- 1Department of Plastic Surgery, Renmin Hospital of Wuhan University, Wuhan, Hubei, China
- 2Department of Dermatology, Union Hospital, Tongji Medical College, Huazhong University of Science and Technology (HUST), Wuhan, China
- 3Department of Plastic and Cosmetic Surgery, Tongji Hospital, Tongji Medical College, Huazhong University of Science and Technology, Wuhan, China
- 4Department of Wound Repair Surgery, Liyuan Hospital, Tongji Medical College, Huazhong University of Science and Technology, Wuhan, Hubei, China
Diabetic wound healing is a complex physiological process often hindered by the underlying metabolic dysfunctions associated with diabetes. Despite existing treatments, there remains a critical need to explore innovative therapeutic strategies to improve patient outcomes. This article comprehensively examines the roles of non-coding RNAs (ncRNAs), specifically microRNAs (miRNAs), long non-coding RNAs (lncRNAs), and circular RNAs (circRNAs), in regulating key phases of the wound healing process: inflammation, angiogenesis, re-epithelialization, and tissue remodeling. Through a deep review of current literature, we discuss recent discoveries of ncRNAs that have been shown to either promote or impair the wound healing process in diabetic wound healing, which were not covered in earlier reviews. This review highlights the specific mechanisms by which these ncRNAs impact cellular behaviors and pathways critical to each healing stage. Our findings indicate that understanding these recently identified ncRNAs provides new insights into their potential roles in diabetic wound healing, thereby contributing valuable knowledge for future research directions in this field.
1 Introduction
Skin repair is a tightly regulated biological procedure, comprising: hemostasis, inflammation, proliferation, and remodeling (1, 2). Hemostasis initiates when the vascular endothelium is damaged, leading to the formation of a platelet plug and a fibrin clot to stop bleeding, a process that takes minutes. The inflammatory phase starts right after hemostasis, this phase begins with an influx of neutrophils, followed by the arrival of monocytes that differentiate into macrophages within the tissues to clear away any remaining cellular remnants and expended neutrophils, lasting between three to five days (3, 4). In the next proliferation phase, the process encompasses the generation of new stromal structures by fibroblasts, the beginning of angiogenesis, extracellular matrix (ECM) formation, and collagen synthesis (5). During the final remodeling phase, the structure of the tissue is refined through the final formation of the ECM and further neovascularization. This phase sees significant contributions from fibroblasts, which produce fibroblast growth factors (FGF), and from vascular endothelial cells (ECs). The remodeling process, pivotal for restoring tissue integrity, can last up to two years (6–8) (Figure 1).
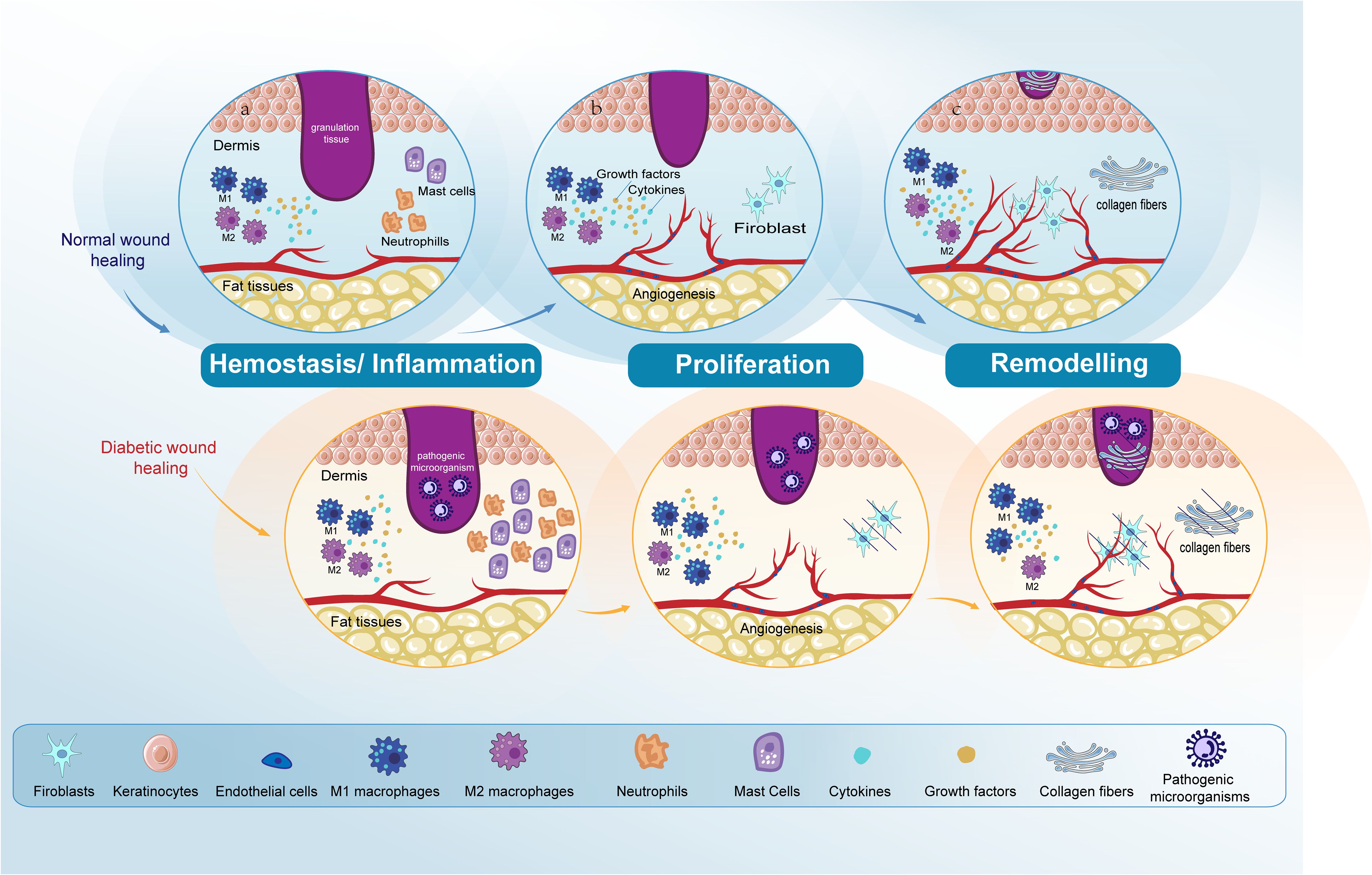
Figure 1. Comparative analysis of normal and diabetic wound healing phases. The figure compares normal wound healing with diabetic wound healing in the Hemostasis/Inflammation, Proliferation, and Remodeling phases. (A) Hemostasis/Inflammation phase: In normal wound healing, this phase is characterized by a balanced inflammatory response. In contrast, diabetic wound healing shows an increased presence of neutrophils and mast cells. M1 macrophage polarization is observed in diabetic wounds, leading to prolonged inflammation and impaired healing. (B) Proliferation phase: In normal wound healing, keratinocytes and fibroblasts proliferate and migrate effectively, and angiogenesis progresses normally. However, in diabetic wounds, there is impaired proliferation and migration of keratinocytes and fibroblasts. Angiogenesis is also impaired due to dysfunctional ECs. (C) Remodeling phase: In normal wound healing, this phase results in the final formation of the ECM and the completion of angiogenesis. Conversely, in diabetic wound healing, both ECM formation and angiogenesis remain impaired. Endothelial cells (ECs); extracellular matrix (ECM).
In comparison to acute wounds, chronic wounds often exhibit pathological irregularities and impairments in different cell functions, such as those of neutrophils, macrophages, ECs, fibroblasts, and keratinocytes (6, 9). Non-healing wounds pose a major challenge to public health (10). Diabetic skin exhibits increased infiltration of inflammatory cells and reduced granulation tissue formation compared to normal skin. The primary factors that complicate diabetic wound healing, in contrast to normal wound healing, include elevated glucose levels, hypoxia, and high levels of reactive oxygen species (ROS) (11, 12). Elevated glucose levels hinder the transition from pro-inflammatory M1 macrophages to anti-inflammatory M2 macrophages, thereby disrupting the resolution of inflammation. Additionally, high glucose concentrations provide increased nutrient availability, promoting bacterial growth and proliferation, which escalates the risk of infection. Furthermore, diabetic wounds often exhibit dysregulated cytokine release by neutrophils during the inflammatory phase, thereby creating a favorable environment for wound infection (13). Beyond this elevated glucose levels rigidify cell membranes and constrict blood vessels, thereby reducing blood flow and decreasing the availability of nutrients and oxygen at the wound site (14). Elevated glucose levels promote the overexpression of matrix metalloproteinases (MMPs) such as MMP-9 and MMP-2, leading to excessive ECM degradation, impaired proliferation of keratinocytes and fibroblasts, and hindered angiogenesis. However, certain MMPs, like MMP-8, can be beneficial for diabetic wound healing (15). Elevated blood glucose levels, a key characteristic of diabetic wound environments, represent the primary barrier to effective healing. Thus, the primary focus in the clinical treatment of diabetic wounds is to manage blood sugar levels effectively (14).
Hypoxia in diabetic patients results from restricted oxygen supply and increased oxygen consumption in the wound. Vascular dysfunction and neuropathy limit oxygen delivery to the wound site. Reduced blood oxygen levels lead to decreased expression of hypoxia-inducible factor-1α (HIF-1α) and its target genes, impairing the cellular response to hypoxia. This disruption affects angiogenesis, resulting in delayed wound healing (16). Hypoxic environments negatively affect various cells, including ECs, macrophages, keratinocytes, and fibroblasts. Consequently, hyperbaric oxygen therapy has been extensively used in the treatment of diabetic foot ulcers (DFUs), although the exact mechanisms of hyperbaric oxygen treatment remain unclear (17).
Oxidative stress arises from an overproduction of ROS and insufficient antioxidant defenses. Hyperglycemia is a key mechanism for inducing oxidative stress, and ROS are crucial regulators of several phases of wound healing (12). Low levels of ROS are necessary to defend against external damage (18). However, excessive oxidative stress and reduced antioxidant capacity are major contributors to non-healing diabetic wounds (19). These factors disrupt normal healing stages by causing abnormalities and as a result, wounds can experience impaired healing progression, leading to numerous clinical complications (6, 20, 21). Importantly, diabetic ulcers stand as the primary reason for lower limb amputations (22). Currently, 10.5% of the global adult population between the ages of 20 and 79 have diabetes, amounting to 537 million people worldwide. Estimates suggest this figure will escalate to 643 million by the year 2030 and further increase to 783 million individuals living with diabetes by 2045 (23, 24). Current basic treatments for diabetic wound healing include blood sugar control, debridement, and infection control using topical or systemic antibiotics. Advanced therapies also play a significant role, including the development of skin substitutes, negative pressure wound therapy, and hyperbaric oxygen therapy. Additionally, new wound dressings that incorporate growth factors and bioengineered tissues are being utilized to enhance the healing process (25, 26).
Despite the availability of treatments for wound healing in diabetic patients, the persistently elevated rate of negative outcomes underscores the critical necessity for pioneering therapeutic methods. In this review, we focused on the role of non-coding RNAs (ncRNAs), including microRNAs (miRNAs), long non-coding RNAs (lncRNAs), and circular RNAs (circRNAs) in diabetic wound healing explaining their roles in this specific process to enhance our comprehension of the complex mechanisms behind DFUs and to devise novel approaches to facilitate the restoration of wounds in diabetic individuals.
2 Non-coding RNAs
NcRNAs, making up 99% of the RNA content in cells, play a pivotal role in influencing specific cellular responses. NcRNAs achieve this by modifying the expression or function of a wide array of downstream targets (27). Depending on the size of their nucleotide sequences, ncRNAs are categorized into two main groups: short ncRNAs comprising less than 200 nucleotide units, and lncRNAs, which are composed of nucleotide chains exceeding 200 units in length (28). NcRNAs operate via diverse molecular processes, such as regulating the expression of specific genes, affecting protein function and activity, and interacting with relevant signaling pathways. Any abnormalities in their expression can lead to the onset of numerous diseases (29, 30). NcRNAs demonstrate connections to numerous disorders linked to diabetes mellitus (DM), including DFUs, diabetic nephropathy, diabetic cardiomyopathy, and diabetic peripheral neuropathy, through their interactions with proteins. Despite this, the specifics of how ncRNAs operate and exert their effects remain largely unknown, leaving many questions about their mechanisms of action unanswered (31–33). While all three types of ncRNAs have both beneficial and detrimental effects at different stages of diabetic wound healing, there are some major differences among them. LncRNAs, in contrast to miRNAs, are longer (over 200 nucleotides in length) and regulate gene expression at various levels, including chromatin modification, transcription, and post-transcriptional processing. They can also interact with and act as sponges for miRNAs to regulate gene expression (34). CircRNAs are unique among these three types due to their closed-loop structure, which gives them resistance to degradation, making them more stable and potentially better biomarkers for different diseases (35). Beyond their role in diabetic wound healing, ncRNAs serve as important resources for diagnosing and potentially treating a range of diseases. These include various types of cancer, cardiovascular diseases, rheumatoid arthritis, tuberculosis, kidney stones, and eye disorders (36–41).
3 Mi-RNAs
MiRNAs belong to a class of endogenous, small molecules of ncRNAs, which serve as the main modulators in the post-transcriptional regulation of gene expression. These molecules carry out their regulatory roles by specifically interacting with the three prime untranslated regions (3’-UTR) of target messenger RNAs (mRNAs) (42, 43), leading to mRNA cleavage, translational repression, or mRNA deadenylation. This intricate mechanism allows miRNAs to control a wide array of biological processes, making them crucial components in the cellular regulatory networks (44–47). The examination of miRNAs expression levels can significantly contribute to the diagnosis of various diseases (48, 49). For instance, the expression of miR-129 and miR-335 is notably reduced in the skin tissues of patients suffering from DM (50). Similarly, miR-296-5p expression is substantially reduced in DM tissues, compared to normal tissues (51). Furthermore, miR-488-3p levels are reduced within the wound tissues of individuals afflicted with DM (52). Conversely, upregulation of miR-222-3p has been obtained from individuals diagnosed with Type 2 diabetes mellitus (T2DM), and a decrease of miR-126-3p within circulating plasma has been documented among individuals suffering from diabetes mellitus (53, 54). In addition, miRNAs play a significant role in modulating the functionality of β-cells, adjusting the expression of genes crucial for maintaining pancreatic β-cell homeostasis (55, 56).
3.1 Inflammation
At the inflammatory stage, macrophages are the key contributors and exhibit significant flexibility, being able to differentiate into diverse phenotypic cells based on the environmental shifts they encounter (57). In diabetic wound healing the process is often impeded at the inflammatory phase due to the impaired shift from M1 to M2 macrophage (58, 59). Pro-inflammatory M1 macrophages are predominant in diabetic wound environments, with minimal presence of anti-inflammatory M2 macrophages. M1 macrophages release pro-inflammatory cytokines, including IL-1β, IL-6, IL-12, IL-18, IL-23, and TNF, which impede diabetic wound healing. In contrast, M2 macrophages produce anti-inflammatory cytokines such as IL-10 and IL-12, as well as growth factors like TGF-β, which facilitate wound healing. Therefore, maintaining a balance between M1 and M2 macrophages is crucial in the diabetic wound healing process (13). Unlike macrophages, neutrophils that evolve into neutrophil extracellular traps (NETs) exacerbate the wound’s inflammatory reaction, adversely affecting diabetic wound healing (60). Here we explore the molecular processes through which various types of miRNAs exert regulatory control over the inflammatory phase.
Multiple research endeavors have reported a reduction in miR-146a levels within macrophages of diabetic patients, underscoring the pivotal function miR-146a plays in regulating the inflammatory stage of impaired wound repair processes (61, 62). This functional significance is noted in the regulation of nuclear factor-kappa B (NF-κB) activation and the interleukin-1 receptor-associated kinase 1 (IRAK1) pathway (63, 64). Investigations into the therapeutic potential of conjugating cerium oxide nanoparticles (CNP) to miR-146a have demonstrated significant benefits in diabetic wound repair (61, 65). Li et al. explored a novel dressing approach by encapsulating miR-146a within exosomes and incorporating these exosomes into a silk fibroin patch (SFP). This innovative dressing demonstrated efficacy in downregulating the expression of IRAK1, Tumor Necrosis Factor-Alpha (TNF-α), Interleukin-1 beta (IL-1β), and Interleukin-6 (IL-6) in vitro in HaCaT cells, as well as IRAK1, and phosphorylated NFκB-p65 in diabetic mice, thereby promoting wound healing (66). Zhou et al. discovered that EXO-miR-146a shifts of macrophages toward the anti-inflammatory M2 macrophage by reducing TNF Receptor Associated Factor 6 (TRAF6) expression under high glucose conditions (67). In summary, miR-146a promotes diabetic wound healing by targeting and regulating key inflammatory molecules and pathways.
Research findings have indicated that miR-145a-5p exerts a beneficial influence on the wound repair processes in diabetic conditions promoting the polarization towards M2 macrophage (68, 69), through modulating the activity of the p21-Activated Kinase 7 (PAK7) and influencing β-catenin signaling in hyperlipidemia (69). The research conducted by Su and colleagues revealed that miR-145-5p, transferred through extracellular vesicles (EVs), directly modulates the expression of the cyclin-dependent kinase inhibitor 1A (CDKN1A), consequently activating the Erk/Akt, thus promoting wound healing in high glucose (HG)-induced human dermal fibroblasts as well as in murine models of diabetes mellitus in vivo (70). Contrarily, Wang and colleagues demonstrated that miR-145-5p exerts a detrimental influence on HG-induced impairment in human foreskin fibroblasts and hinders wound repair proven in murine models of diabetes mellitus. Furthermore, they showed that suppressing the expression of miR-145-5p facilitates an improvement in cellular functionality within fibroblast cultures and promotes the wound repair processes in DFUs mouse models through upregulating platelet-derived growth factor D (PDGFD) expression (71). These studies suggest that miR-145a-5p exhibits both beneficial and detrimental effects on diabetic wound healing.
Ban et al. revealed that treatment with miR-497 leads to a decrease in IL-1β, IL-6, and TNF-α levels thereby facilitating the wound healing process (72). MiR-21 was found to have an inhibition effect on the inflammatory response triggered by lipopolysaccharide (LPS) and increases IL-10 production by interacting with phosphatase and tensin homolog (PTEN) and programmed cell death 4 (PDCD4) genes (73). In other words, these miRNAs promote diabetic wound healing by regulating pro-inflammatory and anti-inflammatory cytokines.
NF-κB serves as a crucial regulator of the inflammatory response by controlling the expression of genes related to pro-inflammatory proteins such as cytokines, chemokines MMPs, growth factors, and proteins involved in apoptosis. Dysregulation of NF-κB leads to the development of inflammatory diseases (74). Consequently, targeting the NF-κB pathway has been proposed as a promising therapeutic strategy for managing diabetic complications. miR-185-5p and miR-132 have been found to have beneficial effects in diabetic wound healing by targeting NF-κB. Wang et al. observed a downregulation of miR-185-5p in the wound tissues of both DFU patients and diabetic rats, with the administration of miR-185-5p mimics shown to accelerate wound repair by inhibiting the expression of NF-κB and intercellular adhesion molecule 1 (ICAM-1) in diabetic rat wound tissue (75). Furthermore, The study by Ge et al. suggests that miR-132-exo promotes diabetic wound healing and improves the viability of skin flaps by facilitating M2 macrophage polarization and enhancing angiogenesis. The M2 macrophage polarization induced by miR-132-exo may be mediated by inhibiting the NF-κB signaling pathway through the suppression of p65 (76). Yang et al. revealed that miR-203a-3p targets inhibitor of cytokine signaling 3 (SOCS3), thereby activating the JAK2/STAT3. This activation leads to M2 macrophage polarization and subsequently enhances diabetic wound repair (77).
3.2 Angiogenesis
Angiogenesis, which involves generating novel blood vessels from existing ones, is a process that demands a carefully controlled mix of signals that either stimulate or inhibit (78–80). Diabetic wounds often have an impaired blood supply due to insufficient angiogenesis (81). Several proangiogenic factors, notably VEGF, FGF, and Epidermal Growth Factor (EGF), serve vital functions in angiogenesis (82, 83). VEGF plays a crucial role in regulating angiogenesis and vascular permeability. In diabetic patients, VEGF levels in the serum and vitreous are associated with blood glucose control (84).
Vascular Endothelial Growth Factor A (VEGFA) is the most studied member of the VEGF family. Research revealed an elevated presence of miR-195-5p and miR-205-5p in EVs from DFU wound fluid, and these miRNAs inhibit angiogenesis by directly targeting the 3′-UTR region of VEGFA mRNA, thereby regulating VEGFA expression (85). MiR-135a-3p negatively regulates angiogenesis, controlling the VEGF-induced activation of the p38MAPK pathway by targeting huntingtin interacting protein 1(HIP1) (86). MiR-615-5p was identified as a key inhibitor of angiogenesis in ECs by impacting the VEGF-AKT/eNOS and specifically interacting with the insulin-like growth factor 2 (IGF2) and Ras association domain-containing protein 2 (RASSF2) genes (87). Wang et al. detected upregulated levels of miR-199a-5p in the wound tissues of patients with DFUs. Subsequently, they demonstrated that in diabetic rats, the suppression of miR-199a-5p enhanced the VEGFA expression and Rho-associated protein kinase 1 (ROCK1), thereby promoting wound healing (88). In contrast, Zhou et al. demonstrated that the inhibition of hsa-miR-199a-5p by SNHG12/NFYC-AS1 promotes diabetic wound healing via the hsa-miR-199a-5p-S100A8/S100A7/XDH pathway (89).
Under diabetic conditions, HIF-1 upregulates the expression of VEGF highlighting the critical role of HIF-1 regulation in angiogenesis (90). Xiao et al. demonstrated that overexpression of miR-1248 promotes wound healing by targeting Cbp/p300-interacting transactivator 2 (CITED2) and subsequently modulating the activity of HIF-1 (91). HIF-1 has been also found to be associated with miR-31-5p in its mechanism of promoting angiogenesis in diabetic wound healing. Several studies have underscored the significant contribution of miR-31-5p in promoting angiogenesis by targeting factor-inhibiting HIF-1 (HIF1AN) (92, 93). Furthermore, it was successfully demonstrated that miR-31-5p encapsulated in exosomes promotes diabetic wound healing by enhancing angiogenesis (94). In patients with DFU, the expression of miR-15a-3p was found to be upregulated in foot skin compared to control subjects without the condition. miR-15a-3p appears to modulate the NOX5/ROS signaling pathway, suggesting a specific mechanistic pathway through which miR-15a-3p impacts diabetic wound healing (95).
There are multiple studies introducing the beneficial role of miR-221-3p in different phases of diabetic wound healing including angiogenesis. MiR-221-3p promotes angiogenesis by directly targeting homeodomain-interacting protein kinase 2 (HIPK2), utilizing both in vitro experiments with HUVECs and in diabetic mice skin tissues (96). Similarly, Hu et al. demonstrated that miR-221-3p enhances skin wound healing by mitigating the negative effects of HG on apoptosis and angiogenesis, specifically through targeting thrombospondin 1 (THBS1) (97). MiR-200b was observed to be upregulated in HUVECs treated with HG, and miR-200 inhibition was found to promote angiogenesis in HUVECs through the interaction with the neurogenic locus notch homolog protein 1 (Notch1) (98). The Wnt/β-catenin signaling pathway is critical for diabetic wound healing, regulating essential processes such as cell proliferation, migration, and differentiation of keratinocytes and fibroblasts, while also promoting the activity of ECs. However, in diabetic wounds, the significant decrease in β-catenin pathway activity leads to impaired wound closure and chronicity, largely due to reduced cytokine production and dysfunctional intracellular signaling that hinders the functionality of key cell types (57, 99). MiR-488-3p accelerates wound healing by activating the cytochrome P450 1B1 (CYP1B1)-mediated Wnt4/β-catenin through targeting MeCP2, thereby promoting the angiogenic response of HUVECs (52).
Blood glucose levels regulate the expression of PTEN, predominantly present in epithelial cells, and it triggers signaling pathways influencing angiogenesis (46). Xu et al. demonstrated that the levels of PTEN are downregulated in individuals with diabetes, and that suppression of miR-152-3p can elevate PTEN levels, thereby improving ECs functions critical to angiogenesis (100). Moreover, sEVs engineered with miR-17-5p, when encapsulated in GelMA Hydrogel, promote diabetic wound healing by stimulating angiogenesis and collagen buildup through PTEN and p21 pathways (101). MiR-17-5p belongs to the miR-17-92 cluster, which regulates ECs functions and modulates angiogenesis through various pathways. However, the roles of the miR-17-92 cluster are not always beneficial (102, 103). Another member of the miR-17-92 cluster, miR-17-3p, has been found to negatively control fetal liver kinase 1 (Flk-1) expression. The miR-17-3p/Flk-1 interaction has a detrimental effect on angiogenesis under diabetic conditions (104). Similarly, miR-92a, another member of the miR-17-92 cluster, has been associated with detrimental effects on angiogenesis. Inhibition of miR-92a has been found to have a beneficial effect on angiogenesis, thereby improving diabetic wound healing. Lucas et al. demonstrated that light-activated antimir-92a improves angiogenesis under diabetic conditions (105, 106). Furthermore, another study demonstrated that a synthetic miR-92a inhibitor (MRG-110) promotes angiogenesis and granulation tissue formation, thereby accelerating wound healing in both mice and pigs (106). These results suggest that the regulatory role of the miR-17-92 cluster is complex and varied, necessitating further research to fully understand their mechanisms in angiogenesis and harness their potential for therapeutic purposes.
The research by Wang and colleagues showed that miR-503 from M1 macrophage-released EVs inhibits insulin-like growth factor 1 receptor (IGF1R) expression in HUVECs, thereby impeding the wound healing process in individuals with diabetes (107). Elevated levels of miR-409-3p were noted in nonhealing skin wound samples from patients relative to uninjured skin tissues. Additionally, miR-409-3p was shown to impair the angiogenic capabilities of HUVECs under high glucose conditions, indicating that miR-409-3p exerts a detrimental effect on wound healing in hyperglycemic environments by altering the BTG2/mTOR signaling pathway (108).
3.3 Re-epithelialization and ECM remodeling
Re-epithelialization and ECM remodeling are key processes in the regenerative phase. Re-epithelialization, essential for restoring intact skin, is primarily facilitated by keratinocytes through their migration, proliferation and differentiation, thereby maintaining and repairing skin function (109). The formation of the ECM holds significant importance in diabetic wound healing (58). In healthy skin, fibroblasts migrate to the injury site to restructure the ECM and remodel dermal collagen. However, in diabetic skin, under glycemic conditions, these cells exhibit decreased proliferation and migration, leading to the impairment and fragmentation of collagen fibers (110).
MiR-155 has been found to be overexpressed in HaCaT cells under high glucose (HG) conditions and in the exosomes of M1 macrophages. Gondaliya et al. demonstrated that MSC-derived exosomes loaded with a miR-155 inhibitor promote wound healing in vitro with HaCaT cells and in vivo in diabetic mice, primarily by restoring FGF-7 levels, thus enhancing keratinocyte migration and re-epithelialization (111). FGF, including FGF-7, play a crucial role in accelerating the healing process of diabetic wounds. However, despite their potential, the clinical application of FGF in treating diabetic wounds is limited, highlighting the need for improved delivery methods and further research into their long-term effects (112). Relatedly, Moura et al. demonstrated that in hyperglycemic conditions, an miR-155 inhibitor promotes wound closure and re-epithelialization in vitro by facilitating scratch closure and upregulating FGF7 expression (113). In their experiments, Dallas et al. utilized an antisense oligonucleotide (antimiR) to inhibit miR-210, mitigating its negative impact on keratinocyte growth and proliferation and thus promoting re-epithelialization (114).
Multiple studies have identified the role of miR-145-5p in various stages of diabetic wound healing. Wang et al. showed that miR-145-5p adversely impacts HG-induced impairment in human foreskin fibroblasts by elevating PDGFD expression, consequently hindering wound healing (71). Conversely, Su et al. found that EVs from placental mesenchymal stem cells counter apoptosis and enhance the proliferation and migration in diabetic wounds by delivering miR-145-5p. MiR-145-5p targets CDKN1A directly, consequently upregulating the Erk/Akt cascade, and thus promoting diabetic wound healing (70). MiR-4645-5p, derived from human bone marrow stem cells (hyBMSCs), was discovered to promote autophagy in HaCaT cells by suppressing the MAPKAPK2-induced AKT-mTORC1, consequently aiding diabetic wound healing (115).
MMPs play a crucial role in the regulation of keratinocytes, and thereby in re-epithelialization and ECM remodeling (116). Li et al. discovered that inhibiting PPARG, targeted by miR-182-5p, results in reduced MMP1 expression and elevated fibronectin 1 (FN1) expression. This suggests that exosomes from human endothelial progenitor cells improve the proliferation, migration, and adhesion of HaCaT cells in HG conditions via the miR-182-5p/PPARG signaling pathway (117). Furthermore, another study by Wang and colleagues found that the upregulation of miR-129 and miR-335 inhibits MMP-9 expression by interacting with specificity protein 1 (Sp1) in keratinocytes and in diabetic rats, thereby promoting diabetic wound healing (50).
Different members of the miR-21 family have been found to positively affect the functions of keratinocytes and fibroblasts, thereby promoting diabetic wound healing. MiR-21-5p loaded in engineered exosomes enhances the proliferation and migration of HaCaT cells via the Wnt/β-catenin pathway in vitro and enhanced diabetic wound healing by promoting collagen remodeling, and vascularization in diabetic rats (118). Furthermore miR-21-5p was found to be involved in wound repair through Centella Asiatica and its compound Asiaticoside. The study by Liu et al. concludes that Asiaticoside-nitric enhances diabetic wound healing by modulating the miRNA-21-5p/TGF-β1/SMAD7/TIMP3 cascade (119). Wu et al. found that miR-21-3p plays a positive role in fibroblast function by downregulating sprouty homolog 1 (SPRY1) and accelerates wound healing (120). Neuro-oncological ventral antigen 1 (NOVA1) has been identified as a direct target of miR-27-3p in fibroblasts, and inhibiting miR-27-3p could promote diabetic wound healing by restoring fibroblast viability (121). We summarized the regulatory mechanism of several key miRNAs in diabetic wound healing (Table 1, Figure 2).
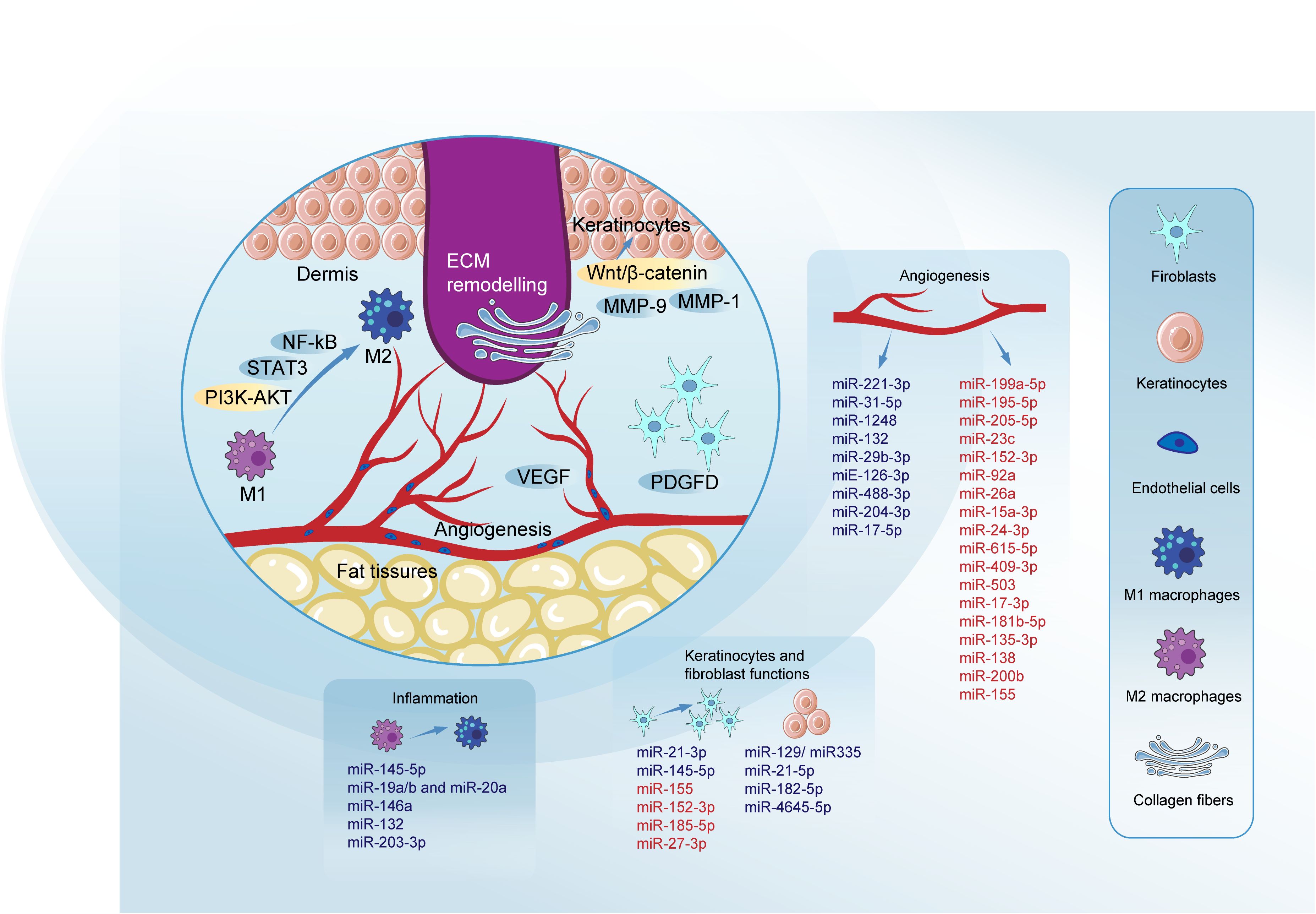
Figure 2. Roles of miRNAs in key phases of diabetic wound healing. MiRNAs modulate key pathways to promote wound healing. The inhibition of NF-κB and activation of the PI3K/AKT signaling pathway are critical, with signal transducer and activator of STAT3 playing a pivotal role in promoting M2 macrophage polarization through pathways such as DYRK1A/STAT3 and JAK2/STAT3. VEGF regulation is crucial, involving pathways like HIF1A/VEGF, VEGF-AKT/eNOS, and VEGF-induced activation of the p38MAPK signaling pathway, which facilitates blood vessel formation. The Wnt/β-catenin pathway is essential for the proliferation and migration of keratinocytes and fibroblasts, crucial for effective re-epithelialization of the wound. MMPs such as MMP-9 and MMP-1 play dual roles in diabetic wound healing. Their effects can be beneficial or detrimental depending on the healing phase. PDGFD is highlighted for its positive impact on fibroblast proliferation and migration, contributing to effective ECM remodeling. Blue denotes miRNAs promoting specific processes, while red indicates those impairing them. Micro RNAs (miRNAs); signal transducer and activator of transcription 3 (STAT3); phosphatidylinositol-3 kinase-AKT (PI3K-AKT); signal transducer and activator of transcription 3 (STAT3); nuclear factor-kappa B (NF-kB); matrix metalloproteinase-1 (MMP-1); matrix metalloproteinase-9 (MMP-9); platelet-derived growth factor D (PDGFD); extracellular matrix (ECM); vascular endothelial growth factor (VEGF); epidermal growth factor (EGF); fibroblast growth factor (FGF).
4 Long non-coding RNA
LncRNAs are sequences of RNA with a length exceeding 200 nucleotides and have many features in common with genes that encode mRNAs, yet they are incapable of synthesizing proteins (122, 123). LncRNAs exert a crucial regulatory influence on the processes of gene transcription and expression through a variety of molecular mechanisms, initially thought to act locally at their synthesis sites to influence nearby gene activity. These transcripts are involved in numerous mechanisms, such as protein synthesis, RNA maturation, stability, and transport, as well as activating or silencing transcriptional genes by modifying chromatin structure (124, 125). Furthermore, lncRNAs exert their influence on gene expression through mRNA splicing, translation, transcription, and genomic imprinting, showcasing their extensive regulatory capacity (125, 126). The link between lncRNAs and diseases, particularly chronic conditions like diabetes, cancer, and cardiovascular diseases, is clear and well-defined (127–130).
4.1 Inflammation
During the inflammatory stage, lncRNAs can serve a regulatory function by various pathways, including the M1 to M2 macrophage polarization (131). LncRNA H19 is the most extensively studied among all lncRNAs. It has been discovered by various studies that lncRNA H19 is significantly decreased in diabetic wounds compared to normal ones (132). Li and colleagues showed that exosomal lncRNA H19 targets miR-130b-3p, regulating PPARγ/STAT3 to promote M2 macrophage polarization. This process aids in promoting fibroblast proliferation and migration, and ECs angiogenesis, thus accelerating the wound healing process (133).
Hu et al. first detected that lncRNA GAS5 triggers the activation of the STAT1 in macrophages, and STAT1 is necessary for the expression of M1 marker genes. Furthermore, they demonstrated that the silencing of lncRNA GAS5 promotes diabetic wound healing. Taken together, their study suggests that a reduction in the levels of lncRNA GAS5 will promote impaired diabetic wound healing by facilitating M2 macrophage polarization (134).
Kuang et al. showed that miR-1914-3p targets milk fat globule-EGF factor 8 protein (MFGE8), influencing macrophage polarization through the TGFB1/SMAD3. Concurrently, miR-1914-3p is targeted by MALAT1, which binds miR-1914-3p competitively to suppress the TGFB1/SMAD3 pathway’s activity. Further studies revealed that KCs-Exo carrying MALAT1 modulates macrophage activities through the TGFB1/SMAD3 and enhances diabetic wound healing in mice (135). LncRNA Lethe has been identified to be involved in the control of ROS generation in macrophages by adjusting NADPH oxidase 2 (NOX2) gene expression through NF-κB signaling (136).
4.2 Angiogenesis
Multiple research findings have highlighted functional links between lncRNAs and the process of angiogenesis. Some nanomaterial delivery systems have played important advantages in drug delivery and therapy (137, 138). Tao et al. explored a new nano-drug delivery system targeting lncRNA using high-yield extracellular vesicle-mimetic nanovesicles (EMNVs), focusing on the effects of EMNVs enriched with lncRNA-H19 (H19EMNVs). Their findings revealed that H19EMNVs enhanced ECs functions, which were previously hindered by HG levels (132). LncRNA HOTAIR, carried by MSC-derived EVs, was observed to enhance angiogenesis in HUVECs in vitro through VEGF upregulation. Additionally, it facilitated the vascularization of the wound bed and improves wound healing in diabetic mice (139).
Under diabetic conditions HIF-1 enhances the expression of VEGF, indicating that the regulation of the HIF-1/VEGF pathway is crucial for diabetic wound healing. Additionally, multiple lncRNAs have been found to exert regulatory effects on this pathway (122). LncRNA GAS5 promotes diabetic wound healing by interacting with TATA-binding protein-associated factor 15 (TAF15) and triggering the HIF1A/VEGF in diabetic mice, while also enhancing the proliferation of HG-induced HUVECs in vitro (140). LncRNA ANRIL was found to be downregulated in DFU. Furthermore, ANRIL overexpression was shown to rescue the HG-impaired function of endothelial progenitor cells (EPCs) by regulating HIF1A mRNA stability through interaction with FUS. It was also discovered that alterations in ANRIL or HIF1A regulate VEGFA expression both in EPCs and in diabetic mice (141). The study by Han and colleagues revealed that lncRNA KLF3-AS1 enhances cell growth and tube formation, while simultaneously suppressing apoptosis in HUVECs under HG conditions, through the reduction in the expression levels of microRNA-383. Additionally, their research identified VEGFA as a direct target of miR-383. Moreover, KLF3-AS1 was found to significantly promote cutaneous wound healing in a diabetic mouse (142). Fu and colleagues explored how keratinocyte-derived exosomal lncRNA LINC01435 influences diabetic wound healing by affecting vascular ECs. Their research indicates that LINC01435 impedes the migration and tube formation capabilities of HUVECs, thereby restraining angiogenesis via the LINC01435/YY1/HDAC8 signaling pathway (143). Elevated levels of lncRNA DLEU1 were detected in the serum of patients with DFUs. Subsequent investigations revealed that miR-96-5p acted as a mediator in the detrimental effects of lncRNA DLEU1 on angiogenesis. Collectively, the findings indicated that lncRNA DLEU1 exerts its negative impact on HUVECs by suppressing cell proliferation, enhancing apoptosis, and increasing oxidative stress through its interaction with miR-96-5p (144).
4.3 Re-epithelialization and ECM formation
LncRNAs are involved in re-epithelialization and ECM remodeling, exerting regulatory control over the activities of keratinocytes and fibroblasts. Specifically, lncRNA-H19 has been demonstrated to enhance the proliferation and migration of HaCaT cells, which were pre-treated with 30 mM glucose for 24 hours, and to inhibit pyroptosis by inhibiting NOD-like receptor family pyrin domain-containing 3 (NLRP3) activation (145). Another study found that lncRNA-H19, particularly enriched in PDGFRα+ dermal fibroblasts, promotes fibroblast proliferation through the modulation of the p53-controlled cell cycle and modulates immune cell infiltration by reducing fibroblast-derived growth differentiation factor 15 (GDF15). Additionally, it was discovered that exosomes from Adipocyte Progenitor Cells (APCs) transport lncRNA-H19 to the injury sites in type 2 diabetes mice, thereby enhancing wound healing that was previously impaired (146). Li et al. found that lncRNA H19 recruits SRF to upregulate connective tissue growth factor (CTGF) levels, which enhances wound healing by promoting fibroblast proliferation and ECM remodeling, thereby accelerating wound healing in diabetic rats through the lncRNA H19/SRF/CTGF pathway (147). Silencing lncRNA H19 inhibits the functions of fibroblasts, with reduced fibrillin-1 (FBN1) but upregulates miR-29b. This led to poor expression of transforming growth factor-beta 1 (TGF-β1), Smad3, fibronectin (FN), and Col-1, and reduced ECM accumulation (148). Qian and colleagues showed that lncRNA-H19 sponges miR-19b by targeting SRY-related high-mobility-group box 9 (SOX9). This activation of SOX9 initiates the Wnt/β-catenin pathway, resulting in enhanced proliferation, migration, and invasion of human skin fibroblasts, thus facilitating wound healing (149). Guo et al. demonstrated that lncRNA H19 could recruit enhancer of zeste homolog 2 (EZH2) mediated histone methylation and regulate HIF-1α to enhance fibroblast activation, leading to the improvement of diabetic wound healing (150). Apart from the beneficial properties of lncRNA-H19, research conducted by Ji and colleagues discovered that reducing H19 levels could promote HaCaT cell proliferation and migration. This effect occurs as H19 competitively interacts with miR-17-5p, leading to an increase in RUNX1 expression (151).
It was detected that lncRNA CASC2 is downregulated in wound tissues of DFU patients. In vitro, lncRNA CASC2 facilitates wound healing by enhancing fibroblast migration, and in vivo, it accelerates wound healing in DFU mice. Furthermore, He et al. demonstrated that lncRNA CASC2 facilitates wound healing in DFU by promoting fibroblast migration and inhibiting apoptosis through the miR-155/HIF-1α pathway (152).
In recent studies, the functions of MALAT1 in regulating the epithelial-mesenchymal transition (EMT) of HaCaT cells have been elucidated. Zhang et al. demonstrated that the suppression of MALAT1 significantly decreases EMT induced by TGF-β1 (153). Additionally, they found that MALAT1 was involved in hyperglycemia-induced EMT in human HaCaT cells by interacting with miR-205 and promoting the levels of Zinc Finger E-box Binding Homeobox 1 (ZEB1) (154). The results indicate that MALAT1 could be a target for diseases characterized by aberrant EMT. Moreover, Shi et al. proposed using MALAT1 as a sponge for miR-142 to indirectly upregulate Sirt1 and nuclear factor erythroid 2-related factor 2 (Nrf2) for treating DFUs in elderly individuals (155). MALAT1 also enhances the activities of keratinocytes by functioning as a competing endogenous RNA (ceRNA), which involves competitive interactions with miR-106a-5p. Furthermore, zinc finger protein 148 (ZNF148)-activated MALAT1 upregulates ZNF148 levels through its competitive interaction with miR-106a-3p, creating a positive feedback loop that further boosts keratinocyte activity (156). Hong et al. demonstrated that the downregulation of lncRNA XIST levels suppresses the proliferation and migration of HG-induced HaCaT cells through the miR-126-3p/EGFR pathway (157).
A significant elevation in the expression of circulating lncRNA NEAT1 was observed in individuals with T2DM, exhibiting a 5.28-fold increase compared to healthy subjects (158). LncRNA NEAT1 plays a crucial regulatory role in various diabetes-related complications, including diabetic retinopathy, diabetic nephropathy, and impaired wound healing associated with diabetes. The study by Yang et al. revealed that NEAT1 can modulate SOX4, thereby influencing the EMT in diabetic retinopathy through its interaction with miR-204. This finding offers a novel perspective and potentially identifies a valuable therapeutic target for addressing diabetic retinopathy (159). Furthermore, the study by Wang et al. demonstrates that lncRNA NEAT1 plays a significant role in the pathogenesis of diabetic nephropathy by modulating extracellular matrix proteins and EMT through the miR-27b-3p/ZEB1 axis (160). Complementary research has demonstrated the capacity of NEAT1 to promote diabetic nephropathy progression via activation of the Akt/mTOR signaling pathway. Considering these findings in conjunction with our results, NEAT1 holds significant potential as both a diagnostic biomarker and a therapeutic target in the management of diabetic nephropathy (161). The key lncRNAs in diabetic wound healing are systematically summarized (Table 2, Figure 3).
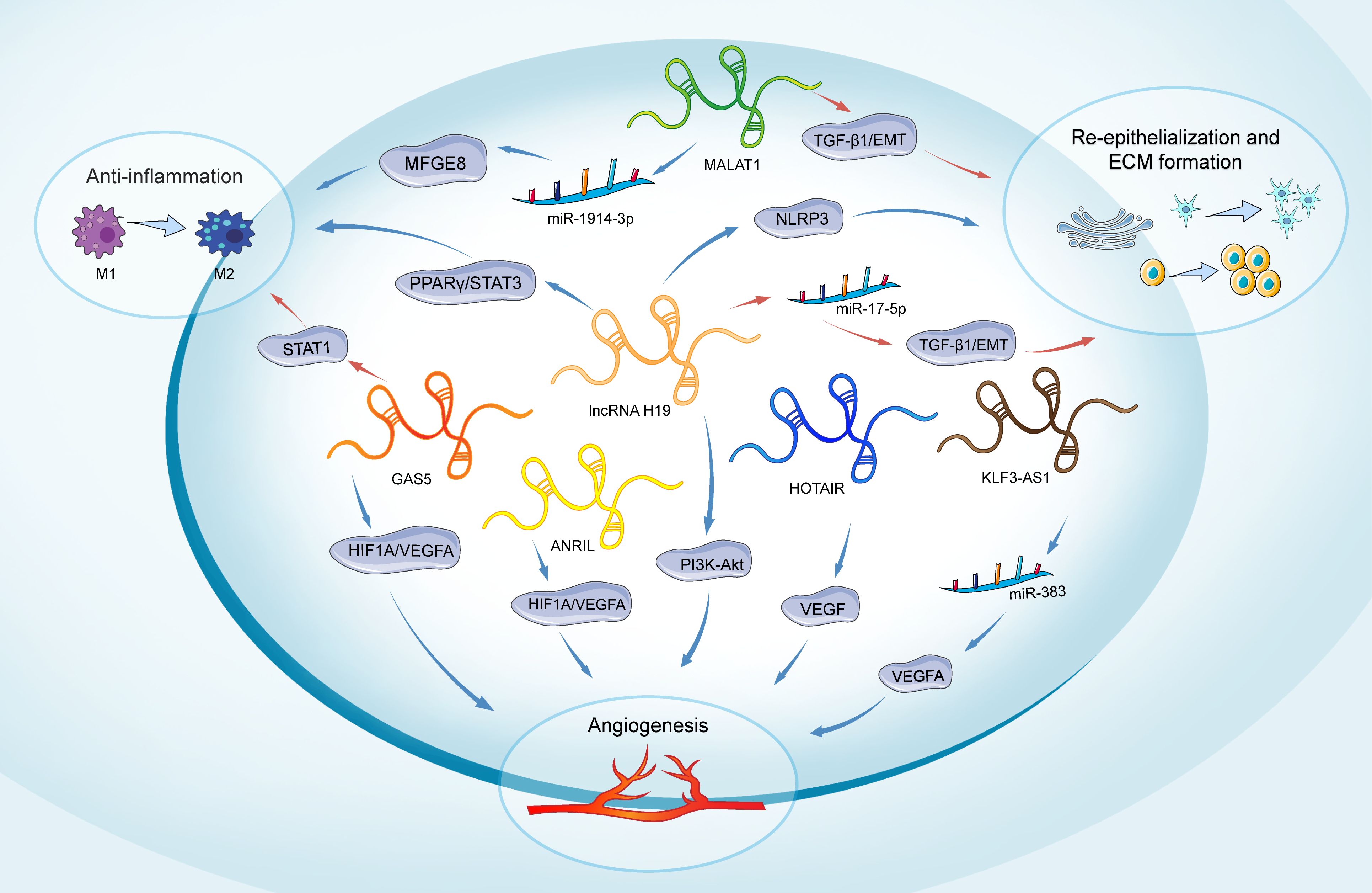
Figure 3. Role of lncRNAs in main phases of diabetic wound healing. The lncRNAs depicted include H19, MALAT1, GAS5, HOTAIR, ANRIL, and KLF3-AS1. H19 participates in all phases of wound healing. It promotes inflammation through the regulation of the PPARγ/STAT3 pathway. In angiogenesis, H19 regulates PI3K-Akt signaling. H19 enhances the proliferation and migration of keratinocytes and inhibits pyroptosis by suppressing the activation of NLRP3. Conversely, H19 can also inhibit keratinocyte proliferation and migration by targeting miR-17-5p and upregulating RUNX1. MALAT1 promotes an anti-inflammatory response by targeting miR-1914-3p and activating MFGE8. Additionally, MALAT1 regulates the EMT in keratinocytes through the regulation of TGFβ1-induced EMT. GAS5 induces STAT1 to promote M1 macrophage activation, while its reduction favors M2 macrophage polarization and benefits wound healing. It also promotes angiogenesis by activating the HIF1A/VEGFA pathway. HOTAIR, ANRIL, and KLF3-AS1 are primarily involved in promoting angiogenesis: HOTAIR activates VEGF to enhance angiogenesis, ANRIL modulates the HIF1A/VEGFA pathway, and KLF3-AS1 promotes angiogenesis by targeting miR-383 and upregulating VEGFA expression. Long non-coding RNAs (lncRNAs); extracellular matrix (ECM); milk fat globule-EGF factor 8 protein (MFGE8); peroxisome proliferator-activated receptor gamma/Signal transducer and activator of transcription 3 (PPARY/STAT3); Signal transducer and activator of transcription 1 (STAT1); hypoxia-inducible factor 1-alpha/Vascular endothelial growth factor A (HIF1A/VEGFA); Phosphatidylinositol-3 Kinase-AKT (PI3K-Akt); Vascular endothelial growth factor (VEGF); Transforming growth factor beta 1/Epithelial-mesenchymal transition (TGF-β1/EMT); NOD-like receptor family pyrin domain-containing 3 (NLRP3).
5 Circular RNAs
CircRNAs, identified as key regulators of cell proliferation, metabolism, apoptosis, and inflammation, present as closed loops without poly A tails (162), establishing their significant involvement in diseases such as diabetes, cardiovascular disease, neurological disorders, and autoimmune disease (163–165). The primary roles of circRNA include serving as transcriptional regulators, functioning as miRNA sponges, acting as biomarkers for clinical diagnosis and treatment, and interacting with RNA-binding proteins (166–168).
Circ-Snhg11 has been identified to have regulatory effects at various stages of diabetic wound healing. Tang et al. demonstrated that circ-Snhg11 in BMSC-Exos improves angiogenesis and promotes diabetic wound healing by targeting miR-144-3p and enhancing SLC7A11/GPX4-mediated anti-ferroptosis signals (169). Furthermore, it was found that increasing levels of circ-Snhg11 counteracts the impaired function of EPCs and enhances M2 macrophage polarization under HG conditions. The study suggests that the upregulation of circ-Snhg11 inhibits ECs damage and promotes M2 macrophage polarization through the miR-144–3p/HIF-1α/VEGF pathway (170). Yin et al. demonstrated that circRps5, carried by ADSCs, promotes diabetic wound healing in diabetic mice by modulating macrophage polarization. Furthermore, miR-124-3p has been identified as a downstream target of circRps5. Thus, circRps5 delivered by ADSC-derived exosomes enhances M2 polarization, thereby facilitating diabetic wound healing through the regulation of miR-124-3p (171).
EPCs play a vital role in diabetic wound healing by proliferating and differentiating to form new blood vessels at sites of injury. However, a high glucose environment leads to EPC dysfunction, and multiple studies have found that circRNAs impact the regulation of EPC functions (172). The study by Shang et al. found that hypoxic pretreatment of EPCs promotes their survival and enhances diabetic wound healing by increasing autophagy through the overexpression of circ-Klhl8. MiR-212-3p was identified as the target of circ-Klhl8, and the downregulation of miR-212-3p promotes SIRT5 expression, thereby facilitating diabetic wound healing (173). In vitro, experiments have shown that exosomes derived from circ-Astn1-modified ADSCs play crucial roles in restoring the function of EPCs by reducing apoptosis under HG conditions. Furthermore, Wang et al. demonstrate that circ-Astn1 in ADSC-derived exosomes promotes diabetic wound healing by targeting miR-138-5p and upregulating SIRT1, which in turn decreases FOXO1 expression (174). mmu_circ_0000250 was also found to regulate EPC functions. Exosomes derived from mmu_circ_0000250-modified ADSCs plays a crucial role in restoring EPC function by activating autophagy, targeting miR-128-3p, and increasing SIRT1 levels (175). Tang et al. demonstrated that both exosomes from ADSCs and those from hypoxic pretreated ADSCs promote wound healing in diabetic mice, however, the exosomes from hypoxic pretreated ADSCs exhibit a greater therapeutic effect, with circ-Erbb2ip playing a significant role in this process. Bioinformatics analyses indicated that miR-670-5p is a target of circ-Erbb2ip, and further revealed that Nrf1 is a downstream target of miR-670-5p. These findings suggest that exosomes derived from hypoxic pretreated ADSCs enhance diabetic wound healing by delivering circ-Erbb2ip, which activates the miR-670-5p/Nrf1 signaling pathway. This pathway, in turn, reduces ROS levels and inflammatory cytokine expression while restoring the angiogenic function of EPCs (176).
Multiple studies have introduced the roles of circRNAs in the regulation of keratinocyte functions. Levels of circRNA-080968 were observed to be considerably elevated in DFUs samples relative to skin from diabetic patients without DFUs. Additionally, it was discovered that circRNA-080968 inversely affects the expressions of miR-326 and miR-766-3p by facilitating their breakdown, which in turn suppresses keratinocyte migration and promotes cell proliferation in DFUs (177). The levels of Circ_PRKDC at the wound edge were found to decrease significantly both 1 day and 7 days after the injury occurred. In vitro studies indicated that circ_PRKDC inhibits human epidermal keratinocyte (HEKs) migration by targeting and reducing miR-31 levels in keratinocytes, with FBN1 being a target of miR-31. Considering the role of FBN1 in promoting apoptosis and inhibiting fibroblast proliferation, it contributes to slower healing in DFU. Han et al. concluded that circ_PRKDC inhibition enhances wound healing by stimulating keratinocyte migration through the miR-31/FBN1 pathway (178). It was initially discovered that hsa_circ_0084443 expression was elevated in DFU, compared to normal wounds, and that this circRNA inhibits keratinocyte migration but promotes their proliferation through various gene regulatory networks (179). Huang et al. demonstrated that circCDK13 is a promising therapeutic agent for diabetic wound healing. They found that circCDK13 cooperates with IGF2BP3, and together they synergistically promote the proliferation and migration of human dermal fibroblasts and HEKs by enhancing the expression of c-MYC and CD44. Furthermore, to maximize the potential application of circCDK13 in wound healing and tissue regeneration, a delivery method was established. CircCDK13 loaded into small EVs promotes the proliferation and migration of human dermal fibroblasts and HEKs in vitro and accelerates diabetic wound healing in db/db diabetic mice (180).
Meng et al. demonstrated that by competitively binding hsa-miR-1273h-5p, hsa_circ_0008500 effectively neutralizes its ability to suppress ETS transcription factor ELK1 (ELK1) expression, playing a crucial role in inhibiting the apoptosis of ADSCs (181). Another study by Meng et al. investigated that circARHGAP12 promotes autophagy against MSC apoptosis under HG conditions by targeting miR-301b-3p and boosting the levels of autophagy-related 16-like 1 (ATG16L1) and unc-51 like autophagy activating kinase 2 (ULK2), thereby facilitating diabetic wound healing (182). Chen et al. demonstrated that exosomes overexpressing circ-ITCH promote wound healing in DFUs mice by alleviating ferroptosis and promoting angiogenesis in HG-treated HUVECs by recruiting TAF15 and activating the Nrf2 (183). Exosomal circHIPK3 has been identified as advantageous for healing diabetic wounds by enhancing angiogenesis through the miR-20b-5p/Nrf2/VEGFA pathway (184).
The study by Yang et al. initially discovered that delivering circ-Amotl1 enhances wound healing in mice. Furthermore, circ-Amotl1 was found to enhance wound healing by facilitating cell proliferation, survival, and migration through the enhanced nuclear translocation of Stat3. Stat3 then binds to the DNA methyltransferase 3A (Dnmt3a) promoter, reducing miR-17-5p expression but upregulating fibronectin levels. The decreased levels of miR-17-5p enhanced the expression of various proteins, which facilitates cell proliferation and migration, thereby accelerating wound repair (185). Table 3 below highlights key circRNAs involved in diabetic wound healing.
6 Limitations and perspectives
This article has reviewed the roles of different classes of ncRNAs, including miRNAs, lncRNAs, and circRNAs, in diabetic wound healing. The detailed examination of these ncRNAs highlights their impact on the regulation of critical processes such as inflammation, angiogenesis, re-epithelialization, and extracellular matrix remodeling, which are important for effective wound repair, particularly in diabetes conditions, where the healing process is widely slow and often incomplete. While most current research studies have investigated the potential mechanisms by which ncRNAs influence diabetic wound healing, several limitations in these studies necessitate further investigation.
Current research mostly focuses on miRNAs, with relatively less attention given to circRNAs, lncRNAs, and other types of ncRNAs. This imbalance might limit our understanding of unique biological functions that these lesser studied ncRNAs could have in wound healing for diagnosis and clinical applications.
Similarly, while much of the existing research has concentrated on fibroblasts, keratinocytes, and ECs, there is a need for more comprehensive studies on the role of immune cells. Immune cells like lymphocytes, dendritic cells, and others significantly influence healing outcomes by being involved in almost all key stages of wound healing and it is important to coordinate the activities of immune cells accurately (186). The imbalance of immune cells can result in the degradation of the immune microenvironment (187). A detailed exploration of ncRNAs’ roles in these immune cells could unveil new mechanisms of immune regulation in diabetic wounds.
Another gap in current studies is the emphasis on the final effect without exploring their specific roles in each phase of wound healing. This gap leaves an incomplete understanding of the temporal dynamics of ncRNAs action during the critical stages of inflammation, proliferation, and remodeling. Conducting phase-specific research could help identify new therapies that target these specific phases, leading to optimized healing processes.
Finally, there is a lack of studies examining the complex interactions among ECs, fibroblasts, keratinocytes, and immune cells in the context of ncRNAs mediation. Understanding how ncRNAs facilitate or impair cell-cell communication and coordination in wound environments could offer valuable insights into the mechanisms of wound healing. Despite these limitations, the research into ncRNAs offers considerable potential for improving the treatment of DFUs. Here we outline several future perspectives that not only address these challenges but also lay the foundation for major advancements in this field.
The application of ncRNAs as biomarkers for diabetic wound healing offers a promising frontier for early diagnosis and prognosis. Prospective studies designed to validate specific ncRNAs as reliable biomarkers could lead to the development of predictive tools for wound healing success or failure. Among ncRNAs, circRNAs are particularly promising due to their unique closed-loop structure, which, unlike linear ncRNAs, provides enhanced stability and resistance to exonucleases (188). Future studies should not only validate ncRNAs in retrospective settings but also prospective analyses. This approach could accelerate the research process, enabling quicker integration of ncRNA biomarkers into clinical practice, thereby enhancing diagnostic accuracy and patient management strategies.
Beyond their potential as biomarkers, ncRNAs also hold promise as therapeutic agents in diabetic wound healing. While current treatments for diabetic wound healing exist, ncRNA-based therapies hold promise for more precisely targeted intervention strategies. By selectively regulating gene expression, ncRNAs can potentially accelerate wound repair and reduce the risk of complications, offering an innovative approach to diabetic wound care.
Recent advancements in nanomedicine have greatly improved the stability, targeting precision, and therapeutic efficacy of exogenous ncRNAs in wound healing. Nanoparticle-based delivery systems, such as lipid nanoparticles and polymer-based carriers, protect ncRNAs from enzymatic degradation, enhancing their stability, bioavailability, and longevity in the bloodstream (189). Functionalized nanoparticles with specific ligands improve targeting efficiency by directing ncRNAs to wound-specific cells like fibroblasts and keratinocytes, thereby enhancing therapeutic outcomes. Additionally, engineered nanoparticles provide controlled, sustained release of ncRNAs, enabling precise regulation of molecular pathways involved in inflammation, angiogenesis, and tissue repair (190). Addressing off-target effects remains a critical consideration in the development of effective miRNA-based therapies, and these recent advances not only accelerate wound healing but also address challenges like off-target effects and long-term safety concerns. Ongoing research is focused on improving nanoparticle biocompatibility and developing condition-responsive systems for more precise ncRNA delivery in clinical applications (191).
While delivering ncRNAs through various pathways can lead to both upregulation and downregulation of ncRNA expression in diabetic wounds, the regulation of endogenous ncRNAs is highly complex and influenced by various metabolic abnormalities. Hyperglycemia in diabetic patients causes an overproduction of ROS, leading to oxidative stress (19, 192). This condition significantly impacts the expression of ncRNAs, particularly those involved in inflammatory responses and tissue repair. For example, in diabetic wounds, hyperglycemia-induced oxidative stress triggers an unregulated and prolonged unfolded protein response (UPR), resulting in a deficiency of inositol-requiring enzyme 1 (IRE1α), which is a key transducer of the UPR that regulates the expression of mRNAs and miRNAs. This deficiency causes an increase in the levels of the miR-200 family and miR-466 (193). Furthermore, inflammatory cytokines such as TNF-α and IL-6 also influence ncRNA expression, further complicating the regulatory processes (194). In addition to metabolic factors, infections are another significant factor that can regulate the expression of endogenous ncRNAs. The presence of pathogens can induce inflammatory responses, leading to alterations in ncRNA expression patterns (195, 196).
However, to turn this potential into reality, more clinical trials are needed. The limited number of clinical trials testing ncRNAs therapies underscores a significant gap in extensive clinical investigation. To advance the field, future research should focus on translating preclinical findings into clinical trials that evaluate the therapeutic efficacy and safety of ncRNA-based interventions. These trials should be comprehensive, involving multi-center collaborations to ensure a diverse patient cohort, with long-term follow-ups to assess both the safety and effectiveness of these therapies. By addressing these aspects, clinical trials can bridge the gap between experimental research and real-world medical applications.
7 Conclusion
The significance of ncRNAs in the diabetic wound healing process cannot be overstated. Through our comprehensive review, we have underscored the importance of miRNAs, lncRNAs, and circRNAs in diabetic wound healing. NcRNAs serve as crucial regulators, modulating critical aspects, such as inflammation, angiogenesis, re-epithelialization, and ECM remodeling. Our analysis highlights the impact of ncRNAs on the activities of fibroblasts, ECs, and keratinocytes, which are central players in wound healing through regulating protein interactions and signaling pathways.
The integration of ncRNAs into clinical practice holds immense promise, leveraging their diagnostic and therapeutic potential. The diagnostic potential of ncRNAs as biomarkers for diabetic wounds and related conditions offers early detection and personalized treatment options. Furthermore, exploring the therapeutic potential by silencing or activating ncRNAs could lead to novel interventions that enhance diabetic wound healing outcomes.
Author contributions
SA: Writing – review & editing, Writing – original draft. JZ: Writing – review & editing, Writing – original draft. JY: Writing – review & editing, Writing – original draft. RT: Writing – review & editing, Writing – original draft. SL: Writing – review & editing, Writing – original draft. SZ: Writing – review & editing, Writing – original draft. YZ: Writing – review & editing, Writing – original draft. ZZ: Writing – review & editing, Writing – original draft.
Funding
The author(s) declare financial support was received for the research, authorship, and/or publication of this article. This work was financially supported by Natural Science Foundation of Hubei Province (2022CFB298), Innovation Fund for Industry-University-Research of Chinese Universities (2021ITA03006), Wuhan University Education and Development Foundation(NO.2002330).
Conflict of interest
The authors declare that the research was conducted in the absence of any commercial or financial relationships that could be construed as a potential conflict of interest.
Publisher’s note
All claims expressed in this article are solely those of the authors and do not necessarily represent those of their affiliated organizations, or those of the publisher, the editors and the reviewers. Any product that may be evaluated in this article, or claim that may be made by its manufacturer, is not guaranteed or endorsed by the publisher.
Glossary
(ECM): Extracellular matrix
(FGF): fibroblast growth factors
(ROS): reactive oxygen species
(ECs): endothelial cells
(VEGF): vascular endothelial growth factor
(VEGFA): Vascular Endothelial Growth Factor A
(DFUs): diabetic foot ulcers
(IDF): International Diabetes Federation
(ncRNAs): non-coding RNAs
(miRNAs): including microRNAs
(lncRNAs): long non-coding RNAs
(circRNAs): circular RNAs
(piRNAs): piwi-interacting RNAs
(snoRNAs): small nucleolar RNAs
(3’-UTR): three prime untranslated regions
(mRNAs): messenger RNAs
(DM): diabetes mellitus
(T2DM): Type 2 diabetes mellitus
(NETs): neutrophil extracellular traps
(HUVECs): Human Umbilical Vein endothelial cells
(GDF6): targeting growth differentiation factor 6
(NF-κB): nuclear factor-kappa B
(IRAK1): interleukin-1 receptor-associated kinase 1
(CNP): cerium oxide nanoparticles
(SFP): silk fibroin patch
(TNF-α): Tumor Necrosis Factor-Alpha
(IL-1β): Interleukin-1 beta
(IL-6): Interleukin-6
(TRAF6): tumor necrosis factor receptor-associated factor 6
(PAK7): p21-Activated Kinase 7
(EVs): extracellular vesicles
(CDKN1A): cyclin-dependent kinase inhibitor 1A
(PDGFD): platelet-derived growth factor D
(ICAM-1): intercellular adhesion molecule 1
(LPS): lipopolysaccharide
(PTEN): phosphatase and tensin homolog
(PDCD4): programmed cell death 4
(DYRK1A): dual-specificity tyrosine-phosphorylation-regulated kinase 1A
(hTERT): human telomerase reverse transcriptase
(IκB): inhibitor of NF-kappa-B
(SOCS3): suppressor of cytokine signaling 3
(EGF): epidermal growth factor
(HIP1): huntingtin interacting protein 1
(IGF2): insulin-like growth factor 2
(RASSF2): Ras association domain-containing protein 2
(ROCK1): Rho-associated protein kinase 1
(CITED2): Cbp/p300-interacting transactivator 2
(HIF-1): hypoxia-inducible factor 1
(HIPK2): homeodomain-interacting protein kinase 2
(THBS1): thrombospondin 1
(NOTCH1): neurogenic locus notch homolog protein 1
(HIF1AN): hypoxia-inducible factor 1-alpha inhibitor
(STARD13): StAR-related lipid transfer protein 13
(SPARC): secreted protein acidic and rich in cysteine
(SDF-1α): stromal cell-derived factor 1-alpha
(PIK3R3): phosphoinositide-3-kinase regulatory subunit 3
(CYP1B1): cytochrome P450 1B1
(MeCP2): methyl-CpG-binding protein 2
(STZ): streptozotocin
(db/db): diabetic mice
(Flk-1): fetal liver kinase 1
(IGF1R): insulin-like growth factor 1 receptor
(NFIC): nuclear factor I/C
(MAPKAPK2): mitogen-activated protein kinase-activated protein kinase 2
(MMP1): matrix metalloproteinase-1
(FN1): fibronectin 1
(SPRY1): sprouty homolog 1
(NOVA1): neuro-oncological ventral antigen 1
(MMP-9): matrix metalloproteinase-9
(Sp1): specificity protein 1
(ADSC): adipose-derived stem cells
(HG): high glucose
(MFGE8): milk fat globule-EGF factor 8 protein
(NOX2): NADPH oxidase 2
(EMNVs): extracellular vesicle-mimetic nanovesicles
(Es): endothelial progenitor cells
(TAF15): TATA-binding protein-associated factor 15
(NLRP3): NOD-like receptor family pyrin domain-containing 3
(GDF15): growth differentiation factor 15
(APCs): antigen-presenting cells
(CTGF): connective tissue growth factor
(FBN1): fibrillin-
(Smad3): Mothers against decapentaplegic homolog 3
(TGF-β1): transforming growth factor beta 1
(FN): fibronectin
(SOX9): SRY-related high-mobility-group box 9
(EZH2): enhancer of zeste homolog 2
(c-Myc): transcription factor
(EMT): epithelial-mesenchymal transition
(ZEB1): Zinc Finger E-box Binding Homeobox 1
(Nrf2): nuclear factor erythroid 2-related factor 2
(ZNF148): zinc finger protein 148
(ELK1): ETS transcription factor ELK1
(FBN): fibrillin
(ELK1): ETS transcription factor ELK1
(ATG16L1): autophagy-related 16-like 1
(ULK2): unc-51 like autophagy activating kinase 2
(Dnmt3a): DNA methyltransferase 3A
(HEKs): human epidermal keratinocyte
(UPR): unfolded protein response
(IRE1α): inositol-requiring enzyme 1
References
1. Alghamdi MA. Metal-organic frameworks for diabetic wound healing. Cureus. (2023) 15:e39557. doi: 10.7759/cureus.39557
2. Baltzis D, Eleftheriadou I, Veves A. Pathogenesis and treatment of impaired wound healing in diabetes mellitus: new insights. Adv Ther. (2014) 31:817–36. doi: 10.1007/s12325-014-0140-x
3. Sangha MS, Deroide F, Meys R. Wound healing, scarring and management. Clin Exp Dermatol. (2024) 49:325–36. doi: 10.1093/ced/llad410
4. Wilkinson HN, Hardman MJ. Wound healing: cellular mechanisms and pathological outcomes. Open Biol. (2020) 10:200223. doi: 10.1098/rsob.200223
5. Sorg H, Sorg CGG. Skin wound healing: of players, patterns, and processes. Eur Surg Res. (2023) 64:141–57. doi: 10.1159/000528271
6. Deng H, Li B, Shen Q, Zhang C, Kuang L, Chen R, et al. Mechanisms of diabetic foot ulceration: A review. J Diabetes. (2023) 15:299–312. doi: 10.1111/1753-0407.13372
7. Yang C, Zhang Z, Gan L, Zhang L, Yang L, Wu P. Application of biomedical microspheres in wound healing. Int J Mol Sci. (2023) 24:7319. doi: 10.3390/ijms24087319
8. Holl J, Kowalewski C, Zimek Z, Fiedor P, Kaminski A, Oldak T, et al. Chronic diabetic wounds and their treatment with skin substitutes. Cells. (2021) 10:655. doi: 10.3390/cells10030655
9. Joorabloo A, Liu T. Engineering exosome-based biomimetic nanovehicles for wound healing. J Control Release. (2023) 356:463–80. doi: 10.1016/j.jconrel.2023.03.013
10. Wen Q, Liu D, Wang X, Zhang Y, Fang S, Qiu X, et al. A systematic review of ozone therapy for treating chronically refractory wounds and ulcers. Int Wound J. (2022) 19:853–70. doi: 10.1111/iwj.13687
11. Lei M, Guo X, Yao Y, Shu T, Ren Z, Yang X, et al. Trelagliptin relieved cognitive impairment of diabetes mellitus rats: Involvement of PI3K/Akt/GSK-3β and inflammation pathway. Exp Gerontol. (2023) 182:112307. doi: 10.1016/j.exger.2023.112307
12. Da Silva J, Leal EC, Carvalho E, Silva EA. Innovative functional biomaterials as therapeutic wound dressings for chronic diabetic foot ulcers. Int J Mol Sci. (2023) 24:9900. doi: 10.3390/ijms24129900
13. Cai Y, Chen K, Liu C, Qu X. Harnessing strategies for enhancing diabetic wound healing from the perspective of spatial inflammation patterns. Bioact Mater. (2023) 28:243–54. doi: 10.1016/j.bioactmat.2023.04.019
14. Chen Y, Wang X, Tao S, Wang Q, Ma P-Q, Li Z-B, et al. Research advances in smart responsive-hydrogel dressings with potential clinical diabetic wound healing properties. Mil Med Res. (2023) 10:37. doi: 10.1186/s40779-023-00473-9
15. Gao M, Nguyen TT, Suckow MA, Wolter WR, Gooyit M, Mobashery S, et al. Acceleration of diabetic wound healing using a novel protease-anti-protease combination therapy. Proc Natl Acad Sci U S A. (2015) 112:15226–31. doi: 10.1073/pnas.1517847112
16. Huang C, Yuan W, Chen J, Wu L-P, You T. Construction of smart biomaterials for promoting diabetic wound healing. Molecules. (2023) 28:1110. doi: 10.3390/molecules28031110
17. Burgess JL, Wyant WA, Abdo Abujamra B, Kirsner RS, Jozic I. Diabetic wound-healing science. Medicina (Kaunas). (2021) 57:1072. doi: 10.3390/medicina57101072
18. Dunnill C, Patton T, Brennan J, Barrett J, Dryden M, Cooke J, et al. Reactive oxygen species (ROS) and wound healing: the functional role of ROS and emerging ROS-modulating technologies for augmentation of the healing process. Int Wound J. (2017) 14:89–96. doi: 10.1111/iwj.12557
19. Deng L, Du C, Song P, Chen T, Rui S, Armstrong DG, et al. The role of oxidative stress and antioxidants in diabetic wound healing. Oxid Med Cell Longev. (2021) 2021:8852759. doi: 10.1155/2021/8852759
20. Liu Y, Liu Y, He W, Mu X, Wu X, Deng J, et al. Fibroblasts: Immunomodulatory factors in refractory diabetic wound healing. Front Immunol. (2022) 13:918223. doi: 10.3389/fimmu.2022.918223
21. Zhao B, Li M, Su Y, Shan S, Qian W, Zhu D, et al. Role of transcription factor FOXM1 in diabetes and its complications (Review). Int J Mol Med. (2023) 52:101. doi: 10.3892/ijmm.2023.5304
22. Fernández-Guarino M, Bacci S, Pérez González LA, Bermejo-Martínez M, Cecilia-Matilla A, Hernández-Bule ML. The role of physical therapies in wound healing and assisted scarring. Int J Mol Sci. (2023) 24:7487. doi: 10.3390/ijms24087487
23. Armstrong DG, Tan T-W, Boulton AJM, Bus SA. Diabetic foot ulcers: A review. JAMA. (2023) 330:62–75. doi: 10.1001/jama.2023.10578
24. Magliano DJ, Boyko EJ. IDF Diabetes Atlas 10th edition scientific committee, in: IDF DIABETES ATLAS (2021). Brussels: International Diabetes Federation. Available online at: http://www.ncbi.nlm.nih.gov/books/NBK581934/ (Accessed April 1, 2024).
25. Perez-Favila A, Martinez-Fierro ML, Rodriguez-Lazalde JG, Cid-Baez MA, Zamudio-Osuna M de J, Martinez-Blanco MDR, et al. Current therapeutic strategies in diabetic foot ulcers. Medicina (Kaunas). (2019) 55:714. doi: 10.3390/medicina55110714
26. Zhang Y, Zhu Y, Ma P, Wu H, Xiao D, Zhang Y, et al. Functional carbohydrate-based hydrogels for diabetic wound therapy. Carbohydr Polym. (2023) 312:120823. doi: 10.1016/j.carbpol.2023.120823
27. Ao X, Ding W, Li X, Xu Q, Chen X, Zhou X, et al. Non-coding RNAs regulating mitochondrial function in cardiovascular diseases. J Mol Med (Berl). (2023) 101:501–26. doi: 10.1007/s00109-023-02305-8
28. Szabóová D, Guľašová Z, Hertelyová Z, Radoňak J. Non-coding RNAs in human cancer and other diseases: overview of the diagnostic potential. Int J Mol Sci. (2023) 24:16213. doi: 10.3390/ijms242216213. 37286733 R.
29. van Zonneveld AJ, Zhao Q, Rotmans JI, Bijkerk R. Circulating non-coding RNAs in chronic kidney disease and its complications. Nat Rev Nephrol. (2023) 19:573–86. doi: 10.1038/s41581-023-00725-w
30. Pekáčová A, Baloun J, Švec X, Šenolt L. Non-coding RNAs in diseases with a focus on osteoarthritis. Wiley Interdiscip Rev RNA. (2023) 14:e1756. doi: 10.1002/wrna.1756
31. Zhang Y, Zhang J, Xu Z, Zhang D, Xia P, Ling J, et al. Regulation of NcRNA-protein binding in diabetic foot. BioMed Pharmacother. (2023) 160:114361. doi: 10.1016/j.biopha.2023.114361
32. Kaur P, Kotru S, Singh S, Munshi A. miRNA signatures in diabetic retinopathy and nephropathy: delineating underlying mechanisms. J Physiol Biochem. (2022) 78:19–37. doi: 10.1007/s13105-021-00867-0
33. Kaur P, Kotru S, Singh S, Munshi A. Role of miRNAs in diabetic neuropathy: mechanisms and possible interventions. Mol Neurobiol. (2022) 59:1836–49. doi: 10.1007/s12035-021-02662-w
34. Li J, Bao H, Huang Z, Liang Z, Lin N, Ni C, et al. Non-coding RNA in cholangiocarcinoma: an update. Front Biosci (Landmark Ed). (2023) 28:173. doi: 10.31083/j.fbl2808173
35. Chen R, Wang SK, Belk JA, Amaya L, Li Z, Cardenas A, et al. Engineering circular RNA for enhanced protein production. Nat Biotechnol. (2023) 41:262–72. doi: 10.1038/s41587-022-01393-0
36. Alahdal M, Elkord E. Non-coding RNAs in cancer immunotherapy: Predictive biomarkers and targets. Clin Transl Med. (2023) 13:e1425. doi: 10.1002/ctm2.1425
37. Singh DD, Kim Y, Choi SA, Han I, Yadav DK. Clinical significance of microRNAs, long non-coding RNAs, and circRNAs in cardiovascular diseases. Cells. (2023) 12:1629. doi: 10.3390/cells12121629
38. Elazazy O, Midan HM, Shahin RK, Elesawy AE, Elballal MS, Sallam A-AM, et al. Long non-coding RNAs and rheumatoid arthritis: Pathogenesis and clinical implications. Pathol Res Pract. (2023) 246:154512. doi: 10.1016/j.prp.2023.154512
39. Jumat MI, Sarmiento ME, Acosta A, Chin KL. Role of non-coding RNAs in tuberculosis and their potential for clinical applications. J Appl Microbiol. (2023) 134:lxad104. doi: 10.1093/jambio/lxad104
40. Wang G, Mi J, Bai J, He Q, Li X, Wang Z. Non-coding RNAs in kidney stones. Biomolecules. (2024) 14:213. doi: 10.3390/biom14020213
41. Moallemi Rad L, Sadoughi MM, Nicknam A, Colagar AH, Hussen BM, Taheri M, et al. The impact of non-coding RNAs in the pathobiology of eye disorders. Int J Biol Macromol. (2023) 239:124245. doi: 10.1016/j.ijbiomac.2023.124245
42. Xu Q-L, Luo Z, Zhang B, Qin G-J, Zhang R-Y, Kong X-Y, et al. Methylation-associated silencing of miR-9-1 promotes nasopharyngeal carcinoma progression and glycolysis via HK2. Cancer Sci. (2021) 112:4127–38. doi: 10.1111/cas.15103
43. Ding L, Lu S, Zhou Y, Lyu D, Ouyang C, Ma Z, et al. The 3’ Untranslated region protects the heart from angiotensin II-induced cardiac dysfunction via AGGF1 expression. Mol Ther. (2020) 28:1119–32. doi: 10.1016/j.ymthe.2020.02.002
44. Deng X, Niu L, Xiao J, Guo Q, Liang J, Tang J, et al. Involvement of intestinal flora and miRNA into the mechanism of coarse grains improving type 2 diabetes: an overview. J Sci Food Agric. (2023) 103:4257–67. doi: 10.1002/jsfa.12270
45. Scherm MG, Daniel C. miRNA regulation of T cells in islet autoimmunity and type 1 diabetes. Curr Diabetes Rep. (2020) 20:41. doi: 10.1007/s11892-020-01325-9
46. Tang Y-B, Uwimana MMP, Zhu S-Q, Zhang L-X, Wu Q, Liang Z-X. Non-coding RNAs: Role in diabetic foot and wound healing. World J Diabetes. (2022) 13:1001–13. doi: 10.4239/wjd.v13.i12.1001
47. Chen L, Zhan CZ, Wang T, You H, Yao R. Curcumin inhibits the proliferation, migration, invasion, and apoptosis of diffuse large B-cell lymphoma cell line by regulating MiR-21/VHL axis. Yonsei Med J. (2020) 61:20–9. doi: 10.3349/ymj.2020.61.1.20
48. Wei J, Li J, Geng D, Peng Y, Yang B, Wu H, et al. Expression of miR-4739 in Gastric cancer and its Relationship with Clinical Pathological Features of Patients. Front Surg. (2022) 9:897583. doi: 10.3389/fsurg.2022.897583
49. Zhou J, Zhang B, Zhang X, Wang C, Xu Y. Identification of a 3-miRNA signature associated with the prediction of prognosis in nasopharyngeal carcinoma. Front Oncol. (2021) 11:823603. doi: 10.3389/fonc.2021.823603
50. Wang W, Yang C, Wang XY, Zhou LY, Lao GJ, Liu D, et al. MicroRNA-129 and -335 promote diabetic wound healing by inhibiting Sp1-mediated MMP-9 expression. Diabetes. (2018) 67:1627–38. doi: 10.2337/db17-1238
51. Liu X, Wang Y, Zhang X, Zhang X, Guo J, Zhou J, et al. MicroRNA-296-5p promotes healing of diabetic wound by targeting sodium-glucose transporter 2 (SGLT2). Diabetes Metab Res Rev. (2019) 35:e3104. doi: 10.1002/dmrr.3104
52. Zuo C, Fan P, Yang Y, Hu C. MiR-488-3p facilitates wound healing through CYP1B1-mediated Wnt/β-catenin signaling pathway by targeting MeCP2. J Diabetes Investig. (2024) 15:145–58. doi: 10.1111/jdi.14099
53. Jie R, Qian J, Tang Y, Li Y, Xu M, Zhao X, et al. Role of increased miR-222-3p expression in peripheral blood and wound marginal tissues of type 2 diabetes mellitus patients with diabetic foot ulcer. Diabetes Metab Syndr Obes. (2023) 16:2419–32. doi: 10.2147/DMSO.S410986
54. Xue W-L, Chen R-Q, Zhang Q-Q, Li X-H, Cao L, Li M-Y, et al. Hydrogen sulfide rescues high glucose-induced migration dysfunction in HUVECs by upregulating miR-126-3p. Am J Physiol Cell Physiol. (2020) 318:C857–69. doi: 10.1152/ajpcell.00406.2019
55. Sharma S, Bhonde R. Applicability of mesenchymal stem cell-derived exosomes as a cell-free miRNA therapy and epigenetic modifiers for diabetes. Epigenomics. (2023) 15:1323–36. doi: 10.2217/epi-2023-0302
56. Natalicchio A, Montagnani M, Gallo M, Marrano N, Faggiano A, Zatelli MC, et al. MiRNA dysregulation underlying common pathways in type 2 diabetes and cancer development: an Italian Association of Medical Oncology (AIOM)/Italian Association of Medical Diabetologists (AMD)/Italian Society of Diabetology (SID)/Italian Society of Endocrinology (SIE)/Italian Society of Pharmacology (SIF) multidisciplinary critical view. ESMO Open. (2023) 8:101573. doi: 10.1016/j.esmoop.2023.101573
57. Nie X, Zhao J, Ling H, Deng Y, Li X, He Y. Exploring microRNAs in diabetic chronic cutaneous ulcers: Regulatory mechanisms and therapeutic potential. Br J Pharmacol. (2020) 177:4077–95. doi: 10.1111/bph.15139
58. Shen J, Zhao X, Zhong Y, Yang P, Gao P, Wu X, et al. Exosomal ncRNAs: The pivotal players in diabetic wound healing. Front Immunol. (2022) 13:1005307. doi: 10.3389/fimmu.2022.1005307
59. Li H, Jing S, Xu H. Effect and mechanism of microRNAs on various diabetic wound local cells. J Diabetes. (2023) 15:955–67. doi: 10.1111/1753-0407.13474
60. Anuradha U, Mehra NK, Khatri DK. Understanding molecular mechanisms and miRNA-based targets in diabetes foot ulcers. Mol Biol Rep. (2024) 51:82. doi: 10.1007/s11033-023-09074-0
61. Zgheib C, Hilton SA, Dewberry LC, Hodges MM, Ghatak S, Xu J, et al. Use of cerium oxide nanoparticles conjugated with microRNA-146a to correct the diabetic wound healing impairment. J Am Coll Surg. (2019) 228:107–15. doi: 10.1016/j.jamcollsurg.2018.09.017
62. Peng X, He F, Mao Y, Lin Y, Fang J, Chen Y, et al. miR-146a promotes M2 macrophage polarization and accelerates diabetic wound healing by inhibiting the TLR4/NF-κB axis. J Mol Endocrinol. (2022) 69:315–27. doi: 10.1530/JME-21-0019
63. Bi X, Zhou L, Liu Y, Gu J, Mi Q-S. MicroRNA-146a deficiency delays wound healing in normal and diabetic mice. Adv Wound Care (New Rochelle). (2022) 11:19–27. doi: 10.1089/wound.2020.1165
64. Huang J, Fu J, Liu B, Wang R, You T. A synthetic curcuminoid analog, (2E,6E)-2,6-bis(2-(trifluoromethyl)benzylidene)cyclohexanone, ameliorates impaired wound healing in streptozotocin-induced diabetic mice by increasing miR-146a. Molecules. (2020) 25:920. doi: 10.3390/molecules25040920
65. Dewberry LC, Niemiec SM, Hilton SA, Louiselle AE, Singh S, Sakthivel TS, et al. Cerium oxide nanoparticle conjugation to microRNA-146a mechanism of correction for impaired diabetic wound healing. Nanomedicine. (2022) 40:102483. doi: 10.1016/j.nano.2021.102483
66. Li Q, Hu W, Huang Q, Yang J, Li B, Ma K, et al. MiR146a-loaded engineered exosomes released from silk fibroin patch promote diabetic wound healing by targeting IRAK1. Signal Transduct Target Ther. (2023) 8:62. doi: 10.1038/s41392-022-01263-w
67. Zhou X, Ye C, Jiang L, Zhu X, Zhou F, Xia M, et al. The bone mesenchymal stem cell-derived exosomal miR-146a-5p promotes diabetic wound healing in mice via macrophage M1/M2 polarization. Mol Cell Endocrinol. (2024) 579:112089. doi: 10.1016/j.mce.2023.112089
68. Hao Y, Yang L, Liu Y, Ye Y, Wang J, Yu C, et al. mmu-miR-145a-5p accelerates diabetic wound healing by promoting macrophage polarization toward the M2 phenotype. Front Med (Lausanne). (2021) 8:775523. doi: 10.3389/fmed.2021.775523
69. Chen H, Gao J, Xu Q, Wan D, Zhai W, Deng L, et al. MiR-145-5p modulates lipid metabolism and M2 macrophage polarization by targeting PAK7 and regulating β-catenin signaling in hyperlipidemia. Can J Physiol Pharmacol. (2021) 99:857–63. doi: 10.1139/cjpp-2020-0539
70. Su J, Wei Q, Ma K, Wang Y, Hu W, Meng H, et al. P-MSC-derived extracellular vesicles facilitate diabetic wound healing via miR-145-5p/ CDKN1A-mediated functional improvements of high glucose-induced senescent fibroblasts. Burns Trauma. (2023) 11:tkad010. doi: 10.1093/burnst/tkad010
71. Wang C, Huang L, Li J, Liu D, Wu B. MicroRNA miR-145-5p inhibits cutaneous wound healing by targeting PDGFD in diabetic foot ulcer. Biochem Genet. (2023) 62:2437–54. doi: 10.1007/s10528-023-10551-1
72. Ban E, Jeong S, Park M, Kwon H, Park J, Song EJ, et al. Accelerated wound healing in diabetic mice by miRNA-497 and its anti-inflammatory activity. BioMed Pharmacother. (2020) 121:109613. doi: 10.1016/j.biopha.2019.109613
73. Das A, Ganesh K, Khanna S, Sen CK, Roy S. Engulfment of apoptotic cells by macrophages: a role of microRNA-21 in the resolution of wound inflammation. J Immunol. (2014) 192:1120–9. doi: 10.4049/jimmunol.1300613
74. Yu H, Lin L, Zhang Z, Zhang H, Hu H. Targeting NF-κB pathway for the therapy of diseases: mechanism and clinical study. Signal Transduct Target Ther. (2020) 5:209. doi: 10.1038/s41392-020-00312-6
75. Wang K-X, Zhao L-L, Zheng L-T, Meng L-B, Jin L, Zhang L-J, et al. Accelerated wound healing in diabetic rat by miRNA-185-5p and its anti-inflammatory activity. Diabetes Metab Syndr Obes. (2023) 16:1657–67. doi: 10.2147/DMSO.S409596
76. Ge L, Wang K, Lin H, Tao E, Xia W, Wang F, et al. Engineered exosomes derived from miR-132-overexpresssing adipose stem cells promoted diabetic wound healing and skin reconstruction. Front Bioeng Biotechnol. (2023) 11:1129538. doi: 10.3389/fbioe.2023.1129538
77. Yang H, Xu H, Wang Z, Li X, Wang P, Cao X, et al. Analysis of miR-203a-3p/SOCS3-mediated induction of M2 macrophage polarization to promote diabetic wound healing based on epidermal stem cell-derived exosomes. Diabetes Res Clin Pract. (2023) 197:110573. doi: 10.1016/j.diabres.2023.110573
78. Dudley AC, Griffioen AW. Pathological angiogenesis: mechanisms and therapeutic strategies. Angiogenesis. (2023) 26:313–47. doi: 10.1007/s10456-023-09876-7
79. Wang G, Lin F, Wan Q, Wu J, Luo M. Mechanisms of action of metformin and its regulatory effect on microRNAs related to angiogenesis. Pharmacol Res. (2021) 164:105390. doi: 10.1016/j.phrs.2020.105390
80. Peng Y, Wang Y, Zhou C, Mei W, Zeng C. PI3K/Akt/mTOR pathway and its role in cancer therapeutics: are we making headway? Front Oncol. (2022) 12:819128. doi: 10.3389/fonc.2022.819128
81. Primer KR, Psaltis PJ, Tan JTM, Bursill CA. The role of high-density lipoproteins in endothelial cell metabolism and diabetes-impaired angiogenesis. Int J Mol Sci. (2020) 21:3633. doi: 10.3390/ijms21103633
82. Lyttle BD, Vaughn AE, Bardill JR, Apte A, Gallagher LT, Zgheib C, et al. Effects of microRNAs on angiogenesis in diabetic wounds. Front Med (Lausanne). (2023) 10:1140979. doi: 10.3389/fmed.2023.1140979
83. Chen W-T, Luo Y, Chen X-M, Xiao J-H. Role of exosome-derived miRNAs in diabetic wound angiogenesis. Mol Cell Biochem. (2023). doi: 10.1007/s11010-023-04874-1
84. Yang J, Liu Z. Mechanistic pathogenesis of endothelial dysfunction in diabetic nephropathy and retinopathy. Front Endocrinol (Lausanne). (2022) 13:816400. doi: 10.3389/fendo.2022.816400
85. Liu J, Wang J, Fu W, Wang X, Chen H, Wu X, et al. MiR-195-5p and miR-205-5p in extracellular vesicles isolated from diabetic foot ulcer wound fluid decrease angiogenesis by inhibiting VEGFA expression. Aging (Albany NY). (2021) 13:19805–21. doi: 10.18632/aging.203393
86. Icli B, Wu W, Ozdemir D, Li H, Haemmig S, Liu X, et al. MicroRNA-135a-3p regulates angiogenesis and tissue repair by targeting p38 signaling in endothelial cells. FASEB J. (2019) 33:5599–614. doi: 10.1096/fj.201802063RR
87. Icli B, Wu W, Ozdemir D, Li H, Cheng HS, Haemmig S, et al. MicroRNA-615-5p regulates angiogenesis and tissue repair by targeting AKT/eNOS (Protein kinase B/endothelial nitric oxide synthase) signaling in endothelial cells. Arterioscler Thromb Vasc Biol. (2019) 39:1458–74. doi: 10.1161/ATVBAHA.119.312726
88. Wang H, Wang X, Liu X, Zhou J, Yang Q, Chai B, et al. miR-199a-5p plays a pivotal role on wound healing via suppressing VEGFA and ROCK1 in diabetic ulcer foot. Oxid Med Cell Longev. (2022) 2022:4791059. doi: 10.1155/2022/4791059
89. Shaorong Z, Xiaodong L, Qiong P, Zhaodong X, Zhuo L, Hechen H, et al. SNHG12/NFYC-AS1 acted as the sponge for hsa-miR-199a-5p to promote the expression of S100A8/S100A7/XDH and was involved in the progression of diabetic foot ulcers. Mol Biotechnol. (2023) 65:2038–48. doi: 10.1007/s12033-023-00692-4
90. Ai X, Yu P, Luo L, Sun J, Tao H, Wang X, et al. Berberis dictyophylla F. inhibits angiogenesis and apoptosis of diabetic retinopathy via suppressing HIF-1α/VEGF/DLL-4/Notch-1 pathway. J Ethnopharmacol. (2022) 296:115453. doi: 10.1016/j.jep.2022.115453
91. Xiao S, Zhang D, Liu Z, Jin W, Huang G, Wei Z, et al. Diabetes-induced glucolipotoxicity impairs wound healing ability of adipose-derived stem cells-through the miR-1248/CITED2/HIF-1α pathway. Aging (Albany NY). (2020) 12:6947–65. doi: 10.18632/aging.103053
92. Chen J, Wang C, Yuan M, Kang Y, Wu Z, Li W, et al. Milk exosomes-mediated miR-31-5p delivery accelerates diabetic wound healing through promoting angiogenesis. Drug Delivery. (2022) 29:214–28. doi: 10.1080/10717544.2021.2023699
93. Chen L, Shen S, Wang S. LncRNA SNHG16 knockdown promotes diabetic foot ulcer wound healing via sponging MiR-31-5p. Tohoku J Exp Med. (2023) 261:283–9. doi: 10.1620/tjem.2023.J078
94. Huang J, Yu M, Yin W, Liang B, Li A, Li J, et al. Development of a novel RNAi therapy: Engineered miR-31 exosomes promoted the healing of diabetic wounds. Bioact Mater. (2021) 6:2841–53. doi: 10.1016/j.bioactmat.2021.02.007
95. Xiong Y, Chen L, Yu T, Yan C, Zhou W, Cao F, et al. Inhibition of circulating exosomal microRNA-15a-3p accelerates diabetic wound repair. Aging (Albany NY). (2020) 12:8968–86. doi: 10.18632/aging.103143
96. Yu Q, Liu L, Zhang X, Chang H, Ma S, Xie Z, et al. MiR-221-3p targets HIPK2 to promote diabetic wound healing. Microvasc Res. (2022) 140:104306. doi: 10.1016/j.mvr.2021.104306
97. Hu K, Liu X, Chang H, Zhang Y, Zhou H, Liu L, et al. MicroRNA-221-3p targets THBS1 to promote wound healing in diabetes. Diabetes Metab Syndr Obes. (2023) 16:2765–77. doi: 10.2147/DMSO.S424847
98. Qiu T-Y, Huang J, Wang L-P, Zhu B-S. Inhibition of miR-200b promotes angiogenesis in endothelial cells by activating the notch pathway. Cell J. (2021) 23:51–60. doi: 10.22074/cellj.2021.7080
99. Jere SW, Houreld NN. Regulatory processes of the canonical Wnt/β-catenin pathway and photobiomodulation in diabetic wound repair. Int J Mol Sci. (2022) 23:4210. doi: 10.3390/ijms23084210
100. Xu Y, Yu T, He L, Ouyang L, Qu Y, Zhou J, et al. Inhibition of miRNA-152-3p enhances diabetic wound repair via upregulation of PTEN. Aging (Albany NY). (2020) 12:14978–89. doi: 10.18632/aging.103557
101. Wei Q, Su J, Meng S, Wang Y, Ma K, Li B, et al. MiR-17-5p-engineered sEVs Encapsulated in GelMA Hydrogel Facilitated Diabetic Wound Healing by Targeting PTEN and p21. Adv Sci (Weinh). (2024) 11:e2307761. doi: 10.1002/advs.202307761
102. Chamorro-Jorganes A, Lee MY, Araldi E, Landskroner-Eiger S, Fernández-Fuertes M, Sahraei M, et al. VEGF-induced expression of miR-17-92 cluster in endothelial cells is mediated by ERK/ELK1 activation and regulates angiogenesis. Circ Res. (2016) 118:38–47. doi: 10.1161/CIRCRESAHA.115.307408
103. Li D, Peng H, Qu L, Sommar P, Wang A, Chu T, et al. miR-19a/b and miR-20a promote wound healing by regulating the inflammatory response of keratinocytes. J Invest Dermatol. (2021) 141:659–71. doi: 10.1016/j.jid.2020.06.037
104. Sun X-J, Chen J-A, Wang L, Li G, Wang A-P. A gene chip study suggests that miR-17-3p is associated with diabetic foot ulcers. Int Wound J. (2023) 20:1525–33. doi: 10.1111/iwj.14007
105. Lucas T, Schäfer F, Müller P, Eming SA, Heckel A, Dimmeler S. Light-inducible antimiR-92a as a therapeutic strategy to promote skin repair in healing-impaired diabetic mice. Nat Commun. (2017) 8:15162. doi: 10.1038/ncomms15162
106. Gallant-Behm CL, Piper J, Dickinson BA, Dalby CM, Pestano LA, Jackson AL. A synthetic microRNA-92a inhibitor (MRG-110) accelerates angiogenesis and wound healing in diabetic and nondiabetic wounds. Wound Repair Regener. (2018) 26:311–23. doi: 10.1111/wrr.12660
107. Wang J, Han Y, Huang F, Tang L, Mu J, Liang Y. Diabetic macrophage small extracellular vesicles-associated miR-503/IGF1R axis regulates endothelial cell function and affects wound healing. Front Immunol. (2023) 14:1104890. doi: 10.3389/fimmu.2023.1104890
108. Bestepe F, Ghanem GF, Fritsche CM, Weston J, Sahay S, Mauro AK, et al. MicroRNA-409-3p/BTG2 signaling axis improves impaired angiogenesis and wound healing in obese mice. FASEB J. (2024) 38:e23459. doi: 10.1096/fj.202302124RR
109. Rousselle P, Braye F, Dayan G. Re-epithelialization of adult skin wounds: Cellular mechanisms and therapeutic strategies. Adv Drug Delivery Rev. (2019) 146:344–65. doi: 10.1016/j.addr.2018.06.019
110. Li X, Li N, Li B, Feng Y, Zhou D, Chen G. Noncoding RNAs and RNA-binding proteins in diabetic wound healing. Bioorg Med Chem Lett. (2021) 50:128311. doi: 10.1016/j.bmcl.2021.128311
111. Gondaliya P, Sayyed AA, Bhat P, Mali M, Arya N, Khairnar A, et al. Mesenchymal stem cell-derived exosomes loaded with miR-155 inhibitor ameliorate diabetic wound healing. Mol Pharm. (2022) 19:1294–308. doi: 10.1021/acs.molpharmaceut.1c00669
112. Liu Y, Liu Y, Deng J, Li W, Nie X. Fibroblast growth factor in diabetic foot ulcer: progress and therapeutic prospects. Front Endocrinol (Lausanne). (2021) 12:744868. doi: 10.3389/fendo.2021.744868
113. Moura J, Sørensen A, Leal EC, Svendsen R, Carvalho L, Willemoes RJ, et al. microRNA-155 inhibition restores Fibroblast Growth Factor 7 expression in diabetic skin and decreases wound inflammation. Sci Rep. (2019) 9:5836. doi: 10.1038/s41598-019-42309-4
114. Dallas A, Trotsyuk A, Ilves H, Bonham CA, Rodrigues M, Engel K, et al. Acceleration of diabetic wound healing with PHD2- and miR-210-targeting oligonucleotides. Tissue Eng Part A. (2019) 25:44–54. doi: 10.1089/ten.TEA.2017.0484
115. Shi Y, Wang S, Liu D, Wang Z, Zhu Y, Li J, et al. Exosomal miR-4645-5p from hypoxic bone marrow mesenchymal stem cells facilitates diabetic wound healing by restoring keratinocyte autophagy. Burns Trauma. (2024) 12:tkad058. doi: 10.1093/burnst/tkad058
116. Gajewska B, Śliwińska-Mossoń M. Association of MMP-2 and MMP-9 polymorphisms with diabetes and pathogenesis of diabetic complications. Int J Mol Sci. (2022) 23:10571. doi: 10.3390/ijms231810571
117. Li P, Hong G, Zhan W, Deng M, Tu C, Wei J, et al. Endothelial progenitor cell derived exosomes mediated miR-182-5p delivery accelerate diabetic wound healing via down-regulating PPARG. Int J Med Sci. (2023) 20:468–81. doi: 10.7150/ijms.78790
118. Lv Q, Deng J, Chen Y, Wang Y, Liu B, Liu J. Engineered human adipose stem-cell-derived exosomes loaded with miR-21-5p to promote diabetic cutaneous wound healing. Mol Pharm. (2020) 17:1723–33. doi: 10.1021/acs.molpharmaceut.0c00177
119. Liu Y, Zhao J, Mu X, Deng J, Wu X, He W, et al. Asiaticoside-nitric oxide promoting diabetic wound healing through the miRNA-21-5p/TGF-β1/SMAD7/TIMP3 signaling pathway. J Ethnopharmacol. (2024) 319:117266. doi: 10.1016/j.jep.2023.117266
120. Wu Y, Zhang K, Liu R, Zhang H, Chen D, Yu S, et al. MicroRNA-21-3p accelerates diabetic wound healing in mice by downregulating SPRY1. Aging (Albany NY). (2020) 12:15436–45. doi: 10.18632/aging.103610
121. Zhang P, Song X, Dong Q, Zhou L, Wang L. miR-27-3p inhibition restore fibroblasts viability in diabetic wound by targeting NOVA1. Aging (Albany NY). (2020) 12:12841–9. doi: 10.18632/aging.103266
122. Ghafouri-Fard S, Shoorei H, Mohaqiq M, Taheri M. Non-coding RNAs regulate angiogenic processes. Vascul Pharmacol. (2020) 133–134:106778. doi: 10.1016/j.vph.2020.106778
123. Wang M, Gan S, Li B, Wang Y. Long non-coding RNA-ATB attenuates the angiotensin II-induced injury of vascular endothelial cell. Ann Clin Lab Sci. (2020) 50:378–82.
124. González-Moro I, Santin I. Long non-coding RNA-regulated pathways in pancreatic β cells: Their role in diabetes. Int Rev Cell Mol Biol. (2021) 359:325–55. doi: 10.1016/bs.ircmb.2021.02.007
125. Chen Y, Li Z, Chen X, Zhang S. Long non-coding RNAs: From disease code to drug role. Acta Pharm Sin B. (2021) 11:340–54. doi: 10.1016/j.apsb.2020.10.001
126. Li X, Zhu J, Zhong Y, Liu C, Yao M, Sun Y, et al. Targeting long noncoding RNA-AQP4-AS1 for the treatment of retinal neurovascular dysfunction in diabetes mellitus. EBioMedicine. (2022) 77:103857. doi: 10.1016/j.ebiom.2022.103857
127. Jia D, He Y, Wang Y, Xue M, Zhu L, Xia F, et al. NEAT1: A novel long non-coding RNA involved in mediating type 2 diabetes and its various complications. Curr Pharm Des. (2022) 28:1342–50. doi: 10.2174/1381612828666220428093207
128. Srivastava SP, Goodwin JE, Tripathi P, Kanasaki K, Koya D. Interactions among Long Non-Coding RNAs and microRNAs Influence Disease Phenotype in Diabetes and Diabetic Kidney Disease. Int J Mol Sci. (2021) 22:6027. doi: 10.3390/ijms22116027
129. Chi Y, Wang D, Wang J, Yu W, Yang J. Long non-coding RNA in the pathogenesis of cancers. Cells. (2019) 8:1015. doi: 10.3390/cells8091015
130. Samra M, Srivastava K. Non-coding RNA and their potential role in cardiovascular diseases. Gene. (2023) 851:147011. doi: 10.1016/j.gene.2022.147011
131. Kuai L, Jiang J-S, Li W, Li B, Yin S-Y. Long non-coding RNAs in diabetic wound healing: Current research and clinical relevance. Int Wound J. (2022) 19:583–600. doi: 10.1111/iwj.13655
132. Tao S-C, Rui B-Y, Wang Q-Y, Zhou D, Zhang Y, Guo S-C. Extracellular vesicle-mimetic nanovesicles transport LncRNA-H19 as competing endogenous RNA for the treatment of diabetic wounds. Drug Delivery. (2018) 25:241–55. doi: 10.1080/10717544.2018.1425774
133. Li B, Qian L, Pi L, Meng X. A therapeutic role of exosomal lncRNA H19 from adipose mesenchymal stem cells in cutaneous wound healing by triggering macrophage M2 polarization. Cytokine. (2023) 165:156175. doi: 10.1016/j.cyto.2023.156175
134. Hu J, Zhang L, Liechty C, Zgheib C, Hodges MM, Liechty KW, et al. Long noncoding RNA GAS5 regulates macrophage polarization and diabetic wound healing. J Invest Dermatol. (2020) 140:1629–38. doi: 10.1016/j.jid.2019.12.030
135. Kuang L, Zhang C, Li B, Deng H, Chen R, Li G. Human Keratinocyte-Derived Exosomal MALAT1 Promotes Diabetic Wound Healing by Upregulating MFGE8 via microRNA-1914-3p. Int J Nanomedicine. (2023) 18:949–70. doi: 10.2147/IJN.S399785
136. Zgheib C, Hodges MM, Hu J, Liechty KW, Xu J. Long non-coding RNA Lethe regulates hyperglycemia-induced reactive oxygen species production in macrophages. PloS One. (2017) 12:e0177453. doi: 10.1371/journal.pone.0177453
137. Wang H, Ding L, Xu F, He L, Ye L, Huang L, et al. Construction of novel amphiphilic chitosan-polylactide graft copolymer nanodroplets for contrast enhanced ultrasound tumor imaging. J Biomater Appl. (2021) 36:613–25. doi: 10.1177/08853282211011766
138. Chen J, Zhao Q, Peng J, Yang X, Yu D, Zhao W. Antibacterial and mechanical properties of reduced graphene-silver nanoparticle nanocomposite modified glass ionomer cements. J Dent. (2020) 96:103332. doi: 10.1016/j.jdent.2020.103332
139. Born LJ, Chang K-H, Shoureshi P, Lay F, Bengali S, Hsu ATW, et al. HOTAIR-loaded mesenchymal stem/stromal cell extracellular vesicles enhance angiogenesis and wound healing. Adv Healthc Mater. (2022) 11:e2002070. doi: 10.1002/adhm.202002070
140. Peng W-X, He P-X, Liu L-J, Zhu T, Zhong Y-Q, Xiang L, et al. LncRNA GAS5 activates the HIF1A/VEGF pathway by binding to TAF15 to promote wound healing in diabetic foot ulcers. Lab Invest. (2021) 101:1071–83. doi: 10.1038/s41374-021-00598-2
141. Wan J, Bao Y, Hou L-J, Li G-J, Du L-J, Ma Z-H, et al. lncRNA ANRIL accelerates wound healing in diabetic foot ulcers via modulating HIF1A/VEGFA signaling through interacting with FUS. J Gene Med. (2023) 25:e3462. doi: 10.1002/jgm.3462
142. Han Z-F, Cao J-H, Liu Z-Y, Yang Z, Qi R-X, Xu H-L. Exosomal lncRNA KLF3-AS1 derived from bone marrow mesenchymal stem cells stimulates angiogenesis to promote diabetic cutaneous wound healing. Diabetes Res Clin Pract. (2022) 183:109126. doi: 10.1016/j.diabres.2021.109126
143. Fu W, Liang D, Wu X, Chen H, Hong X, Wang J, et al. Long noncoding RNA LINC01435 impedes diabetic wound healing by facilitating YY1-mediated HDAC8 expression. iScience. (2022) 25:104006. doi: 10.1016/j.isci.2022.104006
144. Yang M, Gu Y. LncRNA DLEU1 promotes angiogenesis in diabetic foot ulcer wound healing by regulating miR-96-5p. Ir J Med Sci. (2024) 193:241–7. doi: 10.1007/s11845-023-03471-x
145. Yang H, Zhang Y, Du Z, Wu T, Yang C. Hair follicle mesenchymal stem cell exosomal lncRNA H19 inhibited NLRP3 pyroptosis to promote diabetic mouse skin wound healing. Aging (Albany NY). (2023) 15:791–809. doi: 10.18632/aging.204513
146. Yu P, Guo J, Li J, Shi X, Xu N, Jiang Y, et al. lncRNA-H19 in fibroblasts promotes wound healing in diabetes. Diabetes. (2022) 71:1562–78. doi: 10.2337/db21-0724
147. Li B, Zhou Y, Chen J, Wang T, Li Z, Fu Y, et al. Long non-coding RNA H19 contributes to wound healing of diabetic foot ulcer. J Mol Endocrinol. (2020) 65:69–84. doi: 10.1530/JME-19-0242
148. Li B, Zhou Y, Chen J, Wang T, Li Z, Fu Y, et al. Long noncoding RNA H19 acts as a miR-29b sponge to promote wound healing in diabetic foot ulcer. FASEB J. (2021) 35:e20526. doi: 10.1096/fj.201900076RRRRR
149. Qian L, Pi L, Fang B-R, Meng X-X. Adipose mesenchymal stem cell-derived exosomes accelerate skin wound healing via the lncRNA H19/miR-19b/SOX9 axis. Lab Invest. (2021) 101:1254–66. doi: 10.1038/s41374-021-00611-8
150. Guo J-R, Yin L, Chen Y-Q, Jin X-J, Zhou X, Zhu N-N, et al. Autologous blood transfusion augments impaired wound healing in diabetic mice by enhancing lncRNA H19 expression via the HIF-1α signaling pathway. Cell Commun Signal. (2018) 16:84. doi: 10.1186/s12964-018-0290-6
151. Ji W, Sun Z, Cheng Y. LncRNA H19 inhibits keratinocyte cell proliferation and migration by targeting miR-17-5p/RUNX1 axis in chronic wounds. J Burn Care Res. (2023) 45:366–72. doi: 10.1093/jbcr/irad145
152. He M, Tu L, Shu R, Meng Q, Du S, Xu Z, et al. Long Noncoding RNA CASC2 Facilitated Wound Healing through miRNA-155/HIF-1α in Diabetic Foot Ulcers. Contrast Media Mol Imaging. (2022) 2022:6291497. doi: 10.1155/2022/6291497
153. Zhang L, Hu J, Meshkat BI, Liechty KW, Xu J. LncRNA MALAT1 modulates TGF-β1-induced EMT in keratinocyte. Int J Mol Sci. (2021) 22:11816. doi: 10.3390/ijms222111816
154. Zhang L, Hung GC-C, Meng S, Evans R, Xu J. LncRNA MALAT1 Regulates Hyperglycemia Induced EMT in Keratinocyte via miR-205. Noncoding RNA. (2023) 9:14. doi: 10.3390/ncrna9010014
155. Shi R, Chen C, Zhao S, Yuan H, Zhao J, Zhao H. Stem cell therapy with CRISPR/Cas9-mediated MALAT1 delivery modulates miR-142 and rescues wound healing in rats with age-associated diabetic foot ulcers. Arch Gerontol Geriatr. (2024) 118:105283. doi: 10.1016/j.archger.2023.105283
156. Kuang L-W, Zhang C-C, Li B-H, Liu H-Z, Wang H, Li G-C. Identification of the MALAT1/miR-106a-5p/ZNF148 feedback loop in regulating HaCaT cell proliferation, migration and apoptosis. Regener Med. (2023) 18:239–58. doi: 10.2217/rme-2022-0189
157. Hong W, Xiong Z, Wang X, Liao X, Liu M, Jiang Z, et al. Long noncoding RNA XIST promotes cell proliferation and migration in diabetic foot ulcers through the miR-126-3p/EGFR axis. Diabetol Metab Syndr. (2024) 16:35. doi: 10.1186/s13098-024-01260-9
158. Alfaifi M, Ali Beg MM, Alshahrani MY, Ahmad I, Alkhathami AG, Joshi PC, et al. Circulating long non-coding RNAs NKILA, NEAT1, MALAT1, and MIAT expression and their association in type 2 diabetes mellitus. BMJ Open Diabetes Res Care. (2021) 9:e001821. doi: 10.1136/bmjdrc-2020-001821
159. Yang Y, Zhou J, Li WH, Zhou ZX, Xia XB. LncRNA NEAT1 regulated diabetic retinal epithelial-mesenchymal transition through regulating miR-204/SOX4 axis. PeerJ. (2021) 9:e11817. doi: 10.7717/peerj.11817
160. Wang X, Xu Y, Zhu Y-C, Wang Y-K, Li J, Li X-Y, et al. LncRNA NEAT1 promotes extracellular matrix accumulation and epithelial-to-mesenchymal transition by targeting miR-27b-3p and ZEB1 in diabetic nephropathy. J Cell Physiol. (2019) 234:12926–33. doi: 10.1002/jcp.27959
161. Huang S, Xu Y, Ge X, Xu B, Peng W, Jiang X, et al. Long noncoding RNA NEAT1 accelerates the proliferation and fibrosis in diabetic nephropathy through activating Akt/mTOR signaling pathway. J Cell Physiol. (2019) 234:11200–7. doi: 10.1002/jcp.27770
162. Shi Z, Wen Y, Zhang S, Cheng X. Circular RNA MTO1 intercorrelates with microRNA-630, both associate with Enneking stage and/or pathological fracture as well as prognosis in osteosarcoma patients. J Clin Lab Anal. (2021) 35:e23987. doi: 10.1002/jcla.23987
163. Zhou W-Y, Cai Z-R, Liu J, Wang D-S, Ju H-Q, Xu R-H. Circular RNA: metabolism, functions and interactions with proteins. Mol Cancer. (2020) 19:172. doi: 10.1186/s12943-020-01286-3
164. Shang F-F, Luo S, Liang X, Xia Y. Alterations of circular RNAs in hyperglycemic human endothelial cells. Biochem Biophys Res Commun. (2018) 499:551–5. doi: 10.1016/j.bbrc.2018.03.187
165. Kong Z, Han Q, Zhu B, Wan L, Feng E. Circ_0069094 regulates Malignant phenotype and paclitaxel resistance in breast cancer cells via targeting the miR-136-5p/YWHAZ axis. Thorac Cancer. (2023) 14:1831–42. doi: 10.1111/1759-7714.14928
166. Li D, Guo J, Ni X, Sun G, Bao H. The progress and challenges of circRNA for diabetic foot ulcers: A mini-review. Front Endocrinol (Lausanne). (2022) 13:1019935. doi: 10.3389/fendo.2022.1019935
167. Xiong T, Xia L, Song Q. Circular RNA SPI1 expression before and after induction therapy and its correlation with clinical features, treatment response, and survival of acute myeloid leukemia patients. J Clin Lab Anal. (2023) 37:e24835. doi: 10.1002/jcla.24835
168. Chen D, Jiang X, Luo H, Hua Q, Zhang F. CircPTPRM accelerates Malignancy of papillary thyroid cancer via miR-885-5p/DNMT3A axis. J Clin Lab Anal. (2022) 36:e24688. doi: 10.1002/jcla.24688
169. Tang T, Chen L, Zhang M, Wang C, Du X, Ye S, et al. Exosomes derived from BMSCs enhance diabetic wound healing through circ-Snhg11 delivery. Diabetol Metab Syndr. (2024) 16:37. doi: 10.1186/s13098-023-01210-x
170. Shi R, Jin Y, Zhao S, Yuan H, Shi J, Zhao H. Hypoxic ADSC-derived exosomes enhance wound healing in diabetic mice via delivery of circ-Snhg11 and induction of M2-like macrophage polarization. BioMed Pharmacother. (2022) 153:113463. doi: 10.1016/j.biopha.2022.113463
171. Yin D, Shen G. Exosomes from adipose-derived stem cells regulate macrophage polarization and accelerate diabetic wound healing via the circ-Rps5/miR-124-3p axis. Immun Inflammation Dis. (2024) 12:e1274. doi: 10.1002/iid3.1274
172. Pyšná A, Bém R, Němcová A, Fejfarová V, Jirkovská A, Hazdrová J, et al. Endothelial progenitor cells biology in diabetes mellitus and peripheral arterial disease and their therapeutic potential. Stem Cell Rev Rep. (2019) 15:157–65. doi: 10.1007/s12015-018-9863-4
173. Shang B, Xu T, Hu N, Mao Y, Du X. Circ-Klhl8 overexpression increased the therapeutic effect of EPCs in diabetic wound healing via the miR-212-3p/SIRT5 axis. J Diabetes Complications. (2021) 35:108020. doi: 10.1016/j.jdiacomp.2021.108020
174. Wang Z, Feng C, Liu H, Meng T, Huang W-Q, Song K-X, et al. Exosomes from circ-Astn1-modified adipose-derived mesenchymal stem cells enhance wound healing through miR-138-5p/SIRT1/FOXO1 axis regulation. World J Stem Cells. (2023) 15:476–89. doi: 10.4252/wjsc.v15.i5.476
175. Shi R, Jin Y, Hu W, Lian W, Cao C, Han S, et al. Exosomes derived from mmu_circ_0000250-modified adipose-derived mesenchymal stem cells promote wound healing in diabetic mice by inducing miR-128-3p/SIRT1-mediated autophagy. Am J Physiol Cell Physiol. (2020) 318:C848–56. doi: 10.1152/ajpcell.00041.2020
176. Tang W, Du X, Wu Z, Nie Z, Yu C, Gao Y. circ-Erbb2ip from adipose-derived mesenchymal stem cell-derived exosomes promotes wound healing in diabetic mice by inducing the miR-670-5p/Nrf1 axis. Cell Signal. (2024) 121:111245. doi: 10.1016/j.cellsig.2024.111245
177. Fu Z, Jiang Z, Liao X, Liu M, Guo G, Wang X, et al. Upregulation of circ_0080968 in diabetic foot ulcer inhibits wound healing via repressing the migration and promoting proliferation of keratinocytes. Gene. (2023) 883:147669. doi: 10.1016/j.gene.2023.147669
178. Han D, Liu W, Li G, Liu L. Circ_PRKDC knockdown promotes skin wound healing by enhancing keratinocyte migration via miR-31/FBN1 axis. J Mol Histol. (2021) 52:681–91. doi: 10.1007/s10735-021-09996-8
179. Wang A, Toma MA, Ma J, Li D, Vij M, Chu T, et al. Circular RNA hsa_circ_0084443 is upregulated in diabetic foot ulcer and modulates keratinocyte migration and proliferation. Adv Wound Care (New Rochelle). (2020) 9:145–60. doi: 10.1089/wound.2019.0956
180. Huang Q, Chu Z, Wang Z, Li Q, Meng S, Lu Y, et al. circCDK13-loaded small extracellular vesicles accelerate healing in preclinical diabetic wound models. Nat Commun. (2024) 15:3904. doi: 10.1038/s41467-024-48284-3
181. Meng F, Shen F, Chu X, Ling H, Qiao Y, Liu D. Hsa_circ_0008500 inhibits apoptosis of adipose-derived stem cells under high glucose through hsa-miR-1273h-5p/ELK1 axis. Environ Toxicol. (2023) 38:1732–42. doi: 10.1002/tox.23801
182. Meng F, Shen F, Ling H, Jin P, Zhou D, Li Q. CircARHGAP12 Triggers Mesenchymal Stromal Cell Autophagy to Facilitate its Effect on Repairing Diabetic Wounds by Sponging miR-301b-3p/ATG16L1 and miR-301b-3p/ULK2. J Invest Dermatol. (2022) 142:1976–89.e4. doi: 10.1016/j.jid.2021.11.039
183. Chen J, Li X, Liu H, Zhong D, Yin K, Li Y, et al. Bone marrow stromal cell-derived exosomal circular RNA improves diabetic foot ulcer wound healing by activating the nuclear factor erythroid 2-related factor 2 pathway and inhibiting ferroptosis. Diabetes Med. (2023) 40:e15031. doi: 10.1111/dme.15031
184. Liang Z-H, Lin S-S, Pan N-F, Zhong G-Y, Qiu Z-Y, Kuang S-J, et al. UCMSCs-derived exosomal circHIPK3 promotes ulcer wound angiogenesis of diabetes mellitus via miR-20b-5p/Nrf2/VEGFA axis. Diabetes Med. (2023) 40:e14968. doi: 10.1111/dme.14968
185. Yang Z-G, Awan FM, Du WW, Zeng Y, Lyu J, Wu D, et al. The circular RNA interacts with STAT3, increasing its nuclear translocation and wound repair by modulating Dnmt3a and miR-17 function. Mol Ther. (2017) 25:2062–74. doi: 10.1016/j.ymthe.2017.05.022
186. Groppa E, Colliva A, Vuerich R, Kocijan T, Zacchigna S. Immune cell therapies to improve regeneration and revascularization of non-healing wounds. Int J Mol Sci. (2020) 21:5235. doi: 10.3390/ijms21155235
187. Song J, Hu L, Liu B, Jiang N, Huang H, Luo J, et al. The emerging role of immune cells and targeted therapeutic strategies in diabetic wounds healing. J Inflammation Res. (2022) 15:4119–38. doi: 10.2147/JIR.S371939
188. Zhao X, Zhong Y, Wang X, Shen J, An W. Advances in circular RNA and its applications. Int J Med Sci. (2022) 19:975–85. doi: 10.7150/ijms.71840
189. Scheideler M, Vidakovic I, Prassl R. Lipid nanocarriers for microRNA delivery. Chem Phys Lipids. (2020) 226:104837. doi: 10.1016/j.chemphyslip.2019.104837
190. Pandey S, Shaif M, Ansari TM, Shamim A, Kushwaha P. Leveraging potential of nanotherapeutics in management of diabetic foot ulcer. Exp Clin Endocrinol Diabetes. (2022) 130:678–86. doi: 10.1055/a-1749-4909
191. Wonnacott A, Denby L, Coward RJM, Fraser DJ, Bowen T. MicroRNAs and their delivery in diabetic fibrosis. Adv Drug Delivery Rev. (2022) 182:114045. doi: 10.1016/j.addr.2021.114045
192. Jiang G, Jiang T, Chen J, Yao H, Mao R, Yang X, et al. Mitochondrial dysfunction and oxidative stress in diabetic wound. J Biochem Mol Toxicol. (2023) 37:e23407. doi: 10.1002/jbt.23407
193. Qadir MMF, Klein D, Álvarez-Cubela S, Domínguez-Bendala J, Pastori RL. The role of microRNAs in diabetes-related oxidative stress. Int J Mol Sci. (2019) 20:5423. doi: 10.3390/ijms20215423
194. Liu Y, Zhang X, Yang L, Zhou S, Li Y, Shen Y, et al. Proteomics and transcriptomics explore the effect of mixture of herbal extract on diabetic wound healing process. Phytomedicine. (2023) 116:154892. doi: 10.1016/j.phymed.2023.154892
195. Yan Y, Liu X-Y, Lu A, Wang X-Y, Jiang L-X, Wang J-C. Non-viral vectors for RNA delivery. J Control Release. (2022) 342:241–79. doi: 10.1016/j.jconrel.2022.01.008
196. Yang X, Zhang L, Yang Y, Schmid M, Wang Y. miRNA mediated regulation and interaction between plants and pathogens. Int J Mol Sci. (2021) 22:2913. doi: 10.3390/ijms22062913
197. Umehara T, Mori R, Mace KA, Murase T, Abe Y, Yamamoto T, et al. Identification of Specific miRNAs in Neutrophils of Type 2 Diabetic Mice: Overexpression of miRNA-129-2-3p Accelerates Diabetic Wound Healing. Diabetes. (2019) 68:617–30. doi: 10.2337/db18-0313
198. Amin KN, Umapathy D, Anandharaj A, Ravichandran J, Sasikumar CS, Chandra SKR, et al. miR-23c regulates wound healing by targeting stromal cell-derived factor-1α (SDF-1α/CXCL12) among patients with diabetic foot ulcer. Microvasc Res. (2020) 127:103924. doi: 10.1016/j.mvr.2019.103924
199. Cao M, Duan Z, Wang X, Gong P, Zhang L, Ruan B. Curcumin promotes diabetic foot ulcer wound healing by inhibiting miR-152-3p and activating the FBN1/TGF-β Pathway. Mol Biotechnol. (2024) 66:1266–78. doi: 10.1007/s12033-023-01027-z
200. Icli B, Nabzdyk CS, Lujan-Hernandez J, Cahill M, Auster ME, Wara AKM, et al. Regulation of impaired angiogenesis in diabetic dermal wound healing by microRNA-26a. J Mol Cell Cardiol. (2016) 91:151–9. doi: 10.1016/j.yjmcc.2016.01.007
201. Hu K, Liu L, Tang S, Zhang X, Chang H, Chen W, et al. MicroRNA-221-3p inhibits the inflammatory response of keratinocytes by regulating the DYRK1A/STAT3 signaling pathway to promote wound healing in diabetes. Commun Biol. (2024) 7:300. doi: 10.1038/s42003-024-05986-0
202. Xu Y, Ouyang L, He L, Qu Y, Han Y, Duan D. Inhibition of exosomal miR-24-3p in diabetes restores angiogenesis and facilitates wound repair via targeting PIK3R3. J Cell Mol Med. (2020) 24:13789–803. doi: 10.1111/jcmm.15958
203. Lou R, Chen J, Zhou F, Zhang T, Chen X, Wang C, et al. Exosomal miRNA-155-5p from M1-polarized macrophages suppresses angiogenesis by targeting GDF6 to interrupt diabetic wound healing. Mol Ther Nucleic Acids. (2023) 34:102074. doi: 10.1016/j.omtn.2023.102074
204. Huang H, Zhu W, Huang Z, Zhao D, Cao L, Gao X. Adipose-derived stem cell exosome NFIC improves diabetic foot ulcers by regulating miR-204-3p/HIPK2. J Orthop Surg Res. (2023) 18:687. doi: 10.1186/s13018-023-04165-x
205. Wang S, Shi M, Zhou J, Wang W, Zhang Y, Li Y. Circulating Exosomal miR-181b-5p Promoted Cell Senescence and Inhibited Angiogenesis to Impair Diabetic Foot Ulcer via the Nuclear Factor Erythroid 2-Related Factor 2/Heme Oxygenase-1 Pathway. Front Cardiovasc Med. (2022) 9:844047. doi: 10.3389/fcvm.2022.844047
206. Li B, Luan S, Chen J, Zhou Y, Wang T, Li Z, et al. The MSC-Derived Exosomal lncRNA H19 Promotes Wound Healing in Diabetic Foot Ulcers by Upregulating PTEN via MicroRNA-152-3p. Mol Ther Nucleic Acids. (2020) 19:814–26. doi: 10.1016/j.omtn.2019.11.034
207. He Z-Y, Huang M-T, Cui X, Zhou S-T, Wu Y, Zhang P-H, et al. Long noncoding RNA GAS5 accelerates diabetic wound healing and promotes lymphangiogenesis via miR-217/Prox1 axis. Mol Cell Endocrinol. (2021) 532:111283. doi: 10.1016/j.mce.2021.111283
Keywords: diabetic wound, diabetic foot ulcer, non-coding RNA, microRNA, long non-coding RNA, circular RNA
Citation: Aghayants S, Zhu J, Yu J, Tao R, Li S, Zhou S, Zhou Y and Zhu Z (2024) The emerging modulators of non-coding RNAs in diabetic wound healing. Front. Endocrinol. 15:1465975. doi: 10.3389/fendo.2024.1465975
Received: 17 July 2024; Accepted: 23 September 2024;
Published: 08 October 2024.
Edited by:
Johnson Boey, National University Hospital, SingaporeReviewed by:
Zheng Chunlan, Wuhan Institute for Tuberculosis Control, ChinaJun Zhang, Shenzhen Shekou People’s Hospital, China
Xian Zhang, Southeast University, China, in collaboration with reviewer JZ
Copyright © 2024 Aghayants, Zhu, Yu, Tao, Li, Zhou, Zhou and Zhu. This is an open-access article distributed under the terms of the Creative Commons Attribution License (CC BY). The use, distribution or reproduction in other forums is permitted, provided the original author(s) and the copyright owner(s) are credited and that the original publication in this journal is cited, in accordance with accepted academic practice. No use, distribution or reproduction is permitted which does not comply with these terms.
*Correspondence: Yunhua Zhou, MjA0MjI1Njk1NEBxcS5jb20=; Zhanyong Zhu, enl6aHVAd2h1LmVkdS5jbg==
†These authors have contributed equally to this work and share first authorship