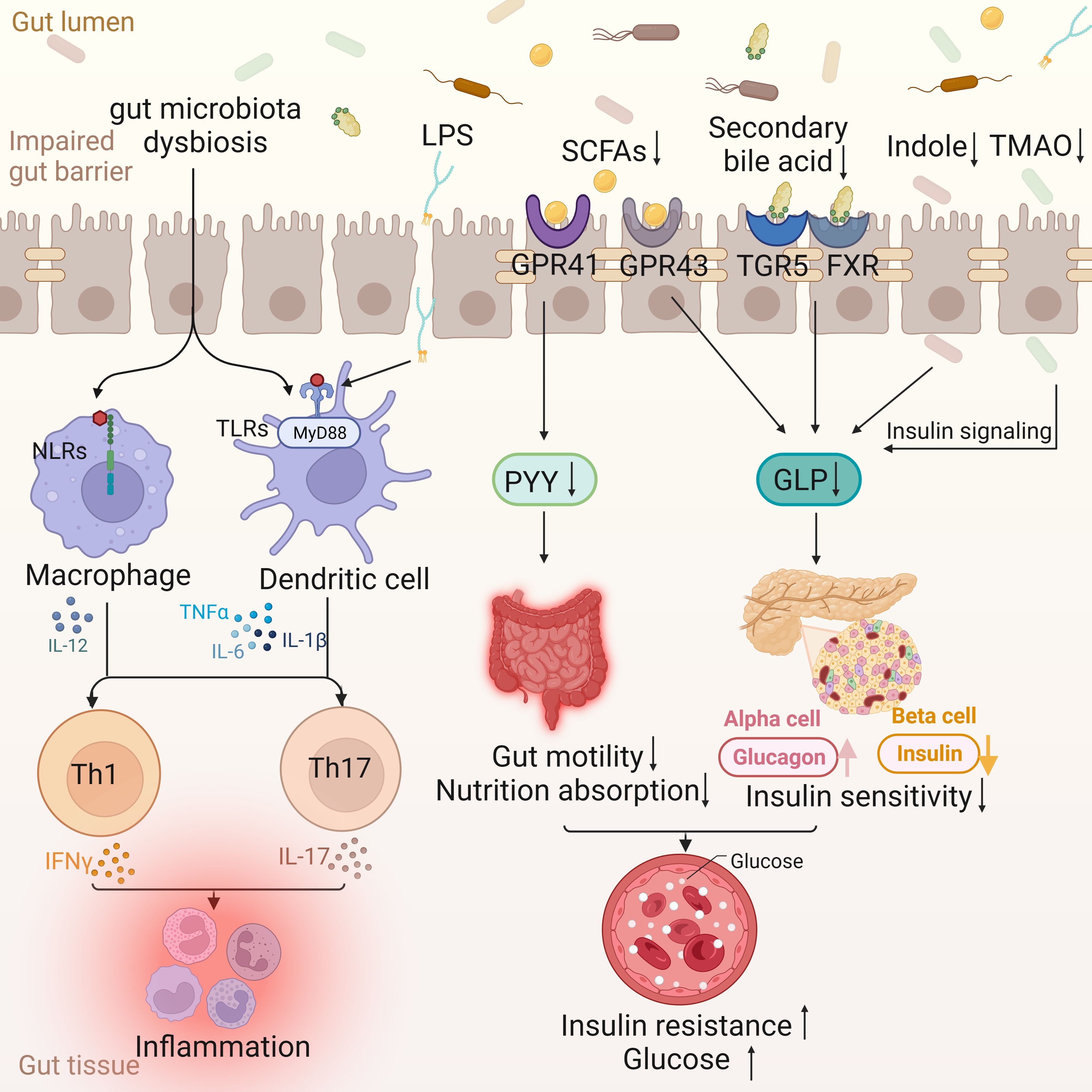
Figure 1. Intestinal changes in T2DM. The disordered gut microflora enters the gut tissue through the impaired gut barrier, activating the nucleotide oligomerization domain (NOD)-like receptors (NLRs) signaling pathway of macrophages and the MyD88-dependent TLRs signaling pathway of dendritic cells, and jointly activating Th 1 and Th 17, leading to the occurrence of inflammation. Lipopolysaccharides (LPS) is also involved in inflammation through Toll-like receptors (TLRs). The decrease of Short-chain fatty acids (SCFAs) leads to the decrease of peptide YY (PYY) through G protein-coupled receptor (GPCR) 41, which in turn leads to the decrease of gut motility and nutrient absorption function. At the same time, the decrease of SCFAs, secondary bile acids, indole and Trimethylamine N-Oxide (TMAO) can lead to the decrease of glucagon-like peptide (GLP) and insulin sensitivity through different ways, and then lead to insulin resistance and blood sugar increase.
2.2 Gut microbiota inT2DM
2.2.1 Alterations and effects of gut microbiota in T2DM
The healthy gut microbiota predominantly consists of anaerobic bacteria, categorized into six phyla: Firmicutes, Bacteroidetes, Proteobacteria, Actinobacteria, Fusobacteria, and Verrucomicrobia (28).
Modifications in the gut microbiota influence the metabolism of the primary intracellular antioxidant, glutathione (GSH), within the host organism (29). Interactions between intestinal epithelial cells and specific symbiotic bacteria lead to the rapid generation of reactive oxygen species (ROS) in host cells, impacting the activation of oxidative stress markers like Nox2 and redox signaling transduction (30). Intestinal inflammation elevates gut permeability, setting off a cascade of events, including infections and systemic inflammation (31, 32).
A distinctive feature of gut microbiota imbalance in T2DM is the disproportionate ratio between Bacteroidetes and Firmicutes, coupled with a significant reduction in carbohydrate membrane transport and butyric acid biosynthetic function (33, 34) (Figure 2). In comparison to their healthy counterparts, T2DM patients exhibit an increase in the abundance of Lactobacillus species and a decrease in Clostridium species in the intestinal tract (28). These bacterial shifts play a role in glucose homeostasis by outcompeting pathogens and reinstating intestinal equilibrium (35). Lactobacillus has been shown to lower blood glucose and body weight while mitigating insulin resistance in high-fat diet-induced T2DM mice (36). Lactobacillus species, particularly Lactobacillus casei, Lactobacillus reuteri, Bifidobacterium bifidum, and Streptococcus thermophiles, can enhance host gut microbial structure and reduce intestinal permeability when consumed in sufficient quantities (37). Another group of bacteria potentially sensitive to insulin is Akkermansia muciniphila, which, when safely ingested, can improve metabolic abnormalities in obese individuals (38). Research indicates that individuals with type 2 diabetes mellitus (T2DM) who are prescribed metformin demonstrate a heightened relative abundance of Akkermansia muciniphila and other microbial groups that produce short-chain fatty acids (SCFAs), in contrast to non-diabetic counterparts (39). This observed phenomenon aligns with findings in human studies, where the increased presence of Akkermansia muciniphila has been associated with the enhanced anti-diabetic effects of metformin in diet-induced obese mice (40). Conversely, T2DM patients exhibit significantly reduced levels of Roseburia intestinalis and Faecalibacterium prausnitzii, both recognized producers of butyrate (41). A randomized double-blind clinical trial has indicated that the incorporation of a freshwater fish-based diet can ameliorate liver fat deposition and other metabolic phenotypes, potentially through the augmentation of fecal Roseburia intestinalis (42). Additionally, a negative correlation has been identified between Bacteroides and T2DM, with diminished levels of specific strains such as Bacteroides 20-3, Bacteroides vulgatus, and Bacteroides intestinalis in individuals with T2D (43). During hyperglycemia, Enterococci increase, while sulfur-producing bacteria such as Bifidobacterium vulgatus decrease, exacerbating diabetes (44). Reduced Bifidobacterium levels lead to elevated plasma endotoxin levels and increased pro-inflammatory cytokines (45). In a study involving 36 adult subjects, healthy individuals had significantly higher levels of Bifidobacterium, whereas T2DM patients had higher levels of Lactobacillus, supporting this conclusion (46). Observational data reveals an elevation in the levels of potentially pathogenic bacteria, notably Escherichia coli and Desulfovibrio, in individuals diagnosed with diabetes (47). Fusobacterium, Ruminococcus, and Blautia consistently emerge as bacterial taxa exhibiting a positive correlation with type 2 diabetes mellitus (T2DM) (48). Furthermore, an augmented abundance of Rikenellaceae has been linked to heightened stress levels and inflammatory responses (49). Muribaculaceae, identified as a beneficial bacterium, plays a pivotal role in positively influencing intestinal energy metabolism while concurrently regulating host blood glucose and lipid levels (50). Clinical studies further reveal that increased levels of Phascolarctobacterium and Bacteroides stercoris are associated with improved insulin sensitivity, linking this metabolic benefit to the success of intestinal endocrine function, gut microbiota, and donor microbial engraftment (51). Moreover, Bacteroides fragilis, a fragile mimic bacterium, is known to generate polysaccharide A, which actively promotes the synthesis of pro-inflammatory cytokines, such as IL-12, thereby fostering Th1 activation (52). The genetic information inherent in these microorganisms correlates with oxidative stress, establishing a direct linkage between alterations in the intestinal microbiota composition and the inflammatory status observed in individuals with T2DM (53).
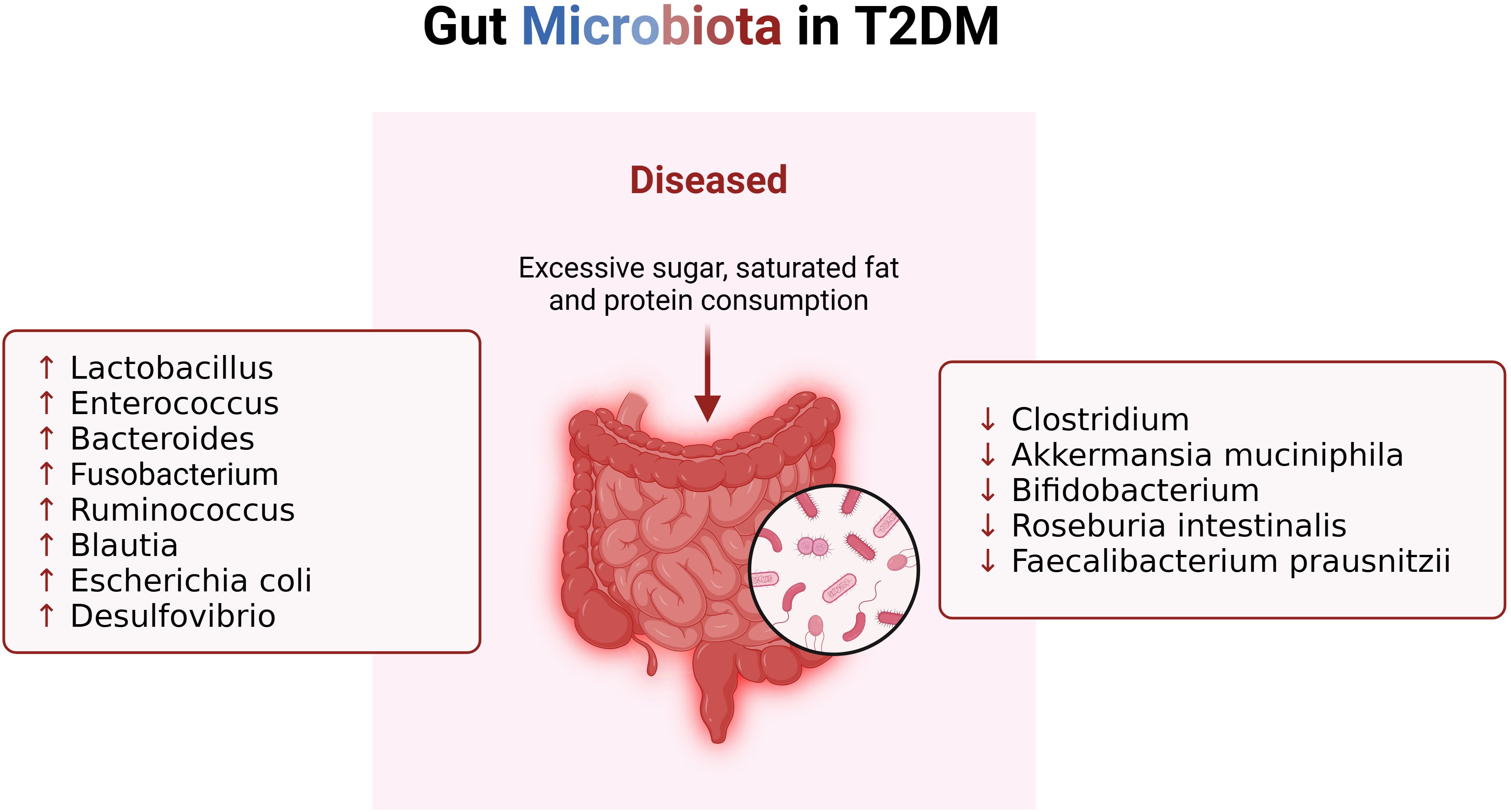
Figure 2. Changes of gut microbiota in patients with T2DM. When T2DM patients consume excessive sugar, saturated fat and protein, their gut microbiota changes, resulting in imbalance of immune system.
Additionally, the use of antibiotics significantly alters the gut microbiota, which may affect diabetes control. Hodoşan et al. (54) reported high rates of antibiotic prescriptions, raising concerns about antibiotic resistance and metabolic effects. Păduraru et al. (55)emphasized the clinical consequences of antibiotic use. It is also essential to explore how these factors influence fecal microbiota transplantation and diabetes management.
2.2.2 Gut microbiota influences immunity in T2DM patients
Dysbiosis has been observed to precipitate an immune system imbalance. The gut microbiota and their metabolic byproducts play a pivotal role in sustaining equilibrium within the T-helper 17/regulatory T cells (Th17/Treg) and gut-associated lymphoid tissue (GALT) systems (56). This intricate regulation extends to the promotion of innate lymphoid cells, natural killer cells, cytotoxic and non-cytotoxic cells, as well as helper lymphocytes, with metabolites like tryptophan being involved in this process (57). Innate lymphoid cells (ILCs) represent crucial components of innate immunity, contributing by producing both regulatory and pro-inflammatory cytokines to support tissue repair, immunity, and inflammation. Notably, research has uncovered elevated levels of ILCs1 in individuals diagnosed with T2DM, which correlates with an increased susceptibility to diabetes. Conversely, an increase in ILC2s contributes to improving glucose homeostasis and preventing insulin resistance (58). Additionally, IL-12, as an immune regulatory factor, plays a crucial role in T2DM and its complications. Its signaling, coupled with receptor binding on pancreatic beta cells, triggers pro-inflammatory cytokines and induces cell apoptosis (59). TLR4 activation inhibits insulin function by triggering signal cascades and transcription factors (such as MyD88, TIRAP, TRIF, IKK, and JNK) involved in innate immune responses. TLR4 is widely expressed in cells and promotes inflammation by recognizing damage-associated molecular pattern proteins (DAMPs) (60, 61), ultimately leading to the development of insulin resistance. Another investigation reported that MyD88, a Toll-like receptor (TLR) adapter, possesses the capacity to modulate glucose and lipid metabolism by inducing alterations in the gut microbiota composition (62).
2.2.3 Impact of gut microbiota metabolites
Microbial metabolites, including short-chain fatty acids (SCFAs), secondary bile acids, trimethylamine N-oxide (TMAO), and indole-3-propionic acid (IPA), play a crucial role in either ameliorating or exacerbating T2DM through signaling mediated by their respective receptors (63).
In the human intestinal tract, SCFAs, produced through carbohydrate fermentation, act as substrates for lipid and glucose production (63). Common SCFA-producing bacteria, such as Ruminococcaceae (cluster IV) and Eubacterium (Cluster XIVa) within the Clostridia order, play a pivotal role in this process (64). SCFA receptors, members of the G-protein coupled receptor (GPCR) family, like GPR41 and GPR43, execute crucial physiological functions upon activation by SCFAs, including regulation of fat and glucose metabolism and the production of peptide YY (PYY) (65). The latter, in turn, regulates intestinal motility and nutrient absorption, modulating adipose-insulin signal transduction, leading to reduced energy expenditure and insulin secretion (66, 67). Intestinal SCFAs contribute to reducing inflammation in adipocytes by stimulating the production of glucagon-like peptide 1 (GLP-1), thereby suppressing nuclear transcription factor NF-κB activity. A decrease in GLP-1 levels results in an increase in the concentration of inflammatory factors (such as IL-8 and TNF-α) released by neutrophils and macrophages (64, 68).
The gut microbiota metabolizes primary bile acids into secondary bile acids through bile acid brine hydrolysis and 7α-dehydrogenase (69). Activation of bile acid receptors, including G protein-coupled bile acid receptor 5 (TGR5) and farnesol X receptor (FXR), regulates the secretion of incretin hormone GLP-1 (70, 71). Some secondary bile acids also maintain intestinal barrier function, preventing excessive growth of intestinal bacteria and migration into host cells (72).
Gut bacteria contribute to the formation of trimethylamine (TMA) from choline, transported to the liver, where it is converted into trimethylamine N-oxide (TMAO), exhibiting atherogenic properties (56). Choline, a crucial nutrient for lipid metabolism and the production of very low-density lipoproteins (VLDLs) in the liver, plays a significant role in the occurrence and maintenance of T2DM, correlating with substantial risks for other metabolic syndromes (73).
Indole-3-propionic acid (IPA), endogenously derived from tryptophan by the gut microbiota, is absorbed by intestinal epithelial cells and enters the bloodstream (74). IPA improves glucose metabolism and possesses antioxidant and anti-inflammatory effects. Plasma IPA may serve as a potential biomarker for diabetes and play a protective role by maintaining β-cell function (75).
3 FMT and T2DM
3.1 Clinical management of T2DM
Current approaches to treating T2DM primarily involve lifestyle modifications, oral medications, injectable drugs, and insulin therapy (76). Diverse diets, such as the Mediterranean diet, low-carbohydrate/high-protein diet, vegetarianism, and veganism, have proven effective in improving metabolic conditions and managing T2DM. Adopting a healthy lifestyle with a balanced, antioxidant-rich diet and regular exercise can significantly delay and/or prevent disease progression (77). The pharmacological arsenal for T2DM includes metformin, sulfonylureas, DPP-4 inhibitors, GLP-1 receptor agonists, and SGLT-2 inhibitors. Beyond targeted medications like GLP-1 agonists and SGLT-2 inhibitors, clinical attention is focused on substantial blood sugar and weight control. Ongoing efforts involve the development of personalized and combination therapies tailored to different genders, ages, and body types (78).
3.2 FMT for T2DM treatment
The effectiveness of fecal microbiota transplantation (FMT) in treating Clostridioides difficile infection (CDI) has been substantiated, addressing 80-90% of recurrent CDI cases unresponsive to antibiotics. Currently, FMT is considered the standard treatment in guidelines for managing recurrent CDI (79). FMT proves to be a safe therapeutic approach, with mild clinical symptoms being the most common adverse reactions observed in all existing clinical cases, including diarrhea, gastrointestinal spasms, nausea, abdominal distension, flatulence, constipation, and fever (80). Furthermore, FMT effectively reshapes the patient’s gut microbiota, and the sustained effects of these microbial changes have been observed in subsequent clinical follow-ups (81, 82).
Given the critical role of the gut microbiota in the human body, scientists are actively exploring optimal strategies for manipulating the gut microbiota to treat various diseases. The most common methods for such manipulation involve antibiotics or probiotic therapy. While antibiotics are life-saving, they can have adverse effects by eradicating beneficial microbiota and promoting antibiotic resistance in harmful bacteria (83). Scientific literature on the in vivo effects of probiotics suggests that these microorganisms can accumulate genetic mutations, transforming into ineffective or even harmful strains. However, the evolutionary capacity of probiotic microbiota can also serve as a novel therapeutic strategy against various diseases (84). In comparison to treatments involving prebiotics and probiotics, FMT directly introduces fecal bacteria into the colon to restore the microbiota.
3.2.1 Preparation and application of FMT
Fecal Microbiota Transplantation (FMT) stands out as a highly efficient therapeutic intervention for microbiota modulation within a confined clinical setting. The procedure entails the transfer of gut microbiota from a meticulously screened healthy donor to a recipient, employing various delivery methods such as oral capsules, enema, or infusion via a nasoenteric tube. The primary objectives of FMT encompass the establishment of colonization resistance, the production of beneficial metabolites, and the restoration of interactions with the mucosal immune system (85). Throughout the FMT process, its impact extends beyond the mere transfer of microbiota, encompassing the restoration of the mucosal immune system, the presence of potentially virulent fungi in the microbial solution, transgenic metabolic byproducts, and notably, the pivotal role played by short-chain fatty acids (86). The earliest recorded use of fecal suspensions dates back to the 4th century when traditional Chinese medicine practitioner Ge Hong employed a concoction known as “yellow soup” to treat food poisoning and severe diarrhea (87).
In the European Consensus Conference, a collaborative effort involving 28 experts from 10 countries produced practical guidelines on indications for FMT, donor selection, fecal suspension preparation, clinical management, and the essential requirements for implementing FMT centers (88). Emphasizing the evaluation of FMT, particular attention is directed towards the selection of healthy donors. Prospective donors undergo comprehensive medical interviews aimed at excluding potential risk factors, ensuring the absence of genetic disorders, autoimmune diseases, infectious diseases, diabetes, and gastrointestinal issues. Eligible donors must not have utilized hormones, antibiotics, or proton pump inhibitors within the preceding 3 months. Additionally, they should not have received vaccinations or other investigational drugs in the last 6 months. Furthermore, prospective donors should not manifest symptoms of anxiety or depression (89). Regarding laboratory preparation methods, two alternative procedures are available: fresh preparation (90) and frozen preparation (91). The first method requires processing donor feces within 6 hours after defecation to preserve anaerobic bacteria integrity. Approximately 30 to 50 grams of feces are diluted with saline solution (NaCl 0.9%). The frozen method involves freezing the fecal suspension with glycerol at -80 degrees Celsius. Glycerol is essential for maintaining bacterial vitality during freezing. On the day of infusion, the frozen fecal suspension should thaw at 37°C and be diluted with 0.9% sodium chloride to the desired volume. Frozen feces are crucial for establishing a fecal bank and providing feces on demand. After preparation, FMT administration can be carried out through various routes, broadly categorized into upper digestive tract methods (nasogastric tube, nasoduodenal tube, nasojejunal tube, and capsules) and lower digestive tract methods (enema, colonoscopy) (90). Detailed analysis indicates that combining FMT with a diverse microbiota from a healthy donor promptly elevates diversity to normal levels, enhances microbial networks, and enriches the core microbial community (92).
Contaminated microorganisms in donor feces may lead to severe adverse events. Therefore, the preparation of fecal microbiota transplantation (FMT) material must exclude ineligible human, animal, or biological samples. Ongoing screening is required for donor feces, even from known donors, to eliminate infectious pathogens (93). For enhanced traceability, fecal samples from donors should be stored at deep low temperatures for at least two years (94). Despite the low occurrence and mild nature of short-term adverse events associated with FMT (95), long-term safety assessments are essential. Additionally, governmental agencies must prioritize the establishment of appropriate and effective regulations for FMT to ensure the safety of patients and donors, promote related research, and prevent treatment misuse (96).
3.2.2 Application of FMT in patients with T2DM
FMT has emerged as an effective strategy for treating T2DM (Figure 3, Table 1). Through the augmentation of short-chain fatty acid (SCFA) production, especially butyrate, Fecal Microbiota Transplantation (FMT) exhibits the capacity to mitigate intestinal permeability, thereby preserving the integrity of the epithelial barrier. Notably, the transplantation of F. prausnitzii has displayed favorable outcomes in transgenic models, underscoring its potential as a therapeutic approach for inflammation and diabetes through the restoration of the intestinal barrier’s structure and function (97). FMT elicits adaptive immune responses within the intestinal tract by activating the Toll-like receptor (TLR) pathway, leading to an accelerated synthesis of immunoglobulins that safeguard the intestinal mucosa (98). Furthermore, FMT demonstrates the ability to modulate the gut microbiota’s composition, influence the secretion of inflammatory cytokines, and regulate glucose and insulin sensitivity (99). Following FMT, there is an observed increase in the diversity of the gut microbiota, with research indicating its regulatory impact on the gut microbiota in type 2 diabetes mellitus (T2DM). This, in turn, results in enhanced glucose metabolism, improved insulin sensitivity, and a reduction in systemic inflammation.
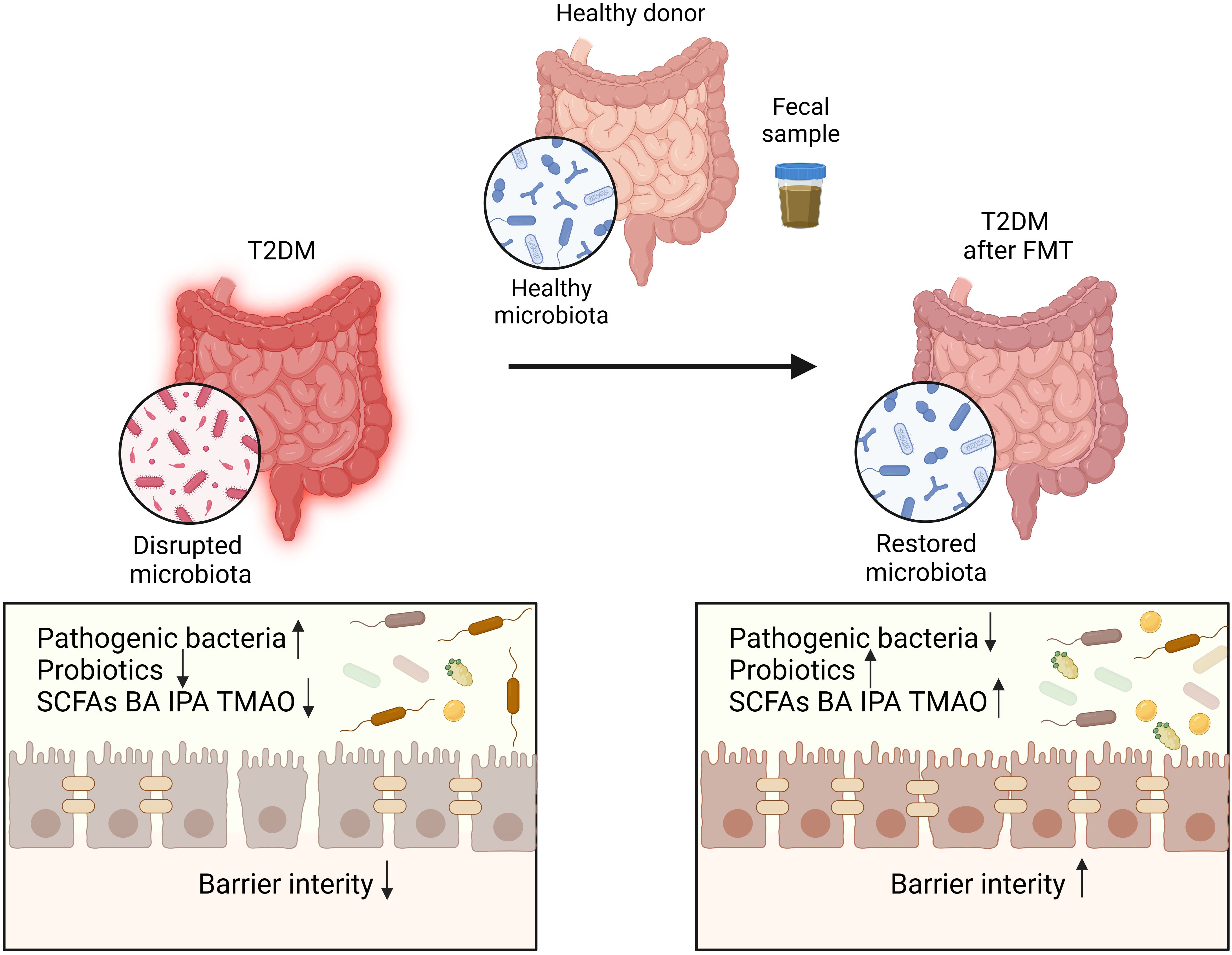
Figure 3. The disrupted microbiota in T2DM patients restored after FMT. Transplantation of healthy microbiota isolated from fecal sample of healthy donor can restore the disrupted microbiota of T2DM patients.
In the context of gene expression in host bacteria, bacteriophages, as key regulators, exert a significant influence, even determining the survival of these bacteria. Prokaryotic viruses, particularly bacteriophages, represent the largest source of genomic material in feces, emphasizing their crucial role (100). Noteworthy observations in cases of recurrent Clostridioides difficile infection suggest that FMT, albeit in a limited number of patients, effectively alleviates diarrhea, pointing to the potential involvement of bacteriophages in preserving host health by regulating the composition and phenotype of the gut microbiota (101). In the study of metabolic syndrome, recipients of healthy donor allogeneic FMT showed a more similar fecal bacteriophage composition to donors compared to non-responders (102). Therefore, the potential of bacteriophage transplantation in relevant indications should not be overlooked.
A. Vrieze et al. (103) conducted a randomized controlled trial to assess the effects of fecal microbiota transplantation (FMT) on male patients with type 2 diabetes mellitus (T2DM). The study revealed that recipients of lean donor feces, facilitated through duodenal infusion (allogeneic transplantation), demonstrated improved insulin sensitivity and increased microbial diversity after six weeks compared to patients undergoing autologous transplantation (self-feces). The most notable changes in microbial composition included an increase in Roseburia intestinalis and Eubacterium hallii, both known producers of butyrate. In a study by Kootte et al. (104), enhanced insulin sensitivity was noted among individuals with obesity and metabolic syndrome who underwent Fecal Microbiota Transplantation (FMT) from lean donors. A study conducted by Allegretti et al. (81) delved into the impacts of FMT administered through oral capsules on obese patients. The findings revealed heightened insulin sensitivity, increased diversity in gut microbiota, and elevated butyrate production post-FMT. This investigative work lays the groundwork for future randomized controlled trials that explore the application of FMT or specific microbial combinations as potential interventions for obesity, metabolic syndrome, diabetes, and other endocrine disorders. In a case study, FMT (administered twice within three months) successfully alleviated blood glucose control, reduced depressive symptoms, and relieved neuropathic pain in a 46-year-old female diabetes patient (105). Additionally, no adverse reactions were observed. In a randomized controlled trial, it was observed that the application of autologous Fecal Microbiota Transplantation (FMT) following diet-induced weight loss preserved favorable metabolic attributes in the study participants (106). Particularly noteworthy was the finding that FMT sourced from obese donors resulted in swift weight gain, highlighting a potential correlation between gut microbiota, obesity, and insulin resistance (107). In fact, repetitive FMT in obese patients with T2DM increased the level and duration of microbial engraftment. Combining lifestyle interventions with FMT induced more favorable changes in recipients’ microbiota, improving lipid profiles and liver stiffness (108). Although another study did not find this impact on sugar metabolism with donor FMT, slight improvements in HbA1c post-FMT were noted. Regional differences among study populations and variations in FMT administration routes should be considered. Additionally, differences in transplanted microbial species, donor and recipient selection, and concurrent changes in microbiota and lifestyle are important factors to take into account (109). Furthermore, gut microbiota may produce metabolites that alter human behavior directly through the blood-brain barrier or indirectly by regulating gut-autonomic nerve activity, leading to changes in satiety and mood. Hence, Fecal Microbiota Transplantations (FMTs) may exert their influence on the gut-brain axis, modulating metabolites linked to emotions and satiety, pivotal factors in insulin resistance development. The metabolic profile of FMT donors might similarly impact the gut-brain axis in human recipients, potentially steering gut microbiota to regulate dopamine and serotonin transporters to the brain (110). Furthermore, allogeneic FMT derived from donors with metabolic syndrome feces led to reduced insulin sensitivity in metabolic syndrome recipients compared to those utilizing donors post-Roux-en-Y gastric bypass surgery (111). It is essential to highlight that these studies did not monitor blood glucose levels during the intervention, and not all participants responded to FMT (104).
In an animal experiment, Wang et al. (112) demonstrated that the reconstruction of the microbiota in type 2 diabetes (T2D) mice through FMT could reverse insulin resistance. In a groundbreaking study, Hu et al. (113) initially indicated that FMT alleviated tubulointerstitial damage in diabetic rats by mediating disruptions in cholesterol balance. Recently, researchers discovered in a preclinical mouse model of diabetic kidney disease (DKD), mirroring changes in human DKD, that FMT prevented weight gain, reduced albuminuria, mitigated local intestinal inflammation, and improved insulin resistance, providing new evidence for the role of FMT in diabetic patients (10). Moreover, Shang et al. (114) recently reported that Fecal Microbiota Transplantation (FMT), in comparison to empagliflozin treatment, exhibited a more pronounced reduction in blood glucose levels and ameliorated pathological damage in diabetic kidney disease (DKD) mice by modulating the gut microbiota. Additionally, FMT and Lactobacillus transplantation demonstrated improvements in testosterone levels and influenced insulin function in a rat model of polycystic ovary syndrome (115). Furthermore, FMT from a healthy donor significantly enhanced the composition of the gut microbiota in db/db mice, leading to reduced plasma glucose and lipid levels, notably with an elevation in the levels of the mucin-degrading bacteriophage A. muciniphila (116). Transfer of fecal samples from type 2 diabetes patients undergoing metformin treatment into germ-free mice resulted in enhanced glucose tolerance in the recipient mice (117). Similarly, investigations indicated that transferring fecal samples from type 2 diabetes patients treated with DPP-4 inhibitors into germ-free mice improved glucose tolerance, particularly in response to impairments induced by high-density lipoprotein cholesterol (118).
4 Discussion
Fecal Microbiota Transplantation (FMT) alone proves insufficient in effectively controlling blood glucose levels. Therefore, combining the modulation of gut microbiota with other established treatments for Diabetes Mellitus (DM), including lifestyle adjustments, medications such as metformin, sodium-glucose co-transporter-2 inhibitors (SGLT2i), GLP-1 receptor agonists, and lipid-lowering drugs like statins, yields improved metabolic parameters, ultimately alleviating the damage brought about by this complex pathophysiology (10).
When contemplating the transfer of microbiota from a donor to a recipient, it is crucial to account for potential variations in microbiota composition arising from disparate lifestyles between the donors and recipients. Therefore, if the recipient’s lifestyle fails to transition toward that of the donor post-FMT, the influence on microbiota composition is likely to attenuate over time (119). Various intervention studies convincingly indicate that diet influences microbiota composition, with changes occurring rapidly within days or weeks (120, 121). These changes can be robust, as demonstrated by studies collecting and performing autologous FMT during weight loss, maintaining the benefits of weight loss and blood sugar control even when specific microbiota characteristics and dietary habits are no longer adhered to (106). Conversely, individual microbiota composition can influence the response to dietary changes (122). These collective observations imply that dietary factors may independently influence the response to FMT by shaping the microbiota composition. Consequently, the standardization of dietary conditions during interventions has the potential to bolster the statistical robustness of FMT, eliminating a notable source of microbiota variability. Furthermore, it is noteworthy that exercise and physical fitness, although exerting a comparatively modest impact compared to diet, also appear to influence microbiota composition (123).
In summary, the introduction of healthy FMT has the potential to modify the gut microbiota, playing a protective role in the progression of T2DM. Nevertheless, a comprehensive understanding of the long-term outcomes of FMT in this context requires further elucidation (124). The imperative moving forward involves the conduct of additional high-quality prospective studies to furnish comprehensive and enduring safety and efficacy data, crucial for informing the clinical application of FMT in the management of T2DM.
Despite its limitations, FMT remains a crucial tool for investigating the causal relationships between the microbiota and a range of chronic diseases (125). To improve the effects of these studies, efforts should be directed towards further standardizing FMT procedures, including dosage response, administration modes, pre-processing, and whether to use fresh, frozen, or alternative pre-processing materials. Furthermore, there is a necessity for improved endpoint definition and more rigorous power calculations to enhance the precision of treatment effect estimation. To achieve consensus in this field, we propose the periodic convening of focused meetings, building upon recently initiated efforts (88).
5 Conclusions
The gut microbiota plays a pivotal role in both the initiation and progression of diabetes, influencing the response of intestinal and extraintestinal tissues to anti-diabetic interventions. This review aims to provide a comprehensive overview of current research on the involvement and mechanisms of the gut microbiota in anti-diabetic therapy, with a specific focus on ameliorating inflammation, regulating glucose homeostasis, and mitigating insulin resistance through the administration of anti-diabetic medications. Despite the intricacies of molecular pathways remaining somewhat elusive, advancements in sequencing technology and bioinformatics have facilitated a profound understanding of the intricate composition and abundance of metabolites originating from gut bacteria within biological samples. Current literature suggests the significant role of various compounds such as lipopolysaccharides (LPS), short-chain fatty acids (SCFAs), bile acids, and indoles in orchestrating the delicate balance of host metabolism. However, the specific bacterial strains responsible for inducing changes in these metabolites and the feasibility of culturing these strains in vitro remain uncertain and warrant further exploration. Additionally, the intricate mechanisms through which altered metabolites exert their regulatory effects on host metabolism need to be elucidated. It is imperative to acknowledge that a substantial portion of insights gleaned thus far emanates from animal experiments. Therefore, a critical challenge is discerning which observed phenotypes in animal models are pertinent to humans and can be faithfully replicated in human subjects. Consequently, an intensified focus on conducting more clinical trials is paramount to elucidate the potential therapeutic efficacy of gut bacteria and their derived metabolites in the context of diabetes.
Author contributions
HW: Data curation, Investigation, Methodology, Writing – original draft. SL: Writing – original draft, Investigation, Methodology, Validation. LZ: Writing – original draft, Formal Analysis, Project administration, Writing – review & editing. NZ: Writing – review & editing, Conceptualization, Project administration, Supervision.
Funding
The author(s) declare financial support was received for the research, authorship, and/or publication of this article. This research is supported by the project of innovative and entrepreneurial talents funding project of Jilin Province (2023QN07), the Education Department (JJKH20211209KJ), the Science and Technology Department of Jilin Province (20230203071SF) and the Finance Department of Jilin Province (JLSWSRCZX2020083). The additional funds support the project code: 3R2231333428.
Acknowledgments
We would like to express our gratitude for the illustration materials provided by BioRender.
Conflict of interest
The authors declare that the research was conducted in the absence of any commercial or financial relationships that could be construed as a potential conflict of interest.
Publisher’s note
All claims expressed in this article are solely those of the authors and do not necessarily represent those of their affiliated organizations, or those of the publisher, the editors and the reviewers. Any product that may be evaluated in this article, or claim that may be made by its manufacturer, is not guaranteed or endorsed by the publisher.
References
1. Demir S, Nawroth PP, Herzig S, Ekim Üstünel B. Emerging targets in type 2 diabetes and diabetic complications. Advanced Sci (Weinheim Baden-Wurttemberg Germany). (2021) 8:e2100275. doi: 10.1002/advs.202100275
2. Ali MK, Pearson-Stuttard J, Selvin E, Gregg EW. Interpreting global trends in type 2 diabetes complications and mortality. Diabetologia. (2022) 65:3–13. doi: 10.1007/s00125-021-05585-2
3. Zheng Y, Ley SH, Hu FB. Global aetiology and epidemiology of type 2 diabetes mellitus and its complications. Nat Rev Endocrinol. (2018) 14:88–98. doi: 10.1038/nrendo.2017.151
4. Goldhaber-Fiebert JD, Li H, Ratanawijitrasin S, Vidyasagar S, Wang XY, Aljunid S, et al. Inpatient treatment of diabetic patients in Asia: evidence from India, China, Thailand and Malaysia. Diabetic Med: J Br Diabetic Assoc. (2010) 27:101–8. doi: 10.1111/j.1464-5491.2009.02874.x
5. Clemente JC, Manasson J, Scher JU. The role of the gut microbiome in systemic inflammatory disease. BMJ. (2018) 360:j5145. doi: 10.1136/bmj.j5145
6. O’Hara AM, Shanahan F. The gut flora as a forgotten organ. EMBO Rep. (2006) 7:688–93. doi: 10.1038/sj.embor.7400731
7. Flint HJ, Duncan SH, Scott KP, Louis P. Links between diet, gut microbiota composition and gut metabolism. Proc Nutr Soc. (2015) 74:13–22. doi: 10.1017/S0029665114001463
8. Maurice CF, Haiser HJ, Turnbaugh PJ. Xenobiotics shape the physiology and gene expression of the active human gut microbiome. Cell. (2013) 152:39–50. doi: 10.1016/j.cell.2012.10.052
9. Caporaso JG, Lauber CL, Costello EK, Berg-Lyons D, Gonzalez A, Stombaugh J, et al. Moving pictures of the human microbiome. Genome Biol. (2011) 12:R50. doi: 10.1186/gb-2011-12-5-r50
10. Bastos RMC, Simplício-Filho A, Sávio-Silva C, Oliveira LFV, Cruz GNF, Sousa EH, et al. Fecal microbiota transplant in a pre-clinical model of type 2 diabetes mellitus, obesity and diabetic kidney disease. Int J Mol Sci. (2022) 23(7):3842. doi: 10.3390/ijms23073842
11. Vendrik KEW, Ooijevaar RE, de Jong PRC, Laman JD, van Oosten BW, van Hilten JJ, et al. Fecal microbiota transplantation in neurological disorders. Front Cell Infect Microbiol. (2020) 10:98. doi: 10.3389/fcimb.2020.00098
12. Zhou Z, Wang H, Tan S, Zhang H, Zhu Y. The alterations of innate immunity and enhanced severity of infections in diabetes mellitus. Immunology. (2023) 171(3):313–23. doi: 10.1111/imm.v171.3
13. Xourafa G, Korbmacher M, Roden M. Inter-organ crosstalk during development and progression of type 2 diabetes mellitus. Nat Rev Endocrinol. (2023) 20(1):27–49. doi: 10.1038/s41574-023-00898-1
14. Ley RE, Lozupone CA, Hamady M, Knight R, Gordon JI. Worlds within worlds: evolution of the vertebrate gut microbiota. Nat Rev Microbiol. (2008) 6:776–88. doi: 10.1038/nrmicro1978
15. Gilbert JA, Blaser MJ, Caporaso JG, Jansson JK, Lynch SV, Knight R. Current understanding of the human microbiome. Nat Med. (2018) 24:392–400. doi: 10.1038/nm.4517
16. Xiong HH, Lin SY, Chen LL, Ouyang KH, Wang WJ. The interaction between flavonoids and intestinal microbes: A review. Foods. (2023) 12(2):320. doi: 10.3390/foods12020320
17. Zhao Y, Gong C, Xu J, Chen D, Yang B, Chen Z, et al. Research progress of fecal microbiota transplantation in liver diseases. J Clin Med. (2023) 12(4):1683. doi: 10.3390/jcm12041683
18. Khanna S, Vazquez-Baeza Y, González A, Weiss S, Schmidt B, Muñiz-Pedrogo DA, et al. Changes in microbial ecology after fecal microbiota transplantation for recurrent C. difficile infection affected by underlying inflammatory bowel disease. Microbiome. (2017) 5:55. doi: 10.1186/s40168-017-0269-3
19. Minton K. Mucosal immunology: Glucose not good for the gut. Nat Rev Immunol. (2018) 18:291. doi: 10.1038/nri.2018.22
20. Lan T, Tang T, Li Y, Duan Y, Yuan Q, Liu W, et al. FTZ polysaccharides ameliorate kidney injury in diabetic mice by regulating gut-kidney axis. Phytomedicine. (2023) 118:154935. doi: 10.1016/j.phymed.2023.154935
21. Hu J, Luo H, Wang J, Tang W, Lu J, Wu S, et al. Enteric dysbiosis-linked gut barrier disruption triggers early renal injury induced by chronic high salt feeding in mice. Exp Mol Med. (2017) 49:e370. doi: 10.1038/emm.2017.122
22. Mu Q, Kirby J, Reilly CM, Luo XM. Leaky gut as a danger signal for autoimmune diseases. Front Immunol. (2017) 8:598. doi: 10.3389/fimmu.2017.00598
23. Mafra D, Kemp JA, Borges NA, Wong M, Stenvinkel P. Gut microbiota interventions to retain residual kidney function. Toxins (Basel). (2023) 15(8):499. doi: 10.3390/toxins15080499
24. Andersen K, Kesper MS, Marschner JA, Konrad L, Ryu M, Kumar Vr S, et al. Intestinal dysbiosis, barrier dysfunction, and bacterial translocation account for CKD-related systemic inflammation. J Am Soc Nephrol. (2017) 28:76–83. doi: 10.1681/ASN.2015111285
25. Shi N, Li N, Duan X, Niu H. Interaction between the gut microbiome and mucosal immune system. Mil Med Res. (2017) 4:14. doi: 10.1186/s40779-017-0122-9
26. Loeser K, Seemann S, König S, Lenhardt I, Abdel-Tawab M, Koeberle A, et al. Protective effect of casperome(®), an orally bioavailable frankincense extract, on lipopolysaccharide- induced systemic inflammation in mice. Front Pharmacol. (2018) 9:387. doi: 10.3389/fphar.2018.00387
27. Geng S, Wang S, Zhu W, Xie C, Li X, Wu J, et al. Curcumin suppresses JNK pathway to attenuate BPA-induced insulin resistance in LO2 cells. BioMed Pharmacother. (2018) 97:1538–43. doi: 10.1016/j.biopha.2017.11.069
28. He L, Yang FQ, Tang P, Gao TH, Yang CX, Tan L, et al. Regulation of the intestinal flora: A potential mechanism of natural medicines in the treatment of type 2 diabetes mellitus. BioMed Pharmacother. (2022) 151:113091. doi: 10.1016/j.biopha.2022.113091
29. Mardinoglu A, Shoaie S, Bergentall M, Ghaffari P, Zhang C, Larsson E, et al. The gut microbiota modulates host amino acid and glutathione metabolism in mice. Mol Syst Biol. (2015) 11:834. doi: 10.15252/msb.20156487
30. Jones RM, Neish AS. Redox signaling mediated by the gut microbiota. Free Radic Biol Med. (2017) 105:41–7. doi: 10.1016/j.freeradbiomed.2016.10.495
31. Liu J, Ting JP, Al-Azzam S, Ding Y, Afshar S. Therapeutic advances in diabetes, autoimmune, and neurological diseases. Int J Mol Sci. (2021) 22(6):2805. doi: 10.3390/ijms22062805
32. Oda Y, Nishi H, Nangaku M. Role of inflammation in progression of chronic kidney disease in type 2 diabetes mellitus: clinical implications. Semin Nephrol. (2023) 43:151431. doi: 10.1016/j.semnephrol.2023.151431
33. Li MY, Duan JQ, Wang XH, Liu M, Yang QY, Li Y, et al. Inulin inhibits the inflammatory response through modulating enteric glial cell function in type 2 diabetic mellitus mice by reshaping intestinal flora. ACS Omega. (2023) 8:36729–43. doi: 10.1021/acsomega.3c03055
34. Qin J, Li Y, Cai Z, Li S, Zhu J, Zhang F, et al. A metagenome-wide association study of gut microbiota in type 2 diabetes. Nature. (2012) 490:55–60. doi: 10.1038/nature11450
35. Fan Y, Pedersen O. Gut microbiota in human metabolic health and disease. Nat Rev Microbiol. (2021) 19:55–71. doi: 10.1038/s41579-020-0433-9
36. Chen S, Han P, Zhang Q, Liu P, Liu J, Zhao L, et al. Lactobacillus brevis alleviates the progress of hepatocellular carcinoma and type 2 diabetes in mice model via interplay of gut microflora, bile acid and NOTCH 1 signaling. Front Immunol. (2023) 14:1179014. doi: 10.3389/fimmu.2023.1179014
37. Zhang Y, Li Y, Wang X, Huang J, Feng X, Shi C, et al. Lactobacillus Plantarum NC8 and its metabolite acetate alleviate type 1 diabetes via inhibiting NLRP3. Microb Pathog. (2023) 182:106237. doi: 10.1016/j.micpath.2023.106237
38. Li J, Yang G, Zhang Q, Liu Z, Jiang X, Xin Y. Function of Akkermansia muciniphila in type 2 diabetes and related diseases. Front Microbiol. (2023) 14:1172400. doi: 10.3389/fmicb.2023.1172400
39. de la Cuesta-Zuluaga J, Mueller NT, Corrales-Agudelo V, Velásquez-Mejía EP, Carmona JA, Abad JM, et al. Metformin is associated with higher relative abundance of mucin-degrading akkermansia muciniphila and several short-chain fatty acid-producing microbiota in the gut. Diabetes Care. (2017) 40:54–62. doi: 10.2337/dc16-1324
40. Shin NR, Lee JC, Lee HY, Kim MS, Whon TW, Lee MS, et al. An increase in the Akkermansia spp. population induced by metformin treatment improves glucose homeostasis in diet-induced obese mice. Gut. (2014) 63:727–35. doi: 10.1136/gutjnl-2012-303839
41. Karlsson FH, Tremaroli V, Nookaew I, Bergström G, Behre CJ, Fagerberg B, et al. Gut metagenome in European women with normal, impaired and diabetic glucose control. Nature. (2013) 498:99–103. doi: 10.1038/nature12198
42. He K, Guo LL, Tang H, Peng X, Li J, Feng S, et al. A freshwater fish-based diet alleviates liver steatosis by modulating gut microbiota and metabolites: A clinical randomized controlled trial in chinese participants with nonalcoholic fatty liver disease. Am J Gastroenterol. (2022) 117:1621–31. doi: 10.14309/ajg.0000000000001885
43. Gurung M, Li Z, You H, Rodrigues R, Jump DB, Morgun A, et al. Role of gut microbiota in type 2 diabetes pathophysiology. EBioMedicine. (2020) 51:102590. doi: 10.1016/j.ebiom.2019.11.051
44. Guo XJ, Dai SX, Lou JD, Ma XX, Hu XJ, Tu LP, et al. Distribution characteristics of oral microbiota and its relationship with intestinal microbiota in patients with type 2 diabetes mellitus. Front Endocrinol (Lausanne). (2023) 14:1119201. doi: 10.3389/fendo.2023.1119201
45. Yang X, Li D, Zhang M, Feng Y, Jin X, Liu D, et al. Ginkgo biloba extract alleviates fatty liver hemorrhagic syndrome in laying hens via reshaping gut microbiota. J Anim Sci Biotechnol. (2023) 14:97. doi: 10.1186/s40104-023-00900-w
46. Roesch LF, Lorca GL, Casella G, Giongo A, Naranjo A, Pionzio AM, et al. Culture-independent identification of gut bacteria correlated with the onset of diabetes in a rat model. Isme J. (2009) 3:536–48. doi: 10.1038/ismej.2009.5
47. Eckburg PB, Bik EM, Bernstein CN, Purdom E, Dethlefsen L, Sargent M, et al. Diversity of the human intestinal microbial flora. Science. (2005) 308:1635–8. doi: 10.1126/science.1110591
48. He Y, Wu W, Wu S, Zheng HM, Li P, Sheng HF, et al. Linking gut microbiota, metabolic syndrome and economic status based on a population-level analysis. Microbiome. (2018) 6:172. doi: 10.1186/s40168-018-0557-6
49. Tilg H, Zmora N, Adolph TE, Elinav E. The intestinal microbiota fuelling metabolic inflammation. Nat Rev Immunol. (2020) 20:40–54. doi: 10.1038/s41577-019-0198-4
50. Lan Y, Sun Q, Ma Z, Peng J, Zhang M, Wang C, et al. Seabuckthorn polysaccharide ameliorates high-fat diet-induced obesity by gut microbiota-SCFAs-liver axis. Food Funct. (2022) 13:2925–37. doi: 10.1039/D1FO03147C
51. Walter J, Maldonado-Gómez MX, Martínez I. To engraft or not to engraft: an ecological framework for gut microbiome modulation with live microbes. Curr Opin Biotechnol. (2018) 49:129–39. doi: 10.1016/j.copbio.2017.08.008
52. Wang Q, McLoughlin RM, Cobb BA, Charrel-Dennis M, Zaleski KJ, Golenbock D, et al. A bacterial carbohydrate links innate and adaptive responses through Toll-like receptor 2. J Exp Med. (2006) 203:2853–63. doi: 10.1084/jem.20062008
53. Natividad JM, Lamas B, Pham HP, Michel ML, Rainteau D, Bridonneau C, et al. Bilophila wadsworthia aggravates high fat diet induced metabolic dysfunctions in mice. Nat Commun. (2018) 9:2802. doi: 10.1038/s41467-018-05249-7
54. Hodoşan V, Daina LG, Zaha DC, Cotrău P, Vladu A, Dorobanţu FR, et al. Pattern of Antibiotic Use among Hospitalized Patients at a Level One Multidisciplinary Care Hospital. Healthcare (Basel). (2023) 11(9):1302. doi: 10.3390/healthcare11091302
55. Ionescu MI, Neagoe D, Crăciun AM, Moldovan OT. The Gram-Negative Bacilli Isolated from Caves-Sphingomonas paucimobilis and Hafnia alvei and a Review of Their Involvement in Human Infections. Int J Environ Res Public Health. (2022) 19(4):2324. doi: 10.3390/ijerph19042324
56. Tanase DM, Gosav EM, Neculae E, Costea CF, Ciocoiu M, Hurjui LL, et al. Role of gut microbiota on onset and progression of microvascular complications of type 2 diabetes (T2DM). Nutrients. (2020) 12(12):3719. doi: 10.3390/nu12123719
57. Daryabor G, Atashzar MR, Kabelitz D, Meri S, Kalantar K. The effects of type 2 diabetes mellitus on organ metabolism and the immune system. Front Immunol. (2020) 11:1582. doi: 10.3389/fimmu.2020.01582
58. Shafiei-Jahani P, Hurrell BP, Galle-Treger L, Helou DG, Howard E, Painter J, et al. DR3 stimulation of adipose resident ILC2s ameliorates type 2 diabetes mellitus. Nat Commun. (2020) 11:4718. doi: 10.1038/s41467-020-18601-7
59. Taylor-Fishwick DA, Weaver JR, Grzesik W, Chakrabarti S, Green-Mitchell S, Imai Y, et al. Production and function of IL-12 in islets and beta cells. Diabetologia. (2013) 56:126–35. doi: 10.1007/s00125-012-2732-9
60. Rohm TV, Meier DT, Olefsky JM, Donath MY. Inflammation in obesity, diabetes, and related disorders. Immunity. (2022) 55:31–55. doi: 10.1016/j.immuni.2021.12.013
61. Wada J, Makino H. Innate immunity in diabetes and diabetic nephropathy. Nat Rev Nephrol. (2016) 12:13–26. doi: 10.1038/nrneph.2015.175
62. Duparc T, Plovier H, Marrachelli VG, Van Hul M, Essaghir A, Ståhlman M, et al. Hepatocyte MyD88 affects bile acids, gut microbiota and metabolome contributing to regulate glucose and lipid metabolism. Gut. (2017) 66:620–32. doi: 10.1136/gutjnl-2015-310904
63. Canfora EE, Meex RCR, Venema K, Blaak EE. Gut microbial metabolites in obesity, NAFLD and T2DM. Nat Rev Endocrinol. (2019) 15:261–73. doi: 10.1038/s41574-019-0156-z
64. Ma Q, Li Y, Li P, Wang M, Wang J, Tang Z, et al. Research progress in the relationship between type 2 diabetes mellitus and intestinal flora. BioMed Pharmacother. (2019) 117:109138. doi: 10.1016/j.biopha.2019.109138
65. Martínez-López YE, Esquivel-Hernández DA, Sánchez-Castañeda JP, Neri-Rosario D, Guardado-Mendoza R, Resendis-Antonio O. Type 2 diabetes, gut microbiome, and systems biology: A novel perspective for a new era. Gut Microbes. (2022) 14:2111952. doi: 10.1080/19490976.2022.2111952
66. Zhou YD, Liang FX, Tian HR, Luo D, Wang YY, Yang SR. Mechanisms of gut microbiota-immune-host interaction on glucose regulation in type 2 diabetes. Front Microbiol. (2023) 14:1121695. doi: 10.3389/fmicb.2023.1121695
67. Makki K, Deehan EC, Walter J, Bäckhed F. The impact of dietary fiber on gut microbiota in host health and disease. Cell Host Microbe. (2018) 23:705–15. doi: 10.1016/j.chom.2018.05.012
68. Scheithauer TPM, Rampanelli E, Nieuwdorp M, Vallance BA, Verchere CB, van Raalte DH, et al. Gut microbiota as a trigger for metabolic inflammation in obesity and type 2 diabetes. Front Immunol. (2020) 11:571731. doi: 10.3389/fimmu.2020.571731
69. Molinaro A, Wahlström A, Marschall HU. Role of bile acids in metabolic control. Trends Endocrinol Metab. (2018) 29:31–41. doi: 10.1016/j.tem.2017.11.002
70. Fiorucci S, Mencarelli A, Palladino G, Cipriani S. Bile-acid-activated receptors: targeting TGR5 and farnesoid-X-receptor in lipid and glucose disorders. Trends Pharmacol Sci. (2009) 30:570–80. doi: 10.1016/j.tips.2009.08.001
71. Yang Q, Vijayakumar A, Kahn BB. Metabolites as regulators of insulin sensitivity and metabolism. Nat Rev Mol Cell Biol. (2018) 19:654–72. doi: 10.1038/s41580-018-0044-8
72. Sommer F, Anderson JM, Bharti R, Raes J, Rosenstiel P. The resilience of the intestinal microbiota influences health and disease. Nat Rev Microbiol. (2017) 15:630–8. doi: 10.1038/nrmicro.2017.58
73. Schiattarella GG, Sannino A, Toscano E, Giugliano G, Gargiulo G, Franzone A, et al. Gut microbe-generated metabolite trimethylamine-N-oxide as cardiovascular risk biomarker: a systematic review and dose-response meta-analysis. Eur Heart J. (2017) 38:2948–56. doi: 10.1093/eurheartj/ehx342
74. Tan YQ, Wang YN, Feng HY, Guo ZY, Li X, Nie XL, et al. Host/microbiota interactions-derived tryptophan metabolites modulate oxidative stress and inflammation via aryl hydrocarbon receptor signaling. Free Radic Biol Med. (2022) 184:30–41. doi: 10.1016/j.freeradbiomed.2022.03.025
75. Tuomainen M, Lindström J, Lehtonen M, Auriola S, Pihlajamäki J, Peltonen M, et al. Associations of serum indolepropionic acid, a gut microbiota metabolite, with type 2 diabetes and low-grade inflammation in high-risk individuals. Nutr Diabetes. (2018) 8:35. doi: 10.1038/s41387-018-0046-9
76. Chen J, Wang Q, Li R, Li Z, Jiang Q, Yan F, et al. The role of sirtuins in the regulatin of oxidative stress during the progress and therapy of type 2 diabetes mellitus. Life Sci. (2023) 333:122187. doi: 10.1016/j.lfs.2023.122187
77. Khemka S, Reddy A, Garcia RI, Jacobs M, Reddy RP, Roghani AK, et al. Role of diet and exercise in aging, Alzheimer’s disease, and other chronic diseases. Ageing Res Rev. (2023) 91:102091. doi: 10.1016/j.arr.2023.102091
78. Nauck MA, Wefers J, Meier JJ. Treatment of type 2 diabetes: challenges, hopes, and anticipated successes. Lancet Diabetes Endocrinol. (2021) 9:525–44. doi: 10.1016/S2213-8587(21)00113-3
79. Boicean A, Bratu D, Bacila C, Tanasescu C, Fleacă RS, Mohor CI, et al. Therapeutic perspectives for microbiota transplantation in digestive diseases and neoplasia-A literature review. Pathogens. (2023) 12(6):766. doi: 10.3390/pathogens12060766
80. Allegretti JR, Mullish BH, Kelly C, Fischer M. The evolution of the use of faecal microbiota transplantation and emerging therapeutic indications. Lancet. (2019) 394:420–31. doi: 10.1016/S0140-6736(19)31266-8
81. Allegretti JR, Kassam Z, Mullish BH, Chiang A, Carrellas M, Hurtado J, et al. Effects of fecal microbiota transplantation with oral capsules in obese patients. Clin Gastroenterol Hepatol. (2020) 18:855–63.e2. doi: 10.1016/j.cgh.2019.07.006
82. Weingarden A, González A, Vázquez-Baeza Y, Weiss S, Humphry G, Berg-Lyons D, et al. Dynamic changes in short- and long-term bacterial composition following fecal microbiota transplantation for recurrent Clostridium difficile infection. Microbiome. (2015) 3:10. doi: 10.1186/s40168-015-0070-0
83. Antushevich H. Fecal microbiota transplantation in disease therapy. Clin Chim Acta. (2020) 503:90–8. doi: 10.1016/j.cca.2019.12.010
84. Alteri CJ. Can the microbiome deliver? A proof-of-concept engineered E. coli PKU therapeutic. Cell Host Microbe. (2019) 25:473–4. doi: 10.1016/j.chom.2019.03.015
85. Sorbara MT, Pamer EG. Microbiome-based therapeutics. Nat Rev Microbiol. (2022) 20:365–80. doi: 10.1038/s41579-021-00667-9
86. Zhang S, Deng F, Chen J, Chen F, Wu Z, Li L, et al. Fecal microbiota transplantation treatment of autoimmune-mediated type 1 diabetes: A systematic review. Front Cell Infect Microbiol. (2022) 12:1075201. doi: 10.3389/fcimb.2022.1075201
87. Zhang F, Luo W, Shi Y, Fan Z, Ji G. Should we standardize the 1,700-year-old fecal microbiota transplantation? Am J Gastroenterol. (2012) 107:1755. doi: 10.1038/ajg.2012.251
88. Cammarota G, Ianiro G, Tilg H, Rajilić-Stojanović M, Kump P, Satokari R, et al. European consensus conference on faecal microbiota transplantation in clinical practice. Gut. (2017) 66:569–80. doi: 10.1136/gutjnl-2016-313017
89. He J, He X, Ma Y, Yang L, Fang H, Shang S, et al. A comprehensive approach to stool donor screening for faecal microbiota transplantation in China. Microb Cell Fact. (2021) 20:216. doi: 10.1186/s12934-021-01705-0
90. Biazzo M, Deidda G. Fecal microbiota transplantation as new therapeutic avenue for human diseases. J Clin Med. (2022) 11(14):4119. doi: 10.3390/jcm11144119
91. Costello SP, Conlon MA, Vuaran MS, Roberts-Thomson IC, Andrews JM. Faecal microbiota transplant for recurrent Clostridium difficile infection using long-term frozen stool is effective: clinical efficacy and bacterial viability data. Aliment Pharmacol Ther. (2015) 42:1011–8. doi: 10.1111/apt.13366
92. Staley C, Kaiser T, Vaughn BP, Graiziger C, Hamilton MJ, Kabage AJ, et al. Durable Long-Term Bacterial Engraftment following Encapsulated Fecal Microbiota Transplantation To Treat Clostridium difficile Infection. mBio. (2019) 10(4):e01586–19. doi: 10.1128/mBio.01586-19
93. Zhang F, Cui B, He X, Nie Y, Wu K, Fan D. Microbiota transplantation: concept, methodology and strategy for its modernization. Protein Cell. (2018) 9:462–73. doi: 10.1007/s13238-018-0541-8
94. Cui B, Li P, Xu L, Peng Z, Xiang J, He Z, et al. Step-up fecal microbiota transplantation (FMT) strategy. Gut Microbes. (2016) 7:323–8. doi: 10.1080/19490976.2016.1151608
95. Marcella C, Cui B, Kelly CR, Ianiro G, Cammarota G, Zhang F. Systematic review: the global incidence of faecal microbiota transplantation-related adverse events from 2000 to 2020. Aliment Pharmacol Ther. (2021) 53:33–42. doi: 10.1111/apt.16148
96. Ma Y, Liu J, Rhodes C, Nie Y, Zhang F. Ethical issues in fecal microbiota transplantation in practice. Am J Bioeth. (2017) 17:34–45. doi: 10.1080/15265161.2017.1299240
97. Ganesan K, Chung SK, Vanamala J, Xu B. Causal relationship between diet-induced gut microbiota changes and diabetes: A novel strategy to transplant faecalibacterium prausnitzii in preventing diabetes. Int J Mol Sci. (2018) 19(12):3720. doi: 10.3390/ijms19123720
98. Paramsothy S, Nielsen S, Kamm MA, Deshpande NP, Faith JJ, Clemente JC, et al. Specific bacteria and metabolites associated with response to fecal microbiota transplantation in patients with ulcerative colitis. Gastroenterology. (2019) 156:1440–54.e2. doi: 10.1053/j.gastro.2018.12.001
99. Duan L, An X, Zhang Y, Jin D, Zhao S, Zhou R, et al. Gut microbiota as the critical correlation of polycystic ovary syndrome and type 2 diabetes mellitus. BioMed Pharmacother. (2021) 142:112094. doi: 10.1016/j.biopha.2021.112094
100. Camarillo-Guerrero LF, Almeida A, Rangel-Pineros G, Finn RD, Lawley TD. Massive expansion of human gut bacteriophage diversity. Cell. (2021) 184:1098–109.e9. doi: 10.1016/j.cell.2021.01.029
101. Ott SJ, Waetzig GH, Rehman A, Moltzau-Anderson J, Bharti R, Grasis JA, et al. Efficacy of sterile fecal filtrate transfer for treating patients with clostridium difficile infection. Gastroenterology. (2017) 152:799–811.e7. doi: 10.1053/j.gastro.2016.11.010
102. Wortelboer K, de Jonge PA, Scheithauer TPM, Attaye I, Kemper EM, Nieuwdorp M, et al. Phage-microbe dynamics after sterile faecal filtrate transplantation in individuals with metabolic syndrome: a double-blind, randomised, placebo-controlled clinical trial assessing efficacy and safety. Nat Commun. (2023) 14:5600. doi: 10.1038/s41467-023-41329-z
103. Vrieze A, Van Nood E, Holleman F, Salojärvi J, Kootte RS, Bartelsman JF, et al. Transfer of intestinal microbiota from lean donors increases insulin sensitivity in individuals with metabolic syndrome. Gastroenterology. (2012) 143:913–6.e7. doi: 10.1053/j.gastro.2012.06.031
104. Kootte RS, Levin E, Salojärvi J, Smits LP, Hartstra AV, Udayappan SD, et al. Improvement of insulin sensitivity after lean donor feces in metabolic syndrome is driven by baseline intestinal microbiota composition. Cell Metab. (2017) 26:611–9.e6. doi: 10.1016/j.cmet.2017.09.008
105. Cai TT, Ye XL, Yong HJ, Song B, Zheng XL, Cui BT, et al. Fecal microbiota transplantation relieve painful diabetic neuropathy: A case report. Med (Baltimore). (2018) 97:e13543. doi: 10.1097/MD.0000000000013543
106. Rinott E, Youngster I, Yaskolka Meir A, Tsaban G, Zelicha H, Kaplan A, et al. Effects of diet-modulated autologous fecal microbiota transplantation on weight regain. Gastroenterology. (2021) 160:158–73.e10. doi: 10.1053/j.gastro.2020.08.041
107. Alang N, Kelly CR. Weight gain after fecal microbiota transplantation. Open Forum Infect Dis. (2015) 2:ofv004. doi: 10.1093/ofid/ofv004
108. Ng SC, Xu Z, Mak JWY, Yang K, Liu Q, Zuo T, et al. Microbiota engraftment after faecal microbiota transplantation in obese subjects with type 2 diabetes: a 24-week, double-blind, randomised controlled trial. Gut. (2022) 71:716–23. doi: 10.1136/gutjnl-2020-323617
109. Yu EW, Gao L, Stastka P, Cheney MC, Mahabamunuge J, Torres Soto M, et al. Fecal microbiota transplantation for the improvement of metabolism in obesity: The FMT-TRIM double-blind placebo-controlled pilot trial. PloS Med. (2020) 17:e1003051. doi: 10.1371/journal.pmed.1003051
110. Hartstra AV, Schüppel V, Imangaliyev S, Schrantee A, Prodan A, Collard D, et al. Infusion of donor feces affects the gut-brain axis in humans with metabolic syndrome. Mol Metab. (2020) 42:101076. doi: 10.1016/j.molmet.2020.101076
111. de Groot P, Scheithauer T, Bakker GJ, Prodan A, Levin E, Khan MT, et al. Donor metabolic characteristics drive effects of faecal microbiota transplantation on recipient insulin sensitivity, energy expenditure and intestinal transit time. Gut. (2020) 69:502–12. doi: 10.1136/gutjnl-2019-318320
112. Wang H, Lu Y, Yan Y, Tian S, Zheng D, Leng D, et al. Promising treatment for type 2 diabetes: fecal microbiota transplantation reverses insulin resistance and impaired islets. Front Cell Infect Microbiol. (2019) 9:455. doi: 10.3389/fcimb.2019.00455
113. Hu ZB, Lu J, Chen PP, Lu CC, Zhang JX, Li XQ, et al. Dysbiosis of intestinal microbiota mediates tubulointerstitial injury in diabetic nephropathy via the disruption of cholesterol homeostasis. Theranostics. (2020) 10:2803–16. doi: 10.7150/thno.40571
114. Shang J, Cui W, Guo R, Zhang Y, Wang P, Yu W, et al. The harmful intestinal microbial community accumulates during DKD exacerbation and microbiome-metabolome combined validation in a mouse model. Front Endocrinol (Lausanne). (2022) 13:964389. doi: 10.3389/fendo.2022.964389
115. Guo Y, Qi Y, Yang X, Zhao L, Wen S, Liu Y, et al. Association between polycystic ovary syndrome and gut microbiota. PloS One. (2016) 11:e0153196. doi: 10.1371/journal.pone.0153196
116. Zhang PP, Li LL, Han X, Li QW, Zhang XH, Liu JJ, et al. Fecal microbiota transplantation improves metabolism and gut microbiome composition in db/db mice. Acta Pharmacol Sin. (2020) 41:678–85. doi: 10.1038/s41401-019-0330-9
117. Wu H, Esteve E, Tremaroli V, Khan MT, Caesar R, Mannerås-Holm L, et al. Metformin alters the gut microbiome of individuals with treatment-naive type 2 diabetes, contributing to the therapeutic effects of the drug. Nat Med. (2017) 23:850–8. doi: 10.1038/nm.4345
118. Liao X, Song L, Zeng B, Liu B, Qiu Y, Qu H, et al. Alteration of gut microbiota induced by DPP-4i treatment improves glucose homeostasis. EBioMedicine. (2019) 44:665–74. doi: 10.1016/j.ebiom.2019.03.057
119. Hanssen NMJ, de Vos WM, Nieuwdorp M. Fecal microbiota transplantation in human metabolic diseases: From a murky past to a bright future? Cell Metab. (2021) 33:1098–110. doi: 10.1016/j.cmet.2021.05.005
120. David LA, Maurice CF, Carmody RN, Gootenberg DB, Button JE, Wolfe BE, et al. Diet rapidly and reproducibly alters the human gut microbiome. Nature. (2014) 505:559–63. doi: 10.1038/nature12820
121. Brüssow H, Parkinson SJ. You are what you eat. Nat Biotechnol. (2014) 32:243–5. doi: 10.1038/nbt.2845
122. Kolodziejczyk AA, Zheng D, Elinav E. Diet-microbiota interactions and personalized nutrition. Nat Rev Microbiol. (2019) 17:742–53. doi: 10.1038/s41579-019-0256-8
123. Cronin O, Barton W, Skuse P, Penney NC, Garcia-Perez I, Murphy EF, et al. A prospective metagenomic and metabolomic analysis of the impact of exercise and/or whey protein supplementation on the gut microbiome of sedentary adults. mSystems. (2018) 3(3):e00044–18. doi: 10.1128/mSystems.00044-18
124. Wu X, Zhao L, Zhang Y, Li K, Yang J. The role and mechanism of the gut microbiota in the development and treatment of diabetic kidney disease. Front Physiol. (2023) 14:1166685. doi: 10.3389/fphys.2023.1166685
Keywords: type 2 diabetes mellitus, fecal microbiota transplantation, gut microbiota, dysbiosis, metabolites
Citation: Wang H, Li S, Zhang L and Zhang N (2024) The role of fecal microbiota transplantation in type 2 diabetes mellitus treatment. Front. Endocrinol. 15:1469165. doi: 10.3389/fendo.2024.1469165
Received: 23 July 2024; Accepted: 22 November 2024;
Published: 13 December 2024.
Edited by:
Åke Sjöholm, Gävle Hospital, SwedenReviewed by:
Cosmin Mihai Vesa, University of Oradea, RomaniaRyan Russell, The University of Texas Rio Grande Valley, United States
Copyright © 2024 Wang, Li, Zhang and Zhang. This is an open-access article distributed under the terms of the Creative Commons Attribution License (CC BY). The use, distribution or reproduction in other forums is permitted, provided the original author(s) and the copyright owner(s) are credited and that the original publication in this journal is cited, in accordance with accepted academic practice. No use, distribution or reproduction is permitted which does not comply with these terms.
*Correspondence: Nan Zhang, emhhbmduYW5Aamx1LmVkdS5jbg==; Luping Zhang, emhhbmdsdXBpbmdAamx1LmVkdS5jbg==
†These authors have contributed equally to this work