- 1Department of Pediatrics, Section of Nutrition, University of Colorado School of Medicine, Aurora, CO, United States
- 2Microbiome and Metabolism Research Unit (MMRU), United States Department of Agriculture - Agricultural Research Service (USDA-ARS), Southeast Area, Little Rock, AR, United States
- 3Arkansas Children’s Nutrition Center, University of Arkansas for Medical Sciences, Little Rock, AR, United States
- 4Department of Pediatrics, University of Arkansas for Medical Sciences, Little Rock, AR, United States
- 5Department of Pediatrics, Section of Neonatology, University of Colorado School of Medicine, Aurora, CO, United States
Background: Maternal undernutrition is the most common cause of fetal growth restriction (FGR) worldwide. FGR increases morbidity and mortality during infancy, as well as contributes to adult-onset diseases including obesity and type 2 diabetes. The role of the maternal or offspring microbiome in growth outcomes following FGR is not well understood.
Methods: FGR was induced by 30% maternal calorie restriction (CR) during the second half of gestation in C57BL/6 mice. Pup weights were obtained on day of life 0, 1, and 7 and ages 3, 4 and 16 weeks. Fecal pellets were collected from pregnant dams at gestational day 18.5 and from offspring at ages 3 and 4 weeks of age. Bacterial genomic DNA was used for amplification of the V4 variable region of the 16S rRNA gene. Multivariable associations between maternal CR and taxonomic abundance were assessed using the MaAsLin2 package. Associations between microbial taxa and offspring outcomes were performed using distance-based redundancy analysis and Pearson correlations.
Results: FGR pups weighed about 20% less than controls. Beta but not alpha diversity differed between control and CR dam microbiomes. CR dams had lower relative abundance of Turicibacter, Flexispira, and Rikenella, and increased relative abundance of Parabacteroides and Prevotella. Control and FGR offspring microbiota differed by beta diversity at ages 3 and 4 weeks. At 3 weeks, FGR offspring had decreased relative abundance of Akkermansia and Sutterella and increased relative abundance of Anaerostipes and Paraprevotella. At 4 weeks, FGR animals had decreased relative abundance of Allobaculum, Sutterella, Bifidobacterium, and Lactobacillus, among others, and increased relative abundance of Turcibacter, Dorea, and Roseburia. Maternal Helicobacter abundance was positively associated with offspring weight. Akkermansia abundance at age 3 and 4 weeks was negatively associated with adult weight.
Conclusions: We demonstrate gut microbial dysbiosis in pregnant dams and offspring at two timepoints following maternal calorie restriction. Additional research is needed to test for functional roles of the microbiome in offspring growth outcomes.
1 Introduction
Fetal growth restriction (FGR), also called intrauterine growth restriction, is the failure of a fetus to meet its growth potential (1, 2). FGR impacts up to 20% of pregnancies in low- and middle-income countries and contributes to both long- and short-term morbidity and mortality (3–6). There are many etiologies of FGR including maternal high blood pressure, preeclampsia, cigarette use, and genetic conditions (5, 7). However, maternal malnutrition is the most common preventable cause worldwide (8). FGR increases morbidity and mortality during infancy, as well as contributes to adult-onset diseases including obesity, chronic kidney disease, metabolic syndrome, and type 2 diabetes (3, 6). Multiple mechanisms contribute to these adverse outcomes such as epigenetic changes leading to altered gene expression (6, 9), decreased lean muscle mass at birth (10, 11), impaired nephron development (12), and diminished pancreatic beta cell mass (13).
Emerging evidence from our group and others suggests a role for the gut microbiome in the pathogenesis of FGR-related adverse outcomes. Multiple studies in mice, rats, pigs, and humans have shown gut microbiome dysbiosis in FGR offspring compared to normal weight controls (14–17). The microbiome plays many roles in overall health including education and modulation of the immune system (18–20), especially important for infants with FGR who are more susceptible to neonatal infections (3, 7). The microbiome may also impact feeding tolerance and development of necrotizing enterocolitis, both conditions with higher prevalence in FGR infants (7, 21–23). The gut microbiome, even in the earliest stages of life, can also predict the development of rapid weight gain, excess adiposity, and childhood obesity (24–27). Microbiome-directed interventions have been shown in multiple studies to influence growth in infancy and childhood. For example, neonatal antibiotic exposure is associated with reduced stature and increased risk of obesity (28, 29). Recent studies demonstrate a direct effect of the infant gut microbiome on intestinal barrier function and neuro-endocrine signaling (25). Microbial dysbiosis may therefore have long lasting effects on health after fetal growth restriction.
There is also increasing evidence that multiple maternal microbiomes impact pregnancy outcomes (30, 31). Fetal growth, establishment of the offspring microbiome, and development of the offspring immune system all depend on the maternal microbiome (14, 24, 32–36). Maternal microbial products such as lipopolysaccharide and metabolites including short-chain fatty acids reach the fetus and influence pregnancy outcomes including fetal growth and ultimately birth weight (33, 37, 38). The maternal gut microbiome changes during pregnancy and is further altered in the context of complications known to impact fetal growth and development, including gestational diabetes, preeclampsia, obesity, and fetal growth restriction (15, 24, 34, 39–43). However, the role of the gut microbiome in the setting of maternal calorie restriction in the pathogenesis of fetal growth restriction or early offspring growth is not fully understood.
In the present study we sought to examine the role of maternal caloric restriction on the maternal gut microbiome at the end of pregnancy (gestational day 18.5) and early offspring gut microbiome (postnatal days 21 and 28). In addition, we investigated potential associations of maternal taxa and maternal-fetal outcomes, as well as offspring taxa with early life weight and weight gain.
2 Methods
2.1 Ethical approval
All animal experiments and procedures were approved by the University of Colorado Institutional Animal Care and Use Committee (AUP#00274) and conducted in compliance with the American Association for Accreditation for Laboratory Animal Care at the Perinatal Research Center at the University of Colorado School of Medicine (Aurora, CO, USA).
2.2 Mouse model of fetal growth restriction
FGR was induced by maternal calorie restriction (CR) during the second half of gestation as previously described (14). Briefly, singly housed female C57BL/6 mice mated to C57BL/6 males were provided with ad lib access to standard laboratory chow (Teklad 2020X, Inotiv, Indianapolis, IN, USA) from gestational day E0-E9. Pregnant dams were randomized to continued ad lib chow (control, n = 7) or provision of chow diet to meet 70% of estimated calorie needs (CR, n = 13) from day E9 through delivery. After delivery, all dams had ad libitum access to standard laboratory chow throughout lactation. In this model, pups from CR dams weigh approximately 20% less at the end of gestation compared to controls, with catch-up weight gain achieved by day of life 2 (14). Only litters with at least 6 surviving pups were used for further study to minimize the effects of litter size on postnatal growth.
2.3 Outcome measures and feces collection
Dams were weighed on an electronic scale before mating and then daily throughout pregnancy. Gestational weight gain was defined as weight on day E18.5 minus weight immediately prior to mating (in grams). Litter size was determined by counting fetuses from a subset of dams euthanized on day E18.5 (n = 4 litters) or by counting pups on the morning after parturition (n = 15 litters). Pup weights were obtained on day of life 0 (n = 3 control litters, n = 4 FGR litters), day of life 1 (n = 6 control litters, n = 9 FGR litters), day of life 7 (n = 7 control litters, n = 8 FGR litters) and ages 3, 4 and 16 weeks. Pups were weaned on day of life 21 to a defined laboratory diet (7% calories from fat, Harlan TD.09283, Inotiv). Fresh fecal pellets were collected from pregnant dams on day E18.5 and from offspring on day of life 21 (n = 2 control litters, n = 3 FGR litters) and day of life 28 (n = 6 control litters, n = 7 FGR litters) by allowing each animal to walk around in a clean cage until one or more fecal pellets were produced. Pellets were frozen and stored at -80°C.
2.4 Fecal DNA isolation and microbiome sequencing
The fecal microbiome was assessed as previously described (14, 24). Briefly, bacterial DNA was extracted using a DNeasy PowerSoil HTP 96 kit (Qiagen, Redwood City, CA) including a bead-beating step on a TissueLyser II in 96-well PowerBead plates (Qiagen). Bacterial genomic DNA was used for amplification of the V4 variable region of the 16S rRNA gene using 515F/806R primers. Paired-end sequencing of pooled amplicons was accomplished on an Illumina MiSeq Instrument (Illumina, San Diego, CA).
2.5 Statistical analysis
Comparison groups were control versus CR dams, and control versus FGR offspring at two separate timepoints, 3 and 4 weeks of age. Statistical analyses and visualizations were performed in R version 4.2.2 or GraphPad Prism version 10.1.1. The fecal microbiome was analyzed as previously described (14, 24). Briefly, QIIME2 was used for data processing. Microbial counts, taxonomy information and sample metadata were imported using the phyloseq package (44). The microbiome package ‘core’ function was used to eliminate taxa that did not have at least 5 counts in 5% of samples. Following pre-processing, the following sample sizes were used in the final analysis; Dams: control n = 7 control, CR n = 13; age 3 weeks: control n = 14 (7 female), FGR n = 14 (6 female); age 4 weeks: control n = 15 (8 female); FGR n = 18 (9 female). Alpha diversity was determined using the microeco package (45) and Student’s t-test (for sex-combined analyses) or two-way ANOVA (for sex-stratified analyses) were used to test differences between groups. Beta diversity was assessed using Aitchison distance, Bray-Curtis dissimilarity, and Jaccard dissimilarity. Multidimensional scaling was used to visualize beta diversity for each group and statistical difference was tested using PERMANOVA with 999 permutations. Multivariable associations between maternal diet and taxonomic abundance were assessed using the MaAsLin2 package (46). Maternal diet was considered a fixed effect and offspring analyses were adjusted for litter. Taxa were agglomerated at the genus level. All P-values were false discovery rate-adjusted (FDR; Benjamini–Hochberg, q-values) and features with q < 0.3 were considered significant (the default value for MaAsLin2). Findings passing an un-adjusted P < 0.05 are also included in results. The OTU relative abundance is visualized on a log-transformed axis in figures. Associations between microbial taxa and offspring outcomes were summarized using distance-based redundancy analysis (db-RDA) Bray–Curtis distances and further tested using Pearson correlations in microeco using the cal_cor function.
3 Results
3.1 Animal characteristics
Calorie restricted (CR) dams gained 17% less weight over the duration of gestation compared to control dams (p=0.037, Table 1). Litter size was slightly higher in CR dams (mean ± SD: 8.54 ± 0.97) compared to control (7.43 ± 1.40; p=0.050). We also confirmed fetal growth restriction (FGR) of the offspring of CR dams with lower weights on day of life 0 by 10% (control 1.31 ± 0.03g; FGR: 1.18 ± 0.06g; p = 0.025). From day of life 1, FGR and control offspring weights did not differ on day of life 1, 7, 21, or 28 (Table 1). FGR offspring used for the Week 4 microbiome dataset had slower weight gain from day 21 (week 3) to day 28 (week 4; p = 0.049). In addition, no significant differences were shown between control and FGR offspring for weight gain at 16 weeks of age (Table 1).
3.2 Caloric restriction during pregnancy impacts maternal fecal microbiome composition
The fecal microbiome from CR dams (n = 13) at gestational day E18.5 was compared to that from control dams (n = 7). We saw no statistical difference for any measure of alpha diversity including Chao1, Shannon, Simpson or Fisher indices (Table 2). Global genus-level composition (beta diversity) differed between CR and control dams by all three distance measures tested (Table 3): Aitchison (Figure 1A), Bray-Curtis, and Jaccard. At the genus level, we found decreased relative abundance of Turicibacter (Figure 1B), Flexispira (Figure 1C), and Rikenella (Figure 1D), and increased relative abundance of Parabacteroides (Figure 1E) and Prevotella in CR dams (all p<0.05, Table 3). CR dams also had lower relative abundance of Bifidobacterium based on q value of < 0.03 (q = 0.29, p = 0.061; Table 4).
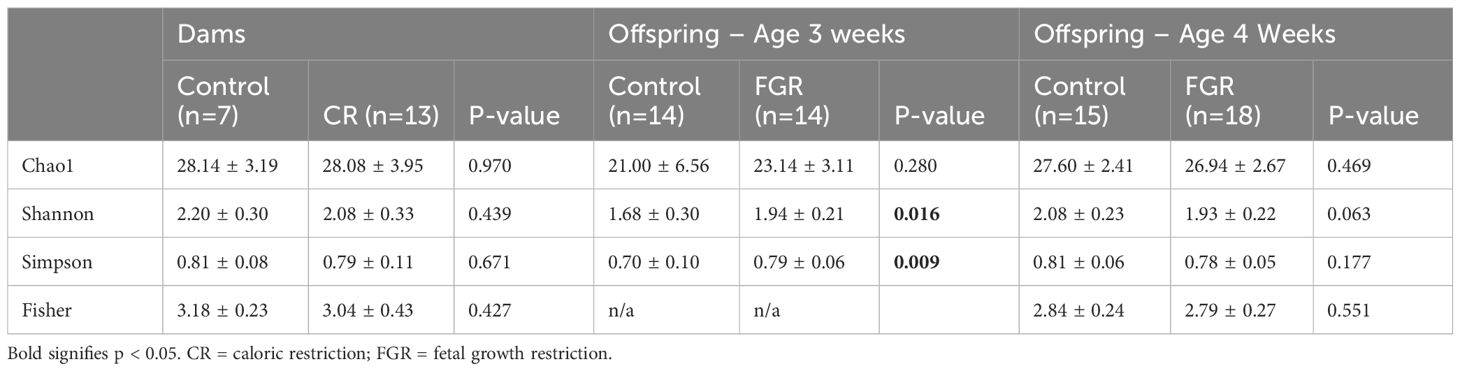
Table 2. Alpha diversity. Values are presented as mean ± SD. P-values were determined by Student’s t-test.
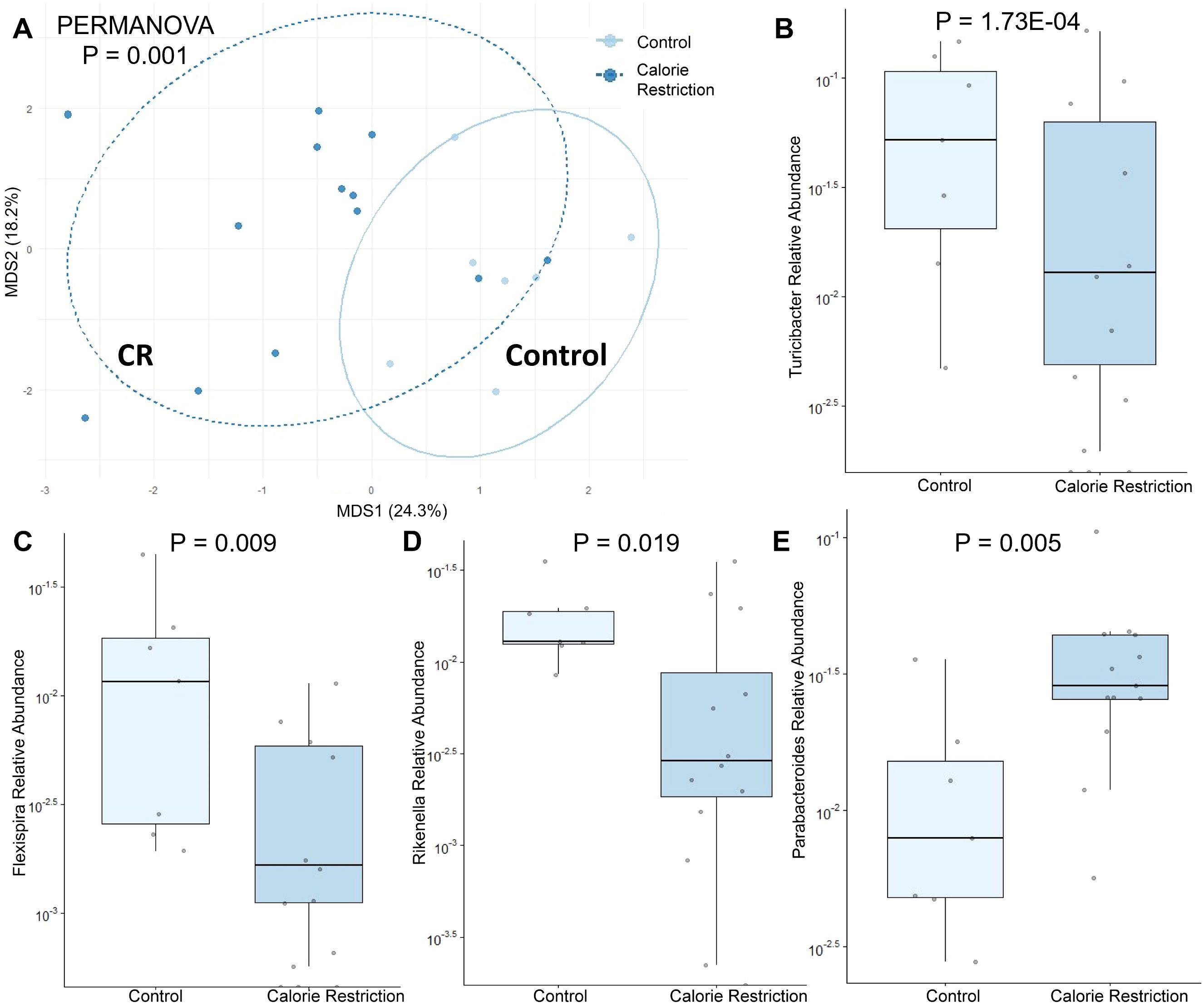
Figure 1. Fecal microbiome differences in control and calorie restricted dams at day E18.5. (A) Aitchison distance in control and calorie restricted dams. Relative abundance of (B) Turicibacter (C) Flexispira (D) Rikenella and (E) Parabacteroides in control and FGR dams. CR, caloric restriction.
3.3 Association of maternal bacterial taxa with pregnancy and early offspring outcomes
We used dbRDA to test for associations between maternal fecal microbial taxa and outcomes of pregnancy (maternal weight gain, litter size) and offspring (average pup weight on day of life 0, 1 and 7). Weight gain during pregnancy showed positive alignment with relative abundance of Mucispirillum and Odoribacter, and negatively alignment with Parabacteroides (Figure 2). The number of pups per litter and average pup weight on day of life 1 were positively aligned with Helicobacter and negatively aligned with Allobaculum and Bifidobacterium abundance. Using Pearson correlation and nominal p-values, several taxa were negatively associated with weight of offspring from CR dams including Bifidobacterium, Blautia, and Coprococcus (Supplementary Figure S1). In control dams, day 1 offspring weight was negatively associated with relative abundance of Roseburia, Ruminococcus, and Flexispira. No maternal taxa were associated with offspring weight at day 7 of life in either CR or control dams. After FDR-correction, the only remaining significant correlation was a negative association between Alistipes abundance in control dams and Day 0 offspring weight.
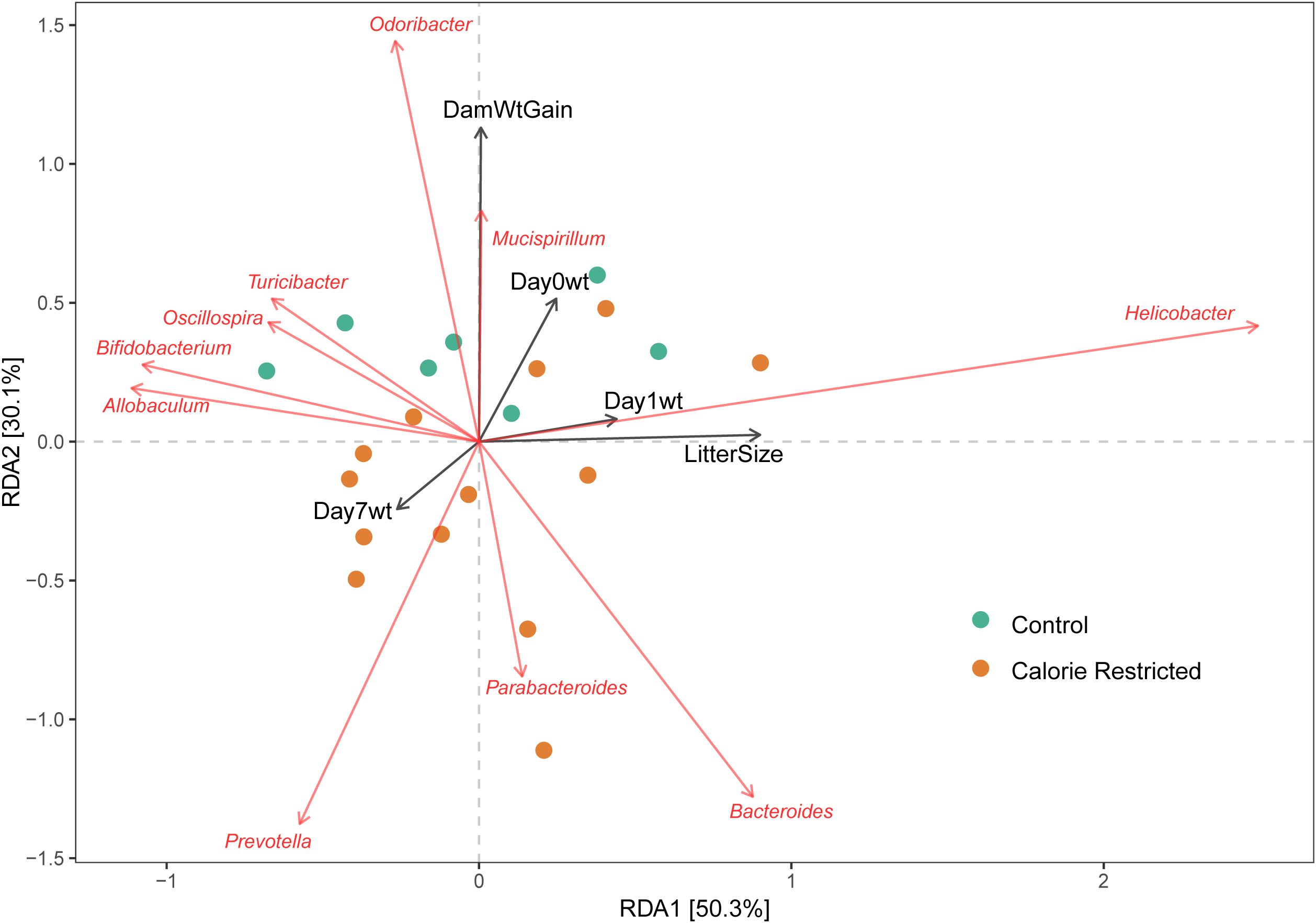
Figure 2. Association of maternal taxa with pregnancy and offspring outcomes. Distance-based redundancy analysis for genus-level taxa with selected outcomes. DamWtGain = Weight gain across pregnancy; Day0Wt, Average pup weight on day of life 0; Day1Wt, Average pup weight on day of life 1; Day7Wt, Average pup weight on day of life 7; LitterSize, Number of pups per litter.
3.4 Impact of fetal growth restriction due to maternal calorie restriction on offspring fecal microbiome composition at age 3 weeks
Analysis of offspring fecal microbiome, regardless of sex, demonstrated no differences in any measure of alpha diversity were detected between control (n = 14) and FGR (n = 14) at 3 weeks of life (Table 2). Beta diversity significantly differed between control and FGR offspring by Aitchison (Figure 3A), Bray-Curtis and Jaccard (Table 3). At the genus level, FGR offspring had decreased relative abundance of Akkermansia (Figure 3B) and Sutterella (Figure 3C) and increased relative abundance of Anaerostipes (Figure 3D) and Paraprevotella (Table 5).
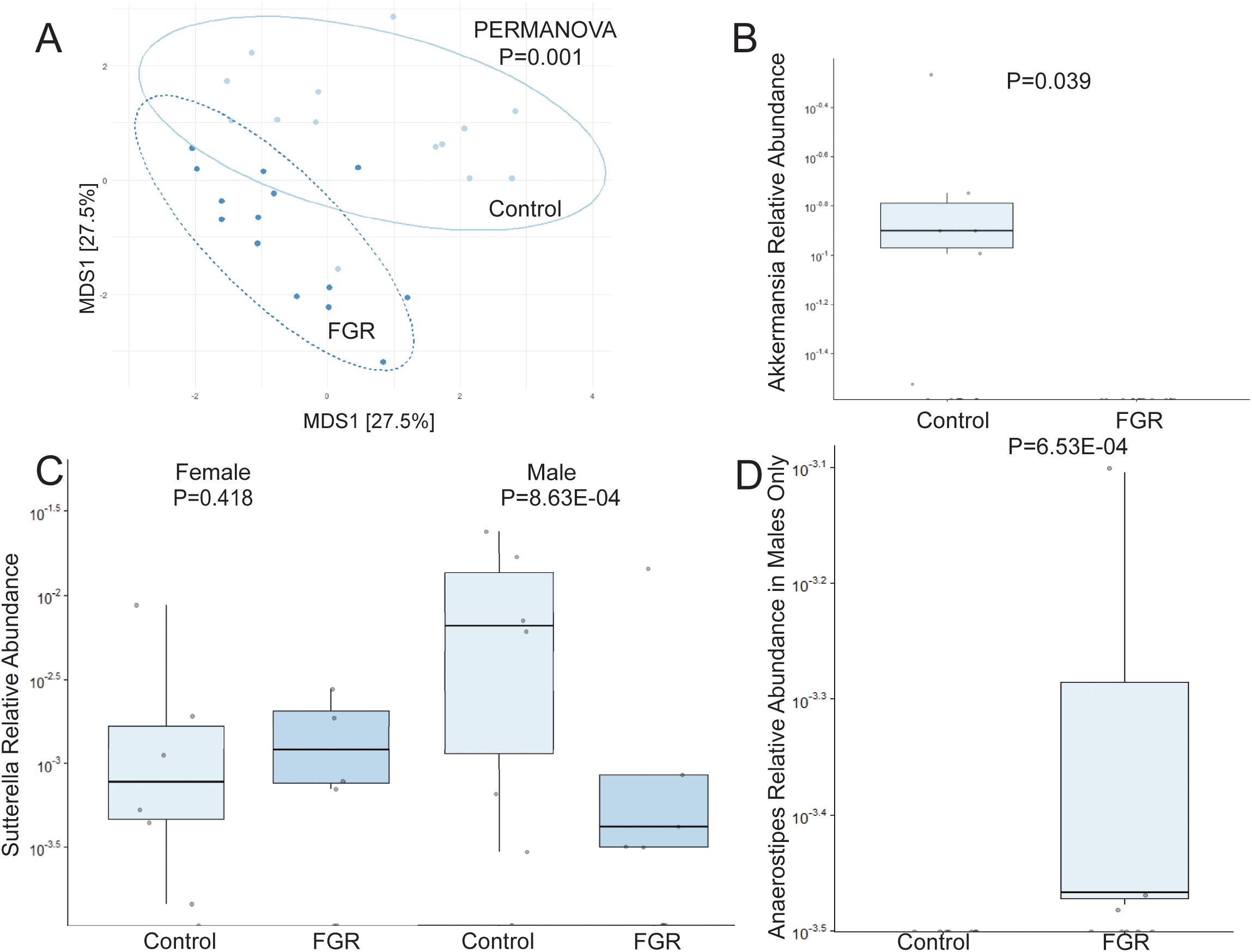
Figure 3. Fecal microbiome differences in control and fetal growth restricted offspring at age 3 weeks. (A) Aitchison distance in control and fetal growth restricted offspring at age 3 weeks. Relative abundance of (B) Akkermansia (C) Sutterella by offspring sex, and (D) Anaerostipes in control and FGR offspring. FGR, fetal growth restriction.
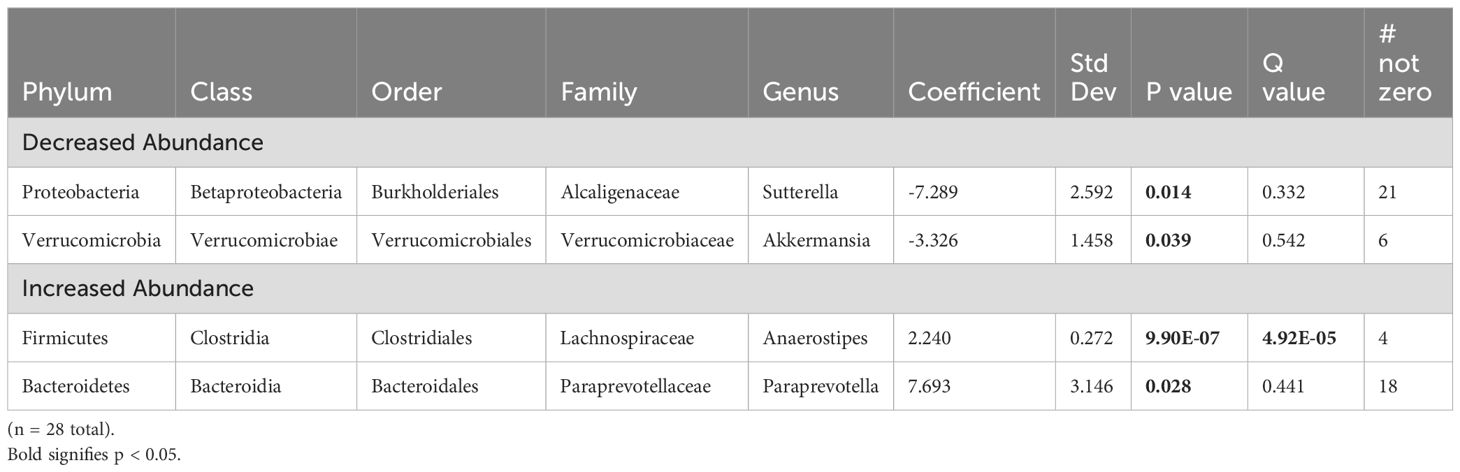
Table 5. Fecal microbiome from fetal growth restricted offspring compared to control offspring at 3 weeks of age.
We performed additional analyses to test for sex-specific differences between males (n = 7 control, n = 8 FGR) and females (n = 7 control, n = 6 FGR). For alpha diversity, two-way ANOVA revealed a significant association between intrauterine growth and microbial diversity (Shannon diversity index p = 0.021, Simpson index p = 0.022) but not richness (Chao1 p = 0.25). There was no significant interaction between sex and growth (all p > 0.1). Beta diversity differed between control and FGR male offspring when assessed by Aitchison and Jaccard distances but not by Bray-Curtis (Table 3). Beta diversity between control and FGR females differed according to all assessment methods (Table 3). Compared to control males, FGR males had increased relative abundance of Anaerostipes and Paraprevotella and decreased relative abundance of Sutterella (Figure 3C; Supplementary Table S1). FGR females had higher relative abundance of Oscillospira and Alistipes compared to control females (Supplementary Table S1).
3.5 Impact of fetal growth restriction due to maternal calorie restriction on offspring fecal microbiome composition at age 4 weeks
Analysis of offspring fecal microbiome, regardless of sex, demonstrated no differences in alpha diversity were detected between control (n = 15) and FGR (n = 18) at 4 weeks of life (Table 2). Beta diversity significantly differed between control and FGR offspring by Aitchison only (Figure 4A; Table 3). At the genus level, FGR animals had decreased relative abundance of Allobaculum, Sutterella (Figure 4B), Bifidobacterium (Figure 4C), Lactobacillus and among others and increased relative abundance of Turcibacter (Figure 4D), Dorea, and Roseburia (Table 6).
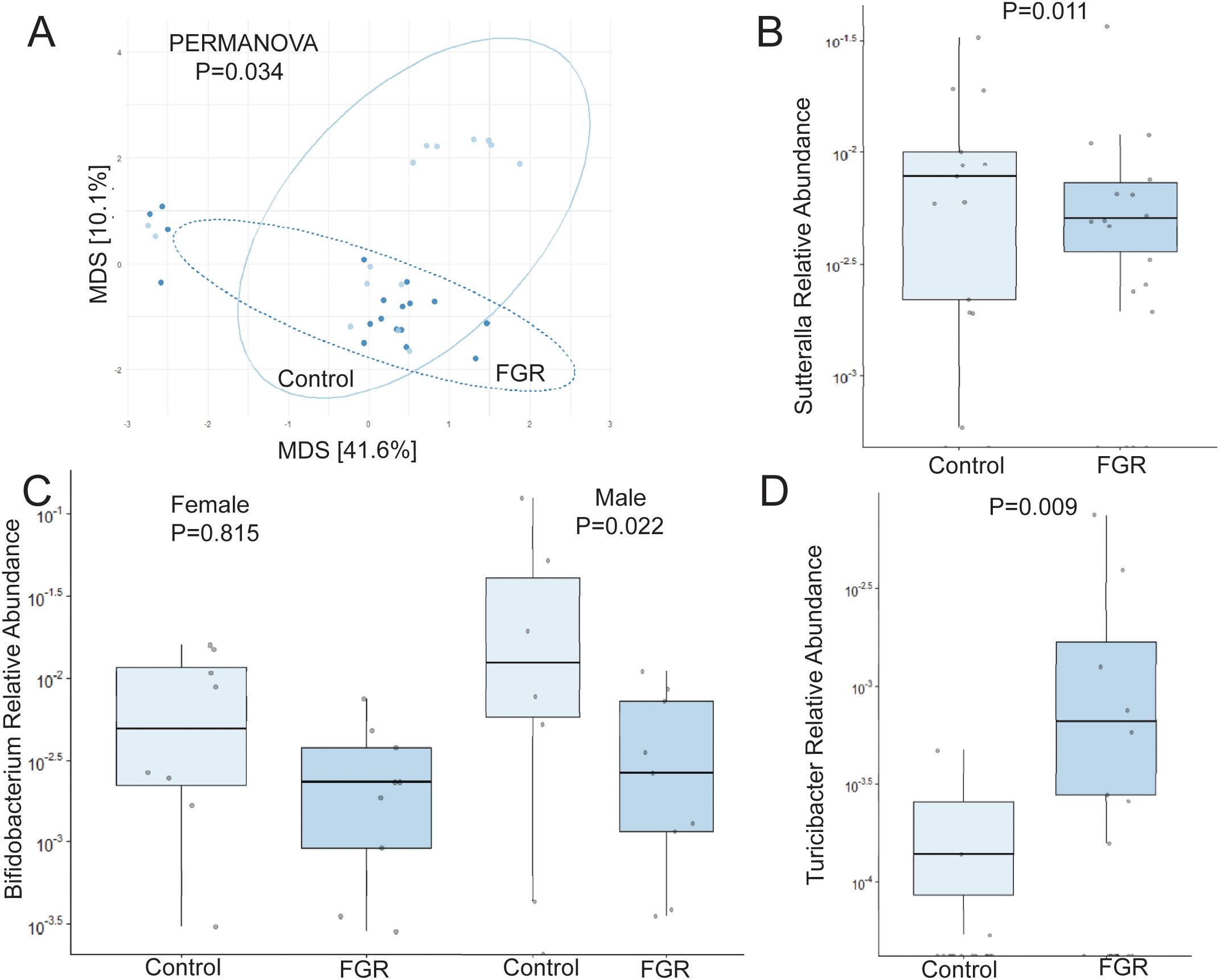
Figure 4. Fecal microbiome differences in control and fetal growth restricted offspring at age 4 weeks. (A) Aitchison distance in control and fetal growth restricted offspring at age 4 weeks. Relative abundance of (B) Sutterella (C) Bifidobacterium by offspring sex, and (D) Turicibacter in control and FGR offspring. FGR, fetal growth restriction.
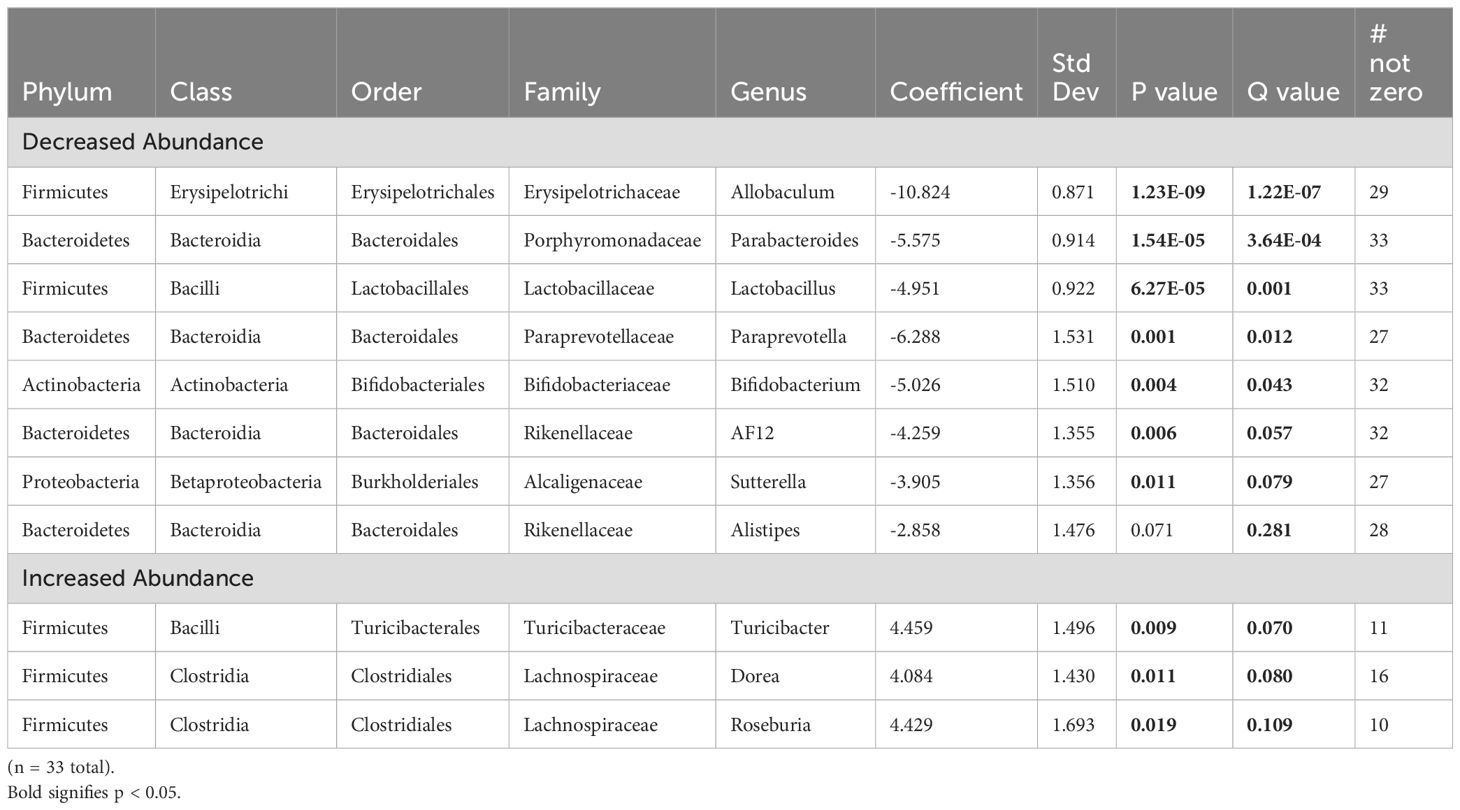
Table 6. Fecal microbiome from fetal growth restricted offspring compared to control offspring at 4 weeks of age.
As above, we tested for sex-specific differences between males (n = 7 control, n = 9 FGR) and females (n = 8 control, n = 9 FGR). For alpha diversity, two-way ANOVA revealed no significant associations between intrauterine growth and microbial diversity, nor any interaction between sex and growth (all p > 0.05). Beta diversity also did not differ between FGR and controls for males or females (Table 2). At the genus level, male FGR offspring had decreased relative abundance of Allobaculum, Bifidobacterium (Figure 4C), Lactobacillus and Alistipes among others and increased relative abundance of Dorea and Roseburia (Supplementary Table S2). FGR females had lower relative abundance of Rikenella, Akkermansia, and Bilophilia, and increased abundance of Dorea and Lactobacillus (Supplementary Table S2).
3.6 Associations between offspring weight and bacterial taxa at ages 3 and 4 weeks
We tested for associations between fecal microbial taxa and offspring weight at 21 and 28 days, and 16 weeks of life as well as weight gain between 21 and 28 days of life. At age 3 weeks, weight was aligned with Helicobacter, Turcibacter, and Prevotella abundances and weight at 4 weeks was negatively aligned with Allobaculum abundance. Weight at age 16 weeks was negatively aligned with Akkermansia abundance both age 3 weeks (Figure 5A) and age 4 weeks (Figure 5B).
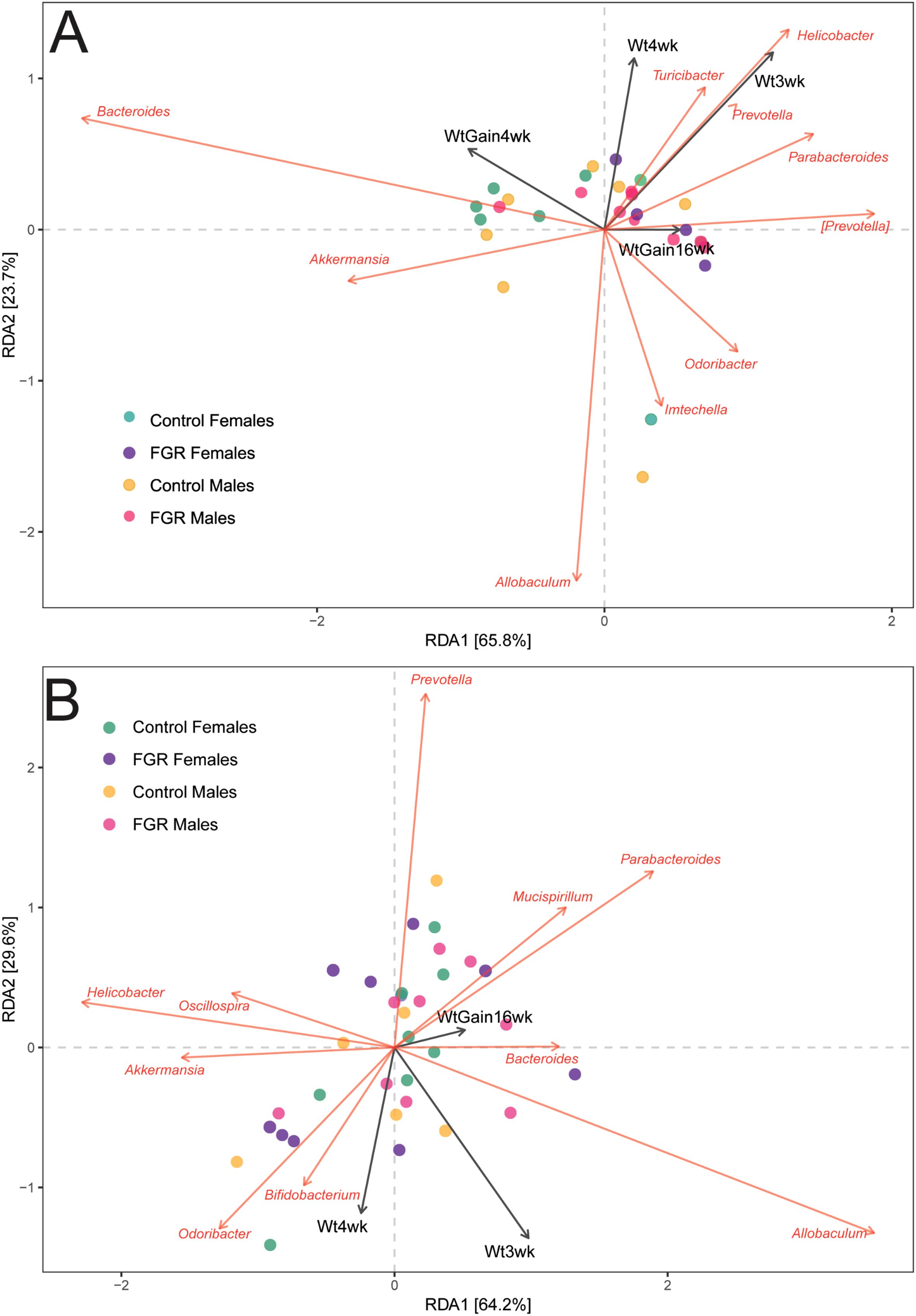
Figure 5. Association of offspring microbial abundance with weight outcomes. Distance-based redundancy analysis for offspring genus-level taxa with selected outcomes. (A) Microbiome at age 3 weeks; (B) Microbiome at age 4 weeks. Wt3wk, weight at age 3 weeks; Wt4wk, weight at age 4 weeks; WtGain4wk, weight gain from age 3 to 4 weeks; WtGain16wk, weight gain from age 3 to 16 weeks.
Using Pearson correlations, negative associations were noted in control animals after between several taxa and weight at age 3 weeks including Bifidobacterium, Dorea, Aldercreutzia, and Anaeroplasma (Supplementary Figure S2). In FGR animals, a positive association was noted between abundance of Dorea and Coprococcus with weight at age 3 weeks (Supplementary Figure S2, top panel) but did not remain significant after FDR correction (Supplementary Figure S2, bottom panel). There was no significant association with taxa at 3 weeks and 16-week weight. At age 4 weeks, no associations between microbial taxa and weight outcomes passed FDR correction in either FGR or control animals. With nominal p-values, we noted a positive association between body weight at 4 weeks and abundance of Bifidobacterium, Flexispira and Lactobacillus, and a negative association with Parabacteroides abundance for control animals only (Supplementary Figure S3). There were no significant associations in FGR animals, nor did we identify any significant association between bacterial taxa at 4 weeks and 16-week weight.
We also performed sex-stratified analyses at age 4 weeks. In males, no associations between microbial taxa and weight outcomes passed FDR correction in either FGR or control animals. With nominal p-values, we noted a positive association between Bifidobacterium and Flexispira abundances and body weight at age 4 weeks and weight gain between weeks 3 and 4, and negative association between Paraprevotella and Ruminococcus abundance and weight gain between weeks 3 and 4 only in control offspring (Supplementary Figure S4). In females, we similarly saw no associations between the microbiome and growth in FGR offspring with FDR correction (Supplementary Figure S5, bottom panel). With nominal p-values, we noted a negative association between relative abundance of Akkermansia and Parabacteroides and weight gain from 3 to 4 weeks, and a positive association between Blautia abundance and weight gain from 3 to 16 weeks (Supplementary Figure S5, top panel). In control females, we noted positive associations between body weight at 4 weeks with multiple taxa including Aldercreutzia, Coprococcus, Dorea, Roseburia, and Ruminococcus (Supplementary Figure S5).
4 Discussion
The ability to prevent fetal growth restriction and/or mitigate its adverse effects would impact the health of millions of children worldwide (3, 8, 47). Gut microbial dysbiosis is being increasingly recognized as a contributing mechanism to adverse pregnancy outcomes, including FGR (15, 48). Here, we used a mouse model of undernutrition to test for differences in maternal and offspring fecal microbial ecology and associations with pregnancy and offspring outcomes. Our findings indicate that calorie restriction during pregnancy impacts maternal gut microbiome with modest associations between microbial taxa and pregnancy outcomes that differed between control and calorie-restricted animals. Fecal microbiome showed further differences in offspring at two different time points but minimal associations with postnatal growth.
The present study builds upon our prior work demonstrating fecal microbial dysbiosis in adult FGR offspring (14). One mechanism underlying offspring dysbiosis could be inheritance of an abnormal microbiome during and after birth (48). It is important to note that our study was not designed to assess vertical transmission of the microbiome. Multiple studies have demonstrated similarities between parental and offspring fecal microbiota in humans (49) and mice (50). However, offspring microbiome in early developmental stages (weaning and immediately postweaning) is quite different than the adult microbiome. Further, coprophagia among mice results in rapid sharing of fecal microbes (51, 52), emphasizing the appropriateness of our study design. Future work could perform paired analyses of dams and their offspring and/or use metagenomic sequencing to make species and strain level comparisons.
Calorie-restricted dams had notable reduction in Turicibacter, a bacterium previously shown to modulate host bile acid and lipid metabolism (53) and to be associated with beneficial short-chain fatty acids (SCFAs) (54) and reduction of inflammation (55, 56). Our results are similar to previous studies which also showed lower Turicibacter abundance in the setting of stress during pregnancy, including heat stress in pigs (57) and HIV infection in humans (58). Turicibacter abundance is also reduced in mouse models of diet-induced obesity (59), colitis (56), and some gastrointestinal cancers (55). Although prior work demonstrated that Turicibacter is highly heritable (60), FGR offspring in this study had increased Turicibacter abundance at age 4 weeks, particularly among males. We did not observe significant differences in Turicibacter abundance at 3 weeks, nor at 16 weeks as previously published (14). Similarly, we previously described lower Turicibacter abundance in 1-month-old infants born to mothers with obesity (24) but not among the mothers during pregnancy at any timepoint (34). Because different strains may influence the role of this taxon (53), additional work is needed to determine the specific species and/or strains present and whether this differs between dams and offspring.
We identified a decrease in Rikenella during pregnancy in CR dams compared to control and in FGR female offspring at 4 weeks. Rikenella species have previously been shown to be decreased in piglets with FGR (61), mice with chronic stress (62) and diabetes (63), humans with obesity (64, 65), and infants born to mothers with gestational diabetes (42). Alistipes, also in the Rikenellaceae family, was decreased in FGR males at age 4 weeks, without detectable differences in dams. In contrast, maternal Alistipes abundance was decreased in human pregnancies impacted by FGR (15) and gestational diabetes (66) and may be associated with impaired glucose homeostasis in pregnancies impacted by overweight (67). The role of Alistipes in health is still being clarified and may depend not only on the species, but also on the specific disease being studied, possibly due to differential production of SCFAs (68). Rikenella and Alistipes were recently found to be higher in female mice exposed to heat stress to induce FGR (69). Therefore, the varying etiologies for FGR may differentially impact the maternal and offspring fecal microbiome and warrants further exploration.
Parabacteroides and Prevotella were enriched in CR dams at the end of pregnancy. Both taxa are important for carbohydrate degradation and are also enriched in women with obesity and gestational diabetes (70–74). Our findings are not consistent with those from humans showing an association between maternal and infant Parabacteroides abundance (72). In our model, 4-week-old male FGR offspring had reduced abundance of both Prevotella and Parabacteroides compared to controls, while in adulthood FGR females had increased Prevotella abundance only when challenged with a high fat diet (14). These findings should be viewed in light of a dynamic microbiome in the early stages of the post-weaning period. Our results highlight the complexity of fecal microbial establishment during infancy.
A notable finding from our prior work was depletion of the beneficial bacterium Akkermansia in adult FGR males (14). In the present work, we report decreased Akkermansia in FGR offspring at age 3 weeks. At that timepoint, 6 of 14 control offspring (3 male, 3 female) had detectable Akkermansia compared to 0 of 14 FGR offspring. There were no differences in CR dams compared to controls, suggesting against impaired vertical transmission as the mechanism for diminished abundance. Akkermansia has been showed to be reduced in pregnancies impacted by pre-eclampsia (39, 75) and gestational diabetes mellitus (76), both associated with adverse fetal weight outcomes. We also demonstrate negative alignment of Akkermansia abundance at ages 3 and 4 weeks with adult weight. Studies in both mice and humans have demonstrated a beneficial role of Akkermansia on insulin sensitivity and excess adiposity (77). Our results suggest that the role of Akkermansia in metabolic health may begin early in the lifecourse.
FGR offspring also had decreased relative abundance of Sutterella at both ages 3 and 4 weeks. In contrast, at age 16 weeks, female FGR offspring had increased relative abundance of Sutterella without difference among males (14). It is currently unclear whether the health impacts of Sutterella change across the lifespan. A recent report showed a positive association between Sutterella, specifically S. wadsworthensis, with obesity in children and adolescents (78). Some Sutterella species appear to have modest pro-inflammatory effects and may have a role in immune system modulation (79). Despite high reported heritability of Sutterella in humans based on maternal fecal samples (49), we did not detect statistical differences in dams at the end of pregnancy.
There is emerging evidence that maternal gut bacteria impact feto-placental development including offspring body weight in both animal models and humans (32, 33, 37, 38). Therefore, we examined if maternal or offspring taxa were associated with maternal gestational weight gain, fetal weight, and offspring weight. Our results revealed associations between several maternal taxa and offspring weight early in infancy, particularly a negative association with Bifidobacterium abundance. Our results contrast with a prior study showing benefit of Bifidobacterium supplementation on fetal weight gain in germ-free mice (32). One study in humans did not find any specific association between Bifidobacterium species and birth weight (38). The discrepancies could be related to the different conditions of pregnancy or potentially to different species of Bifidobacterium (80). At age 4 weeks we noted a positive association between body weight and Bifidobacterium abundance in offspring. Work in human infants showed a negative association of early life Bifidobacterium abundance with rapid infancy weight gain (26) while supplementation of malnourished infants with B. infantis resulted in faster weight gain (81). It is possible that Bifidobacterium species support a healthy weight trajectory with precise effects varying by the larger environmental context.
As with all research, the present study has some limitations. There are multiple other mouse models of FGR including low protein diet without calorie restriction (82), heat stress (69), hypoxia (83), uterine artery ligation (84, 85), administration of thromboxane A2 analogs (86), and genetic manipulation (87). Whether the results presented here predict changes in other pre-clinical models of FGR is unknown. However, our results are relevant in part because maternal malnutrition is one of the most common causes of FGR worldwide (8, 88). In addition, calorie restriction produces a more severe phenotype compared to many other models (14). Our ability to detect sex differences in offspring may have been limited by statistical power due to necessary adjustment for co-housing, especially at age 3 weeks. Reduced housing density after weaning may strengthen our findings in future studies but the logistics of such a study may be moderated by animal welfare concerns (52). Compared to other models of FGR, we do not see a prolonged postnatal impact on offspring growth and weight, as in our model offspring complete catch up growth by day 2 (14). We suspect this is due to adequate and possibly increased consumption of milk in the first two days of life compared to control animals, but this has not been tested. The similarity in offspring body weight at age 21 and 28 days could explain the absence of significant associations with taxa and offspring weight/gain.
In conclusion, we demonstrate gut microbial dysbiosis in pregnant dams and offspring at two timepoints following maternal calorie restriction. We also report associations between specific bacterial taxa and offspring fetal and postnatal growth. Combined with results from others, the results presented here may suggest a functional role of the microbiome in mediating the relationship between maternal malnutrition during pregnancy and adverse growth outcomes in offspring. Interventions targeting the microbiome either during pregnancy or in young offspring may help optimize growth and improve health.
Data availability statement
The original contributions presented in the study are publicly available. This data can be found in the Sequence Read Archive database (https://www.ncbi.nlm.nih.gov/sra) with accession number PRJNA1162649.
Ethics statement
The animal study was approved by University of Colorado Institutional Animal Care and Use Committee. The study was conducted in accordance with the local legislation and institutional requirements.
Author contributions
SG: Writing – review & editing, Writing – original draft, Visualization, Methodology, Investigation, Formal analysis, Data curation, Conceptualization. MR: Writing – review & editing, Writing – original draft, Visualization, Investigation, Formal analysis, Data curation. SC: Writing – review & editing, Methodology, Formal analysis, Data curation. CW: Funding acquisition, Writing – review & editing, Supervision, Conceptualization. PR: Writing – review & editing, Supervision, Funding acquisition, Conceptualization. KS: Visualization, Writing – review & editing, Supervision, Methodology, Funding acquisition, Formal analysis, Data curation, Conceptualization.
Funding
The author(s) declare financial support was received for the research, authorship, and/or publication of this article. These studies were supported in part by a grant from the Ludeman Family Center for Women’s Health Research (SG), and NIH grants 1R01HD111557 (PR) and R01HD107700 (CW). SG was supported by NIH T32DK007658. MR is supported by USDA-ARS Project #6026-51000-012-000D. CW was supported by R01DK084842. KS is supported in part by grants from the NIH (5P30DK048520 and 1R01HD102726) and funds from the Department of Pediatrics, University of Colorado Anschutz Medical Campus and the Anschutz Health and Wellness Center.
Acknowledgments
The authors would like to thank Lijun Zheng for assistance with animal care.
Conflict of interest
The authors declare that the research was conducted in the absence of any commercial or financial relationships that could be construed as a potential conflict of interest.
Publisher’s note
All claims expressed in this article are solely those of the authors and do not necessarily represent those of their affiliated organizations, or those of the publisher, the editors and the reviewers. Any product that may be evaluated in this article, or claim that may be made by its manufacturer, is not guaranteed or endorsed by the publisher.
Supplementary material
The Supplementary Material for this article can be found online at: https://www.frontiersin.org/articles/10.3389/fendo.2024.1423464/full#supplementary-material
Supplementary Figure S1 | Association of maternal genus-level microbial abundance with pregnancy and offspring outcomes without false discovery rate adjustment. Red-blue spectrum signifies positive to negative correlations, respectively. P values determined by Pearson correlations. *p < 0.05, **p < 0.01, ***p < 0.001, BW, body weight; wt, weight.
Supplementary Figure S2 | Association of offspring genus-level microbial abundance at 3 weeks with weight outcomes. Results are shown without (top) and with (bottom) False Discovery Rate Adjustment. Red-blue spectrum signifies positive to negative correlations, respectively. P values determined by Pearson correlations. *p < 0.05, **p < 0.01, ***p < 0.001, BW, body weight; wt, weight.
Supplementary Figure S3 | Association of offspring genus-level microbial abundance at 4 weeks with weight outcomes without false discovery rate adjustment. Red-blue spectrum signifies positive to negative correlations, respectively. P values determined by Pearson correlations. *p < 0.05, **p < 0.01, ***p < 0.001, BW, body weight; wk, week; wt, weight.
Supplementary Figure S4 | Association of male offspring genus-level microbial abundance at 4 weeks with weight outcomes without false discovery rate adjustment. Red-blue spectrum signifies positive to negative correlations, respectively. P values determined by Pearson correlations. *p < 0.05, **p < 0.01, ***p < 0.001, BW, body weight.
Supplementary Figure S5 | Association of female offspring genus-level microbial abundance at 4 weeks with weight outcomes. Results are shown without (top) and with (bottom) False Discovery Rate Adjustment. Red-blue spectrum signifies positive to negative correlations, respectively. P values determined by Pearson correlations. *p < 0.05, **p < 0.01, ***p < 0.001, BW, body weight; wk, week; wt, weight.
Abbreviations
CR, calorie restriction; dbRDA, distance-based redundancy analysis; FDR, False Discovery Rate; FGR, fetal growth restriction.
References
1. Beune IM, Bloomfield FH, Ganzevoort W, Embleton ND, Rozance PJ, van Wassenaer-Leemhuis AG, et al. Consensus based definition of growth restriction in the newborn. J Pediatr. (2018) 196:71–6.e1. doi: 10.1016/j.jpeds.2017.12.059
2. Lees CC, Romero R, Stampalija T, Dall’Asta A, DeVore GA, Prefumo F, et al. Clinical Opinion: The diagnosis and management of suspected fetal growth restriction: an evidence-based approach. Am J Obstet Gynecol. (2022) 226:366–78. doi: 10.1016/j.ajog.2021.11.1357
3. D’Agostin M, Di Sipio Morgia C, Vento G, Nobile S. Long-term implications of fetal growth restriction. World J Clin Cases. (2023) 11:2855–63. doi: 10.12998/wjcc.v11.i13.2855
4. Longo S, Bollani L, Decembrino L, Di Comite A, Angelini M, Stronati M. Short-term and long-term sequelae in intrauterine growth retardation (IUGR). J Matern Fetal Neonatal Med. (2013) 26:222–5. doi: 10.3109/14767058.2012.715006
5. Kesavan K, Devaskar SU. Intrauterine growth restriction: postnatal monitoring and outcomes. Pediatr Clin North Am. (2019) 66:403–23. doi: 10.1016/j.pcl.2018.12.009
6. Gantenbein KV, Kanaka-Gantenbein C. Highlighting the trajectory from intrauterine growth restriction to future obesity. Front Endocrinol (Lausanne). (2022) 13:1041718. doi: 10.3389/fendo.2022.1041718
7. Malhotra A, Allison BJ, Castillo-Melendez M, Jenkin G, Polglase GR, Miller SL. Neonatal morbidities of fetal growth restriction: pathophysiology and impact. Front Endocrinol (Lausanne). (2019) 10:55. doi: 10.3389/fendo.2019.00055
8. Hunter PJ, Awoyemi T, Ayede AI, Chico RM, David AL, Dewey KG, et al. Biological and pathological mechanisms leading to the birth of a small vulnerable newborn. Lancet. (2023) 401:1720–32. doi: 10.1016/S0140-6736(23)00573-1
9. Goyal D, Limesand SW, Goyal R. Epigenetic responses and the developmental origins of health and disease. J Endocrinol. (2019) 242:T105–T19. doi: 10.1530/JOE-19-0009
10. Manapurath R, Gadapani B, Pereira-da-Silva L. Body composition of infants born with intrauterine growth restriction: A systematic review and meta-analysis. Nutrients. (2022) 14:1085. doi: 10.3390/nu14051085
11. Hesse H, Palmer C, Rigdon CD, Galan HL, Hobbins JC, Brown LD. Differences in body composition and growth persist postnatally in fetuses diagnosed with severe compared to mild fetal growth restriction. J Neonatal Perinatal Med. (2022) 15:589–98. doi: 10.3233/NPM-210872
12. Sutherland MR, Black MJ. The impact of intrauterine growth restriction and prematurity on nephron endowment. Nat Rev Nephrol. (2023) 19:218–28. doi: 10.1038/s41581-022-00668-8
13. Boehmer BH, Limesand SW, Rozance PJ. The impact of IUGR on pancreatic islet development and beta-cell function. J Endocrinol. (2017) 235:R63–76. doi: 10.1530/JOE-17-0076
14. Gilley SP, Zarate MA, Zheng L, Jambal P, Yazza DN, Chintapalli SV, et al. Metabolic and fecal microbial changes in adult fetal growth restricted mice. Pediatr Res. (2023) 95:647–59. doi: 10.1038/s41390-023-02869-8
15. Tao Z, Chen Y, He F, Tang J, Zhan L, Hu H, et al. Alterations in the gut microbiome and metabolisms in pregnancies with fetal growth restriction. Microbiol Spectr. (2023) 11:e0007623. doi: 10.1128/spectrum.00076-23
16. Maggiotto LV, Ghosh S, Shin BC, Ganguly A, Lagishetty V, Jacobs JP, et al. Variation in the early life and adult intestinal microbiome of intra-uterine growth restricted rat offspring exposed to a high fat and fructose diet. Nutrients. (2023) 15:217. doi: 10.3390/nu15010217
17. Yang J, Hou L, Wang J, Xiao L, Zhang J, Yin N, et al. Unfavourable intrauterine environment contributes to abnormal gut microbiome and metabolome in twins. Gut. (2022) 71:2451–62. doi: 10.1136/gutjnl-2021-326482
18. Faas MM, Liu Y, Borghuis T, van Loo-Bouwman CA, Harmsen H, de Vos P. Microbiota induced changes in the immune response in pregnant mice. Front Immunol. (2019) 10:2976. doi: 10.3389/fimmu.2019.02976
19. Walker WA. The importance of appropriate initial bacterial colonization of the intestine in newborn, child, and adult health. Pediatr Res. (2017) 82:387–95. doi: 10.1038/pr.2017.111
20. Gensollen T, Iyer SS, Kasper DL, Blumberg RS. How colonization by microbiota in early life shapes the immune system. Science. (2016) 352:539–44. doi: 10.1126/science.aad9378
21. Sajankila N, Wala SJ, Ragan MV, Volpe SG, Dumbauld Z, Purayil N, et al. Current and future methods of probiotic therapy for necrotizing enterocolitis. Front Pediatr. (2023) 11:1120459. doi: 10.3389/fped.2023.1120459
22. Yuan Z, Yan J, Wen H, Deng X, Li X, Su S. Feeding intolerance alters the gut microbiota of preterm infants. PloS One. (2019) 14:e0210609. doi: 10.1371/journal.pone.0210609
23. Liu L, Ao D, Cai X, Huang P, Cai N, Lin S, et al. Early gut microbiota in very low and extremely low birth weight preterm infants with feeding intolerance: a prospective case-control study. J Microbiol. (2022) 60:1021–31. doi: 10.1007/s12275-022-2180-2
24. Gilley SP, Ruebel ML, Sims C, Zhong Y, Turner D, Lan RS, et al. Associations between maternal obesity and offspring gut microbiome in the first year of life. Pediatr Obes. (2022) 17:e12921. doi: 10.1111/ijpo.12921
25. Yong GJM, Porsche CE, Sitarik AR, Fujimura KE, McCauley K, Nguyen DT, et al. Precocious infant fecal microbiome promotes enterocyte barrier dysfuction, altered neuroendocrine signaling and associates with increased childhood obesity risk. Gut Microbes. (2024) 16:2290661. doi: 10.1080/19490976.2023.2290661
26. Alderete TL, Jones RB, Shaffer JP, Holzhausen EA, Patterson WB, Kazemian E, et al. Early life gut microbiota is associated with rapid infant growth in Hispanics from Southern California. Gut Microbes. (2021) 13:1961203. doi: 10.1080/19490976.2021.1961203
27. Odiase E, Frank DN, Young BE, Robertson CE, Kofonow JM, Davis KN, et al. The gut microbiota differ in exclusively breastfed and formula-fed United States infants and are associated with growth status. J Nutr. (2023) 153:2612–21. doi: 10.1016/j.tjnut.2023.07.009
28. Uzan-Yulzari A, Turta O, Belogolovski A, Ziv O, Kunz C, Perschbacher S, et al. Neonatal antibiotic exposure impairs child growth during the first six years of life by perturbing intestinal microbial colonization. Nat Commun. (2021) 12:443. doi: 10.1038/s41467-020-20495-4
29. Bailey LC, Forrest CB, Zhang P, Richards TM, Livshits A, DeRusso PA. Association of antibiotics in infancy with early childhood obesity. JAMA Pediatr. (2014) 168:1063–9. doi: 10.1001/jamapediatrics.2014.1539
30. Powell AM, Ali Khan FZ, Ravel J, Elovitz MA. Untangling associations of microbiomes of pregnancy and preterm birth. Clin Perinatol. (2024) 51:425–39. doi: 10.1016/j.clp.2024.02.009
31. Shen L, Wang W, Hou W, Jiang C, Yuan Y, Hu L, et al. The function and mechanism of action of uterine microecology in pregnancy immunity and its complications. Front Cell Infect Microbiol. (2022) 12:1025714. doi: 10.3389/fcimb.2022.1025714
32. Lopez-Tello J, Schofield Z, Kiu R, Dalby MJ, van Sinderen D, Le Gall G, et al. Maternal gut microbiota Bifidobacterium promotes placental morphogenesis, nutrient transport and fetal growth in mice. Cell Mol Life Sci. (2022) 79:386. doi: 10.1007/s00018-022-04379-y
33. Barrientos G, Ronchi F, Conrad ML. Nutrition during pregnancy: Influence on the gut microbiome and fetal development. Am J Reprod Immunol. (2024) 91:e13802. doi: 10.1111/aji.13802
34. Ruebel ML, Gilley SP, Sims CR, Zhong Y, Turner D, Chintapalli SV, et al. Associations between maternal diet, body composition and gut microbial ecology in pregnancy. Nutrients. (2021) 13:3295. doi: 10.3390/nu13093295
35. Strobel KM, Juul SE, Hendrixson DT. Maternal nutritional status and the microbiome across the pregnancy and the post-partum period. Microorganisms. (2023) 11:1569. doi: 10.3390/microorganisms11061569
36. Browne HP, Shao Y, Lawley TD. Mother-infant transmission of human microbiota. Curr Opin Microbiol. (2022) 69:102173. doi: 10.1016/j.mib.2022.102173
37. Pronovost GN, Yu KB, Coley-O’Rourke EJL, Telang SS, Chen AS, Vuong HE, et al. The maternal microbiome promotes placental development in mice. Sci Adv. (2023) 9:eadk1887. doi: 10.1126/sciadv.adk1887
38. Dreisbach C, Prescott S, Siega-Riz AM, McCulloch J, Habermeyer L, Dudley D, et al. Composition of the maternal gastrointestinal microbiome as a predictor of neonatal birth weight. Pediatr Res. (2023) 94:1158–65. doi: 10.1038/s41390-023-02584-4
39. Lv LJ, Li SH, Li SC, Zhong ZC, Duan HL, Tian C, et al. Early-onset preeclampsia is associated with gut microbial alterations in antepartum and postpartum women. Front Cell Infect Microbiol. (2019) 9:224. doi: 10.3389/fcimb.2019.00224
40. Zhao Y, Wang B, Zhao X, Cui D, Hou S, Zhang H. The effect of gut microbiota dysbiosis on patients with preeclampsia. Front Cell Infect Microbiol. (2022) 12:1022857. doi: 10.3389/fcimb.2022.1022857
41. Li X, Yu D, Wang Y, Yuan H, Ning X, Rui B, et al. The intestinal dysbiosis of mothers with gestational diabetes mellitus (GDM) and its impact on the gut microbiota of their newborns. Can J Infect Dis Med Microbiol. (2021) 2021:3044534. doi: 10.1155/2021/3044534
42. Soderborg TK, Carpenter CM, Janssen RC, Weir TL, Robertson CE, Ir D, et al. Gestational diabetes is uniquely associated with altered early seeding of the infant gut microbiota. Front Endocrinol (Lausanne). (2020) 11:603021. doi: 10.3389/fendo.2020.603021
43. Tu X, Duan C, Lin B, Li K, Gao J, Yan H, et al. Characteristics of the gut microbiota in pregnant women with fetal growth restriction. BMC Pregnancy Childbirth. (2022) 22:297. doi: 10.1186/s12884-022-04635-w
44. McMurdie PJ, Holmes S. phyloseq: an R package for reproducible interactive analysis and graphics of microbiome census data. PloS One. (2013) 8:e61217. doi: 10.1371/journal.pone.0061217
45. Liu C, Cui Y, Li X, Yao M. microeco: an R package for data mining in microbial community ecology. FEMS Microbiol Ecol. (2021) 97:fiaa255. doi: 10.1093/femsec/fiaa255
46. Mallick H, Rahnavard A, McIver LJ, Ma S, Zhang Y, Nguyen LH, et al. Multivariable association discovery in population-scale meta-omics studies. PloS Comput Biol. (2021) 17:e1009442. doi: 10.1371/journal.pcbi.1009442
47. Lawn JE, Ohuma EO, Bradley E, Idueta LS, Hazel E, Okwaraji YB, et al. Small babies, big risks: global estimates of prevalence and mortality for vulnerable newborns to accelerate change and improve counting. Lancet. (2023) 401:1707–19. doi: 10.1016/S0140-6736(23)00522-6
48. Simon D, Kellermayer R. Disturbed pediatric gut microbiome maturation in the developmental origins of subsequent chronic disease. J Pediatr Gastroenterol Nutr. (2023) 76:123–7. doi: 10.1097/MPG.0000000000003664
49. Podlesny D, Fricke WF. Strain inheritance and neonatal gut microbiota development: A meta-analysis. Int J Med Microbiol. (2021) 311:151483. doi: 10.1016/j.ijmm.2021.151483
50. Wymore Brand M, Proctor AL, Hostetter JM, Zhou N, Friedberg I, Jergens AE, et al. Vertical transmission of attaching and invasive E. coli from the dam to neonatal mice predisposes to more severe colitis following exposure to a colitic insult later in life. PloS One. (2022) 17:e0266005. doi: 10.1371/journal.pone.0266005
51. Vasquez KS, Willis L, Cira NJ, Ng KM, Pedro MF, Aranda-Díaz A, et al. Quantifying rapid bacterial evolution and transmission within the mouse intestine. Cell Host Microbe. (2021) 29:1454–68.e4. doi: 10.1016/j.chom.2021.08.003
52. Russell A, Copio JN, Shi Y, Kang S, Franklin CL, Ericsson AC. Reduced housing density improves statistical power of murine gut microbiota studies. Cell Rep. (2022) 39:110783. doi: 10.1016/j.celrep.2022.110783
53. Lynch JB, Gonzalez EL, Choy K, Faull KF, Jewell T, Arellano A, et al. Gut microbiota Turicibacter strains differentially modify bile acids and host lipids. Nat Commun. (2023) 14:3669. doi: 10.1038/s41467-023-39403-7
54. Zhong Y, Nyman M, Fak F. Modulation of gut microbiota in rats fed high-fat diets by processing whole-grain barley to barley malt. Mol Nutr Food Res. (2015) 59:2066–76. doi: 10.1002/mnfr.201500187
55. Chu F, Li Y, Meng X, Li Y, Li T, Zhai M, et al. Gut microbial dysbiosis and changes in fecal metabolic phenotype in precancerous lesions of gastric cancer induced with N-methyl-N’-nitro-N-nitrosoguanidine, sodium salicylate, ranitidine, and irregular diet. Front Physiol. (2021) 12:733979. doi: 10.3389/fphys.2021.733979
56. Jones-Hall YL, Kozik A, Nakatsu C. Ablation of tumor necrosis factor is associated with decreased inflammation and alterations of the microbiota in a mouse model of inflammatory bowel disease. PloS One. (2015) 10:e0119441. doi: 10.1371/journal.pone.0119441
57. He J, Zheng W, Tao C, Guo H, Xue Y, Zhao R, et al. Heat stress during late gestation disrupts maternal microbial transmission with altered offspring’s gut microbial colonization and serum metabolites in a pig model. Environ pollut. (2020) 266:115111. doi: 10.1016/j.envpol.2020.115111
58. Chandiwana P, Munjoma PT, Mazhandu AJ, Li J, Baertschi I, Wyss J, et al. Antenatal gut microbiome profiles and effect on pregnancy outcome in HIV infected and HIV uninfected women in a resource limited setting. BMC Microbiol. (2023) 23:4. doi: 10.1186/s12866-022-02747-z
59. Jiao N, Baker SS, Nugent CA, Tsompana M, Cai L, Wang Y, et al. Gut microbiome may contribute to insulin resistance and systemic inflammation in obese rodents: a meta-analysis. Physiol Genomics. (2018) 50:244–54. doi: 10.1152/physiolgenomics.00114.2017
60. Goodrich JK, Davenport ER, Beaumont M, Jackson MA, Knight R, Ober C, et al. Genetic determinants of the gut microbiome in UK twins. Cell Host Microbe. (2016) 19:731–43. doi: 10.1016/j.chom.2016.04.017
61. Tang W, Zhang W, Azad MAK, Ma C, Zhu Q, Kong X. Metabolome, microbiome, and gene expression alterations in the colon of newborn piglets with intrauterine growth restriction. Front Microbiol. (2022) 13:989060. doi: 10.3389/fmicb.2022.989060
62. Gao X, Cao Q, Cheng Y, Zhao D, Wang Z, Yang H, et al. Chronic stress promotes colitis by disturbing the gut microbiota and triggering immune system response. Proc Natl Acad Sci U S A. (2018) 115:E2960–e9. doi: 10.1073/pnas.1720696115
63. Cai TT, Ye XL, Li RR, Chen H, Wang YY, Yong HJ, et al. Resveratrol modulates the gut microbiota and inflammation to protect against diabetic nephropathy in mice. Front Pharmacol. (2020) 11:1249. doi: 10.3389/fphar.2020.01249
64. Palmas V, Pisanu S, Madau V, Casula E, Deledda A, Cusano R, et al. Gut microbiota markers associated with obesity and overweight in Italian adults. Sci Rep. (2021) 11:5532. doi: 10.1038/s41598-021-84928-w
65. Peters BA, Shapiro JA, Church TR, Miller G, Trinh-Shevrin C, Yuen E, et al. A taxonomic signature of obesity in a large study of American adults. Sci Rep. (2018) 8:9749. doi: 10.1038/s41598-018-28126-1
66. Teixeira RA, Silva C, Ferreira AC, Martins D, Leite-Moreira A, Miranda IM, et al. The association between gestational diabetes and the microbiome: A systematic review and meta-analysis. Microorganisms. (2023) 11:1749. doi: 10.3390/microorganisms11071749
67. Lotankar M, Mokkala K, Houttu N, Koivuniemi E, Sørensen N, Nielsen HB, et al. Distinct diet-microbiota-metabolism interactions in overweight and obese pregnant women: a metagenomics approach. Microbiol Spectr. (2022) 10:e0089321. doi: 10.1128/spectrum.00893-21
68. Parker BJ, Wearsch PA, Veloo ACM, Rodriguez-Palacios A. The genus alistipes: gut bacteria with emerging implications to inflammation, cancer, and mental health. Front Immunol. (2020) 11:906. doi: 10.3389/fimmu.2020.00906
69. Zheng X, Ma W, Wang Y, Wu C, Wang J, Ma Z, et al. Heat stress-induced fetal intrauterine growth restriction is associated with elevated LPS levels along the maternal intestine-placenta-fetus axis in pregnant mice. J Agric Food Chem. (2023) 71:19592–609. doi: 10.1021/acs.jafc.3c07058
70. Hasain Z, Mokhtar NM, Kamaruddin NA, Mohamed Ismail NA, Razalli NH, Gnanou JV, et al. Gut microbiota and gestational diabetes mellitus: A review of host-gut microbiota interactions and their therapeutic potential. Front Cell Infect Microbiol. (2020) 10:188. doi: 10.3389/fcimb.2020.00188
71. Kuang YS, Lu JH, Li SH, Li JH, Yuan MY, He JR, et al. Connections between the human gut microbiome and gestational diabetes mellitus. Gigascience. (2017) 6:1–12. doi: 10.1093/gigascience/gix058
72. Stanislawski MA, Dabelea D, Wagner BD, Sontag MK, Lozupone CA, Eggesbo M. Pre-pregnancy weight, gestational weight gain, and the gut microbiota of mothers and their infants. Microbiome. (2017) 5:113. doi: 10.1186/s40168-017-0332-0
73. Ridaura VK, Faith JJ, Rey FE, Cheng J, Duncan AE, Kau AL, et al. Gut microbiota from twins discordant for obesity modulate metabolism in mice. Science. (2013) 341:1241214. doi: 10.1126/science.1241214
74. Verdam FJ, Fuentes S, de Jonge C, Zoetendal EG, Erbil R, Greve JW, et al. Human intestinal microbiota composition is associated with local and systemic inflammation in obesity. Obes (Silver Spring). (2013) 21:E607–15. doi: 10.1002/oby.20466
75. Chen X, Li P, Liu M, Zheng H, He Y, Chen MX, et al. Gut dysbiosis induces the development of pre-eclampsia through bacterial translocation. Gut. (2020) 69:513–22. doi: 10.1136/gutjnl-2019-319101
76. Cortez RV, Taddei CR, Sparvoli LG, Angelo AGS, Padilha M, Mattar R, et al. Microbiome and its relation to gestational diabetes. Endocrine. (2019) 64:254–64. doi: 10.1007/s12020-018-1813-z
77. Ghotaslou R, Nabizadeh E, Memar MY, Law WMH, Ozma MA, Abdi M, et al. The metabolic, protective, and immune functions of Akkermansia muciniphila. Microbiol Res. (2023) 266:127245. doi: 10.1016/j.micres.2022.127245
78. Squillario M, Bonaretti C, La Valle A, Di Marco E, Piccolo G, Minuto N, et al. Gut-microbiota in children and adolescents with obesity: inferred functional analysis and machine-learning algorithms to classify microorganisms. Sci Rep. (2023) 13:11294. doi: 10.1038/s41598-023-36533-2
79. Hiippala K, Kainulainen V, Kalliomäki M, Arkkila P, Satokari R. Mucosal prevalence and interactions with the epithelium indicate commensalism of sutterella spp. Front Microbiol. (2016) 7:1706. doi: 10.3389/fmicb.2016.01706
80. Stuivenberg GA, Burton JP, Bron PA, Reid G. Why are bifidobacteria important for infants? Microorganisms. (2022) 10:278. doi: 10.3390/microorganisms10020278
81. Barratt MJ, Nuzhat S, Ahsan K, Frese SA, Arzamasov AA, Sarker SA, et al. Bifidobacterium infantis treatment promotes weight gain in Bangladeshi infants with severe acute malnutrition. Sci Transl Med. (2022) 14:eabk1107. doi: 10.1126/scitranslmed.abk1107
82. Berends LM, Dearden L, Tung YCL, Voshol P, Fernandez-Twinn DS, Ozanne SE. Programming of central and peripheral insulin resistance by low birthweight and postnatal catch-up growth in male mice. Diabetologia. (2018) 61:2225–34. doi: 10.1007/s00125-018-4694-z
83. Wang KCW, Larcombe AN, Berry LJ, Morton JS, Davidge ST, James AL, et al. Foetal growth restriction in mice modifies postnatal airway responsiveness in an age and sex-dependent manner. Clin Sci (Lond). (2018) 132:273–84. doi: 10.1042/CS20171554
84. Janot M, Cortes-Dubly ML, Rodriguez S, Huynh-Do U. Bilateral uterine vessel ligation as a model of intrauterine growth restriction in mice. Reprod Biol Endocrinol. (2014) 12:62. doi: 10.1186/1477-7827-12-62
85. Habli M, Jones H, Aronow B, Omar K, Crombleholme TM. Recapitulation of characteristics of human placental vascular insufficiency in a novel mouse model. Placenta. (2013) 34:1150–8. doi: 10.1016/j.placenta.2013.09.011
86. Gibbins KJ, Gibson-Corley KN, Brown AS, Wieben M, Law RC, Fung CM. Effects of excess thromboxane A2 on placental development and nutrient transporters in a Mus musculus model of fetal growth restriction. Biol Reprod. (2018) 98:695–704. doi: 10.1093/biolre/ioy006
87. Kaur G, Porter CBM, Ashenberg O, Lee J, Riesenfeld SJ, Hofree M, et al. Mouse fetal growth restriction through parental and fetal immune gene variation and intercellular communications cascade. Nat Commun. (2022) 13:4398. doi: 10.1038/s41467-022-32171-w
Keywords: microbiome, fetal growth restriction, calorie restriction, pregnancy, growth
Citation: Gilley SP, Ruebel ML, Chintapalli SV, Wright CJ, Rozance PJ and Shankar K (2024) Calorie restriction during gestation impacts maternal and offspring fecal microbiome in mice. Front. Endocrinol. 15:1423464. doi: 10.3389/fendo.2024.1423464
Received: 25 April 2024; Accepted: 28 August 2024;
Published: 04 October 2024.
Edited by:
Xiaomin Xiao, First Affiliated Hospital of Jinan University, ChinaReviewed by:
Yunyun Liu, Sun Yat-sen Memorial Hospital, ChinaNicoletta Di Simone, Humanitas University, Italy
Bei Shi, China Medical University, China
Copyright © 2024 Gilley, Ruebel, Chintapalli, Wright, Rozance and Shankar. This is an open-access article distributed under the terms of the Creative Commons Attribution License (CC BY). The use, distribution or reproduction in other forums is permitted, provided the original author(s) and the copyright owner(s) are credited and that the original publication in this journal is cited, in accordance with accepted academic practice. No use, distribution or reproduction is permitted which does not comply with these terms.
*Correspondence: Stephanie P. Gilley, U3RlcGhhbmllLkdpbGxleUBjdWFuc2NodXR6LmVkdQ==