- 1The 1st Affiliate Hospital of Changsha Medical University, Changsha Medical University, Changsha, China
- 2Hunan Key Laboratory of the Research and Development of Novel Pharmaceutical Preparations, School of Pharmaceutical Science, Changsha Medical University, Changsha, China
- 3School of Life Sciences, Beijing University of Chinese Medicine, Beijing, China
- 4The Hunan Provincial Key Laboratory of the TCM Agricultural Biogenomics, Changsha Medical University, Changsha, China
Diabetes and its complications significantly affect individuals’ quality of life. The etiology of diabetes mellitus and its associated complications is complex and not yet fully understood. There is an increasing emphasis on investigating the effects of endocrine disruptors on diabetes, as these substances can impact cellular processes, energy production, and utilization, ultimately leading to disturbances in energy homeostasis. Mitochondria play a crucial role in cellular energy generation, and any impairment in these organelles can increase susceptibility to diabetes. This review examines the most recent epidemiological and pathogenic evidence concerning the link between endocrine disruptors and diabetes, including its complications. The analysis suggests that endocrine disruptor-induced mitochondrial dysfunction—characterized by disruptions in the mitochondrial electron transport chain, dysregulation of calcium ions (Ca2+), overproduction of reactive oxygen species (ROS), and initiation of signaling pathways related to mitochondrial apoptosis—may be key mechanisms connecting endocrine disruptors to the development of diabetes and its complications.
Introduction
According to the most recent epidemiological survey data from 2021, an estimated 537 million individuals were diagnosed with diabetes, 541 million had impaired glucose tolerance, and diabetes was associated with 6.7 million deaths (1). Given the continual increase in the diabetic patient population, projections suggest that the global diabetic population will rise to 783 million by the year 2045 (1). Effective management of blood glucose levels and clinical outcomes for diabetic patients remains suboptimal, often resulting in complications that affect multiple organs, including the eyes, kidneys, heart, and peripheral nerves (2–10). Diabetes and its complications pose a significant burden (11, 12). Diabetes has various known risk factors (13–16), and methods for diabetes prevention and treatment have been investigated and developed (17–20). However, the current understanding of the pathogenesis of diabetes and its associated complications remains incomplete, which hinders the development of effective clinical prevention and treatment strategies.
Endocrine disruptors encompass a wide array of exogenous chemicals, comprising approximately 1,000 types, including substances such as nonylphenol, pesticides, metals, fungicides, and others (21). These substances are ubiquitous in human living environments, present in items such as furniture, paint, flooring, electronic devices, toys, food packaging, bottled beverages, cosmetics, receipts, clothing, food, contact lenses, and dental sealants. As modern civilization advances, the growing demand for novel chemicals has heightened human exposure to endocrine-disrupting chemicals (EDCs). It is estimated that 147 of these substances may persist in the environment or are produced in large quantities, thereby posing a significant risk to human health (22, 23). A growing body of research indicates that EDCs increase the risk of various metabolic diseases, including obesity (24–31), diabetes and its complications (32–43), and metabolic syndrome (44–51) . For example, studies have demonstrated that long-term daily exposure to EDCs, even at concentrations below the established tolerance thresholds for individual substances, can significantly increase the risk of diabetes in both women and men (52). However, these studies did not explore the specific mechanisms that underlying the influence of endocrine disruptors on diabetes and its complications.
The increasing number of studies establishing a connection between endocrine disruptors and diabetes, along with its complications, via mitochondrial dysfunction, may be attributed to the interaction between mitochondria and hormone secretion, the generation of reactive oxygen species (ROS), and their effects on metabolic homeostasis (53–57). Mitochondria are essential cellular components that play a crucial role in energy metabolism (58). Adenosine triphosphate (ATP) is primarily produced in the mitochondria through the coupling of the tricarboxylic acid cycle (TCA) with oxidative phosphorylation (OXPHOS) (59, 60). Disruption in this process caused by endocrine disruptors can result in mitochondrial damage and dysfunction, leading to increased production of superoxide free radicals and ultimately raising the risk of developing diabetes (61). Nonetheless, further exploration is required to elucidate the exact role of endocrine disruptors in initiating diabetes and its complications through mitochondrial dysfunction. This study will review the most recent literature from the past five years, beginning with an overview of the latest evidence regarding various endocrine disruptors associated with the onset of diabetes and its complications. Following this, the study will elucidate the mechanisms through which mitochondrial dysfunction contributes to the development of diabetes induced by endocrine disruptors. Lastly, it will outline how mitochondrial dysfunction, triggered by endocrine disruptors, leads to diabetic complications. Table 1 summarizes the literature cited in this review, primarily from clinical studies.
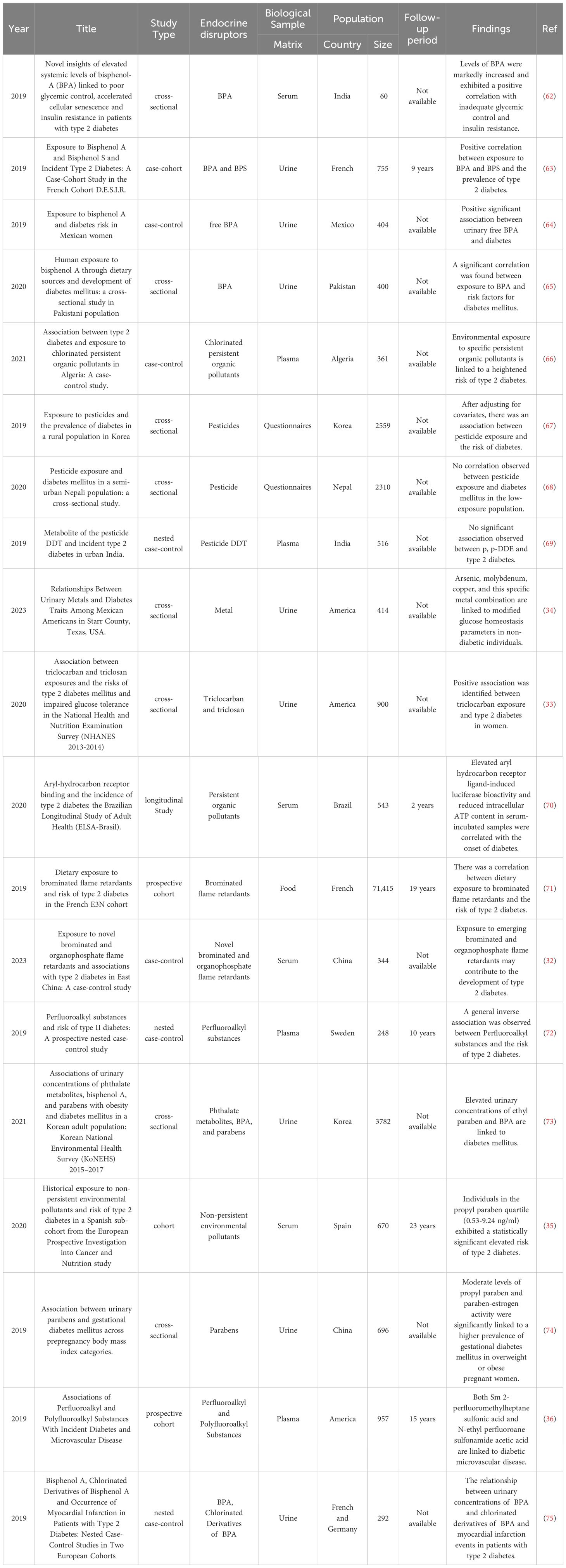
Table 1. Endocrine disruptors and their relationship with diabetes and its complications in clinical studies.
Endocrine disruptors and diabetes mellitus
Endocrine disruptors are primarily classified into two groups: persistent endocrine disruptors and non-persistent endocrine disruptors. Studies consistently shown that among persistent endocrine disruptors, the relationship between persistent organic pollutants (POPs) and type 2 diabetes (T2D) is notably significant (76). Major POPs include organochlorine pesticides (OCPs) such as dichlorodiphenyldichloroethylene (DDE) and its metabolite chlordane, brominated flame retardants (BFRs), and per- and polyfluoroalkyl substances (PFAS). A prospective study conducted in Brazil enrolled 71 diabetes cases and 472 randomly selected controls. The study assessed AhR ligand bioactivity (AhRL), which reflects the cumulative effect of various POPs, and intracellular ATP levels, indicative of mitochondrial inhibitory substances (MIS). The findings indicated that, in comparison with the control group, the diabetes group exhibited a significant 6.2% reduction in MIS-ATP levels (P < 0.0001). In crude analysis, participants with AhRL levels above the median had a higher risk of diabetes (hazard ratio (HR) = 1.85; 95% confidence interval (CI): 1.11-3.06), while those with MIS-ATP levels below median faced an increased diabetes risk (HR = 3.62; 95% CI: 2.06-6.38). Furthermore, individuals with elevated AhRL and low MIS-ATP showed a significantly heightened diabetes risk when compared with those with low AhRL and high MIS-ATP (HR = 8.15; 95% CI: 2.86-23.25), which was slightly attenuated after adjusting for smoking (HR = 6.9; 95% CI: 2.4-20) (70). This indirectly suggests that over an extended period exposure to POPs may lead to the development of diabetes. Additionally, findings from a case-control study conducted in Algeria indicated that, after adjusting for known risk factors for T2D in Algeria, environmental exposure to OCPs could significantly increase the likelihood of developing T2D. Specifically, the study highlighted associations with 4,4′-DDE (odds ratio (OR): 12.58; 95% CI: 4.76-33.26) and hexachlorobenzene (OR: 3.69; 95% CI: 1.90-7.15) (66). Moreover, findings from a meta-analysis study suggested a significantly elevated risk of diabetes associated with increased exposure to the organochlorine insecticide chlordane (77). In addition to OCPs, there is increasing evidence that exposure to organophosphorus pesticides may also increase the risk of T2D (78). Additionally, a cross-sectional study conducted in Korea revealed significant associations between pesticide exposure variables and the prevalence of diabetes in the Korean population, after adjusting for potential risk factors such as age, gender, monthly income, and education level. These variables included pesticide use (OR: 1.58; 95% CI: 1.13-2.21), duration of use (>20 years) (OR: 1.51; 95% CI: 1.07-2.14), frequency of use (more than 10 days per year) (OR: 1.53; 95% CI: 1.09-2.15), intensity of exposure (low intensity: OR: 1.55; 95% CI: 1.07-2.24, high intensity: OR: 1.53; 95% CI: 1.06-2.22), and cumulative exposure index (high exposure: OR: 1.54; 95% CI: 1.03-2.30). This association was particularly pronounced among individuals who were overweight or obese (67). Conversely, no correlation was observed between pesticide exposure and T2D in study populations predominantly comprising farmers in Nepal and India (68, 69). Thus, the current data on pesticide-induced diabetes lack consistency, which may stem from variations in experimental designs. In studies from Korea and Nepal, pesticide exposure was assessed solely through questionnaires, which are vulnerable to recall bias. Conversely, the study from India evaluated pesticide exposure using biomarkers, specifically measuring blood p,p-DDE concentration once, which may not accurately reflect lifetime exposure. Moreover, the use of a single-pesticide model in the study does not eliminate the potential confounding effects of other pesticide residues. BFRs are prominent POPs. Findings from a French E3N cohort study suggested that dietary exposure to BFRs might increase the risk of developing T2D (71). Moreover, a case-control study conducted in China, involving 172 individuals with T2D and 172 controls, indicated that exposure to novel BFRs and organophosphorus flame retardants may contribute to the development of T2D (32). PFAS constitute a substantial category of highly fluorinated organic compounds known for their exceptional persistence. A conditional logistic regression from a prospective nested case-control study found that, after adjusting for gender, age, sample year, diet, and body mass index, long-term exposure to PFAS was potentially associated with reduced insulin resistance in individuals without T2D (72).
Non-persistent endocrine disruptors mainly include bisphenol A (BPA), heavy metals, and fungicides. BPA is a primary component of polycarbonate plastics, and studies have detected BPA in over 95% of the global population (79), making it one of the most widespread endocrine disruptors. Several observational studies have shown a significant correlation between exposure to BPA and insulin resistance, disrupted glucose homeostasis, and the development of T2D in diverse racial or population groups (62–65). Parabens are antimicrobial preservatives frequently employed in consumer goods and are classified as non-persistent environmental contaminants. This group encompasses methylparaben, ethyl paraben, propylparaben, and butylparaben. A cross-sectional study conducted in Korea identified ethyl paraben as potential risk factors for T2D after adjusting for covariates (73). Additionally, a cohort study conducted in Spain (n=670) assessed exposure through chemical analysis of serum samples collected at recruitment. After a median follow-up of 23 years, 182 cases (27%) of T2D were diagnosed. The results of the Cox proportional hazards model showed that individuals with propylparaben levels between 0.53 and 9.24 ng/ml had a statistically significant increased risk of T2D (HR = 1.668, P = 0.012) (35). Nevertheless, a study conducted in China did not observe a relationship between paraben exposure and gestational diabetes mellitus in the overall population (74). A study involving 414 Mexican Americans revealed that elevated levels of urinary arsenic, molybdenum, copper, and a combination of these metals could disrupt insulin-glucose homeostasis and fasting insulin levels (34). In another cross-sectional study involving 900 American participants, after adjusting for potential confounders, exposure to fungicides (specifically triclocarban) was found to elevate the risk of T2D in women (OR: 1.79; 95% CI: 1.05–2.05) (33). Studies on non-persistent endocrine disruptors, despite using exposure assessments like biomarkers such as blood or urine measurements to mitigate recall bias from questionnaire surveys, are inherently limited by their observational nature. They cannot definitively rule out the influence of other potential confounding factors. EDCs tend to be highly correlated with each other, and individuals are commonly exposed to mixtures of various types, which complicates the precise determination of the independent effects of each compound. Mendelian randomization (MR) analysis is an epidemiological method that uses genetic variation associated with the exposure being studied as a tool to reduce biases from confounding factors and address reverse causation (80–83). Thus, future research could benefit from MR studies to explore causal relationships between EDC exposure and health outcomes.
Recent research on the relationship between EDCs and diabetes has increased, yet determining which type of EDC has a greater impact on diabetes remains challenging. This difficulty arises not only from the vast variety of EDCs and their widespread presence in human environments (84), but also from the fact that EDCs rarely act independently. They often work synergistically and cumulatively, with long latency periods of exposure (21). Diseases caused by EDCs may not become apparent during the exposure period but might manifest in adulthood or old age. Moreover, the adverse effects of EDCs can be transmitted to subsequent generations, even if those generations are not directly exposed (85). These factors collectively increase the complexity of studying the effects of EDCs on human health.
The role of mitochondrial dysfunction in mediating the impact of endocrine disruptors on diabetes
Diabetes is well recognized as a disease stemming from pancreatic β-cell dysfunction influenced by various factors, often involving ROS generation, primarily originating from mitochondria. Mitochondria serve a pivotal role in ATP production via OXPHOS (86). Under normal circumstances, acetyl-CoA derived from lipid and glucose metabolism enters the mitochondrial matrix to engage in the TCA cycle. During this process, substrates undergo oxidation, yielding CO2, flavin adenine dinucleotide (FADH2), and nicotinamide adenine dinucleotide (NADH) (59, 86–88). Electrons from NADH and FADH2 are accepted by Complexes I and II, respectively, and subsequently transferred through ubiquinone (Q) and cytochrome c (C) to Complexes III and IV. Molecular oxygen acts as the final electron acceptor at Complex IV, converting to water. Throughout this redox process, Complexes I, II, and IV facilitate proton pumping from the matrix into the intermembrane space, thereby establishing an electrochemical gradient (membrane potential) critical for ATP synthesis (86, 89). The integrity of this mitochondrial electron transport chain is essential for sustained electron transfer. Disruption of this chain can lead to heightened ROS production, diminished ATP levels, disturbances in calcium ion (Ca2+) homeostasis, alterations in membrane permeability, structural impairments in mitochondria, and potential cellular demise (90–92).
Ca2+ acts as a signaling molecule that enhances OXPHOS, including the TCA cycle and the subsequent electron transport chain. An animal study found that when zebrafish liver mitochondria were exposed to OCP mixture, the mitochondria in the exposed group absorbed Ca2+ more quickly than those in the unexposed group. Additionally, the activity of mitochondrial complex III was reduced (93). This suggests that the OCP mixture can promote calcium influx by regulating mitochondrial calcium channels, leading to mitochondrial swelling. Consequently, this affects OXPHOS and disrupts the electron transport chain, causing the mitochondrial membrane potential to collapse (94), limiting ATP production, and resulting in a significant accumulation of ROS, ultimately leading to cell death (95). Additionally, another animal study exposed mitochondria isolated from pre-diabetic rats to a combination of low concentrations of arsenic trioxide (ATO) (IC25 = 40 μM) and hyperglycemic conditions (glucose levels at 20, 40, 80, and 160 mM) or pyruvate (PYR, 20,40,80,160 mM). The results showed that when mitochondria were exposed to various concentrations of glucose alone or in combination with a lower dose of ATO, there were no adverse effects on the mitochondria. However, exposure to a higher concentration of PYR(≥40mM) with ATO (40 μM) resulted in decreased mitochondrial dehydrogenase activity (complex II), increased mitochondrial ROS (mtROS) production, lipid peroxidation, glutathione depletion, and ultimately, mitochondrial membrane damage and swelling (96). In an experiment with RIN-m5F cells, it was found that the activation of ROS-activated JNK signaling is a key mechanism in methylmercury (MeHg)-induced mitochondria-dependent apoptosis. After treatment with MeHg (2μM), there was mitochondrial dysfunction, evidenced by the depolarization of the mitochondrial membrane potential and increased cytochrome c (C) release. Apoptotic responses included elevated protein expression levels of cleaved caspase-3 and -7, as well as the upstream caspase-9, annexin V-Cy3 binding, and an increased pro-apoptotic (Bax, Bak, p53)/anti-apoptotic (Bcl-2) mRNA ratio. Additionally, there was JNK phosphorylation, increased protein expression of ERK1/2 and Akt, and a significant increase in intracellular ROS production (97). Another in vitro experiment using the MIN6 cell model found that cadmium treatment induces mitochondrial dysfunction. Following cadmium treatment, there was a significant increase in intracellular ROS and mtROS levels, a marked decrease in ATP production, significant depolarization of the mitochondrial membrane potential, a reduction in mitochondrial DNA copy number, and suppression of the expression of mitochondrial transcription factor A (TFAM) (98). In conclusion, irrespective of the specific EDCs individuals are exposed to and the signaling pathways involved, it is evident that these disruptions can instigate mitochondrial apoptosis, resulting in β-cell dysfunction, and ultimately contributing to the onset of diabetes. Figure 1 encapsulates the primary theme of the review, highlighting the interconnections between endocrine disruptors, diabetes, and mitochondrial dysfunction.
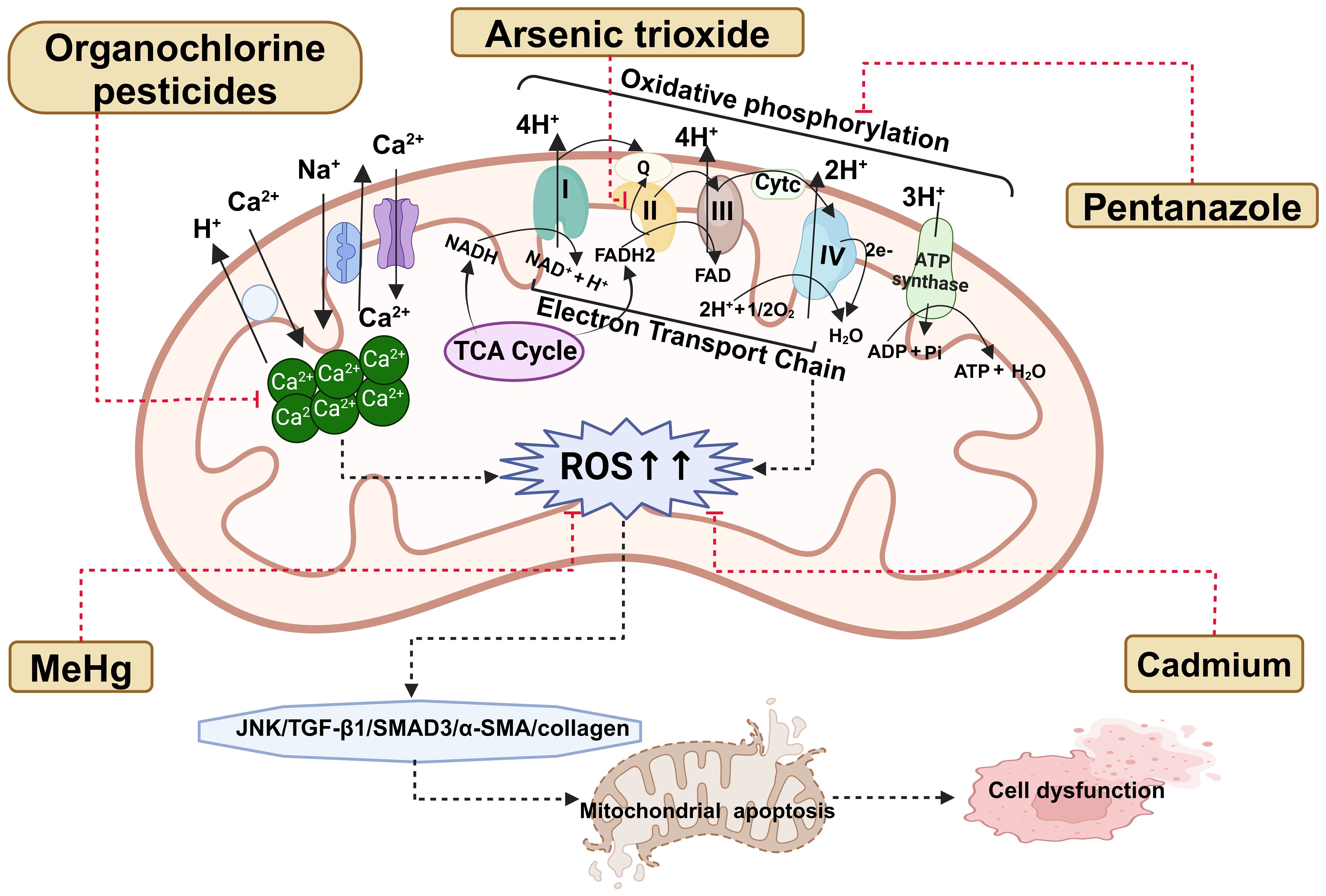
Figure 1. The pathways linking endocrine disruptors to diabetes and its complications through mitochondrial dysfunction are discussed within this review. ROS, reactive oxygen species; TCA, tricarboxylic acid cycle; ADP, adenosine diphosphate; ATP, Adenosine triphosphate; H+, Hydrogen ion; Ca2+, calcium ions; Na+, sodium ion; I, complex I; II, complex II; III, complex III; IV, complex IV; Q, ubiquinone; Cytc, cytochrome c; FAD, flavin adenine dinucleotide; FADH2, flavin adenine dinucleotide; NADH, nicotinamide adenine dinucleotide; NAD+, nicotinamide adenine dinucleotide; O2, oxygen; H2O, Water.
Endocrine disruptors and diabetic complications
Diabetes complications primarily encompass microvascular lesions affecting organs such as the kidneys and retina, as well as macrovascular lesions involving the heart and peripheral blood vessels. Currently, there is limited research directly investigating the impact of endocrine disruptors on diabetic complications. Most studies primarily focus on investigating the direct harmful effects of endocrine disruptors on organs such as the kidneys, retina, and heart. In a prospective cohort study conducted in the United States, 957 non-diabetic adults with elevated fasting and post-load glucose levels were enrolled. Participants were randomized into either an intensive lifestyle intervention focusing on diet, physical activity, and behavioral correction, or a placebo medication group. Plasma concentrations of six PFAS compounds were measured at baseline and after 2 years of follow-up. After a 15-year follow-up period, the occurrence of microvascular diseases was assessed, adjusting for potential risk factors. Results from a multivariable logistic regression model indicated that N-ethyl-perfluorooctane sulfonamido acetic acid (OR 1.17, 95% CI 1.05-1.31) and perfluorodimethylhexane sulfonic acid (OR 1.18, 95% CI 1.04-1.35) may increase the risk of microvascular disease (36). An observational study carried out in America identified a link between elevated levels of dioxins/furans and renal insufficiency (99). Furthermore, a distinct study suggested that prenatal exposure to air pollution could have adverse effects on fetal kidney function, while exposure to green spaces might confer a beneficial impact (100). Moreover, a review concentrating on the influence of chemical agents on the retina underscored the retinal toxicity associated with insecticides such as chlorpyrifos, thiamethoxam (THIA), and lefenuron, along with fungicides like triphenyltin and thiamine. These substances were observed to cause structural alterations in retinal cells and have the potential to result in serious conditions like retinal detachment, fundus hemorrhage, and pupil constriction (101). A recent systematic review unveiled that increased levels of circulating OCPs and polychlorinated biphenyls are associated with a heightened risk of coronary heart disease (102). Additionally, an observational study carried out in Honolulu, America, showcased a positive association between age-adjusted incidence of cardiovascular disease (CVD) and elevated levels of pesticide exposure during the initial 10 years of follow-up (HR = 1.46, 95% CI = 1.10-1.95). This relationship retained statistical significance even after adjusting for other CVD risk factors (HR = 1.42, 95% CI = 1.05-1.92) (103).
Diabetic nephropathy (DN) is recognized as one of the most prevalent microvascular complications of diabetes. BPA, a prevalent endocrine disruptor, has been associated with proteinuria, which may serve as a predictor for the progression of chronic kidney disease (104). Furthermore, an animal study illustrated that chronic exposure to BPA exacerbated renal function impairment in diabetic rats, resulting in hemodynamic disturbances and dysfunction (105). Coronary artery disease is a prevalent complication in diabetes that affects large vessels and stands as the leading cause of mortality among diabetic complications. Two nested case-control studies were independently conducted across European cohorts to investigate the association between BPA exposure and myocardial infarction (MI) occurrence in patients with T2D. Each MI case identified during the study period was matched with a control from the same cohort based on age, sex, and individual cardiovascular history. The analysis focused on baseline urinary BPA levels, which indicated exposure rates of 31% in the ESTHER cohort and 18% in the SURDIAGENE cohort. A meta-analysis combining both studies revealed a significant correlation between detectable urinary BPA levels and MI occurrence, yielding an adjusted OR of 1.97 (95% CI: 1.05-3.70, P = 0.04) (75). The aforementioned studies indicate that prolonged exposure to BPA may contribute to damage in diabetic vasculature. However, diabetes complications carry significant morbidity and mortality rates, and given the diverse array of endocrine disruptors, future research could explore the effects of various types of endocrine disruptors on diabetes complications. Additionally, as most of these studies are observational rather than causal investigations, they cannot entirely dismiss the potential confounding effects of factors such as critical periods of exposure during pregnancy and early childhood, as well as the influence of genetic polymorphisms on the outcomes.
The role of mitochondrial dysfunction in mediating the impact of endocrine disruptors on diabetic complications
An animal experiment suggested that overactivation of renal mitochondrial activity could contribute to metabolic disorders and the development of early DN (106). Moreover, another animal study and cell study showed that cadmium exposure exacerbated kidney injury in diabetic rats by inhibiting autophagy (54). The study revealed that compared to the normal control group, diabetic rats exposed to cadmium exhibited significantly increased levels of malondialdehyde, reduced levels of glutathione (GSH), decreased activity of superoxide dismutase (SOD), decreased activation of catalase (CAT), elevated ROS levels, and an increased number of autophagosomes. Furthermore, the expression of autophagy-related proteins, markers of apoptosis, and fibrosis markers was significantly higher in the exposed group compared to the unexposed group (54). SOD functions by converting superoxide radicals into molecular oxygen and hydrogen peroxide, thus eliminating them. CAT then aids in converting hydrogen peroxide into water and oxygen. GSH plays a role in scavenging ROS (107). When the activity of these enzymes decreases, there will be a notable accumulation of ROS. Subsequent to cadmium chloride exposure, there is a substantial rise in ROS production in renal cells, resulting in oxidative damage to cellular macromolecules, increased lipid peroxidation, protein oxidation, DNA damage, and fragmentation. This cascade activates the transforming growth factor (TGF)-β1/SMAD3/α-SMA/collagen signaling pathway, ultimately leading to renal fibrosis (107). Additionally, another animal study indicated that cadmium chloride exposure triggers renal fibrosis by activating the TGF-β1/mothers against decapentaplegic homolog (Smad)/collagen IV signaling pathway, stimulating proinflammatory mediators, inhibiting the activation of nuclear factor erythroid 2-related factor 2, and enhancing apoptotic signaling (108). An animal study showed that acute exposure to a sublethal dose of THIA led to cell death and structural damage in the eyes of flies (109). Furthermore, in another animal study, female rats were orally administered cypermethrin (CYP) daily from day 7 of gestation until delivery. The sensory retinas of the rats in the CYP subgroup displayed vacuolation in the inner and outer plexiform layers, dilation of hyperemic blood vessels, hyalinization, and disruptions in the photoreceptive layer (110). Mitochondrial dysfunction reduces ATP production and utilization, resulting in heart damage (111, 112). Several studies have documented a strong correlation between mtROS and CVDs (113, 114). An animal study demonstrated that tebuconazole, a commonly used agricultural drug, could accumulate in the heart, leading to a decrease in ATP content in mouse hearts. TFAM plays a crucial role in regulating mitochondrial biogenesis (115), OXPHOS governs the final phases of the eukaryotic mitochondrial respiratory chain (116). The mRNA levels of TFAM and OXPHOS complex subunits were notably reduced in the hearts of mice exposed to the tebuconazole, implying that it impedes mitochondrial biosynthesis and disrupts respiratory chain function, thereby worsening mitochondrial cardiac dysfunction (117). Figure 1 also depicts the interaction between endocrine disruptors, diabetic complications, and mitochondrial dysfunction.
Conclusion
Epidemiological evidence indicates that exposure to EDCs increases the risk of diabetes and its complications. Proposed mechanisms suggest that EDCs may induce mitochondrial dysfunction through several pathways, including disruption of the electron transport chain, perturbation of Ca2+ homeostasis, increased production of ROS, and activation of mitochondrial apoptotic pathways. However, due to the diverse range of EDCs and their cumulative effects in the human body, isolating the independent impact of a single EDC on disease development poses significant challenges. There is a pressing need for enhanced methods to extract and analyze EDCs effectively. Moreover, since most current studies are observational, they are limited in their ability to fully elucidate the precise mechanisms through which EDCs influence disease development. Future research efforts should incorporate more animal and cellular studies to complement these observational findings and provide deeper insights into these mechanisms.
Author contributions
KH: Writing – original draft. RC: Writing – original draft. SX: Writing – original draft. YD: Writing – original draft. ZW: Writing – original draft. MB: Writing – review & editing. BH: Writing – review & editing. SL: Writing – review & editing.
Funding
The author(s) declare financial support was received for the research, authorship, and/or publication of this article. This study was funded by grant 82100040 from National Natural Science Foundation of China, grant 23A0662, 23B0872 from Scientific Research Foundation of Hunan Provincial Education Department, and grant 2022CYY012 from ESI Special Project of Changsha Medical University.
Conflict of interest
The authors declare that the research was conducted in the absence of any commercial or financial relationships that could be construed as a potential conflict of interest.
Publisher’s note
All claims expressed in this article are solely those of the authors and do not necessarily represent those of their affiliated organizations, or those of the publisher, the editors and the reviewers. Any product that may be evaluated in this article, or claim that may be made by its manufacturer, is not guaranteed or endorsed by the publisher.
Abbreviations
Ca2+, calcium ions; ROS, reactive oxygen species; EDCs, endocrine-disrupting chemicals; ATP, Adenosine triphosphate; TCA, tricarboxylic acid cycle; OXPHOS, oxidative phosphorylation; POPs, persistent organic pollutants; T2D, type 2 diabetes; OCPs, organochlorine pesticides; DDE, dichlorodiphenyldichloroethylene; BFRs, brominated flame retardants; PFAS, Per- and polyfluoroalkyl substances; AhRL, AhR ligand bioactivity; MIS, mitochondrial inhibitory substances; HR, hazard ratio; CI, confidence interval; OR, odds ratio; BPA, bisphenol A; MR, Mendelian randomization; FADH2, flavin adenine dinucleotide; NADH, nicotinamide adenine dinucleotide; ATO, arsenic trioxide; PYR, pyruvate; mtROS, mitochondrial ROS; MeHg, methylmercury; TFAM, mitochondrial transcription factor A; THIA, thiamethoxam; CVD, cardiovascular disease; DN, diabetic nephropathy; MI, myocardial infarction; GSH, glutathione; SOD, superoxide dismutase; CAT, catalase; TGF, transforming growth factor; CYP, cypermethrin.
References
1. Magliano DJ, Boyko EJ, I.D.F.D.A.t.e.s. committee. IDF Diabetes Atlas. Brussels: International Diabetes Federation (2021).
2. Yang YY, Shi LX, Li JH, Yao LY, Xiang DX. Piperazine ferulate ameliorates the development of diabetic nephropathy by regulating endothelial nitric oxide synthase. Mol Med Rep. (2019) 19:2245–53. doi: 10.3892/mmr
3. Xu Z, Zhang P, Chen Y, Jiang J, Zhou Z, Zhu H. Comparing SARC-CalF with SARC-F for screening sarcopenia in adults with type 2 diabetes mellitus. Front Nutr. (2022) 9:803924. doi: 10.3389/fnut.2022.803924
4. Luo M, Cao Q, Wang D, Tan R, Shi Y, Chen J, et al. The impact of diabetes on postoperative outcomes following spine surgery: A meta-analysis of 40 cohort studies with 2.9 million participants. Int J Surg. (2022) 104:106789. doi: 10.1016/j.ijsu.2022.106789
5. Yu T, Xu B, Bao M, Gao Y, Zhang Q, Zhang X, et al. Identification of potential biomarkers and pathways associated with carotid atherosclerotic plaques in type 2 diabetes mellitus: A transcriptomics study. Front Endocrinol (Lausanne). (2022) 13:981100. doi: 10.3389/fendo.2022.981100
6. Su M, Hu R, Tang T, Tang W, Huang C. Review of the correlation between Chinese medicine and intestinal microbiota on the efficacy of diabetes mellitus. Front Endocrinol (Lausanne). (2022) 13:1085092. doi: 10.3389/fendo.2022.1085092
7. Chen J, Li X, Liu H, Zhong D, Yin K, Li Y, et al. Bone marrow stromal cell-derived exosomal circular RNA improves diabetic foot ulcer wound healing by activating the nuclear factor erythroid 2-related factor 2 pathway and inhibiting ferroptosis. Diabetes Med. (2023) 40:e15031. doi: 10.1111/dme.15031
8. Chen R, Xu S, Ding Y, Li L, Huang C, Bao M, et al. Dissecting causal associations of type 2 diabetes with 111 types of ocular conditions: a Mendelian randomization study. Front Endocrinol (Lausanne). (2023) 14:1307468. doi: 10.3389/fendo.2023.1307468
9. Chen Y, Tan S, Liu M, Li J. LncRNA TINCR is downregulated in diabetic cardiomyopathy and relates to cardiomyocyte apoptosis. Scand Cardiovasc J. (2018) 52:335–9. doi: 10.1080/14017431.2018.1546896
10. Mi W, Xia Y, Bian Y. Meta-analysis of the association between aldose reductase gene (CA)n microsatellite variants and risk of diabetic retinopathy. Exp Ther Med. (2019) 18:4499–509. doi: 10.3892/etm
11. Liang D, Cai X, Guan Q, Ou Y, Zheng X, Lin X. Burden of type 1 and type 2 diabetes and high fasting plasma glucose in Europe, 1990-2019: a comprehensive analysis from the global burden of disease study 2019. Front Endocrinol (Lausanne). (2023) 14:1307432. doi: 10.3389/fendo.2023.1307432
12. Liang X, Zhang J, Wang Y, Wu Y, Liu H, Feng W, et al. Comparative study of microvascular structural changes in the gestational diabetic placenta. Diabetes Vasc Dis Res. (2023) 20:14791641231173627. doi: 10.1177/14791641231173627
13. Zhou Y, Chai X, Yang G, Sun X, Xing Z. Changes in body mass index and waist circumference and heart failure in type 2 diabetes mellitus. Front Endocrinol (Lausanne). (2023) 14:1305839. doi: 10.3389/fendo.2023.1305839
14. Schmittdiel J, Vijan S, Fireman B, Lafata JE, Oestreicher N, Selby JV. Predicted quality-adjusted life years as a composite measure of the clinical value of diabetes risk factor control. Med Care. (2007) 45:315–21. doi: 10.1097/01.mlr.0000254582.85666.01
15. Leong A, Rahme E, Dasgupta K. Spousal diabetes as a diabetes risk factor: a systematic review and meta-analysis. BMC Med. (2014) 12:12. doi: 10.1186/1741-7015-12-12
16. Li JM, Li X, Chan LWC, Hu R, Zheng T, Li H, et al. Lipotoxicity-polarised macrophage-derived exosomes regulate mitochondrial fitness through Miro1-mediated mitophagy inhibition and contribute to type 2 diabetes development in mice. Diabetologia. (2023) 66:2368–86. doi: 10.1007/s00125-023-05992-7
17. Aloke C, Egwu CO, Aja PM, Obasi NA, Chukwu J, Akumadu BO, et al. Current advances in the management of diabetes mellitus. Biomedicines. (2022) 10:2436. doi: 10.3390/biomedicines10102436
18. Blaslov K, Naranđa FS, Kruljac I, Renar IP. Treatment approach to type 2 diabetes: Past, present and future. World J Diabetes. (2018) 9:209–19. doi: 10.4239/wjd.v9.i12.209
19. Xiao D, Guo Y, Li X, Yin JY, Zheng W, Qiu XW, et al. The Impacts of SLC22A1 rs594709 and SLC47A1 rs2289669 Polymorphisms on Metformin Therapeutic Efficacy in Chinese Type 2 Diabetes Patients. Int J Endocrinol. (2016) 2016:4350712. doi: 10.1155/2016/4350712
20. Zuberi Z, Sauli E, Cun L, Deng J, Li WJ, He XL, et al. Insulin-delivery methods for children and adolescents with type 1 diabetes. Ther Adv Endocrinol Metab. (2020) 11:2042018820906016. doi: 10.1177/2042018820906016
21. Yilmaz B, Terekeci H, Sandal S, Kelestimur F. Endocrine disrupting chemicals: exposure, effects on human health, mechanism of action, models for testing and strategies for prevention. Rev Endocr Metab Disord. (2020) 21:127–47. doi: 10.1007/s11154-019-09521-z
22. Żwierełło W, Maruszewska A, Skórka-Majewicz M, Goschorska M, Baranowska-Bosiacka I, Dec K, et al. The influence of polyphenols on metabolic disorders caused by compounds released from plastics - Review. Chemosphere. (2020) 240:124901. doi: 10.1016/j.chemosphere.2019.124901
23. Kunysz M, Mora-Janiszewska O, Darmochwał-Kolarz D. Epigenetic modifications associated with exposure to endocrine disrupting chemicals in patients with gestational diabetes mellitus. Int J Mol Sci. (2021) 22:4693. doi: 10.3390/ijms22094693
24. Chen M, Lv C, Zhang S, Tse LA, Hong X, Liu X, et al. Bisphenol A substitutes and childhood obesity at 7 years: a cross-sectional study in Shandong, China. Environ Sci Pollut Res Int. (2023) 30:73174–84. doi: 10.1007/s11356-023-27578-x
25. Gontarz-Nowak K, Szklarz M, Szychlińska M, Matuszewski W, Bandurska-Stankiewicz E. A brief look at hashimoto's disease, adrenal incidentalomas, obesity and insulin resistance-could endocrine disruptors be the other side of the same coin? Medicina (Kaunas). (2023) 59:1234. doi: 10.3390/medicina59071234
26. Khalil WJ, Akeblersane M, Khan AS, Moin ASM, Butler AE. Environmental pollution and the risk of developing metabolic disorders: obesity and diabetes. Int J Mol Sci. (2023) 24:8870. doi: 10.3390/ijms24108870
27. Varghese SV, Hall JM. Bisphenol A substitutes and obesity: a review of the epidemiology and pathophysiology. Front Endocrinol (Lausanne). (2023) 14:1155694. doi: 10.3389/fendo.2023.1155694
28. Wu Q, Li G, Zhao CY, Na XL, Zhang YB. Association between phthalate exposure and obesity risk: A meta-analysis of observational studies. Environ Toxicol Pharmacol. (2023) 102:104240. doi: 10.1016/j.etap.2023.104240
29. Dalamaga M, Kounatidis D, Tsilingiris D, Vallianou NG, Karampela I, Psallida S, et al. The role of endocrine disruptors bisphenols and phthalates in obesity: current evidence, perspectives and controversies. Int J Mol Sci. (2024) 25:675. doi: 10.3390/ijms25010675
30. Mondal S, Basu S, Ghosh S, Guria S, Mukherjee S. Diethyl phthalate, a plasticizer, induces adipocyte inflammation and apoptosis in mice after long-term dietary administration. J Biochem Mol Toxicol. (2024) 38:e23561. doi: 10.1002/jbt.23561
31. Zhang S, Dai L, Wan Z, Huang Z, Zou M, Guan H. Sex-specific associations of bisphenol A and its substitutes with body fat distribution among US adults: NHANES 2011-2016. Environ Sci Pollut Res Int. (2024) 31:7948–58. doi: 10.1007/s11356-023-31589-z
32. Zhang G, Meng L, Guo J, Guan X, Liu M, Han X, et al. Exposure to novel brominated and organophosphate flame retardants and associations with type 2 diabetes in East China: A case-control study. Sci Total Environ. (2023) 871:162107. doi: 10.1016/j.scitotenv.2023.162107
33. Xie X, Lu C, Wu M, Liang J, Ying Y, Liu K, et al. Association between triclocarban and triclosan exposures and the risks of type 2 diabetes mellitus and impaired glucose tolerance in the National Health and Nutrition Examination Survey (NHANES 2013-2014). Environ Int. (2020) 136:105445. doi: 10.1016/j.envint.2019.105445
34. Weiss MC, Shih YH, Bryan MS, Jackson BP, Aguilar D, Hanis CL, et al. Relationships between urinary metals and diabetes traits among mexican americans in starr county, texas, USA. Biol Trace Elem Res. (2023) 201:529–38. doi: 10.1007/s12011-022-03165-y
35. Salamanca-Fernández E, Iribarne-Durán LM, Rodríguez-Barranco M, Vela-Soria F, Olea N, Sánchez-Pérez MJ, et al. Historical exposure to non-persistent environmental pollutants and risk of type 2 diabetes in a Spanish sub-cohort from the European Prospective Investigation into Cancer and Nutrition study. Environ Res. (2020) 185:109383. doi: 10.1016/j.envres.2020.109383
36. Cardenas A, Hivert MF, Gold DR, Hauser R, Kleinman KP, Lin PD, et al. Associations of perfluoroalkyl and polyfluoroalkyl substances with incident diabetes and microvascular disease. Diabetes Care. (2019) 42:1824–32. doi: 10.2337/dc18-2254
37. Sargis RM, Simmons RA. Environmental neglect: endocrine disruptors as underappreciated but potentially modifiable diabetes risk factors. Diabetologia. (2019) 62:1811–22. doi: 10.1007/s00125-019-4940-z
38. Shi P, Yan H, Fan X, Xi S. A benchmark dose analysis for urinary cadmium and type 2 diabetes mellitus. Environ Pollut. (2021) 273:116519. doi: 10.1016/j.envpol.2021.116519
39. Vanni R, Bussuan RM, Rombaldi RL, Arbex AK. Endocrine disruptors and the induction of insulin resistance. Curr Diabetes Rev. (2021) 17:e102220187107. doi: 10.2174/1573399816666201022121254
40. Yan D, Jiao Y, Yan H, Liu T, Yan H, Yuan J. Endocrine-disrupting chemicals and the risk of gestational diabetes mellitus: a systematic review and meta-analysis. Environ Health. (2022) 21:53. doi: 10.1186/s12940-022-00858-8
41. Dagar M, Kumari P, Mirza AMW, Singh S, Ain NU, Munir Z, et al. The hidden threat: endocrine disruptors and their impact on insulin resistance. Cureus. (2023) 15:e47282. doi: 10.7759/cureus.47282
42. Ding E, Deng F, Fang J, Li T, Hou M, Liu J, et al. Association between organophosphate ester exposure and insulin resistance with glycometabolic disorders among older chinese adults 60-69 years of age: evidence from the China BAPE study. Environ Health Perspect. (2023) 131:47009. doi: 10.1289/EHP11896
43. Jiang W, Ding K, Huang W, Xu F, Lei M, Yue R. Potential effects of bisphenol A on diabetes mellitus and its chronic complications: A narrative review. Heliyon. (2023) 9:e16340. doi: 10.1016/j.heliyon.2023.e16340
44. Ihenacho U, Guillermo C, Wilkens LR, Franke AA, Tseng C, Li Y, et al. Association of endocrine disrupting chemicals with the metabolic syndrome among women in the multiethnic cohort study. J Endocr Soc. (2023) 7:bvad136. doi: 10.1210/jendso/bvad136
45. Durward-Akhurst SA, Schultz NE, Norton EM, Rendahl AK, Besselink H, Behnisch PA, et al. Associations between endocrine disrupting chemicals and equine metabolic syndrome phenotypes. Chemosphere. (2019) 218:652–61. doi: 10.1016/j.chemosphere.2018.11.136
46. Milošević N, Milanović M, Sudji J, Bosić Živanović D, Stojanoski S, Vuković B, et al. Could phthalates exposure contribute to the development of metabolic syndrome and liver disease in humans? Environ Sci Pollut Res Int. (2020) 27:772–84. doi: 10.1007/s11356-019-06831-2
47. Zamora AN, Jansen EC, Goodrich JM, Téllez-Rojo MM, Song PXK, Meeker JD, et al. Cross-sectional associations between phthalates, phenols, and parabens with metabolic syndrome risk during early-to-mid adolescence among a cohort of Mexican youth. Environ Res. (2023) 236:116706. doi: 10.1016/j.envres.2023.116706
48. Reina-Pérez I, Artacho-Cordón F, Mustieles V, Castellano-Castillo D, Cardona F, Jiménez-Díaz I, et al. Cross-sectional associations of persistent organic pollutants measured in adipose tissue and metabolic syndrome in clinically diagnosed middle-aged adults. Environ Res. (2023) 222:115350. doi: 10.1016/j.envres.2023.115350
49. Zamora AN, Jansen EC, Tamayo-Ortiz M, Goodrich JM, Sánchez BN, Watkins DJ, et al. Exposure to phenols, phthalates, and parabens and development of metabolic syndrome among mexican women in midlife. Front Public Health. (2021) 9:620769. doi: 10.3389/fpubh.2021.620769
50. Haverinen E, Fernandez MF, Mustieles V, Tolonen H. Metabolic syndrome and endocrine disrupting chemicals: an overview of exposure and health effects. Int J Environ Res Public Health. (2021) 18:13047. doi: 10.3390/ijerph182413047
51. Mérida DM, Moreno-Franco B, Marquès M, León-Latre M, Laclaustra M, Guallar-Castillón P. Phthalate exposure and the metabolic syndrome: A systematic review and meta-analysis. Environ Pollut. (2023) 333:121957. doi: 10.1016/j.envpol.2023.121957
52. Lin J-Y, Yin R-X. Exposure to endocrine-disrupting chemicals and type 2 diabetes mellitus in later life. Exposure Health. (2023) 15:199–229. doi: 10.1007/s12403-022-00486-0
53. Marroqui L, Tudurí E, Alonso-Magdalena P, Quesada I, Nadal Á, Dos Santos RS. Mitochondria as target of endocrine-disrupting chemicals: implications for type 2 diabetes. J Endocrinol. (2018) 239:R27–45. doi: 10.1530/JOE-18-0362
54. Ma Y, Yue C, Sun Q, Wang Y, Gong Z, Zhang K, et al. Cadmium exposure exacerbates kidney damage by inhibiting autophagy in diabetic rats. Ecotoxicol Environ Saf. (2023) 267:115674. doi: 10.1016/j.ecoenv.2023.115674
55. Li Y, Ou S, Liu Q, Gan L, Zhang L, Wang Y, et al. Genistein improves mitochondrial function and inflammatory in rats with diabetic nephropathy via inhibiting MAPK/NF-κB pathway. Acta Cir Bras. (2022) 37:e370601. doi: 10.1590/acb370601
56. Chow J, Rahman J, Achermann JC, Dattani MT, Rahman S. Mitochondrial disease and endocrine dysfunction. Nat Rev Endocrinol. (2017) 13:92–104. doi: 10.1038/nrendo.2016.151
57. Brand MD. Mitochondrial generation of superoxide and hydrogen peroxide as the source of mitochondrial redox signaling. Free Radic Biol Med. (2016) 100:14–31. doi: 10.1016/j.freeradbiomed.2016.04.001
58. Reddam A, McLarnan S, Kupsco A. Environmental chemical exposures and mitochondrial dysfunction: a review of recent literature. Curr Environ Health Rep. (2022) 9:631–49. doi: 10.1007/s40572-022-00371-7
59. Kuretu A, Arineitwe C, Mothibe M, Ngubane P, Khathi A, Sibiya N. Drug-induced mitochondrial toxicity: Risks of developing glucose handling impairments. Front Endocrinol (Lausanne). (2023) 14:1123928. doi: 10.3389/fendo.2023.1123928
60. Guo Z, Li Z, Zhang M, Bao M, He B, Zhou X. LncRNA FAS-AS1 upregulated by its genetic variation rs6586163 promotes cell apoptosis in nasopharyngeal carcinoma through regulating mitochondria function and Fas splicing. Sci Rep. (2023) 13:8218. doi: 10.1038/s41598-023-35502-z
61. Choi JR, Park S, Kim S-K, Kim J-Y, Lee K, Oh S-S, et al. Mitochondrial DNA content and deletion ratio are associated with metabolic syndrome in a general population exposed to pesticide. Mol Cell Toxicol. (2020) 16:347–54. doi: 10.1007/s13273-020-00079-5
62. Soundararajan A, Prabu P, Mohan V, Gibert Y, Balasubramanyam M. Novel insights of elevated systemic levels of bisphenol-A (BPA) linked to poor glycemic control, accelerated cellular senescence and insulin resistance in patients with type 2 diabetes. Mol Cell Biochem. (2019) 458:171–83. doi: 10.1007/s11010-019-03540-9
63. Rancière F, Botton J, Slama R, Lacroix MZ, Debrauwer L, Charles MA, et al. Exposure to bisphenol A and bisphenol S and incident type 2 diabetes: A case-cohort study in the french cohort D.E.S.I.R. Environ Health Perspect. (2019) 127:107013. doi: 10.1289/EHP5159
64. Murphy L, Mérida-Ortega Á, Cebrián ME, Hernández-Garciadiego L, Gómez-Ruiz H, Gamboa-Loira B, et al. Exposure to bisphenol A and diabetes risk in Mexican women. Environ Sci Pollut Res. (2019) 26:26332–8. doi: 10.1007/s11356-019-05731-9
65. Haq MEU, Akash MSH, Sabir S, Mahmood MH, Rehman K. Human exposure to bisphenol A through dietary sources and development of diabetes mellitus: a cross-sectional study in Pakistani population. Environ Sci Pollut Res. (2020) 27:26262–75. doi: 10.1007/s11356-020-09044-0
66. Mansouri EH, Reggabi M. Association between type 2 diabetes and exposure to chlorinated persistent organic pollutants in Algeria: A case-control study. Chemosphere. (2021) 264:128596. doi: 10.1016/j.chemosphere.2020.128596
67. Park S, Kim S-K, Kim J-Y, Lee K, Choi JR, Chang S-J, et al. Exposure to pesticides and the prevalence of diabetes in a rural population in Korea. NeuroToxicology. (2019) 70:12–8. doi: 10.1016/j.neuro.2018.10.007
68. Hansen MRH, Gyawali B, Neupane D, Jørs E, Sandbæk A, Kallestrup P, et al. Pesticide exposure and diabetes mellitus in a semi-urban Nepali population: a cross-sectional study. Int Arch Occup Environ Health. (2020) 93:513–24. doi: 10.1007/s00420-019-01508-2
69. Jaacks LM, Yadav S, Panuwet P, Kumar S, Rajacharya GH, Johnson C, et al. Metabolite of the pesticide DDT and incident type 2 diabetes in urban India. Environ Int. (2019) 133:105089. doi: 10.1016/j.envint.2019.105089
70. Duncan BB, Castilhos CD, Bracco PA, Schmidt MI, Kang S, Im S, et al. Aryl-hydrocarbon receptor binding and the incidence of type 2 diabetes: the Brazilian Longitudinal Study of Adult Health (ELSA-Brasil). Environ Health. (2020) 19:105. doi: 10.1186/s12940-020-00658-y
71. Ongono JS, Dow C, Gambaretti J, Severi G, Boutron-Ruault M-C, Bonnet F, et al. Dietary exposure to brominated flame retardants and risk of type 2 diabetes in the French E3N cohort. Environ Int. (2019) 123:54–60. doi: 10.1016/j.envint.2018.11.040
72. Donat-Vargas C, Bergdahl IA, Tornevi A, Wennberg M, Sommar J, Kiviranta H, et al. Perfluoroalkyl substances and risk of type II diabetes: A prospective nested case-control study. Environ Int. (2019) 123:390–8. doi: 10.1016/j.envint.2018.12.026
73. Lee I, Park YJ, Kim MJ, Kim S, Choi S, Park J, et al. Associations of urinary concentrations of phthalate metabolites, bisphenol A, and parabens with obesity and diabetes mellitus in a Korean adult population: Korean National Environmental Health Survey (KoNEHS) 2015–2017. Environ Int. (2021) 146:106227. doi: 10.1016/j.envint.2020.106227
74. Li Y, Xu S, Li Y, Zhang B, Huo W, Zhu Y, et al. Association between urinary parabens and gestational diabetes mellitus across prepregnancy body mass index categories. Environ Res. (2019) 170:151–9. doi: 10.1016/j.envres.2018.12.028
75. Hu C, Schöttker B, Venisse N, Limousi F, Saulnier PJ, Albouy-Llaty M, et al. Bisphenol A, chlorinated derivatives of bisphenol A and occurrence of myocardial infarction in patients with type 2 diabetes: nested case-control studies in two european cohorts. Environ Sci Technol. (2019) 53:9876–83. doi: 10.1021/acs.est.9b02963
76. Hinault C, Caroli-Bosc P, Bost F, Chevalier N. Critical overview on endocrine disruptors in diabetes mellitus. Int J Mol Sci. (2023) 24:4537. doi: 10.3390/ijms24054537
77. Mendes V, Ribeiro C, Delgado I, Peleteiro B, Aggerbeck M, Distel E, et al. The association between environmental exposures to chlordanes, adiposity and diabetes-related features: a systematic review and meta-analysis. Sci Rep. (2021) 11:14546. doi: 10.1038/s41598-021-93868-4
78. Czajka M, Matysiak-Kucharek M, Jodłowska-Jędrych B, Sawicki K, Fal B, Drop B, et al. Organophosphorus pesticides can influence the development of obesity and type 2 diabetes with concomitant metabolic changes. Environ Res. (2019) 178:108685. doi: 10.1016/j.envres.2019.108685
79. vom Saal FS, Hughes C. An extensive new literature concerning low-dose effects of bisphenol A shows the need for a new risk assessment. Environ Health Perspect. (2005) 113:926–33. doi: 10.1289/ehp.7713
80. Fu Q, Chen R, Ding Y, Xu S, Huang C, He B, et al. Sodium intake and the risk of various types of cardiovascular diseases: a Mendelian randomization study. Front Nutr. (2023) 10:1250509. doi: 10.3389/fnut.2023.1250509
81. Fu Q, Chen R, Xu S, Ding Y, Huang C, He B, et al. Assessment of potential risk factors associated with gestational diabetes mellitus: evidence from a Mendelian randomization study. Front Endocrinol (Lausanne). (2023) 14:1276836. doi: 10.3389/fendo.2023.1276836
82. Jiang Y, Chen R, Xu S, Ding Y, Zhang M, Bao M, et al. Endocrine and metabolic factors and the risk of idiopathic pulmonary fibrosis: a Mendelian randomization study. Front Endocrinol (Lausanne). (2023) 14:1321576. doi: 10.3389/fendo.2023.1321576
83. Jiang Y, Chen R, Xu S, Ding Y, Zhang M, Bao M, et al. Assessing causal associations of hyperparathyroidism with blood counts and biochemical indicators: a Mendelian randomization study. Front Endocrinol (Lausanne). (2023) 14:1295040. doi: 10.3389/fendo.2023.1295040
84. Duh-Leong C, Maffini MV, Kassotis CD, Vandenberg LN, Trasande L. The regulation of endocrine-disrupting chemicals to minimize their impact on health. Nat Rev Endocrinol. (2023) 19:600–14. doi: 10.1038/s41574-023-00872-x
85. Kabir ER, Rahman MS, Rahman I. A review on endocrine disruptors and their possible impacts on human health. Environ Toxicol Pharmacol. (2015) 40:241–58. doi: 10.1016/j.etap.2015.06.009
86. Will Y, Shields JE, Wallace KB. Drug-induced mitochondrial toxicity in the geriatric population: challenges and future directions. Biol (Basel). (2019) 8:32. doi: 10.3390/biology8020032
87. Sergi D, Naumovski N, Heilbronn LK, Abeywardena M, O'Callaghan N, Lionetti L, et al. Mitochondrial (Dys)function and insulin resistance: from pathophysiological molecular mechanisms to the impact of diet. Front Physiol. (2019) 10:532. doi: 10.3389/fphys.2019.00532
88. Kim CW, Choi KC. Effects of anticancer drugs on the cardiac mitochondrial toxicity and their underlying mechanisms for novel cardiac protective strategies. Life Sci. (2021) 277:119607. doi: 10.1016/j.lfs.2021.119607
89. Lee H, Gao Y, Ko E, Lee J, Lee H-K, Lee S, et al. Nonmonotonic response of type 2 diabetes by low concentration organochlorine pesticide mixture: Findings from multi-omics in zebrafish. J Hazardous Materials. (2021) 416:125956. doi: 10.1016/j.jhazmat.2021.125956
90. Zia A, Farkhondeh T, Pourbagher-Shahri AM, Samarghandian S. The roles of mitochondrial dysfunction and reactive oxygen species in aging and senescence. Curr Mol Med. (2022) 22:37–49. doi: 10.2174/1566524021666210218112616
91. Zhao RZ, Jiang S, Zhang L, Yu ZB. Mitochondrial electron transport chain, ROS generation and uncoupling (Review). Int J Mol Med. (2019) 44:3–15. doi: 10.3892/ijmm
92. Bauer TM, Murphy E. Role of mitochondrial calcium and the permeability transition pore in regulating cell death. Circ Res. (2020) 126:280–93. doi: 10.1161/CIRCRESAHA.119.316306
93. Ko E, Choi M, Shin S. Bottom-line mechanism of organochlorine pesticides on mitochondria dysfunction linked with type 2 diabetes. J Hazard Mater. (2020) 393:122400. doi: 10.1016/j.jhazmat.2020.122400
94. Giorgi C, Marchi S, Pinton P. The machineries, regulation and cellular functions of mitochondrial calcium. Nat Rev Mol Cell Biol. (2018) 19:713–30. doi: 10.1038/s41580-018-0052-8
95. de Ridder I, Kerkhofs M, Lemos FO, Loncke J, Bultynck G, Parys JB. The ER-mitochondria interface, where Ca2+ and cell death meet. Cell Calcium. (2023) 112:102743. doi: 10.1016/j.ceca.2023.102743
96. Kalo MB, Rezaei M. In vitro toxic interaction of arsenic and hyperglycemia in mitochondria: an important implication of increased vulnerability in pre-diabetics. Environ Sci Pollut Res Int. (2022) 29:28375–85. doi: 10.1007/s11356-022-18513-7
97. Yang CY, Liu SH, Su CC, Fang KM, Yang TY, Liu JM, et al. Methylmercury induces mitochondria- and endoplasmic reticulum stress-dependent pancreatic β-cell apoptosis via an oxidative stress-mediated JNK signaling pathway. Int J Mol Sci. (2022) 23:2858. doi: 10.3390/ijms23052858
98. Hong H, He H, Lin X, Hayuehashi T, Xu J, Zhang J, et al. Cadmium exposure suppresses insulin secretion through mtROS-mediated mitochondrial dysfunction and inflammatory response in pancreatic beta cells. J Trace Elements Med Biol. (2022) 71:126952. doi: 10.1016/j.jtemb.2022.126952
99. Jain RB. Trends in concentrations of selected dioxins and furans across various stages of kidney function for US adults. Environ Sci pollut Res. (2021) 28:43763–76. doi: 10.1007/s11356-021-13844-3
100. Rahmani Sani A, Abroudi M, Heydari H, Adli A, Miri M, Mehrabadi S, et al. Maternal exposure to ambient particulate matter and green spaces and fetal renal function. Environ Res. (2020) 184:109285. doi: 10.1016/j.envres.2020.109285
101. Souza Monteiro de Araújo D, Brito R, Pereira-Figueiredo D, Dos Santos-Rodrigues A, De Logu F, Nassini R, et al. Retinal toxicity induced by chemical agents. Int J Mol Sci. (2022) 23:8182. doi: 10.3390/ijms23158182
102. Mohammadkhani MA, Shahrzad S, Haghighi M, Ghanbari R, Mohamadkhani A. Insights into organochlorine pesticides exposure in the development of cardiovascular diseases: A systematic review. Arch Iran Med. (2023) 26:592–9. doi: 10.34172/aim.2023.86
103. Berg ZK, Rodriguez B, Davis J, Katz AR, Cooney RV, Masaki K. Association between occupational exposure to pesticides and cardiovascular disease incidence: the kuakini honolulu heart program. J Am Heart Assoc. (2019) 8:e012569. doi: 10.1161/JAHA.119.012569
104. Tong S, Yang S, Li T, Gao R, Hu J, Luo T, et al. Role of neutrophil extracellular traps in chronic kidney injury induced by bisphenol-A. J Endocrinol. (2019) 241:125–34. doi: 10.1530/JOE-18-0608
105. Wu B, Zhao Q, Li Z, Min Z, Shi M, Nie X, et al. Environmental level bisphenol A accelerates alterations of the reno-cardiac axis by the MAPK cascades in male diabetic rats: An analysis based on transcriptomic profiling and bioinformatics. Environ Pollut. (2021) 287:117671. doi: 10.1016/j.envpol.2021.117671
106. Wu M, Li S, Yu X, Chen W, Ma H, Shao C, et al. Mitochondrial activity contributes to impaired renal metabolic homeostasis and renal pathology in STZ-induced diabetic mice. Am J Physiol Renal Physiol. (2019) 317:F593–f605. doi: 10.1152/ajprenal.00076.2019
107. Joardar S, Dewanjee S, Bhowmick S, Dua TK, Das S, Saha A, et al. Rosmarinic acid attenuates cadmium-induced nephrotoxicity via inhibition of oxidative stress, apoptosis, inflammation and fibrosis. Int J Mol Sci. (2019) 20:2027. doi: 10.3390/ijms20082027
108. Das S, Dewanjee S, Dua TK, Joardar S, Chakraborty P, Bhowmick S, et al. Carnosic acid attenuates cadmium induced nephrotoxicity by inhibiting oxidative stress, promoting Nrf2/HO-1 signalling and impairing TGF-β1/smad/collagen IV signalling. Molecules. (2019) 24:4176. doi: 10.3390/molecules24224176
109. Li X, Liu J, Wang X. Exploring the multilevel hazards of thiamethoxam using Drosophila melanogaster. J Hazard Mater. (2020) 384:121419. doi: 10.1016/j.jhazmat.2019.121419
110. Mohey Issa N, Al-Gholam MA. The effect of N-acetylcysteine on the sensory retina of male albino rats exposed prenatally to cypermethrin. Folia Morphol (Warsz). (2021) 80:140–8. doi: 10.5603/FM.a2020.0043
111. Jin X, Xue B, Ahmed RZ, Ding G, Li Z. Fine particles cause the abnormality of cardiac ATP levels via PPARα-mediated utilization of fatty acid and glucose using in vivo and in vitro models. Environ Pollut. (2019) 249:286–94. doi: 10.1016/j.envpol.2019.02.083
112. Shukla P, Mukherjee S. Mitochondrial dysfunction: An emerging link in the pathophysiology of polycystic ovary syndrome. Mitochondrion. (2020) 52:24–39. doi: 10.1016/j.mito.2020.02.006
113. Peoples JN, Saraf A, Ghazal N, Pham TT, Kwong JQ. Mitochondrial dysfunction and oxidative stress in heart disease. Exp Mol Med. (2019) 51:1–13. doi: 10.1038/s12276-019-0355-7
114. Hu H, Lin Y, Xu X, Lin S, Chen X, Wang S. The alterations of mitochondrial DNA in coronary heart disease. Exp Mol Pathol. (2020) 114:104412. doi: 10.1016/j.yexmp.2020.104412
115. Jiang X, Wang J. Down-regulation of TFAM increases the sensitivity of tumour cells to radiation via p53/TIGAR signalling pathway. J Cell Mol Med. (2019) 23:4545–58. doi: 10.1111/jcmm.14350
116. Shi Z, Li XB, Peng ZC, Fu SP, Zhao CX, Du XL, et al. Berberine protects against NEFA-induced impairment of mitochondrial respiratory chain function and insulin signaling in bovine hepatocytes. Int J Mol Sci. (2018) 19:1691. doi: 10.3390/ijms19061691
Keywords: endocrine disruptor, type 2 diabetes mellitus, diabetic complications, mitochondrial dysfunction, reactive oxygen species
Citation: He K, Chen R, Xu S, Ding Y, Wu Z, Bao M, He B and Li S (2024) Environmental endocrine disruptor-induced mitochondrial dysfunction: a potential mechanism underlying diabetes and its complications. Front. Endocrinol. 15:1422752. doi: 10.3389/fendo.2024.1422752
Received: 24 April 2024; Accepted: 08 July 2024;
Published: 15 August 2024.
Edited by:
Rui Zhang, University of Jinan, ChinaReviewed by:
Luís Pedro Rato, Instituto Politécnico da Guarda, PortugalZheng Xiang, Liaoning University, China
Copyright © 2024 He, Chen, Xu, Ding, Wu, Bao, He and Li. This is an open-access article distributed under the terms of the Creative Commons Attribution License (CC BY). The use, distribution or reproduction in other forums is permitted, provided the original author(s) and the copyright owner(s) are credited and that the original publication in this journal is cited, in accordance with accepted academic practice. No use, distribution or reproduction is permitted which does not comply with these terms.
*Correspondence: Meihua Bao, bWhiYW83OEAxNjMuY29t; Binsheng He, aGJzY3NtdUAxNjMuY29t; Sen Li, c2VubGlAY29ubmVjdC5oa3UuaGs=
†These authors have contributed equally to this work and share first authorship