- 1Institute for Fetology, First Affiliated Hospital of Soochow University, Suzhou, China
- 2Key Laboratory of Birth Regulation and Control Technology of National Health Commission of China, Shandong Provincial Maternal and Child Health Care Hospital Affiliated to Qingdao University, Jinan, China
- 3Genetics and Prenatal Diagnosis Center, Fuyang People’s Hospital, Fuyang, China
- 4Department of Oncology and Hematology, Suzhou Hospital, Affiliated Hospital of Medical School, Nanjing University, Suzhou, Jiangsu, China
Glucocorticoids (GCs) are steroid hormones fundamental to the body’s normal physiological functions and are pivotal in fetal growth and development. During gestation, the mother’s cortisol concentration (active GCs) escalates to accommodate the requirements of fetal organ development and maturation. A natural placental GCs barrier, primarily facilitated by 11β hydroxysteroid dehydrogenase 2, exists between the mother and fetus. This enzyme transforms biologically active cortisol into biologically inactive corticosterone, thereby mitigating fetal GCs exposure. However, during pregnancy, the mother may be vulnerable to adverse factor exposures such as stress, hypoxia, caffeine, and synthetic GCs use. In these instances, maternal serum GCs levels may surge beyond the protective capacity of the placental GCs barrier. Moreover, these adverse factors could directly compromise the placental GCs barrier, resulting in excessive fetal exposure to GCs. It is well-documented that prenatal GCs exposure can detrimentally impact the offspring’s cardiovascular system, particularly in relation to blood pressure, vascular function, and heart function. In this review, we succinctly delineate the alterations in GCs levels during pregnancy and the potential mechanisms driving these changes, and also analyze the possible causes of prenatal GCs exposure. Furthermore, we summarize the current advancements in understanding the adverse effects and mechanisms of prenatal GCs exposure on the offspring’s cardiovascular system.
1 Introduction
Glucocorticoids (GCs) are critical stress hormones involved in various fundamental processes, including metabolic homeostasis, cognition, and development (1, 2). They exhibit potent anti-inflammatory and immunosuppressive effects, making synthetic GCs such as dexamethasone and betamethasone, a common clinical treatment for a broad spectrum of autoimmune, inflammatory, and allergic diseases (2, 3). GCs not only serve as effective therapeutic agents for numerous diseases but also function as essential steroid hormones for maintaining the body’s normal physiological functions, particularly for fetal growth and development (4, 5). During the second and third trimester, endogenous GCs can stimulate the development and maturation of fetal organs such as the lungs and kidneys (5). This process can also be induced prematurely by exogenous GCs. Consequently, GCs are frequently administered clinically to pregnant women at risk of preterm labor to decrease the mortality rate among premature infants.
Despite the significant role of GCs in treating various diseases and maintaining physiological functions, chronic or excessive exposure to GCs has been linked to an increased risk of cardiovascular diseases (6, 7). GCs exert direct effects on the heart and blood vessels, mediated by glucocorticoid and mineralocorticoid receptors and modulated by the local metabolism of GCs via the enzyme 11β-hydroxysteroid dehydrogenase (11βHSD). This direct impact on the cardiovascular system can impair vascular and cardiac functions and potentially resulting in cardiovascular diseases (8).
Recent findings suggest that preterm infants treated prenatally with betamethasone (synthetic GCs) exhibit a transient physiological peak in circulating GCs bioactivity (9). This implies that prenatal exposure to GCs may lead to elevated fetal circulating cortisol concentrations, potentially exerting long-term effects on the cardiovascular system of the developing fetus. When there is an excessive amount of active GCs in the fetal blood circulation, it is bound to affect the development and function of the fetal cardiovascular system, and further lay the foundation for the occurrence of cardiovascular system health problems after birth.
Increasing evidence now suggests that many adult cardiovascular diseases may be linked to intrauterine growth and development (10, 11). The theory that adult cardiovascular diseases have fetal origins has been extensively corroborated by numerous studies over the past two decades (11, 12). Recently, due to the rising prevalence of prenatal GCs exposure, many researchers have sought to investigate the long-term effects of prenatal GCs exposure on the cardiovascular system of offspring (13–15). Studies have demonstrated that excessive prenatal GCs exposure can negatively impact the cardiovascular system of both the fetus and the adult offspring, particularly in relation to blood pressure, vascular function, and cardiac development (14–16). This review primarily focuses on the detrimental effects and mechanisms associated with prenatal GCs exposure on the cardiovascular system of the offspring, as well as potential contributing factors to prenatal GCs exposure and their mechanisms.
2 GCs synthesis and changes during pregnancy
GCs are synthesized in the adrenal cortex through the enzymatic processing of cholesterol (17), with cortisol (also known as hydrocortisone) accounting for 90% of the total. GCs secretion can be categorized into basal secretion, which occurs under normal physiological conditions, and stress secretion, which occurs in response to stress. Both types of secretion are regulated by the hypothalamic-pituitary-adrenocortical (HPA) axis. In response to psychological or physiological stress, neurons in the paraventricular nucleus (PVN) of the hypothalamus synthesize corticotropin-releasing hormone (CRH) and release it into the pituitary portal vein (18). This stimulates the anterior pituitary gland to release adrenocorticotropic hormone (ACTH) into the bloodstream, which in turn triggers the synthesis and secretion of GCs from the adrenocortical zona fasciculata (19, 20). As a fat-soluble steroid hormone, GCs can easily penetrate the cell membrane and bind to the cytoplasmic glucocorticoid receptor (GR). The GR then translocates to the nucleus and binds to the glucocorticoid response element (GRE) in the target region, thereby regulating the transcription and translation of target genes and eliciting corresponding gene effects (Figure 1) (21, 22). Cortisol, a steroid hormone crucial for early gestational pregnancy establishment (23, 24), also plays a vital role in fetal development. A significant increase in fetal serum GCs levels before birth is essential for the normal development of fetal lungs and other organs (25). Studies have demonstrated a substantial rise in both total cortisol and biologically active plasma free cortisol concentrations in pregnant women (26, 27).
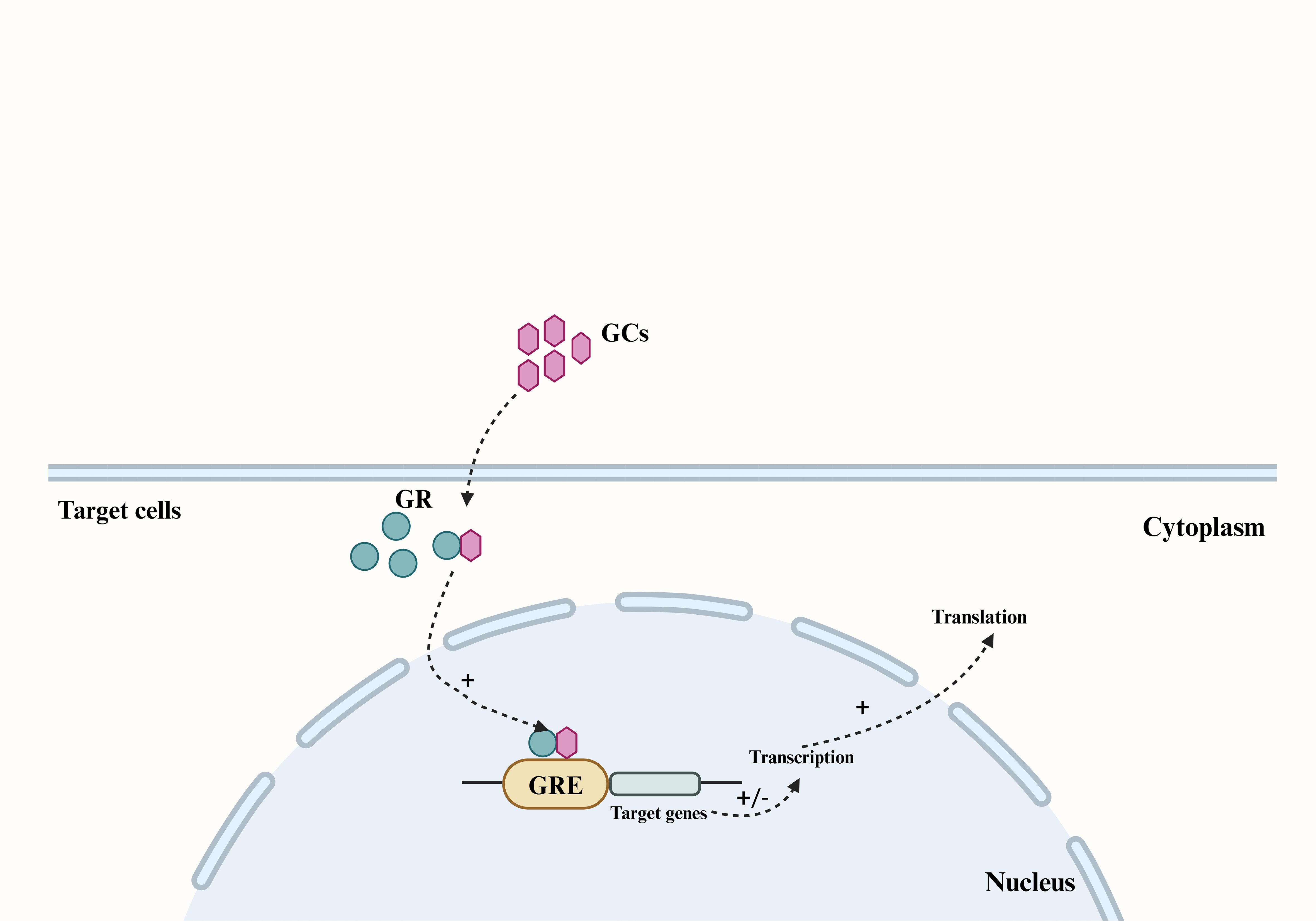
Figure 1. The main pathway by which GCs produce genetic effects in their target cells. The fat-soluble steroid hormones GCs can penetrate the cell membrane of target cells and bind to the GR in the cytoplasm, and cause the GR to translocate to the nucleus and bind to the GRE in the target region, thereby regulating the transcription and translation of the target gene. GCs, Glucocorticoids; GR, glucocorticoid receptor; GRE, glucocorticoid response element.
3 Placental GCs barrier
The human placenta, is characterized by the direct interface between maternal blood circulation and placental villi. These villi, the site of nutrient and gas exchange between the mother and fetus, are enveloped by two layers of trophoblasts (28). The syncytiotrophoblast, one of these layers, is situated in the intervillous space of the chorionic villi and serves as the primary defense line, shielding the fetus from potentially harmful maternal substances (28). Although the fetal HPA axis can secrete a minimal amount of cortisol in late pregnancy, approximately 50% of fetal cortisol originates from the mother via placental blood circulation. The maternal endogenous cortisol concentration during pregnancy is 5-10 times higher than that in the fetus. This concentration gradient is largely maintained by the placental GCs barrier, which is reinforced by 11β-hydroxysteroid dehydrogenase 2 (11βHSD2) (28). 11βHSD2, a glucocorticoid-inactivating enzyme, has a high affinity for cortisol and can convert biologically active cortisol into inactive cortisone, thereby protecting the fetus from the detrimental effects of maternal GCs overdose (Figure 2) (28). 11βHSD2 is present on the surface of the chorionic villi early in pregnancy and plays a crucial role in safeguarding embryonic development (28). As fetal age increases, the activity and expression of 11βHSD2 rise correspondingly until late gestation when it starts to decline (29, 30).
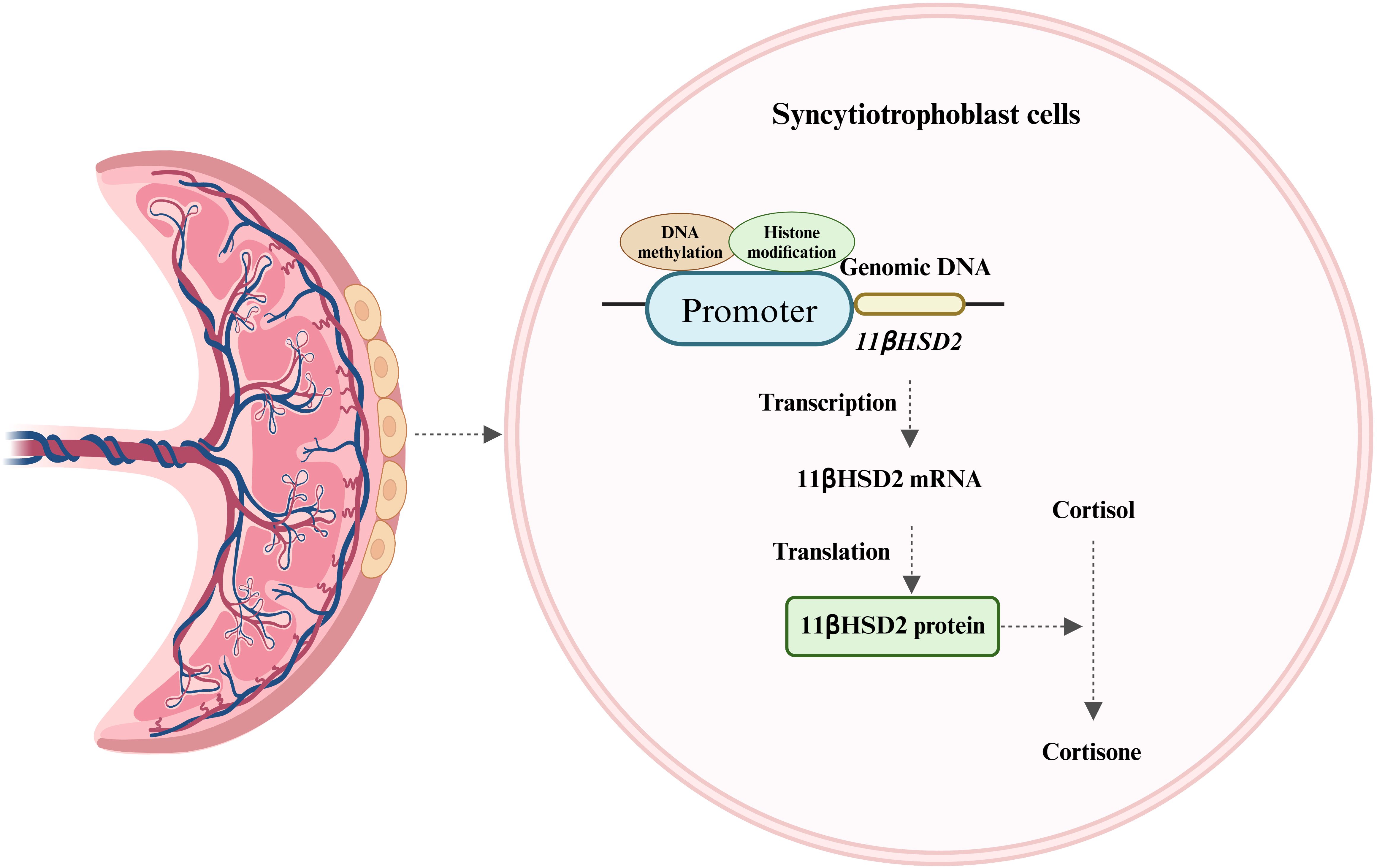
Figure 2. Expression of 11βHSD2 and its major role. 11βHSD2, expressed in the syncytiotrophoblast of placental villi, converts biologically active cortisol to inactive cortisone and is regulated by DNA methylation and histone modifications.
It is currently understood that the expression of 11βHSD2 is strictly regulated by several mechanisms, including DNA methylation and histone modification. The methylation pattern within the promoter of the 11βHSD2 gene is established during trophoblast fate determination (28). Rasoul, et al. reported that the methylation level of the CpG island in the promoter is a determinant of whether 11βHSD2 gene expression is repressed (31). Research has also demonstrated that the regulation of the 11βHSD2 gene is influenced by human chorionic gonadotropin (hCG) (32, 33). The specific mechanism involves hCG triggering the cAMP/PKA pathway, which in turn inactivates the PRB-e2F1-EZH2 pathway. This sequence of events results in a decrease in histone H3 lysine 27 methylation and an increase in acetylation levels at the 11βHSD2 gene promoter region. Consequently, the transcription factor specificity protein 1 (Sp1) can bind to the 11βHSD2 gene promoter, thereby enhancing its transcription (32, 33). Furthermore, studies have indicated that the activity of placental 11βHSD2 can be affected by various factors (34, 35). For instance, elevated levels of cortisol in the maternal environment during pregnancy or the prenatal use of synthetic GCs can diminish 11βHSD2 activity (9).
Given the crucial role of 11βHSD2 in the placental GCs barrier and in safeguarding the fetus from the risks of excessive GCs exposure, even minor alterations in its expression and activity can have detrimental effects on fetal development. Therefore, the mechanisms regulating the expression and activity of 11βHSD2 in the placenta warrant further investigation and clarification.
4 Possible causes of prenatal GCs exposure
During pregnancy, the placental GCs barrier provides a degree of protection to the fetus from maternal excess GCs. However, under certain exceptional circumstances, such as impaired placental barrier or maternal exposure to high levels of GCs, the fetus can be adversely affected by GCs. Prenatal GCs exposure can occur via two primary pathways: endogenous and exogenous. The endogenous pathway primarily results from overproduction of GCs and impairment of the placental GCs barrier, while the exogenous pathway involves the use of synthetic GCs, typically for maternal immune disorders and to prevent complications in preterm infants (Figure 3).
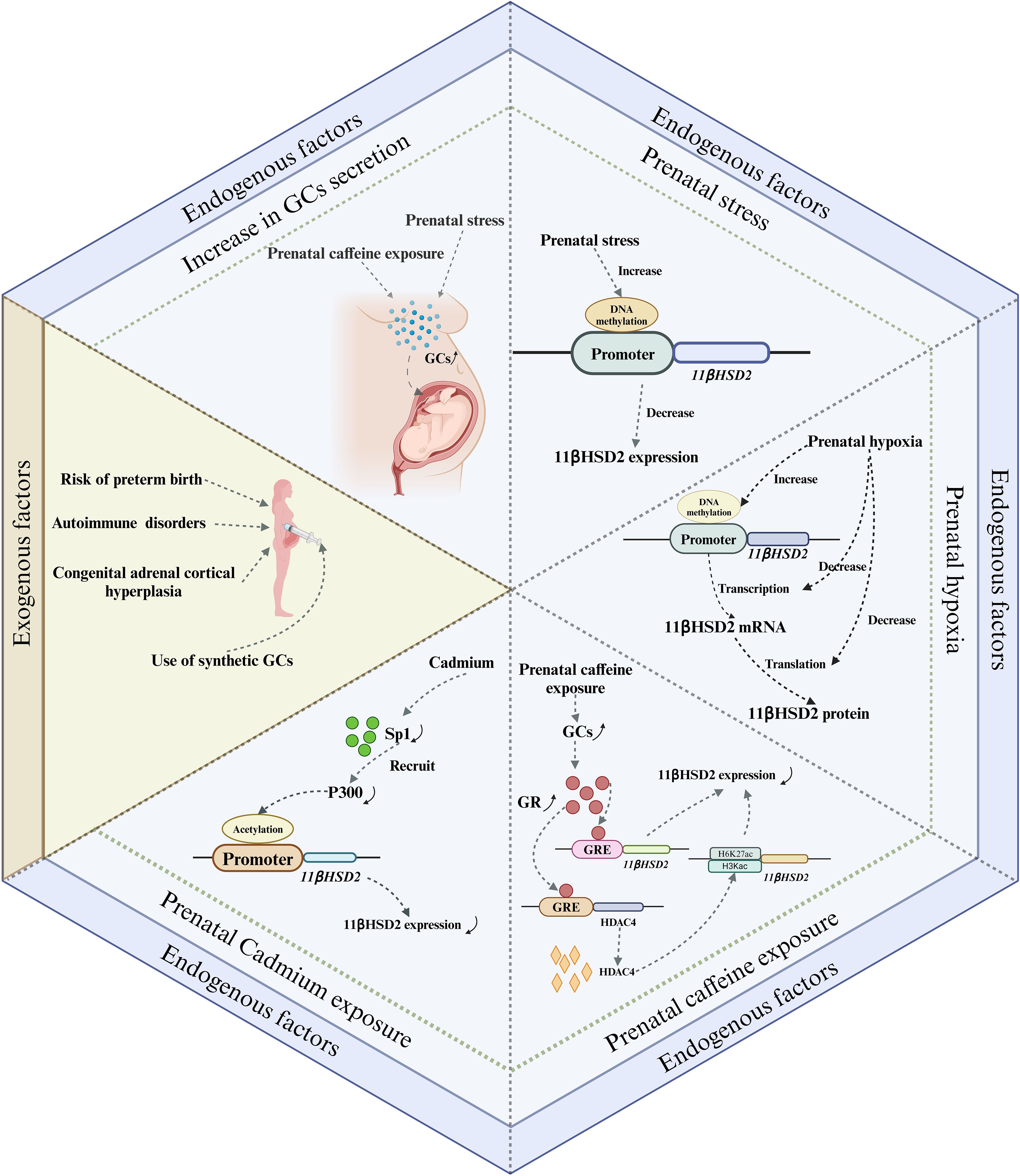
Figure 3. Major pathways of prenatal GCs exposure and their possible mechanisms. There are two main endogenous and exogenous routes of prenatal fetal exposure to GCs. The endogenous pathway is mainly due to overproduction of GCs and impaired placental GCs barrier, whereas the exogenous pathway is mainly due to the use of synthetic GCs, which are commonly used for the treatment of maternal immune disorders and the prevention of preterm birth complications. Among these, impairment of the placental GCs barrier caused by exposure to adverse factors occurs mainly by affecting the expression of 11βHSD2. GCs, Glucocorticoids; GR, glucocorticoid receptor; GRE, glucocorticoid response element.
4.1 Endogenous factors
4.1.1 Increase in GCs secretion
GCs are the final product of the HPA axis. Any disruption to the HPA axis can potentially lead to an abnormal surge in the body’s GCs secretion. This, in turn, may result in the fetus being exposed to excessive GCs. Maternal plasma cortisol levels are known to escalate and reach their peak during late gestation, with serum cortisol levels tripling compared to those in non-pregnant women. Concurrently, the concentrations of placental 11βHSD2 decrease in late pregnancy. This reduction allows more cortisol to permeate the placenta (36), thereby increasing the fetus’s susceptibility to excessive GCs during late gestation. Therefore, from a physiological perspective, late pregnancy presents a critical window for fetal exposure to cortisol. In addition to physiological factors, exposure to adverse prenatal conditions can also contribute to elevated maternal GCs secretion. Prenatal stress, for instance, has been shown to increase serum corticosterone concentrations of pregnant rats (37, 38). In a study by Takahashi et al., it was found that the rise in plasma corticosterone concentrations in pregnant rats, induced by prenatal stress, primarily occurred 24 and 48 hours post-stress exposure. This increase was accompanied by a significant decrease in maternal steroid-binding globulin levels, suggesting that stress primarily triggers an increase in the circulating levels of free corticosterone in vivo (39). In addition, Ge et al. reported that prenatal exposure to caffeine also increased maternal serum, fetal serum and placental corticosterone levels, which may be due to epigenetic regulation of the RYR/JNK/YB-1 pathway affecting cortisol efflux function (40). In conclusion, an increase in GCs secretion, driven by a combination of physiological and pathological factors, is the primary cause of fetal exposure to excessive GCs.
4.1.2 Impaired placental GCs barrier
4.1.2.1 Prenatal stress
Both human and animal studies indicate that prenatal acute and chronic stress may differentially impact the expression of 11βHSD2 in the placenta. Acute stress appears to stimulate placental 11βHSD2 expression (32, 41), whereas chronic stress seems to inhibit it (42–45). The up-regulation of placental 11βHSD2 expression in response to acute stress may represent an immediate protective mechanism by the fetus to counteract a sudden surge in maternal GCs. Conversely, the suppression of placental 11βHSD2 expression under chronic stress may serve as a survival strategy for the fetus, given that chronic stress often predisposes to preterm labor (46). This inhibition allows more cortisol to permeate the fetal circulation, thereby expediting the maturation of crucial fetal organs to ensure survival. However, this survival strategy is a double-edged sword, as it results in growth restriction and consequently elevates the risk of chronic disease development later in life. Currently, while the mechanism through which acute stress stimulates placental 11βHSD2 expression remains elusive, emerging evidence suggests that the down-regulation of placental 11βHSD2 expression induced by chronic stress may be associated with DNA methylation (47–49). For instance, Catherine et al. revealed that prenatal stress increased DNA methylation at specific CpG sites within the 11βHSD2 gene promoter in the placenta (48). Although the detailed mechanisms by which chronic stress during pregnancy leads to high DNA methylation of the 11βHSD2 gene are not clear, increased expression of DNA methyltransferases may be involved in this process (48).
4.1.2.2 Prenatal hypoxia
Prenatal hypoxia, a common pregnancy complication, is often induced by a variety of factors including maternal, placental, and fetal conditions. This condition can disrupt the placental GCs barrier by impacting 11βHSD2 expression (50, 51). A murine study demonstrated that maternal hypoxia during mid- to late-gestation not only modified placental morphology but also resulted in reduced expressions of 11βHSD2 and GR in the placenta (50, 52). Similarly, human studies have reported a reduction in placental 11βHSD2 expression during fetal asphyxia in late-stage intrauterine growth restriction pregnancies (51). In vitro studies have corroborated these findings, showing inhibited induction of 11βHSD2 when cells are exposed to hypoxic conditions (53, 54). Concurrently, both maternal and fetal plasma cortisol levels, as well as the ratio of fetal to maternal plasma cortisol levels, were found to be elevated in cases of prenatal hypoxia (52). This suggests that prenatal hypoxia suppresses 11βHDS2 expression and enhances the rate of maternal cortisol crossing the placenta. Additionally, the abundance of 11βHSD2 mRNA was also diminished in placentas affected by prenatal hypoxia, indicating that the reduction in 11βHSD2 expression may be associated with transcriptional inactivation. This implies that prenatal hypoxia may reprogram the expression of the 11βHSD2 gene through DNA methylation (52).
4.1.2.3 Prenatal caffeine exposure
Caffeine is widely found in coffee, tea, soft drinks, foods and medications, and it is also very common today for women to consume caffeine during pregnancy. Caffeine, due to its high fat solubility, can traverse the placental barrier and enter the fetus. Its slow metabolism and excretion within the fetus can lead to its accumulation, potentially causing detrimental effects on fetal health. One such effect is the impairment of the placental GCs barrier. This may be attributed to caffeine’s inhibitory effect on the expression of 11βHSD2 in the placenta (55), resulting in an excess of corticosterone. This, in turn, leads to elevated levels of circulating corticosterone in the fetus, thereby increasing the risk of various chronic diseases in the offspring (56–58). Currently, the exact mechanism through which prenatal caffeine exposure inhibits placental 11βHSD2 expression remains unclear. However, emerging evidence points towards the role of epigenetic modifications (59, 60).
4.1.2.4 Exposure to other adverse prenatal factors
In recent years, studies have found that exposure to heavy metals during pregnancy can also damage the barrier function of the placental GCs (61, 62). For example, prenatal exposure to cadmium disrupts the placental GCs barrier, leading to fetal exposure to high levels of cortisol, ultimately affecting the health of newborns and their offspring (61, 62). Fan et al. found that prenatal cadmium exposure can affect the binding of P300 and 11βHSD2 gene promoter regions by reducing Sp1 expression, thereby reducing acetylation modifications in the 11βHSD2 gene promoter region, and subsequently inhibiting the expression of 11βHSD2 (61). Chen et al. speculated that prenatal cadmium exposure inhibits placental 11βHSD2 expression by downregulating the cAMP/PKA/Sp1 signaling pathway (62).
In addition to the above adverse factors, recent studies have found that prenatal nicotine exposure and alcohol consumption have also differentially affected the function of the placental GCs barrier to varying degrees (62, 63). For example, Chen et al. found that prenatal nicotine exposure can lead to elevated maternal serum corticosterone levels, and significantly reduced placental 11βHSD2 mRNA levels (63). Liang et al. established a prenatal alcohol exposure model in pregnant mice and found that after prenatal alcohol exposure, serum corticosterone levels significantly increased, and placental 11βHSD2 expression decreased (64). Furthermore, it is discovered that the nutritional status of the mother during pregnancy can also impact the expression of placental 11βHSD2. Generally, excessive nutrition or obesity during pregnancy results in a reduction in placental 11βHSD2 expression (65, 66), while malnutrition has no effect on the expression of 11βHSD2 in the placenta (67). Currently, although a large quantity of studies has indicated that many prenatal adverse factors lead to a decrease in the expression of placental 11βHSD2 (Table 1), the underlying mechanism remains unclear, and further research and clarification are urgently required in the future.
In contrast to the function of 11βHSD2, 11βHSD1 has the ability to convert inactive GCs into active GCs, and 11βHSD1 and 11βHSD2 jointly regulate the level of active GCs in the local tissues of the fetus. In the fetal cardiovascular system, once there is any abnormality in the expression or activity of 11βHSD1/2, it will cause a change in the level of active GCs within the cardiovascular system, and then have a long-term influence on the development of the fetal cardiovascular system and the health of the offspring’s cardiovascular system. At present, although there are relatively numerous relevant research reports on the influence of prenatal adverse factors on the expression or activity of 11βHSD1/2 in fetal tissues (68–70), the research on the fetal cardiovascular system is rather limited. Hence, this review did not carry out a detailed overview of the research progress in this aspect.
4.2 Exogenous factors
The main route of prenatal exogenous GCs exposure is the use of synthetic GCs. GCs play a crucial role in promoting fetal organ maturation. Particularly in pregnant women threatened with preterm labor, synthetic GCs are widely used to accelerate lung maturation, thereby greatly improving the survival of preterm neonates (64). In addition, GCs use will be unavoidable when the mother has an autoimmune disease, such as connective tissue disease. What’s more, in order to suppress the overproduction of adrenal androgens in the fetuses of pregnant women at high risk of congenital adrenocortical hyperplasia, small doses of dexamethasone are usually given to pregnant women in the early stages of gestation in clinical treatment, which is effective in reducing the male genitalia of fetuses whose mother with congenital adrenocortical hyperplasia (71, 72). In conclusion, the clinical application of antenatal GCs has inevitable characteristics, especially for premature infants. Although they can promote lung maturation in premature fetuses, increase the rate of live births, and reduce the incidence of neonatal respiratory distress syndrome, their use also has long-term adverse effects on maternal and infant health.
5 Effects of prenatal GCs on offspring cardiac functions
Currently, a considerable amount of research indicates that prenatal GCs exposure can have long-term adverse effects on the heart development and function of fetuses and offspring (72, 73). For example, Langdown et al. found that offspring exposed to prenatal dexamethasone exhibit disrupted cardiac glucose metabolism, along with significant activation of the Akt/protein kinase B pathway and upregulation of GLUT1 expression in cardiac tissues (74). GLUT1 is a glucose transporter and a major mediator of basal glucose uptake in the heart. They further demonstrated that prenatal dexamethasone exposure upregulates cardiac GLUT1 expression through activation of the Akt/PKB pathway, leading to disrupted cardiac glucose metabolism in offspring (74). Cardiac uncoupling protein (UCP)-mediated metabolic adaptations define cardiac cell function in the heart (75), they found that prenatal GCs exposure also affects the expression of UCP in the hearts of fetal and adult offspring. Male fetuses exposed to prenatal GCs showed significantly increased expression of cardiac UCP2 and UCP3 proteins after birth, however, in adult male offspring exposed to GCs prenatally, the expression of UCP2 and UCP3 proteins was significantly decreased (76). In addition, overexpression of the conserved Ca2+-binding protein calreticulin impairs cardiac function (77). Normally, cardiac calreticulin expression decreases between 2-3 weeks of age and remains suppressed into adulthood. Langdown et al. observed that the impact of prenatal dexamethasone exposure on postnatal cardiac calsequestrin expression is minimal, but the decrease in postnatal cardiac calreticulin expression is eliminated, and adult cardiac calreticulin expression significantly increases (78). Given the known association between excessive cardiac calreticulin expression and impaired cardiac function, upregulation of cardiac calreticulin may increase the risk of adult heart disease due to prenatal overexposure to GCs.
Prenatal GCs exposure was also reported to alter the development of cardiac adrenergic and sympathetic processes in offspring (79). Bian et al. found that rat offspring exposed to prenatal dexamethasone had insufficient and decreased levels of norepinephrine, indicating that prenatal GC exposure can impair the development and activity of the fetal heart sympathetic nerve projections (79). Furthermore, their study also found that prenatal exposure to high doses of GCs interferes with the development of β-adrenergic receptor-mediated cell signaling in the rat offspring heart, enhances dose-dependent stimulation of adenylyl cyclase activity mediated by β-receptors, and increases cardiac adenylyl cyclase response (80). Similar to the effects observed in rodents, Vries et al. found that prenatal dexamethasone exposure can lead to changes in cardiac metabolism and HPA axis function in chlorocebus aethiops offspring (81). In order to further reveal the repair capacity of offspring hearts exposed to prenatal GCs for “secondary hits” such as ischemia-reperfusion (I/R), researchers constructed an I/R model (82, 83). Peng et al. found that compared to the normal group, offspring exposed to GCs prenatally after I/R had more pronounced cardiac dysfunction due to increased apoptosis of myocardial cells. They further found that bone morphogenetic protein 4 (BMP4), which is involved in myocardial cell differentiation and development pathways, was significantly downregulated in myocardial cells of offspring exposed to prenatal GCs, with a significant increase in DNA methylation in the gene promoter region (82). Prenatal GCs exposure inhibited BMP4 expression in offspring myocardial cells, thereby inhibiting the binding activity of the transcription factor HIF-1α induced by myocardial ischemia, weakening the protective effect of BMP4 on myocardial cells, ultimately resulting in more pronounced cardiac dysfunction after I/R (83). These results suggest that excessive prenatal GC exposure increases the susceptibility of offspring hearts to “secondary hits” such as I/R, due to impaired the protective effect of BMP4 on myocardial cells caused by high methylation of the BMP4 promoter region. The application of DNA methylation inhibitors may be a potential therapeutic approach for the cardiac dysfunction in offspring exposed to prenatal GCs (82, 83).
In conclusion, prenatal GCs exposure not only has adverse effects on the myocardium and cardiac function of newborns and offspring, but also leads to pathological changes in myocardial tissue in newborns and offspring (Figure 4, Table 2). For example, offspring exposed to prenatal GCs show a significant decrease in ventricular weight, myocardial cell hypertrophy, and increased collagen deposition (84). Although there is a considerable amount of research in this area currently, it mainly focuses on the description of pathological phenomena. Further research is urgently needed to uncover and elucidate the underlying molecular mechanisms.
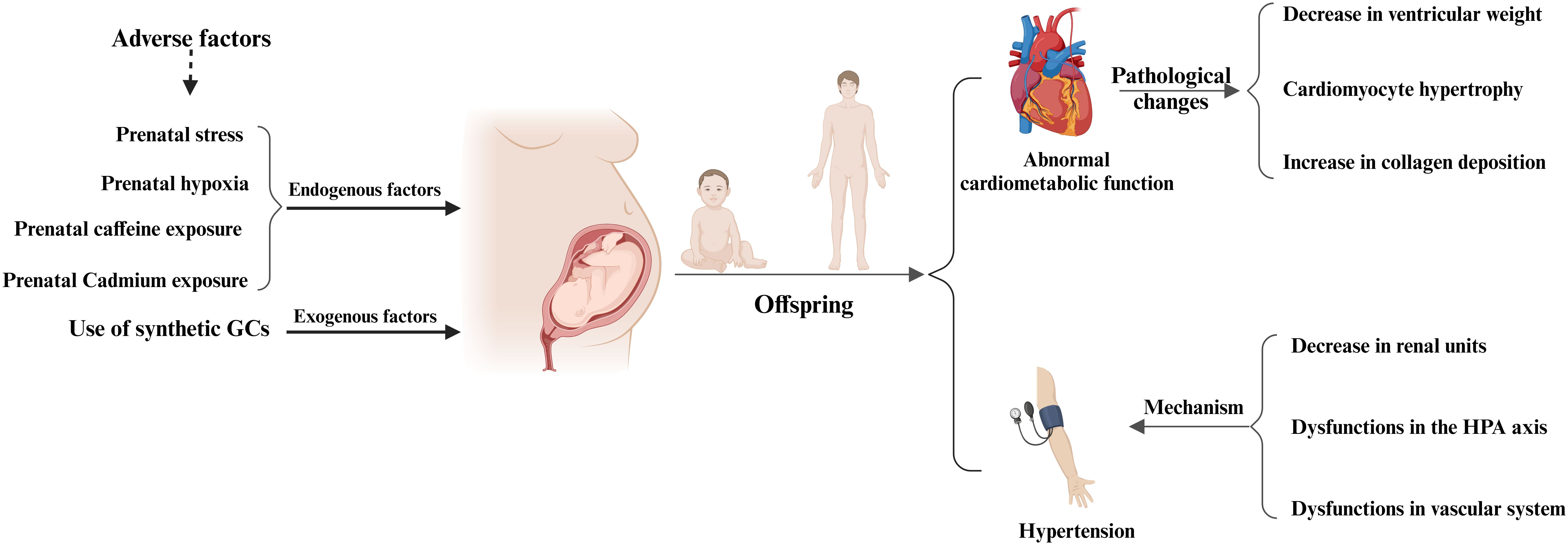
Figure 4. Prenatal glucocorticoids exposure and adverse cardiovascular effects in offspring. Maternal exposure to adverse factors or the use of synthetic GCs during pregnancy can adversely affect the cardiovascular system of the offspring, mainly in the form of abnormal cardiac function in the offspring, pathologic changes in the adult heart, and the development of hypertension in the offspring. Pathologic changes in the adult heart were mainly characterized by a significant decrease in ventricular weight, cardiomyocyte hypertrophy, and increased collagen deposition, whereas the development of hypertension in the offspring was mainly associated with a decrease in renal units, abnormal HPA function, and vascular dysfunction.
6 Effects of prenatal GCs exposure on the offspring vascular system
Currently, a large number of clinical investigations and animal experiments have studied the adverse effects of prenatal GCs exposure on the vascular system of fetal and offspring. In this chapter, we will focus on summarizing the latest research progress on the changes in blood pressure and mechanisms of prenatal GC exposure in fetuses and offspring (Figure 4).
6.1 Clinical cohort follow-up evidences
In clinical practice, GCs are often used prenatally to prevent complications in preterm infants. A large number of clinical follow-up studies have investigated the long-term effects of prenatal GCs therapy on the vascular system of infants, children, and adults (85–87). Mildenhall et al. found that in infants exposed to more than one course of prenatal corticosteroids, 67% had blood pressure above the normal range, while in infants exposed to a single course of corticosteroids, 24% had blood pressure above the normal range (85). Huh et al. tracked nearly 300 premature infants and found a positive correlation between GCs levels in umbilical cord blood at birth and systolic blood pressure at age 3 (86). However, McKinlay et al. found in a follow-up study of 320 premature children that repeated doses of betamethasone exposure prenatally did not increase blood pressure in school-age children compared to a single course of betamethasone exposure (87). Doyle et al. conducted a cohort study of 210 preterm infants and evaluated the relationship between prenatal corticosteroid therapy in preterm infants and blood pressure at age 14. Children exposed to prenatal corticosteroids had higher systolic and diastolic blood pressure compared to children not exposed to corticosteroids (88). This suggests that prenatal corticosteroid therapy is associated with increased systolic and diastolic blood pressure in adolescence and may lead to clinical hypertension after birth. Dessens et al. conducted a randomized controlled trial on subjects receiving GCs and placebo treatment, recording blood pressure in 81 subjects aged 20 (89). They found that subjects who received one course of prenatal corticosteroid therapy had lower average systolic blood pressure (89). Dalziel et al. conducted a 30-year follow-up study and found that offspring exposed to betamethasone prenatally did not have significant differences in blood pressure and cardiovascular disease history compared to the placebo group (90).
In conclusion, current clinical follow-up experimental results indicate that premature infants who have undergone prenatal GCs therapy have significantly higher blood pressure during infancy, childhood, and adolescence compared to the normal age-matched blood pressure range. However, current clinical follow-up cohorts have not found a correlation between prenatal GCs therapy and adult blood pressure. Nevertheless, due to the long follow-up time, small number of participants, heterogeneity, and varying follow-up quality, further research with larger sample sizes and higher quality follow-up cohorts is needed to investigate the relationship between prenatal GCs therapy and adult blood pressure.
6.2 Animal model experimental evidences
The time required for animal studies is much shorter and easier to explore the mechanisms behind diseases compared to clinical studies. Therefore, many animal models of prenatal GCs exposure have been developed, primarily in sheep and rodents. Recent experimental findings suggest that prenatal GCs exposure may result in elevated blood pressure in offspring (91, 92). In a sheep model of prenatal GCs exposure, dexamethasone (0.28 mg/day/kg) was administered from days 22 to 29 of gestation. The sheep offspring exhibited significantly elevated blood pressure from 4 to 19 months of age (93).Another study demonstrated that even a brief exposure to relatively higher levels of cortisol within the normal physiological range in the early stage of gestation (where plasma cortisol concentrations rose from 51 μmol/L to 390 μmol/L) led to increased basal mean arterial pressures in the offspring of sheep, independently of the sex of the offspring (94). Similarly, guinea pig models of prenatal GC exposure showed that prenatal dexamethasone exposure increased mean arterial pressure in mature male guinea pig offspring (16). In the Sprague-Dawley rat model, prenatal dexamethasone exposure also resulted in hypertension in offspring rats at 16 weeks of age (95). Apart from sheep and rodents, prenatal GCs exposure has been found to elevate blood pressure in non-human primates such as baboons (92, 96) and long-tailed black-jawed monkeys (81).
Overall, animal experiments have generally confirmed that prenatal GCs exposure leads to elevated blood pressure in offspring, with a potential sex difference in the susceptibility to GCs exposure: male offspring appear to be more vulnerable compared to females (97). For example, Alhamoud et al. found that adult male offspring of rats treated with prenatal dexamethasone had significantly higher blood pressure compared to the control group, while this effect was not observed in female offspring. Furthermore, protein or albumin excretion was also higher in the male offspring of the prenatal dexamethasone-treated group. These results suggest a sex difference in the impact of prenatal dexamethasone exposure on offspring blood pressure, with male offspring being more susceptible (97). Similarly, Khurana et al. conducted a study using a rat model and confirmed the presence of a sex difference in the effects of prenatal GCs exposure on offspring blood pressure. Male offspring exposed to prenatal GCs showed a more significant increase in blood pressure compared to female offspring (98).
Interestingly, the timing of prenatal GCs exposure also played a role in the development of hypertension in offspring. Exposure to dexamethasone during days 26 to 28 of gestation led to elevated basal mean arterial pressure in sheep offspring (93, 99), while exposure late in gestation did not have a significant effect (100). In addition, the effect of prenatal GCs exposure on offspring blood pressure appears to be related to the dose of GCs exposure. Prenatal exposure to a low dose of dexamethasone (10 μg/kg/d) did not significantly impact blood pressure in female mice offspring (98). These findings suggest that there is a threshold for the effects of GCs on offspring blood pressure, and using low doses may have a lesser impact on the offspring. Therefore, when using synthetic GCs for prenatal treatment, it is important to consider both the amount and duration of their use.
6.3 Mechanism of elevated blood pressure in prenatal GCs-exposed offspring
It is known that the occurrence of hypertension is closely related to impaired kidney development, impaired vascular structure and function, as well as abnormalities in the HPA axis. In this chapter, we will focus on summarizing the molecular mechanisms by which prenatal GCs exposure leads to elevated blood pressure in offspring (Table 3).
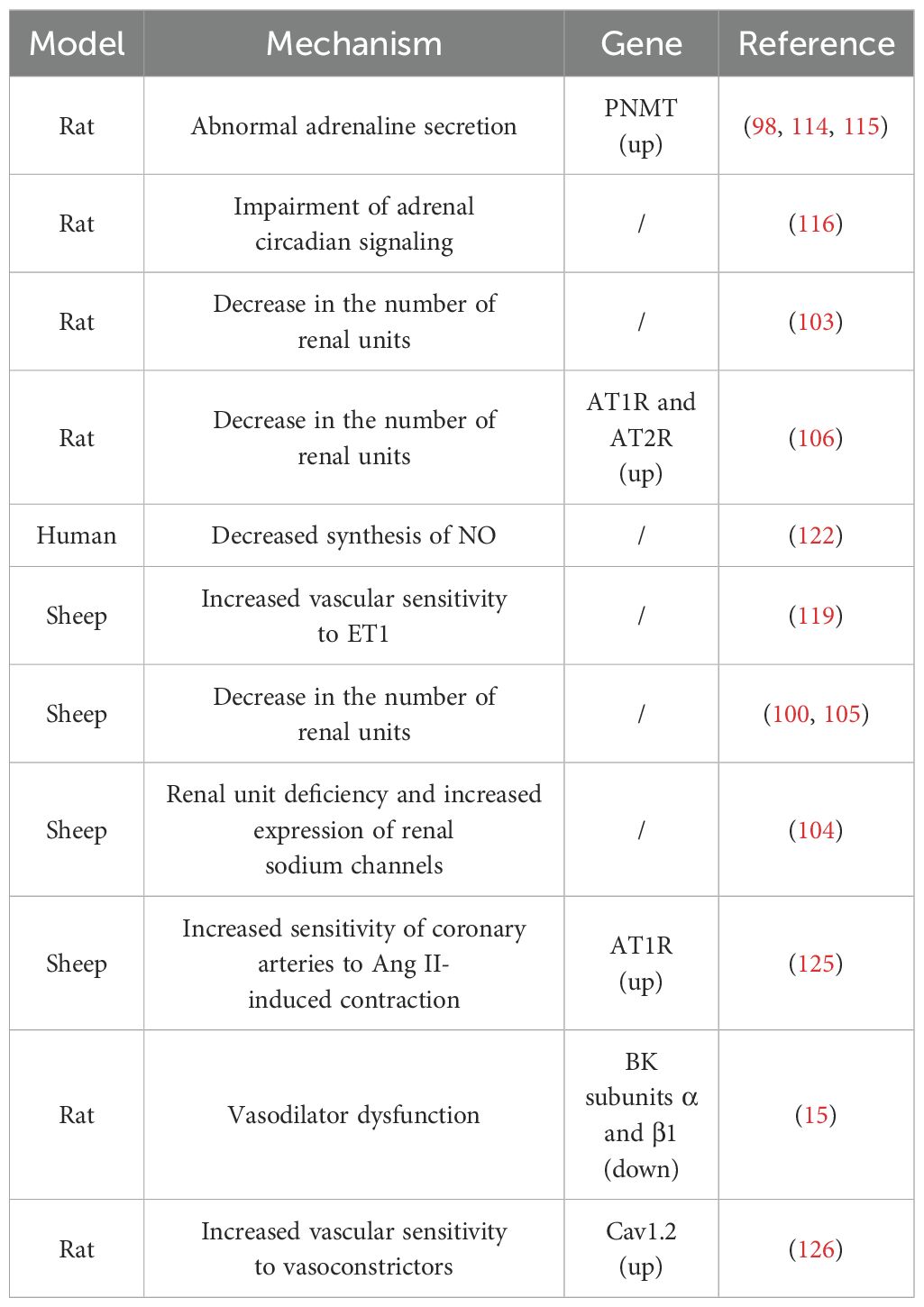
Table 3. Possible mechanisms and associated genes for elevated blood pressure in offspring due to prenatal GC exposure.
6.3.1 Decreases in renal units
There is mounting evidence that a reduced number of renal units at birth significantly contributes to adult susceptibility to hypertension (101, 102). Ortiz et al. observed that the peak postpartum blood pressure in rat offspring, due to prenatal GCs exposure, corresponded with the period of maximum reduction in renal units (103). A study on prenatal dexamethasone-exposed sheep offspring revealed that prenatal exposure to dexamethasone led to a deficiency of renal units in the offspring, accompanied by an increased expression of fetal renal sodium channels, which persisted in the offspring (104). Furthermore, Wintour et al. discovered that the reduction in renal units in prenatal GCs-exposed offspring was linked with grossly enlarged and dilated proximal tubules, as well as an increased accumulation of collagen type I and III in the tubular interstitium and periadventitia of the renal cortical vessels (105). These studies collectively suggest that prenatal GCs exposure can disrupt fetal kidney development, leading to a decrease in the number of nephrons in offspring after birth. This, in turn, results in elevated blood pressure in the offspring. In addition, the inhibition of the Renin-Angiotensin System (RAS) during kidney development is thought to be the primary cause of the decrease in nephron number (100, 106). Moritz et al. reported that exposure to high concentrations of dexamethasone in fetal lambs can lead to significant changes in the fetal kidney RAS (100). Singh et al. reported that prenatal exposure to corticosterone in rat embryos can amplify the expression of angiotensin receptors, potentially limiting the growth and proliferation of kidney cells in offspring, thereby leading to a reduction in nephron units (106). Shalout et al. found that candesartan could normalize blood pressure in offspring exposed to prenatal betamethasone by inhibiting the expression and function of angiotensin receptors (107). Taken together, the coarsening and dilatation of the proximal renal tubules, the increased accumulation of type I and type III collagen in the tubulointerstitial and perivascular endothelium of the renal cortex, and the suppression of the RAS are all potential mechanisms through which exposure to prenatal GCs results in reduced renal units in the offspring and accordingly leads to hypertension in the offspring.
6.3.2 Dysfunctions in the HPA axis
The HPA axis plays a crucial role in regulating the circulating levels of GCs and is the primary neuroendocrine system in mammals that provides a rapid response and defense against stress. Dysfunction of the HPA axis is considered an important factor in the development of hypertension. Clinical and animal studies in recent years have shown that prenatal GCs exposure not only leads to dysfunction of the fetal HPA axis, but also has long-term adverse effects on the HPA axis function of offspring after birth (108–111). For example, Matthews et al. found that prenatal GCs exposure can permanently alter the HPA function and other endocrine system regulations in offspring before puberty, during puberty, and in aging in a gender-dependent manner (112). HPA axis dysfunction is typically manifested as abnormal secretion of epinephrine. Excessive secretion of epinephrine is closely related to the occurrence of hypertension. Several studies have shown that offspring exposed to prenatal GCs have abnormal secretion of epinephrine and related gene expression, which may be important reasons for the development of hypertension in offspring exposed to prenatal GCs (98, 113, 114).
Phenylethanolamine N-methyltransferase (PNMT) is an enzyme involved in the biosynthesis of epinephrine. Nguyen et al. reported that male rat offspring exposed to prenatal dexamethasone had elevated blood pressure, and increased plasma epinephrine levels and PNMT expression (98, 114). Lamothe et al. found that DNA methyltransferases (DNMTs) and histone deacetylases (HDACs) are involved in regulating PNMT expression. To further elucidate the direct relationship between PNMT expression, and the development of hypertension in prenatal GCs-exposed offspring, they evaluated the effects of the HDAC and DNMT inhibitors on blood pressure and PNMT expression. They found that both inhibitors successfully reduced PNMT expression and epinephrine levels, and restored blood pressure to normal levels in prenatal GCs-exposed offspring (115). These results further suggest that increased PNMT gene expression and dysfunction of the HPA axis are important reasons for the development of hypertension in prenatal GCs-exposed offspring. In addition, Tharmalingam et al. performed a whole transcriptome analysis of adrenal gland of prenatal GCs-exposed offspring. They found that prenatal GCs-exposed adrenal glands have impaired circadian signaling, and suggested that changes in adrenal circadian rhythms may be a potential molecular mechanism contributing to the development of hypertension (116).
6.3.3 Dysfunctions in vascular system
Blood pressure is influenced by multiple factors, and besides being related to kidney and the HPA axis, the regulation of blood pressure cannot be separated from vascular functions. Hypertension is closely related to dysfunction in vascular constriction and dilation (117, 118). It can be inferred that the increase in blood pressure in offspring caused by prenatal GCs exposure may be related to vascular dysfunction. Endothelial cells are single-layer flat cells covering the inner surface of blood vessels, capable of secreting various factors involved in the regulation of vascular function and blood pressure. Endothelin-1 (ET1) is the most effective vasoconstrictor secreted by endothelial cells. Lee et al. found that ET1 caused stronger vasoconstriction in sheep arteries exposed to prenatal dexamethasone (119). Nitric oxide (NO) is the most significant vasodilator secreted by endothelial cells, and its abnormal synthesis is related to the progression of hypertension (120, 121). Evidence suggests that one of the mechanisms by which GCs lead to vascular dysfunction is that excessive GCs can promote oxidative stress in vascular tissue, which can disrupt the availability of endothelial NO by reducing the synthesis of NO and promoting its degradation, leading to vascular dysfunction in patients with GCs excess (122). Molnar et al. evaluated the vascular endothelial function of prenatal GCs-exposed offspring and found that NO synthesis capacity was significantly reduced, and NO-dependent vascular dilation function was decreased (123). Although the mechanism is not yet clear, given the importance of NO and other endothelial secretory factors in blood pressure regulation, this provides important information for understanding the mechanisms by which prenatal GCs exposure leads to hypertension in offspring.
Vascular smooth muscle cells are the main cell type in blood vessels, and their sensitivity to classical vasoconstrictors determines the vascular resistance and regulates blood pressure. As the main effector of the RAS, Angiotensin II (Ang II) is a classic vasoconstrictor, which mainly causes vasoconstriction and blood pressure elevation through its receptor AT1R. Candesartan is an AT1R blocker which prevents Ang II from binding to them, thereby reducing blood vessel constriction and lowering blood pressure (124). Roghair et al. found that prenatal GCs exposure upregulates the expression of coronary artery AT1R receptors, enhancing the sensitivity of coronary arteries to Ang II-induced constriction (125).
BK (high-conductance Ca2+-activated K+ channels) and Cav1.2 (L-type Ca2+) channels are the main K+ and Ca2+ ion channels, and play an important role in vascular constriction and dilation function. Xu et al. found that prenatal GCs exposure reduces the expression of BK subunits α and β1, causing dysfunction in BK channel-mediated vascular dilation in fetal and adult offspring (15). In addition, Xu et al. also found that prenatal GCs exposure increases Cav1.2 expression, further increasing the sensitivity of blood vessels to classical vasoconstrictors such as prostaglandins and serotonin in fetal and adult offspring (126). Molecular mechanism studies have revealed that the abnormal expression of BK and Cav1.2 in offspring vascular system caused by prenatal GCs exposure is related to DNA methylation and histone modification (15, 126, 127). The above studies suggest that prenatal GCs exposure reprograms the expressions of key vascular regulatory factors in the fetal vascular system through epigenetic modifications, which accompany the offspring throughout their lives and affect the vascular functions, leading to an increase in blood pressure in offspring.
7 Conclusions
GCs, products of the HPA axis, play a crucial role in promoting fetal growth, development, and organ maturation when present in appropriate amounts. In almost all placental mammals, placental GCs barrier is enforced by 11βHSD2, which primarily functions to shield the fetus from an excess of maternal GCs. However, when maternal circulating GCs levels are excessive or placental GCs barrier is compromised, the fetus may be exposed to an excess of GCs. Prenatal GCs exposure can occur through two primary pathways: endogenous and exogenous. The endogenous pathway is primarily a result of GCs overproduction and impairment of the placental GCs barrier. The exogenous pathway typically involves the administration of synthetic GCs, often used to manage maternal immune disorders and prevent complications in preterm infants. There is substantial evidence suggesting that prenatal exposure to adverse factors such as stress, hypoxia, caffeine, and nicotine can impair the placental GCs barrier via reducing 11βHSD2 expression, leading to intrauterine fetal GCs exposure. Consequently, prenatal GCs exposure is characterized by its diversity, concealment, ubiquity, and at times, unavoidable clinical application.
Currently, a large number of clinical studies and animal experiments have confirmed that prenatal GCs-exposed offspring is often manifested as abnormal myocardial function and elevated blood pressure (15, 90, 126). Most of the current research on the cardiac development and function is descriptive of pathological phenomena, and further research is urgently needed to reveal and elucidate its potential mechanisms. Compared to the heart, research on the blood pressure is more comprehensive. Based on the results of clinical and animal studies, it is known that prenatal GCs exposure affects the blood pressure of newborn offspring to a certain extent (128, 129). However, due to the relative lack of clinical research data, the impact of prenatal GCs exposure on the blood pressure of human adult offspring is not yet clear. In sheep and rodent animal models, current results confirm that prenatal GCs exposure leads to elevated blood pressure in adult offspring, with a certain gender difference, as male offspring are more susceptible to the effects of GCs exposure. Additionally, the timing and dosage of prenatal GCs exposure also affect the blood pressure response of adult offspring. Further research indicates that the effects of prenatal GCs exposure on offspring blood pressure are related to various mechanisms such as reduced renal unit, HPA axis and vascular dysfunction.
Through reviewing and summarizing the related research, it can be confirmed that prenatal GCs exposure is closely related to the occurrence of cardiovascular diseases in offspring. The current findings mainly come from animal experiments. Because there are certain differences between animals and humans in terms of physiology and pathology, the experimental results cannot be completely equivalent to the human situation. Therefore, there is an urgent need to further prove it through a larger sample size and higher-quality clinical cohort follow-up investigations. Increasing evidence suggests that environmental stress can cause a series of trait changes in the parental generation (F0), some of which can be passed on to the offspring (F1) and even to subsequent generations (F2 or more). GCs are important stress hormones secreted by the body in response to external stress stimuli. Whether the pathological traits of the cardiovascular system in F1 offspring caused by prenatal GCs exposure can be transmitted across generations and the related mechanisms are still blank and worth exploring and revealing. Furthermore, the specific adverse effects and mechanisms in the fetal and offspring cardiovascular system caused by prenatal GCs exposure still need further exploration and clarification. Obtaining more information from mechanistic studies will not only understand the early developmental origins of cardiovascular diseases, providing new theoretical knowledge for the early prevention and treatment of such diseases, but also provide new insights for clinical intervention to mitigate the negative effects of prenatal GCs application. At the same time, it will also help establish new prenatal GCs treatment regimens, including optimal formulations, administration timing, and efficacy at different stages of pregnancy.
Author contributions
CZ: Writing – review & editing, Writing – original draft. LH: Writing – review & editing. LL: Writing – review & editing, Formal analysis. FD: Writing – review & editing, Investigation. MZ: Writing – review & editing, Investigation. CW: Writing – review & editing, Project administration. JQ: Writing – review & editing, Resources. QG: Writing – review & editing.
Funding
The author(s) declare financial support was received for the research, authorship, and/or publication of this article. Supported partly by the Ministry of Science and Technology (2019YFA0802600), the National Natural Science Foundation of China (82271724, 82271712, 81873841), the Jiangsu Provincial Health Committee (M2021087), and the Natural Science Foundation of Jiangsu Province (BK20221243).
Conflict of interest
The authors declare that the research was conducted in the absence of any commercial or financial relationships that could be construed as a potential conflict of interest.
Publisher’s note
All claims expressed in this article are solely those of the authors and do not necessarily represent those of their affiliated organizations, or those of the publisher, the editors and the reviewers. Any product that may be evaluated in this article, or claim that may be made by its manufacturer, is not guaranteed or endorsed by the publisher.
References
1. Timmermans S, Souffriau J, Libert C. A general introduction to glucocorticoid biology. Front Immunol. (2019) 10:1545. doi: 10.3389/fimmu.2019.01545
2. Rhen T, Cidlowski JA. Antiinflammatory action of glucocorticoids–new mechanisms for old drugs. N Engl J Med. (2005) 353:1711–23. doi: 10.1056/NEJMra050541
3. Roozendaal B, de Quervain DJ. Glucocorticoid therapy and memory function: lessons learned from basic research. Neurology. (2005) 64:184–5. doi: 10.1212/01.WNL.0000151711.27744.95
4. Liggins GC. The role of cortisol in preparing the fetus for birth. Reprod Fertil Dev. (1994) 6:141–50. doi: 10.1071/RD9940141
5. Fowden AL. Endocrine regulation of fetal growth. Reprod Fertil Dev. (1995) 7:351–63. doi: 10.1071/RD9950351
6. Pujades-Rodriguez M, Morgan AW, Cubbon RM, Wu J. Dose-dependent oral glucocorticoid cardiovascular risks in people with immune-mediated inflammatory diseases: A population-based cohort study. PLoS Med. (2020) 17:e1003432. doi: 10.1371/journal.pmed.1003432
7. Wei L, MacDonald TM, Walker BR. Taking glucocorticoids by prescription is associated with subsequent cardiovascular disease. Ann Intern Med. (2004) 141:764–70. doi: 10.7326/0003-4819-141-10-200411160-00007
8. Walker BR. Glucocorticoids and cardiovascular disease. Eur J Endocrinol. (2007) 157:545–59. doi: 10.1530/EJE-07-0455
9. Kajantie E, Raivio T, Jänne OA, Hovi P, Dunkel L, Andersson S. Circulating glucocorticoid bioactivity in the preterm newborn after antenatal betamethasone treatment. J Clin Endocrinol Metab. (2004) 89:3999–4003. doi: 10.1210/jc.2004-0013
10. Hennessy E, Alberman E. Adult cardiovascular risk factors in premature babies. Lancet. (2000) 356:938–9. doi: 10.1016/S0140-6736(05)73918-0
11. Gao Q, Tang J, Chen J, Jiang L, Zhu X, Xu Z. Epigenetic code and potential epigenetic-based therapies against chronic diseases in developmental origins. Drug Discovery Today. (2014) 19:1744–50. doi: 10.1016/j.drudis.2014.05.004
12. Jaddoe VW. Fetal nutritional origins of adult diseases: challenges for epidemiological research. Eur J Epidemiol. (2008) 23:767–71. doi: 10.1007/s10654-008-9304-9
13. Seckl JR, Meaney MJ. Glucocorticoid programming. Ann N Y Acad Sci. (2004) 1032:63–84. doi: 10.1196/annals.1314.006
14. Xu T, Fan X, Zhao M, Wu M, Li H, Ji B, et al. DNA methylation-reprogrammed ang II (Angiotensin II) type 1 receptor-early growth response gene 1-protein kinase C ϵ Axis underlies vascular hypercontractility in antenatal hypoxic offspring. Hypertension. (2021) 77:491–506. doi: 10.1161/HYPERTENSIONAHA.120.16247
15. Xu T, Zhao M, Li H, Zhou X, Liu B, Sun M, et al. Antenatal Dexamethasone Exposure Impairs the High-Conductance Ca(2+)-Activated K(+) Channels via Epigenetic Alteration at Gene Promoter in Male Offspring. Arterioscler Thromb Vasc Biol. (2020) 40:e284–95. doi: 10.1161/ATVBAHA.120.314905
16. Banjanin S, Kapoor A, Matthews SG. Prenatal glucocorticoid exposure alters hypothalamic-pituitary-adrenal function and blood pressure in mature male Guinea pigs. J Physiol. (2004) 558:305–18. doi: 10.1113/jphysiol.2004.063669
17. Miller WL, Auchus RJ. The molecular biology, biochemistry, and physiology of human steroidogenesis and its disorders. Endocr Rev. (2011) 32:81–151. doi: 10.1210/er.2010-0013
18. Spiga F, Walker JJ, Terry JR, Lightman SL. HPA axis-rhythms. Compr Physiol. (2014) 4:1273–98. doi: 10.1002/cphy.c140003
19. Dallman MF, Akana SF, Cascio CS, Darlington DN, Jacobson L, Levin N. Regulation of ACTH secretion: variations on a theme of B. Recent Prog Horm Res. (1987) 43:113–73. doi: 10.1016/B978-0-12-571143-2.50010-1
20. Dallman MF, Jones MT. Corticosteroid feedback control of ACTH secretion: effect of stress-induced corticosterone ssecretion on subsequent stress responses in the rat. Endocrinology. (1973) 92:1367–75. doi: 10.1210/endo-92-5-1367
21. Yamamoto KR. Steroid receptor regulated transcription of specific genes and gene networks. Annu Rev Genet. (1985) 19:209–52. doi: 10.1146/annurev.ge.19.120185.001233
22. Yang M, Chen J, Wei W. Dimerization of glucocorticoid receptors and its role in inflammation and immune responses. Pharmacol Res. (2021) 166:105334. doi: 10.1016/j.phrs.2020.105334
23. Michael AE, Papageorghiou AT. Potential significance of physiological and pharmacological glucocorticoids in early pregnancy. Hum Reprod Update. (2008) 14:497–517. doi: 10.1093/humupd/dmn021
24. Bhaumik S, Lockett J, Cuffe J, Clifton VL. Glucocorticoids and their receptor isoforms: roles in female reproduction, pregnancy, and foetal development. Biol (Basel). (2023) 12(8):1104. doi: 10.3390/biology12081104
25. Chatuphonprasert W, Jarukamjorn K, Ellinger I. Physiology and pathophysiology of steroid biosynthesis, transport and metabolism in the human placenta. Front Pharmacol. (2018) 9:1027. doi: 10.3389/fphar.2018.01027
26. Lee JH, Torpy DJ. Adrenal insufficiency in pregnancy: Physiology, diagnosis, management and areas for future research. Rev Endocr Metab Disord. (2023) 24:57–69. doi: 10.1007/s11154-022-09745-6
27. Stickel S, Eickhoff SB, Habel U, Stickeler E, Goecke TW, Lang J, et al. Endocrine stress response in pregnancy and 12 weeks postpartum - Exploring risk factors for postpartum depression. Psychoneuroendocrinology. (2021) 125:105122. doi: 10.1016/j.psyneuen.2020.105122
28. Zhu P, Wang W, Zuo R, Sun K. Mechanisms for establishment of the placental glucocorticoid barrier, a guard for life. Cell Mol Life Sci. (2019) 76:13–26. doi: 10.1007/s00018-018-2918-5
29. McTernan CL, Draper N, Nicholson H, Chalder SM, Driver P, Hewison M, et al. Reduced placental 11beta-hydroxysteroid dehydrogenase type 2 mRNA levels in human pregnancies complicated by intrauterine growth restriction: an analysis of possible mechanisms. J Clin Endocrinol Metab. (2001) 86:4979–83. doi: 10.1210/jcem.86.10.7893
30. Schoof E, Girstl M, Frobenius W, Kirschbaum M, Repp R, Knerr I, et al. Course of placental 11beta-hydroxysteroid dehydrogenase type 2 and 15-hydroxyprostaglandin dehydrogenase mRNA expression during human gestation. Eur J Endocrinol. (2001) 145:187–92. doi: 10.1530/eje.0.1450187
31. Alikhani-Koopaei R, Fouladkou F, Frey FJ, Frey BM. Epigenetic regulation of 11 beta-hydroxysteroid dehydrogenase type 2 expression. J Clin Invest. (2004) 114:1146–57. doi: 10.1172/JCI21647
32. Ni XT, Duan T, Yang Z, Guo CM, Li JN, Sun K. Role of human chorionic gonadotropin in maintaining 11beta-hydroxysteroid dehydrogenase type 2 expression in human placental syncytiotrophoblasts. Placenta. (2009) 30:1023–8. doi: 10.1016/j.placenta.2009.10.005
33. Zuo R, Liu X, Wang W, Li W, Ying H, Sun K. A repressive role of enhancer of zeste homolog 2 in 11β-hydroxysteroid dehydrogenase type 2 expression in the human placenta. J Biol Chem. (2017) 292:7578–87. doi: 10.1074/jbc.M116.765800
34. Stark MJ, Wright IM, Clifton VL. Sex-specific alterations in placental 11beta-hydroxysteroid dehydrogenase 2 activity and early postnatal clinical course following antenatal betamethasone. Am J Physiol Regul Integr Comp Physiol. (2009) 297:R510–4. doi: 10.1152/ajpregu.00175.2009
35. Murphy VE, Zakar T, Smith R, Giles WB, Gibson PG, Clifton VL. Reduced 11beta-hydroxysteroid dehydrogenase type 2 activity is associated with decreased birth weight centile in pregnancies complicated by asthma. J Clin Endocrinol Metab. (2002) 87:1660–8. doi: 10.1210/jcem.87.4.8377
36. Jung C, Ho JT, Torpy DJ, Rogers A, Doogue M, Lewis JG, et al. A longitudinal study of plasma and urinary cortisol in pregnancy and postpartum. J Clin Endocrinol Metab. (2011) 96:1533–40. doi: 10.1210/jc.2010-2395
37. Barbazanges A, Piazza PV, Le Moal M, Maccari S. Maternal glucocorticoid secretion mediates long-term effects of prenatal stress. J Neurosci. (1996) 16:3943–9. doi: 10.1523/JNEUROSCI.16-12-03943.1996
38. Sapolsky RM, Krey LC, McEwen BS. The neuroendocrinology of stress and aging: the glucocorticoid cascade hypothesis. Endocr Rev. (1986) 7:284–301. doi: 10.1210/edrv-7-3-284
39. Takahashi LK, Turner JG, Kalin NH. Prolonged stress-induced elevation in plasma corticosterone during pregnancy in the rat: implications for prenatal stress studies. Psychoneuroendocrinology. (1998) 23:571–81. doi: 10.1016/S0306-4530(98)00024-9
40. Ge C, Xu D, Yu P, Fang M, Guo J, Xu D, et al. P-gp expression inhibition mediates placental glucocorticoid barrier opening and fetal weight loss. BMC Med. (2021) 19:311. doi: 10.1186/s12916-021-02173-4
41. van Beek JP, Guan H, Julan L, Yang K. Glucocorticoids stimulate the expression of 11beta-hydroxysteroid dehydrogenase type 2 in cultured human placental trophoblast cells. J Clin Endocrinol Metab. (2004) 89:5614–21. doi: 10.1210/jc.2004-0113
42. O'Donnell KJ, Bugge Jensen A, Freeman L, Khalife N, O'Connor TG, Glover V. Maternal prenatal anxiety and downregulation of placental 11β-HSD2. Psychoneuroendocrinology. (2012) 37:818–26. doi: 10.1677/joe.1.06374
43. Welberg LA, Thrivikraman KV, Plotsky PM. Chronic maternal stress inhibits the capacity to up-regulate placental 11beta-hydroxysteroid dehydrogenase type 2 activity. J Endocrinol. (2005) 186(3):R7–R12. doi: 10.1677/joe.1.06374
44. Cuffe JS, O'Sullivan L, Simmons DG, Anderson ST, Moritz KM. Maternal corticosterone exposure in the mouse has sex-specific effects on placental growth and mRNA expression. Endocrinology. (2012) 153:5500–11. doi: 10.1210/en.2012-1479
45. Mairesse J, Lesage J, Breton C, Bréant B, Hahn T, Darnaudéry M, et al. Maternal stress alters endocrine function of the feto-placental unit in rats. Am J Physiol Endocrinol Metab. (2007) 292:E1526–33. doi: 10.1152/ajpendo.00574.2006
46. Olson DM, Severson EM, Verstraeten BS, Ng JW, McCreary JK, Metz GA. Allostatic load and preterm birth. Int J Mol Sci. (2015) 16:29856–74. doi: 10.3390/ijms161226209
47. Togher KL, Togher KL, O'Keeffe MM, O'Keeffe MM, Khashan AS, Khashan AS, et al. Epigenetic regulation of the placental HSD11B2 barrier and its role as a critical regulator of fetal development. Epigenetics. (2014) 9:816–22. doi: 10.4161/epi.28703
48. Jensen Peña C, Monk C, Champagne FA. Epigenetic effects of prenatal stress on 11β-hydroxysteroid dehydrogenase-2 in the placenta and fetal brain. PLoS One. (2012) 7:e39791. doi: 10.1371/journal.pone.0039791
49. Conradt E, Lester BM, Appleton AA, Armstrong DA, Marsit CJ. The roles of DNA methylation of NR3C1 and 11β-HSD2 and exposure to maternal mood disorder in utero on newborn neurobehavior. Epigenetics. (2013) 8:1321–9. doi: 10.4161/epi.26634
50. Cuffe JS, Walton SL, Singh RR, Spiers JG, Bielefeldt-Ohmann H, Wilkinson L, et al. Mid- to late term hypoxia in the mouse alters placental morphology, glucocorticoid regulatory pathways and nutrient transporters in a sex-specific manner. J Physiol. (2014) 592:3127–41. doi: 10.1113/jphysiol.2014.272856
51. Börzsönyi B, Demendi C, Pajor A, Rigó J Jr., Marosi K, Agota A, et al. Gene expression patterns of the 11β-hydroxysteroid dehydrogenase 2 enzyme in human placenta from intrauterine growth restriction: the role of impaired feto-maternal glucocorticoid metabolism. Eur J Obstet Gynecol Reprod Biol. (2012) 161:12–7. doi: 10.1016/j.ejogrb.2011.12.013
52. Ji B, Lei J, Xu T, Zhao M, Cai H, Qiu J, et al. Effects of prenatal hypoxia on placental glucocorticoid barrier: Mechanistic insight from experiments in rats. Reprod Toxicol. (2022) 110:78–84. doi: 10.1016/j.reprotox.2022.03.016
53. Hardy DB, Yang K. The expression of 11 beta-hydroxysteroid dehydrogenase type 2 is induced during trophoblast differentiation: effects of hypoxia. J Clin Endocrinol Metab. (2002) 87:3696–701. doi: 10.1210/jcem.87.8.8720
54. Alfaidy N, Gupta S, DeMarco C, Caniggia I, Challis JR. Oxygen regulation of placental 11 beta-hydroxysteroid dehydrogenase 2: physiological and pathological implications. J Clin Endocrinol Metab. (2002) 87:4797–805. doi: 10.1210/jc.2002-020310
55. Xu D, Zhang B, Liang G, Ping J, Kou H, Li X, et al. Caffeine-induced activated glucocorticoid metabolism in the hippocampus causes hypothalamic-pituitary-adrenal axis inhibition in fetal rats. PLoS One. (2012) 7:e44497. doi: 10.1371/journal.pone.0044497
56. Lv F, Fan G, Wan Y, Chen Y, Ni Y, Huang J, et al. Intrauterine endogenous high glucocorticoids program ovarian dysfunction in female offspring secondary to prenatal caffeine exposure. Sci Total Environ. (2021) 789:147691. doi: 10.1016/j.scitotenv.2021.147691
57. Pei LG, Yuan C, Guo YT, Kou H, Xia LP, Zhang L, et al. Prenatal caffeine exposure induced high susceptibility to metabolic syndrome in adult female offspring rats and its underlying mechanisms. Reprod Toxicol. (2017) 71:150–8. doi: 10.1016/j.reprotox.2017.06.045
58. Tan Y, Lu K, Li J, Ni Q, Zhao Z, Magdalou J, et al. Prenatal caffeine exprosure increases adult female offspring rat's susceptibility to osteoarthritis via low-functional programming of cartilage IGF-1 with histone acetylation. Toxicol Lett. (2018) 295:229–36. doi: 10.1016/j.toxlet.2018.06.1221
59. Shi H, Li B, Gao H, He H, Wu Z, Magdaloud J, et al. Intrauterine programming of cartilaginous 11β-HSD2 induced by corticosterone and caffeine mediated susceptibility to adult osteoarthritis. Ecotoxicol Environ Saf. (2022) 239:113624. doi: 10.1016/j.ecoenv.2022.113624
60. Xiao H, Wu Z, Li B, Shangguan Y, Stoltz JF, Magdalou J, et al. The low-expression programming of 11β-HSD2 mediates osteoporosis susceptibility induced by prenatal caffeine exposure in male offspring rats. Br J Pharmacol. (2020) 177:4683–700. doi: 10.1111/bph.15225
61. Fan F, Shen W, Wu S, Chen N, Tong X, Wang F, et al. Sp1 participates in the cadmium-induced imbalance of the placental glucocorticoid barrier by suppressing 11β-HSD2 expression. Environ pollut. (2020) 261:113976. doi: 10.1016/j.envpol.2020.113976
62. Chen N, Tong X, Wu S, Xu X, Chen Q, Wang F. Cadmium induces placental glucocorticoid barrier damage by suppressing the cAMP/PKA/Sp1 pathway and the protective role of taurine. Toxicol Appl Pharmacol. (2022) 440:115938. doi: 10.1016/j.taap.2022.115938
63. Chen M, Wang T, Liao ZX, Pan XL, Feng YH, Wang H. Nicotine-induced prenatal overexposure to maternal glucocorticoid and intrauterine growth retardation in rat. Exp Toxicol Pathol. (2007) 59:245–51. doi: 10.1016/j.etp.2007.05.007
64. Liang G, Chen M, Pan XL, Zheng J, Wang H. Ethanol-induced inhibition of fetal hypothalamic-pituitary-adrenal axis due to prenatal overexposure to maternal glucocorticoid in mice. Exp Toxicol Pathol. (2011) 63:607–11. doi: 10.1016/j.etp.2010.04.015
65. Chin EH, Schmidt KL, Martel KM, Wong CK, Hamden JE, Gibson WT, et al. A maternal high-fat, high-sucrose diet has sex-specific effects on fetal glucocorticoids with little consequence for offspring metabolism and voluntary locomotor activity in mice. PLoS One. (2017) 12:e0174030. doi: 10.1371/journal.pone.0174030
66. Bellisario V, Panetta P, Balsevich G, Baumann V, Noble J, Raggi C, et al. Maternal high-fat diet acts as a stressor increasing maternal glucocorticoids' signaling to the fetus and disrupting maternal behavior and brain activation in C57BL/6J mice. Psychoneuroendocrinology. (2015) 60:138–50. doi: 10.1016/j.psyneuen.2015.06.012
67. Garbrecht MR, Lamb FS. Placental HSD2 expression and activity is unaffected by maternal protein consumption or gender in C57BL/6 mice. ISRN Endocrinol. (2013) 2013:867938. doi: 10.1155/2013/867938
68. Maniam J, Antoniadis C, Morris MJ, Early-Life Stress HPA. Axis adaptation, and mechanisms contributing to later health outcomes. Front Endocrinol (Lausanne). (2014) 5:73. doi: 10.3389/fendo.2014.00073
69. Lan N, Chiu MP, Ellis L, Weinberg J. Prenatal alcohol exposure and prenatal stress differentially alter glucocorticoid signaling in the placenta and fetal brain. Neuroscience. (2017) 342:167–79. doi: 10.1016/j.neuroscience.2015.08.058
70. Maeyama H, Hirasawa T, Tahara Y, Obata C, Kasai H, Moriishi K, et al. Maternal restraint stress during pregnancy in mice induces 11β-HSD1-associated metabolic changes in the livers of the offspring. J Dev Orig Health Dis. (2015) 6:105–14. doi: 10.1017/S2040174415000100
71. Weinberg J, Sliwowska JH, Lan N, Hellemans KG. Prenatal alcohol exposure: foetal programming, the hypothalamic-pituitary-adrenal axis and sex differences in outcome. J Neuroendocrinol. (2008) 20:470–88. doi: 10.1111/j.1365-2826.2008.01669.x
72. Iqbal U, Brien JF, Kapoor A, Matthews SG, Reynolds JN. Chronic prenatal ethanol exposure increases glucocorticoid-induced glutamate release in the hippocampus of the near-term foetal Guinea pig. J Neuroendocrinol. (2006) 18:826–34. doi: 10.1111/j.1365-2826.2006.01479.x
73. Roberts D, Brown J, Medley N, Dalziel SR. Antenatal corticosteroids for accelerating fetal lung maturation for women at risk of preterm birth. Cochrane Database Syst Rev. (2017) 3:Cd004454. doi: 10.1002/14651858.CD004454.pub3
74. Langdown ML, Holness MJ, Sugden MC. Early growth retardation induced by excessive exposure to glucocorticoids in utero selectively increases cardiac GLUT1 protein expression and Akt/protein kinase B activity in adulthood. J Endocrinol. (2001) 169:11–22. doi: 10.1677/joe.0.1690011
75. Kurian J, Yuko AE, Kasatkin N, Rigaud VOC, Busch K, Harlamova D, et al. Uncoupling protein 2-mediated metabolic adaptations define cardiac cell function in the heart during transition from young to old age. Stem Cells Transl Med. (2021) 10:144–56. doi: 10.1002/sctm.20-0123
76. Langdown ML, Smith ND, Sugden MC, Holness MJ. Excessive glucocorticoid exposure during late intrauterine development modulates the expression of cardiac uncoupling proteins in adult hypertensive male offspring. Pflugers Arch. (2001) 442:248–55. doi: 10.1007/s004240100519
77. Song L, Alcalai R, Arad M, Wolf CM, Toka O, Conner DA, et al. Calsequestrin 2 (CASQ2) mutations increase expression of calreticulin and ryanodine receptors, causing catecholaminergic polymorphic ventricular tachycardia. J Clin Invest. (2007) 117:1814–23. doi: 10.1172/JCI31080
78. Langdown ML, Holness MJ, Sugden MC. Effects of prenatal glucocorticoid exposure on cardiac calreticulin and calsequestrin protein expression during early development and in adulthood. Biochem J. (2003) 371:61–9. doi: 10.1042/bj20021771
79. Bian X, Seidler FJ, Slotkin TA. Fetal dexamethasone exposure interferes with establishment of cardiac noradrenergic innervation and sympathetic activity. Teratology. (1993) 47:109–17. doi: 10.1002/tera.1420470203
80. Bian XP, Seidler FJ, Slotkin TA. Promotional role for glucocorticoids in the development of intracellular signalling: enhanced cardiac and renal adenylate cyclase reactivity to beta-adrenergic and non-adrenergic stimuli after low-dose fetal dexamethasone exposure. J Dev Physiol. (1992) 17:289–97.
81. de Vries A, Holmes MC, Heijnis A, Seier JV, Heerden J, Louw J, et al. Prenatal dexamethasone exposure induces changes in nonhuman primate offspring cardiometabolic and hypothalamic-pituitary-adrenal axis function. J Clin Invest. (2007) 117:1058–67. doi: 10.1172/JCI30982
82. Peng J, Zhou Y, Zhang Z, Wang Z, Gao L, Zhang X, et al. The detrimental effects of glucocorticoids exposure during pregnancy on offspring's cardiac functions mediated by hypermethylation of bone morphogenetic protein-4. Cell Death Dis. (2018) 9:834. doi: 10.1038/s41419-018-0841-1
83. Gao LT, Yuan JQ, Zhang ZY, Zhao HM, Gao L. Hypermethylation of the Bmp4 promoter dampens binding of HIF-1α and impairs its cardiac protective effects from oxidative stress in prenatally GC-exposed offspring. Cell Mol Life Sci. (2023) 80:58. doi: 10.1007/s00018-023-04703-0
84. Bal MP, de Vries WB, Steendijk P, Homoet-van der Kraak P, van der Leij FR, Baan J, et al. Histopathological changes of the heart after neonatal dexamethasone treatment: studies in 4-, 8-, and 50-week-old rats. Pediatr Res. (2009) 66:74–9. doi: 10.1203/PDR.0b013e3181a283a0
85. Mildenhall LF, Battin MR, Morton SM, Bevan C, Kuschel CA, Harding JE. Exposure to repeat doses of antenatal glucocorticoids is associated with altered cardiovascular status after birth. Arch Dis Child Fetal Neonatal Ed. (2006) 91:F56–60. doi: 10.1136/adc.2004.065300
86. Huh SY, Andrew R, Rich-Edwards JW, Kleinman KP, Seckl JR, Gillman MW. Association between umbilical cord glucocorticoids and blood pressure at age 3 years. BMC Med. (2008) 6:25. doi: 10.1186/1741-7015-6-25
87. McKinlay CJ, Cutfield WS, Battin MR, Dalziel SR, Crowther CA, Harding JE. Cardiovascular risk factors in children after repeat doses of antenatal glucocorticoids: an RCT. Pediatrics. (2015) 135:e405–15. doi: 10.1542/peds.2014-2408
88. Doyle LW, Ford GW, Davis NM, Callanan C. Antenatal corticosteroid therapy and blood pressure at 14 years of age in preterm children. Clin Sci (Lond). (2000) 98:137–42. doi: 10.1042/cs0980137
89. Dessens AB, Haas HS, Koppe JG. Twenty-year follow-up of antenatal corticosteroid treatment. Pediatrics. (2000) 105:E77. doi: 10.1542/peds.105.6.e77
90. Dalziel SR, Walker NK, Parag V, Mantell C, Rea HH, Rodgers A, et al. Cardiovascular risk factors after antenatal exposure to betamethasone: 30-year follow-up of a randomised controlled trial. Lancet. (2005) 365:1856–62. doi: 10.1016/S0140-6736(05)66617-2
91. Tangalakis K, Lumbers ER, Moritz KM, Towstoless MK, Wintour EM. Effect of cortisol on blood pressure and vascular reactivity in the ovine fetus. Exp Physiol. (1992) 77:709–17. doi: 10.1113/expphysiol.1992.sp003637
92. Koenen SV, Mecenas CA, Smith GS, Jenkins S, Nathanielsz PW. Effects of maternal betamethasone administration on fetal and maternal blood pressure and heart rate in the baboon at 0. 7 gestation. Am J Obstet Gynecol. (2002) 186:812–7. doi: 10.1067/mob.2002.121654
93. Dodic M, May CN, Wintour EM, Coghlan JP. An early prenatal exposure to excess glucocorticoid leads to hypertensive offspring in sheep. Clin Sci (Lond). (1998) 94:149–55. doi: 10.1042/cs0940149
94. Dodic M, Hantzis V, Duncan J, Rees S, Koukoulas I, Johnson K, et al. Programming effects of short prenatal exposure to cortisol. FASEB J. (2002) 16:1017–26. doi: 10.1096/fj.01-1045com
95. Tain YL, Chen CC, Sheen JM, Yu HR, Tiao MM, Kuo HC, et al. Melatonin attenuates prenatal dexamethasone-induced blood pressure increase in a rat model. J Am Soc Hypertens. (2014) 8:216–26. doi: 10.1016/j.jash.2014.01.009
96. Smith LM, Altamirano AK, Ervin MG, Seidner SR, Jobe AH. Prenatal glucocorticoid exposure and postnatal adaptation in premature newborn baboons ventilated for six days. Am J Obstet Gynecol. (2004) 191:1688–94. doi: 10.1016/j.ajog.2004.04.010
97. Alhamoud I, Legan SK, Gattineni J, Baum M. Sex differences in prenatal programming of hypertension by dexamethasone. Exp Biol Med (Maywood). (2021) 246:1554–62. doi: 10.1177/15353702211003294
98. Khurana S, Grandbois J, Tharmalingam S, Murray A, Graff K, Nguyen P, et al. Fetal programming of adrenal PNMT and hypertension by glucocorticoids in WKY rats is dose and sex-dependent. PLoS One. (2019) 14:e0221719. doi: 10.1371/journal.pone.0221719
99. Dodic M, Abouantoun T, O'Connor A, Wintour EM, Moritz KM. Programming effects of short prenatal exposure to dexamethasone in sheep. Hypertension. (2002) 40:729–34. doi: 10.1161/01.HYP.0000036455.62159.7E
100. Moritz KM, Johnson K, Douglas-Denton R, Wintour EM, Dodic M. Maternal glucocorticoid treatment programs alterations in the renin-angiotensin system of the ovine fetal kidney. Endocrinology. (2002) 143:4455–63. doi: 10.1210/en.2002-220534
101. Brennan S, Watson DL, Rudd DM, Kandasamy Y. Kidney growth following preterm birth: evaluation with renal parenchyma ultrasonography. Pediatr Res. (2023) 93:1302–6. doi: 10.1038/s41390-022-01970-8
102. Brenner BM, Chertow GM. Congenital oligonephropathy and the etiology of adult hypertension and progressive renal injury. Am J Kidney Dis. (1994) 23:171–5. doi: 10.1016/S0272-6386(12)80967-X
103. Ortiz LA, Quan A, Weinberg A, Baum M. Effect of prenatal dexamethasone on rat renal development. Kidney Int. (2001) 59:1663–9. doi: 10.1046/j.1523-1755.2001.0590051663.x
104. Moritz KM, De Matteo R, Dodic M, Jefferies AJ, Arena D, Wintour EM, et al. Prenatal glucocorticoid exposure in the sheep alters renal development in utero: implications for adult renal function and blood pressure control. Am J Physiol Regul Integr Comp Physiol. (2011) 301:R500–9. doi: 10.1152/ajpregu.00818.2010
105. Wintour EM, Moritz KM, Johnson K, Ricardo S, Samuel CS, Dodic M. Reduced nephron number in adult sheep, hypertensive as a result of prenatal glucocorticoid treatment. J Physiol. (2003) 549:929–35. doi: 10.1113/jphysiol.2003.042408
106. Singh RR, Cullen-McEwen LA, Kett MM, Boon WM, Dowling J, Bertram JF, et al. Prenatal corticosterone exposure results in altered AT1/AT2, nephron deficit and hypertension in the rat offspring. J Physiol. (2007) 579:503–13. doi: 10.1113/jphysiol.2006.125773
107. Shaltout HA, Rose JC, Figueroa JP, Chappell MC, Diz DI, Averill DB. Acute AT(1)-receptor blockade reverses the hemodynamic and baroreflex impairment in adult sheep exposed to antenatal betamethasone. Am J Physiol Heart Circ Physiol. (2010) 299:H541–7. doi: 10.1152/ajpheart.00100.2010
108. Braun T, Challis JR, Newnham JP, Sloboda DM. Early-life glucocorticoid exposure: the hypothalamic-pituitary-adrenal axis, placental function, and long-term disease risk. Endocr Rev. (2013) 34:885–916. doi: 10.1210/er.2013-1012
109. Jellyman JK, Valenzuela OA, Fowden AL, HORSE SPECIES. SYMPOSIUM: Glucocorticoid programming of hypothalamic-pituitary-adrenal axis and metabolic function: Animal studies from mouse to horse. J Anim Sci. (2015) 93:3245–60. doi: 10.2527/jas.2014-8612
110. Tegethoff M, Pryce C, Meinlschmidt G. Effects of intrauterine exposure to synthetic glucocorticoids on fetal, newborn, and infant hypothalamic-pituitary-adrenal axis function in humans: a systematic review. Endocr Rev. (2009) 30:753–89. doi: 10.1210/er.2008-0014
111. Irwin JL, Meyering AL, Peterson G, Glynn LM, Sandman CA, Hicks LM, et al. Maternal prenatal cortisol programs the infant hypothalamic-pituitary-adrenal axis. Psychoneuroendocrinology. (2021) 125:105106. doi: 10.1016/j.psyneuen.2020.105106
112. Matthews SG, Owen D, Kalabis G, Banjanin S, Setiawan EB, Dunn EA, et al. Fetal glucocorticoid exposure and hypothalamo-pituitary-adrenal (HPA) function after birth. Endocr Res. (2004) 30:827–36. doi: 10.1081/erc-200044091
113. Nyirenda MJ, Welberg LA, Seckl JR. Programming hyperglycaemia in the rat through prenatal exposure to glucocorticoids-fetal effect or maternal influence? J Endocrinol. (2001) 170:653–60. doi: 10.1677/joe.0.1700653
114. Nguyen P, Khurana S, Peltsch H, Grandbois J, Eibl J, Crispo J, et al. Prenatal glucocorticoid exposure programs adrenal PNMT expression and adult hypertension. J Endocrinol. (2015) 227:117–27. doi: 10.1530/JOE-15-0244
115. Lamothe J, Khurana S, Tharmalingam S, Williamson C, Byrne CJ, Khaper N, et al. The role of DNMT and HDACs in the fetal programming of hypertension by glucocorticoids. Oxid Med Cell Longev. (2020) 2020:5751768. doi: 10.1155/2020/5751768
116. Tharmalingam S, Khurana S, Murray A, Lamothe J, Tai TC. Whole transcriptome analysis of adrenal glands from prenatal glucocorticoid programmed hypertensive rodents. Sci Rep. (2020) 10:18755. doi: 10.1038/s41598-020-75652-y
117. Wilson C, Zhang X, Buckley C, Heathcote HR, Lee MD, McCarron JG. Increased vascular contractility in hypertension results from impaired endothelial calcium signaling. Hypertension. (2019) 74:1200–14. doi: 10.1161/HYPERTENSIONAHA.119.13791
118. Tykocki NR, Boerman EM, Jackson WF. Smooth muscle ion channels and regulation of vascular tone in resistance arteries and arterioles. Compr Physiol. (2017) 7:485–581. doi: 10.1002/cphy.c160011
119. Lee JH, Zhang J, Massmann GA, Figueroa JP. Antenatal betamethasone increases vascular reactivity to endothelin-1 by upregulation of CD38/cADPR signaling. J Dev Orig Health Dis. (2014) 5:56–62. doi: 10.1017/S2040174413000512
120. Cyr AR, Huckaby LV, Shiva SS, Zuckerbraun BS. Nitric oxide and endothelial dysfunction. Crit Care Clin. (2020) 36:307–21. doi: 10.1016/j.ccc.2019.12.009
121. Förstermann U, Sessa WC. Nitric oxide synthases: regulation and function. Eur Heart J. (2012) 33:829–37, 837a-837d. doi: 10.1093/eurheartj/ehr304
122. Iuchi T, Akaike M, Mitsui T, Ohshima Y, Shintani Y, Azuma H, et al. Glucocorticoid excess induces superoxide production in vascular endothelial cells and elicits vascular endothelial dysfunction. Circ Res. (2003) 92:81–7. doi: 10.1161/01.RES.0000050588.35034.3C
123. Molnar J, Howe DC, Nijland MJ, Nathanielsz PW. Prenatal dexamethasone leads to both endothelial dysfunction and vasodilatory compensation in sheep. J Physiol. (2003) 547:61–6. doi: 10.1113/jphysiol.2002.032565
124. Lieber A, Millasseau S, Mahmud A, Bourhis L, Mairesse S, Protogerou A, et al. Cardiovascular prevention: relationships between arterial aging and chronic drug treatment. J Hum Hypertens. (2011) 25:524–31. doi: 10.1038/jhh.2011.28
125. Roghair RD, Lamb FS, Bedell KA, Smith OM, Scholz TD, Segar JL. Late-gestation betamethasone enhances coronary artery responsiveness to angiotensin II in fetal sheep. Am J Physiol Regul Integr Comp Physiol. (2004) 286:R80–8. doi: 10.1152/ajpregu.00421.2003
126. Xu T, Ji B, Li L, Lei J, Zhao M, Sun M, et al. Antenatal dexamethasone exposure impairs vascular contractile functions via upregulating IP3 receptor 1 and cav1.2 in adult male offspring. Hypertension. (2022) 79:1997–2007. doi: 10.1161/HYPERTENSIONAHA.122.19040
127. Lei J, Zhao M, Deng F, Xu T, Ji B, Wang X, et al. Prenatal dexamethasone exposure impaired vascular reactivity in adult male offspring cerebral arteries. J Mol Cell Cardiol. (2023) 181:46–56. doi: 10.1016/j.yjmcc.2023.05.008
128. Gyamfi-Bannerman C, Thom EA, Blackwell SC, Tita AT, Reddy UM, Saade GR, et al. Antenatal betamethasone for women at risk for late preterm delivery. N Engl J Med. (2016) 374:1311–20. doi: 10.1056/NEJMoa1516783
Keywords: prenatal GCs exposure, 11β hydroxysteroid dehydrogenase 2, placental GCs barrier, cardiovascular system, offspring
Citation: Zhao C, He L, Li L, Deng F, Zhang M, Wang C, Qiu J and Gao Q (2024) Prenatal glucocorticoids exposure and adverse cardiovascular effects in offspring. Front. Endocrinol. 15:1430334. doi: 10.3389/fendo.2024.1430334
Received: 14 June 2024; Accepted: 30 August 2024;
Published: 16 September 2024.
Edited by:
Hong Liu, UC Davis Health, United StatesReviewed by:
Sruthi Alahari, University of Toronto, CanadaVicki Lee Clifton, The University of Queensland, Australia
Wang Xuequan, Wenzhou Medical University, China
Copyright © 2024 Zhao, He, Li, Deng, Zhang, Wang, Qiu and Gao. This is an open-access article distributed under the terms of the Creative Commons Attribution License (CC BY). The use, distribution or reproduction in other forums is permitted, provided the original author(s) and the copyright owner(s) are credited and that the original publication in this journal is cited, in accordance with accepted academic practice. No use, distribution or reproduction is permitted which does not comply with these terms.
*Correspondence: Qinqin Gao, amVubnlxZ2FvQDEyNi5jb20=; Junlan Qiu, cWl1anVubGFuZEAxNjMuY29t
†These authors have contributed equally to this work