- 1Department of Ophthalmology, Beijing Hospital, National Center of Gerontology, Institute of Geriatric Medicine, Chinese Academy of Medical Sciences, Beijing, China
- 2Graduate School of Peking Union Medical College, Beijing, China
Insulin resistance (IR) is becoming a worldwide medical and public health challenge as an increasing prevalence of obesity and metabolic disorders. Accumulated evidence has demonstrated a strong relationship between IR and a higher incidence of several dramatically vision-threatening retinal diseases, including diabetic retinopathy, age-related macular degeneration, and glaucoma. In this review, we provide a schematic overview of the associations between IR and certain ocular diseases and further explore the possible mechanisms. Although the exact causes explaining these associations have not been fully elucidated, underlying mechanisms of oxidative stress, chronic low-grade inflammation, endothelial dysfunction and vasoconstriction, and neurodegenerative impairments may be involved. Given that IR is a modifiable risk factor, it may be important to identify patients at a high IR level with prompt treatment, which may decrease the risk of developing certain ocular diseases. Additionally, improving IR through the activation of insulin signaling pathways could become a potential therapeutic target.
1 Introduction
An acceleration of the prevalence of insulin resistance (IR) and metabolic syndrome (MetS) is becoming a worldwide public health challenge with an increasing rate of obesity (1). Evidence has indicated that IR has a strong association with the development of the cardiovascular diseases (2, 3). Insulin resistance is a pathological condition in which the ability of insulin to influence glucose uptake via insulin-dependent transportation is impaired, thus a higher-than-normal concentration is required to maintain a normal glucose level (4). That is, insulin-dependent cells fail to respond to a normal circulatory level of insulin due to decreased insulin sensitivity. IR is the hallmark of type 2 diabetes mellitus (T2DM) and MetS, representing a common feature of a cluster of metabolic abnormalities in T2DM and MetS, such as obesity, hyperinsulinemia, dyslipidemia, and high blood pressure (5).
In detail, increased IR, conversely correlated with insulin sensitivity, could be attributed to reduced capacity of insulin receptors to bind with insulin. At the molecular level, the explanations for decreased affinity of insulin receptors may include a declining number of insulin receptors, a mutation in insulin receptors, and autoimmune antibodies against insulin receptors (6). The interaction of insulin receptors with their ligands has been considered a key determinant for functional signal transduction, any interference in this process would lead to IR (6). Previous studies mainly investigated the effect of IR on traditionally thought insulin-sensitive tissues, such as liver, skeletal muscle, and adipose tissue, but accumulating evidence has suggested that IR plays a crucial role in the central nervous system (CNS) and retina (7, 8), since insulin signal pathway is a key determinant for cell survival, especially for neural cells (9). Although inconsistent results exist regarding the connection between IR and ocular diseases, increasing studies have postulated that IR is associated with glaucoma and several retinal diseases, particularly diabetic retinopathy (DR) and age-related macular degeneration (AMD), which account for the majority of vision loss in middle-aged and elderly populations (10, 11). To the best of our knowledge, currently established treatment options for retinal diseases are mainly limited to advanced stages, which only partially suffice to slow disease progression. Consequently, identification and treatment of IR in retina, which acts as a potential common pathogenesis and a modifiable risk factor (12), are imperative for the early management of these retinal diseases. In this review, we tend to summarize current evidence supporting the association between IR and certain ocular diseases, including DR, AMD, and glaucoma, and further explore the underlying pathophysiological mechanisms explaining these associations. Given that IR is a modifiable risk factor, investigating the intrinsic relationships between retinal diseases and IR would provide new insights for the administration of certain ocular diseases and even become potential therapeutic targets.
2 Insulin and insulin receptors in the retina
Insulin signal pathways are triggered by the binding of insulin to insulin receptor, a heterotetramer composed of two extracellular α subunits and two transcellular β subunits linked by disulfide bonds. Binding of insulin to the α subunit of insulin receptor leads to structural changes in the β subunit and triggers its intrinsic tyrosine kinase activity (13). The insulin receptor autophosphorylates its β subunit in tyrosine residues and initiates a cascade of downstream effects, such as recruitment of protein substrates of the insulin receptor kinase including the insulin receptor substrates (IRSs), SHC transforming protein, and casitas B-lineage lymphoma (Cbl) (13, 14). IRSs are key substrates in insulin signal transduction by binding to phosphatidylinositol-3-kinase (PI3K) and inducing downstream pathways. Indeed, any factors that reduce IRS-1 phosphorylation or induce serine IRS-1 phosphorylation at the 307 site by some kinases, such as IKKβ/NF-κB and c-Jun N-terminal kinase (JNK), would impair insulin signal transduction and lead to insulin resistance (15, 16). In addition to common target organs (liver, skeletal muscles, adipose tissue, etc.), the insulin receptor has been reported to be expressed constitutively and broadly throughout the retina on neuronal, endothelial, and retinal pigmented epithelial (RPE) cells (17). However, different to the fluctuation with circulating insulin levels during periods of fasting and feeding in the liver and skeletal muscle, the activity of insulin receptor in retina is maintained in a relatively stable state owing to the exquisite regulation of blood retina barrier (BRB) between the plasma and neural retina (18). And the transport of insulin across the BRB to retinal endothelial cells is significantly slower than other vascular beds. Collectively, the retina is not a major target for immediate nutrient metabolism as is skeletal muscle, but the steady-state transport of insulin to the neural retina may provide a stable trophic signal (18). In addition, another interesting observation is that retina and numerous other extra-pancreatic tissues express insulin and insulin-like molecules as a paracrine hormone (19).
In general, regardless of insulin action or production site, insulin signal characteristics in retina resembles what has been described in other system. Specially, the insulin receptor/IRS/PI3K/Akt pathway has received much attention and plays a role in many cellular processes, such as glucose uptake and cellular survival (20) (Figure 1). Meanwhile, insulin resistance associated with diabetes and obesity appears to be due to pathway-selective impairment of PI3K/Akt signaling, whereas SHC/Ras/mitogen activated protein kinase (MAPK) is largely unaffected, thereby tipping the balance of insulin’s actions (21). This imbalance would favor abnormal vasoreactivity, mitogenesis, and other pathways implicated in microangiopathy.
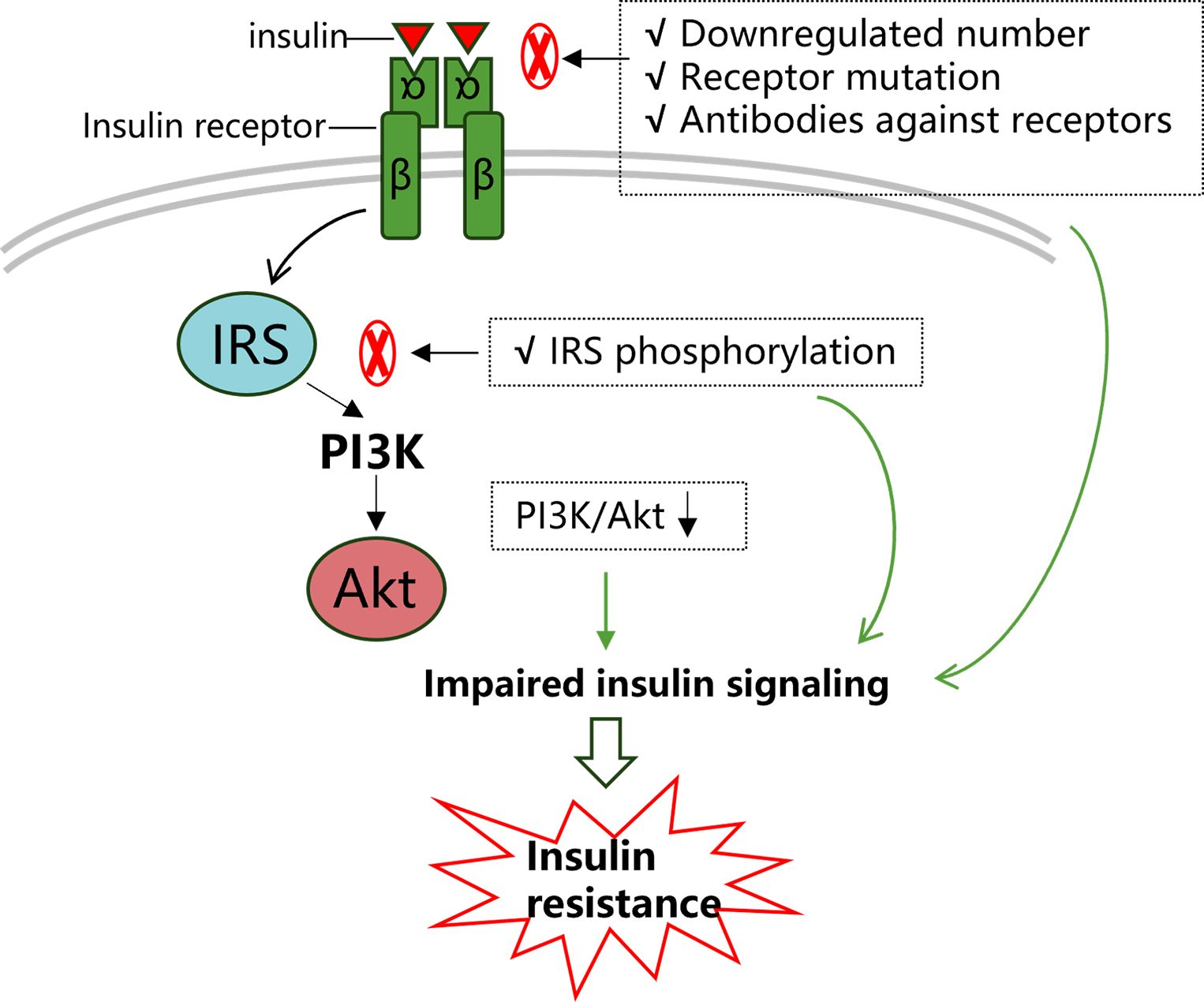
Figure 1 Insulin signaling transduction and insulin resistance. Insulin signaling pathways are complicated, among which the IRS/PI3K/Akt pathway has received much attention as it is well conserved and plays a role in many cellular processes. Firstly, as the capacity of insulin receptors to bind with ligands is a key factor in transduction, a downregulated number of insulin receptors and decreased insulin receptor affinity would significantly restrict normal insulin signaling. The latter could be due to mutations in insulin receptors and autoimmune antibodies against insulin receptors. Subsequently, any factors that reduce IRS phosphorylation or induce IRS phosphorylation at certain sites (such as serine IRS-1 phosphorylation at the 307 site) would impair downstream pathway transduction. Collectively, in this process, any abnormalities in these agents may impair normal insulin signaling transduction and lead to insulin resistance.
3 The measurement and quantification of IR
Methods to quantify IR could be divided into two categories: dynamic tests and static tests. Dynamic tests mainly encompass the hyperinsulinemic euglycemic glucose clamp (HEGC), the frequently sampled intravenous glucose tolerance test (FSIVGTT), and the oral glucose tolerance test (OGTT) (22). Among these dynamic tests, the HEGC technique is the gold standard for measuring IR, and OGTT is the most applied dynamic test. Nevertheless, dynamic methods are resource and time consuming, thus limiting their application in clinical practice. On the contrary, static tests to estimate IR are more available and simpler, among which the homeostatic model assessment of IR (HOMA-IR) is the most commonly used, which is calculated just with the parameters of fasting glucose and insulin (23). Meanwhile, various glycemic indies and serum biomarkers, including insulin, C-peptide, glucose levels, and glycated hemoglobin (HbA1c), are measured to assess pancreatic β-cell function and sensitivity. Other anthropometric characteristics are also used to evaluate IR, such as body mass index (BMI), waist-to-hip circumference ratio (24).
4 The presence and evidence of IR in the retina
4.1 Diabetic retinopathy
T2DM and MetS are two representative diseases of IR, and the latter is considered preclinical diabetes. Individuals with MetS were reported to have a 5-fold increased risk for the development of T2DM (25). Although the diagnosis of T2DM is definite with blood glucose values meeting specific requirements, many patients may experience preclinical diabetes. In this prediabetes period, patients would undergo an unstable hyperglycemic state with a series of metabolic disorders, also known as MetS (4). IR is thought to be involved in the pathophysiology of MetS and diabetes. That is, T2DM is characterized in the first step by the fact that insulin action is impaired and pancreatic β-cells need to synthesize and secrete more insulin to compensate for this IR state, which could lead to hyperinsulinemia. In later stages, the insulin production is lower than normal, and hypoinsulinemia would be observed with the progressive reduced number and functional exhaustion of pancreatic β-cells (26).
DR, one of the most important microvascular complications in diabetes, has been regarded as a neurovascular coupling impairment recently, which includes both retinal neurodegeneration and vasculopathy (27). Whereas hyperglycemia has long been thought to be the primary driver in the progression of diabetic complications, impaired insulin signaling is also suspected to contribute to the retinal pathology. An experimental study suggested that both restoration of glycemic control and retinal insulin signaling can normalize diabetes-induced retinal abnormality via mediating Akt kinase activity, the expression of inflammatory mediators, and lipid synthetic pathway (28).
Meanwhile, it is easy to understand that intensive hypoglycemic therapy in T1DM has been shown to effectively prevent the occurrence and progression of DR (29). However, previous trials evaluating the effects of intensive glycemic control in patients with T2DM have provided inconsistent results (30, 31). The difference of intensive glycemic control may imply that beyond hyperglycemia, inherent risk factor of IR could contribute to the microvascular outcomes in the cohort of T2DM. Similarly, another evidence for the presence of IR has been shown in a study investigating structural and functional changes in adolescents with type 1 and type 2 diabetes. Adolescents with T2DM appeared to be more affected than those with T1DM with more significant multifocal electroretinography (mfERG) implicit time delays and retinal thinning (32). The authors attributed the reason for presenting with more abnormalities in T2DM to the explanation that T2DM patients are faced with more challenges that are inherent to IR, such as elevated BMI, hyperinsulinemia, and dyslipidemia. Additionally, Karaca et al. found that patients of MetS had similar neurodegeneration pattern to those with diabetes with no presence of DR (4). This indicates that inherent mechanisms of MetS, such as IR and adipose tissue-derived inflammation, could contribute to neurodegeneration independent of the diabetic level of hyperglycemia (4).
In addition to reflecting the role of IR in DR indirectly, recent studies have also quantitatively evaluated the relevance of IR-related parameters and DR. It is suggested that IR exacerbates DR, even as an independent predictor (33–35). Moreover, recent advancements in optical coherence tomography (OCT) technology have offered a non-invasive, repeatable monitoring tool for DR with its automated segmentation function. In a previous study, the insulin secretory/resistance parameters, including the fasting insulin level and HOMA-IR and HOMA-B scores in T2DM without DR, revealed the very early microvascular changes measured by OCT angiography (24). Likewise, our previous study indicated that IR (reflected by HOMA-IR values) was an independent risk factor for retinal ganglion cell-inner plexiform layer (GC-IPL) thinning, reflecting retinal neurodegeneration in early T2DM (36). Additionally, a relationship between cystoid macular edema and IR was found by Zapara et al., as IR is a proinflammatory state (37).
Previous experimental studies at the cellular level can also help shed light on the role of IR in DR. Müller cells, activated in the initial stage of DR with increased expression of glial fibrillary acidic protein (GFAP) and other cytokines, play an important role in maintaining retinal homeostasis (38). Experimental data have demonstrated that similar with that in retinal endothelial cells, hyperglycemia environment incudes increased tumor necrosis factor (TNF)-α and suppressors of cytokine-signaling 3 (SOCS3) levels, which subsequently inhabit insulin signaling through the phosphorylation of IRS-1Ser307, a key component of insulin signaling transduction (39). Meanwhile, Silence of insulin receptor substrate (IRS)-1 increases cell death in retinal Müller cells (40, 41). In turn, the mutation of the serine 307 site on IRS-1 could block the inhibitory actions of TNF-α and SOCS3 in insulin signaling, and thereby prevent apoptosis of rat retinal Müller cells (rMC-1) (42). These studies suggests that drug targeted insulin signaling (such as certain IRS-1 sites) could be effective in protecting against diabetic damage to retina.
4.2 Glaucoma
Glaucoma represents a group of neurodegenerative diseases characterized by progressive retinal ganglion cells dysfunction and death. Although intraocular pressure (IOP) is the only modifiable risk factor for glaucoma patients, hypotensive therapies are not sufficient to prevent optic nerve degeneration and visual loss. Obviously, other pathological mechanisms are involved. Despite inconsistent evidence of the association between glaucoma and diabetes (43–45), people with diabetes might be at an increased risk of developing glaucoma after adjusting for some confounding values (46–48). The intrinsic mechanism of this connection could be attributed to IR, a comorbidity of a constellation of metabolic abnormalities (49). IR in the retina involves damage to neurons, blood vessels, and glia [(50)]. A Mendelian randomization study showed that a genetic predisposition to T2DM was associated with an increased risk of primary open angle glaucoma (POAG) in the European population [(51)]. A nationwide population-based longitudinal cohort study found that diabetes status is a predictor of glaucoma development in postmenopausal women. In particular, patients treated with insulin were associated with higher risk of glaucoma (52). Potential reasons for this phenomenon could be that the use of insulin may be a marker for diabetes severity indicating insulin resistance and high glycemic burden (52). The interplay between diabetes and glaucoma, especially POAG, implies that underlying physiological mechanism of IR in diabetes may contribute to this correlation. Possible mechanisms were also postulated in previous studies, including increased IOP, vascular mechanism, and tissue remodeling of the trabecular meshwork and lamina cribrosa (53, 54). An experimental study showed that IR in the CNS induced by intracerebroventricular injection of S961, a potent and specific blocker of insulin receptor, existed independent of systemic IR and overt diabetes (10). In this study, ocular tissue as an extension of the brain was involved in a series of pathological processes brought about by downregulated insulin signaling, including elevated IOP, morphological changes of the trabecular meshwork, ciliary body dysfunction, and apoptosis in the retina and optic nerve (10). This suggests that IR plays a critical role in the pathogenesis of glaucoma, and improving insulin sensitivity could be a potential therapeutic target.
IR could increase the risk of glaucoma through damage to retinal ganglion cells (RGCs) and axons directly or through glial dysfunction and IOP elevation indirectly. Decreasing insulin sensitivity disrupts the balance of insulin signaling transduction, particularly mediated by the PI3K/Akt pathway which serves as a central component modulating insulin-induced neuronal survival (50). In RGCs, activated Akt prevents the transcriptional activity of p53 and decreases the expression of pro-apoptotic proteins, such as Bad, caspase 9, and glycogen synthase kinase 3 beta (GSK3β) (55). And dendritic retraction of RGCs is one of the earliest pathological changes in glaucoma (56). Agostinone et al. demonstrated that insulin signaling promotes dendrite and synapse regeneration after axonal injury through insulin-dependent mTOR1/2 activation (57). Other potential biological mechanisms explaining IR-induced RGCs impairment in glaucoma include mitochondrial dysfunction, Tau hyperphosphorylation, and amyloid disposition (50).
In addition to RGCs themselves, extracellular environment is also crucial for RGCs survival and axonal transduction, including vascular and glial components. In IR conditions, the imbalance between NO and endothelin-1 via PI3K and MAPK-dependent signaling may underlie the vascular endothelial disturbances in glaucoma (58), corresponding to the vascular theory in the development of glaucoma resulting from decreasing perfusion of the optic nerve and intraneural ischemia. Insulin can affect glial activation, participating in neuroinflammation, which is an inextricable pathological process with IR in neurodegenerative diseases (59). Müller cells, astrocytes, and microglial cells are three major types of glia in the retina that maintain retinal homeostasis by taking part in neuroinflammation and regulating neurotrophic molecules (60). Under pathological conditions, reactive activation of Müller cells is involved in inflammation and cell survival processes due to their intrinsic role in counteracting stress damage (61). In addition, Müller cells could regulate the process of oxidative stress through the expression of glutathione (GSH) and glutamate transporters (62, 63). Meanwhile, as mentioned above, insulin signaling pathways (particularly through the PI3K/Akt pathway) in Müller cells are crucial in the defense of oxidative and inflammatory damage (41, 42). Astrocytes, positioned between the vasculature and neurons, form a cellular network with neurons and other cell types to integrate insulin signaling responses in retina. Decreasing insulin signaling has been shown to impact glycogen synthesis (decreasing expression of the insulin-responsive glucose transporter, GLUT-4) and metabolite redistribution (decreasing gap junctions composed of connexin 43 (Cx43)) in astrocytes (64–66). Like other neuronal cells in the central nervous system, RGCs require high energetic and metabolic support. It is conceivable that any pathological changes in the extracellular milieu could influence the survival and functions of RGCs, which would become more susceptible to mechanical (IOP elevation) and chemical (inflammatory factors) stress. Collectively, improving IR or insulin signaling activation could be a promising target for glaucoma treatment.
4.3 Age related macular degeneration
AMD is a leading cause of irreversible vision loss in the elderly population in developed countries (67). Aging worldwide leads to an exponential growth of individuals affected by AMD, and the projected number of this population is 288 million in 2024 (68). AMD can be classified into two forms: dry (also known as nonexudative or atrophic) and wet (also known as exudative and or neovascular). Early AMD is usually asymptomatic, while severe vision loss occurs rapidly in late AMD, which could be further categorized into neovascular AMD and geographic atrophy. The pathogenesis of AMD is complicated with multiple risk factors, including age, genetic variation, systemic diseases, diet, obesity and smoking (69). At present, anti-vascular endothelial growth factor (VEGF) is the first-line treatment for wet AMD, but there is no effective treatment for early AMD or delaying its progression.
According to the International Diabetes Federation (IDF) criteria, MetS represents a constellation of metabolic abnormalities involving centrally distributed obesity, decreased high-density lipoprotein cholesterol, elevated triglycerides, elevated blood pressure, and hyperglycemia. Among these components of MetS, IR is a core feature (70). MetS has long been postulated to be associated with AMD. A previous study provided evidence that a wild-type mouse model of MetS fed with a “fast food” diet showed retinal ultrastructural changes relevant to AMD, including basal laminar deposits, focal thickening of Bruch’s membrane, and a significant loss of retinal pigment epithelium (RPE) cells (71). This in vivo study suggests that a “fast food’’ diet mimicking MetS is sufficient to induce altered retinal morphology, as some features seen in AMD. Furthermore, some epidemiologic evidence from the Blue Mountains Eye Study supported that MetS, obesity, high glucose, and triglycerides were predictors of progression to late AMD with a 10-year follow-up period (72). In addition to MetS, the interplay between AMD and diabetes has attracted increasing attention from various data sources, designs, and ethnic groups (73–77).
Although the potential mechanisms have not been fully elucidated, the increased risk of AMD in individuals with MetS or diabetes is thought to be associated with IR-induced oxidative stress with the accumulation of advanced glycation end products (AGEs) in multiple tissues, including photoreceptors, RPE, Bruch’s membrane, and choroidal circulation (74). Second, IR associated metabolic abnormalities, like hyperglycemia and dyslipidemia, disrupt the homeostasis of the retina by inducing inflammatory responses (78). The accumulation of AGEs and inflammatory activations would lead to RPE/photoreceptors dysfunction and even cellular death, outer retinal hypoxia, and a higher likelihood of developing drusen and abnormal deposits (74, 79, 80). Third, AGEs accumulation may upregulate VEGF expression in RPE cells, which plays an important role in the neovascularization of both exudative AMD and diabetic retinopathy (75, 81). Recently, relevant studies demonstrated a dose-dependent decrease in developing AMD among participants who had taken metformin, the first-line medication used to treat T2DM patients and improve insulin sensitivity (82, 83). These results provide evidence from another perspective regarding the key role of IR in the occurrence and progression of AMD, and improving IR may exert a beneficial impact on the management of AMD patients. Moreover, a biomedical study presented that unlike RPE cells obtained from non-AMD normal control eyes, insulin signaling transduction through phosphorylated ERK 1/2 was impaired in the proliferation of RPE cells from patients with AMD (84). Since insulin is a mitogen for human RPE cells (85), further investigation of insulin related kinase signaling abnormalities in RPE cells from AMD patients may help explore new therapeutic targets.
There is no denying that these data provide new insights into the pathogenesis of AMD, although inconsistent opinions exist regarding the relationships between MetS/T2DM and AMD progression (73, 86–88). As mentioned above, either MetS or T2DM is not a distinct disease entity but a sequence of broader underlying metabolic abnormalities characterized by IR. Herein, we may conclude that inherent IR-related molecular mechanisms in MetS/T2DM patients aggravate the occurrence and progression of AMD. Taken together, further epidemiological and longitudinal studies are needed to investigate the exact relationships between AMD and MetS or T2DM. More biological experiments involving IR associated signal pathways would help elucidate the molecular mechanisms behind these relationships and provide additional strategies for the prevention of AMD.
5 Possible mechanisms of IR associated with retinopathies
As insulin signaling transduction is complicated, involving many enzymes and proteins, any disturbances in the transduction pathways would cause IR. While the exact physiological mechanisms of the interplay between IR and associated retinopathies have not been completely ascertained, potential mechanisms of oxidative stress, subclinical inflammation, vascular mechanisms, and neural impairments may be involved (Figure 2). In this review, we would explore these mechanisms in detail as below. In addition, it should be noted that some other mechanisms have also been postulated previously, including mitochondrial dysfunction, endoplasmic reticulum stress, etc (6).
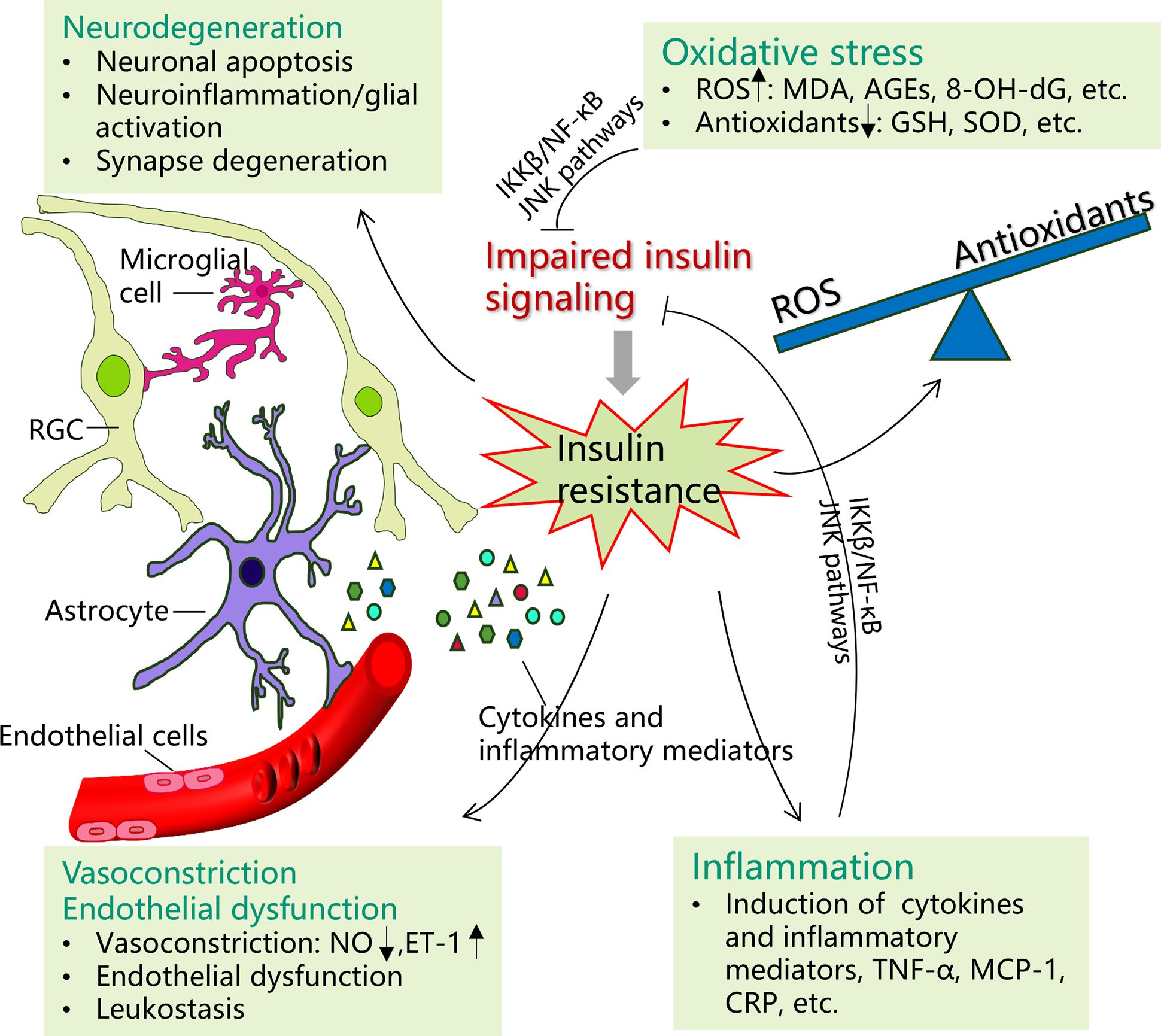
Figure 2 Potential mechanisms of insulin resistance on certain ocular diseases. Possible pathophysiological mechanisms of the interplay between insulin resistance (IR) and certain ocular diseases may include oxidative stress, inflammation, vasoconstriction and endothelial dysfunction, and neurodegeneration. Insulin resistance promotes the production of reactive oxygen species (ROS) (malondialdehyde (MDA), advanced glycation end products (AGEs), 8‐hydroxy‐2′‐deoxyguanosine (8‐OH‐dG), etc.), while the production of antioxidants is decreased, such as glutathione (GSH) and superoxide oxidase (SOD). The imbalance between the ROS and antioxidants would cause oxidative stress, which in turn induce IR by impairing insulin signal transduction. Many inflammatory factors and cytokines, particularly tumor necrosis factor-α (TNF-α), monocyte chemotactic protein-1 (MCP-1), and C-reactive protein (CRP), are upregulated in IR. In turn, both inflammatory agents and oxidative stress can induce IR through activating IKK-β/NF-κB and JNK pathways. Under IR, increasing neuronal apoptosis, glial reactive activation (upregulated neural inflammation), and synapse degeneration are involved. IR additionally contributes to vascular dysfunction by causing nitric oxide/endothelin-1 imbalance (vasoconstriction) and endothelial cell dysfunction.
5.1 Oxidative stress
Although retinal abnormalities in IR patients could be explained by chronic dyslipidemia, hyperinsulinemia and adipose inflammation, the core mechanism of these factors could be attributed to mitochondrial dysfunction and oxidative stress (89). Indeed, there is emerging evidence that retinopathy in IR models is initiated and propagated by multiple metabolic toxicities associated with excess production of reactive oxygen species (ROS) (90). Fernandes and colleagues observed increased oxidative stress and peroxynitrite-mediated protein oxidation in retinas from diabetic GK rats, a model characterized by mild hyperglycemia, insulin resistance, normolipidemia, and hyperinsulinemia (91). An increase in markers of oxidative stress, including gp91phox, MDA and 8OH-dG, was observed in the inner retina of high-fat diet (HFD) mice, indicating increased oxidative DNA and lipid peroxidation injury were present in obese and insulin-resistant animals compared to chow diet (CD) fed controls (92). Generally, oxidative stress is defined as an imbalance between the production of reactive oxygen species (ROS) and endogenous antioxidants, which includes enzymic antioxidants such as superoxide oxidase (SOD), catalase (CAT) and glutathione peroxidase, and non-enzymic antioxidants such as GSH (93–95). Accordingly, when the redox balance is upset, the oxidative damage would occur in which the level of ROS exceeds the capacity of antioxidative defense system to neutralize them. Overproduction of ROS results in oxidative damage to cell structure and subsequently modifying cell function, including lipids loss and increased membrane permeability, modifying nucleic acids leading to mutations and apoptosis, as well as oxidatively modified proteins (96). Collectively, hyperglycemia, oxidative stress, and redox homeostasis changes are fundamental events in the pathogenesis of IR associated retinopathies, contributing to the production of pro-inflammatory cytokines, abnormalities of multiple retinal cells, and extracellular matrix remodeling (97).
Under IR circumstances, disturbances of oxidative and antioxidative products have been reported in the tissues of liver, skeletal muscle, kidney and brain (22, 98–100). Additionally, the retina is particularly sensitive to oxidative stress, considering the high oxygen demand and consumption and its high concentration of polyunsaturated fatty acids (PUFA) in the outer segments of photoreceptor (101). Although PUFA are thought to protect against oxidative and inflammatory damage, they may also serve as a substrate for free radicals under special conditions as they provide an available source of electrons (94). Therefore, oxidative stress under IR conditions with overproduction of ROS plays a prominent role in retinopathies.
5.2 Chronic low-grade inflammation
It is recognized that subclinical chronic inflammation and activation of the immune system are involved in the pathogenesis of IR-related metabolic disorders and even seem to be independent risk factors (102). Pro-inflammatory cytokines (interleukin (IL)-1β, IL-6, and TNF-α), chemokines, and acute-phase proteins such as C-reactive protein (CRP) are elevated in IR (103, 104). These pro-inflammatory makers promote IR by interfering with the c-JUN N-terminal kinase (JNK), nuclear factor-kappa B (NF-κB), and the NADPH oxidative pathways (105), which could activate serine phosphorylation of IRS-1 and further block insulin signaling proteins (such as PI3K-Akt and AMPK) (106). Meanwhile, inflammatory reaction and oxidative stress are two interrelated processes (79). In this process, the activation of oxidative stress may regulate inflammatory genes and lead to inflammation, which would in turn aggregate oxidative stress. In addition, it is reported that SOCS proteins induced by inflammation cytokines can trigger IR and inhibit pro-survival insulin signaling pathways in retina (20), supporting that SOCS-mediated IRS degradation inhabits insulin signaling transduction via insulin receptor. Accordingly, these studies provide evidence about a link between IR and inflammation.
5.3 Endothelial dysfunction and vasoconstriction
Endothelial dysfunction, closely related to IR, is the core of microvascular complications (107). To some extent, IR has a close relationship with systemic metabolic abnormalities, such as hyperglycemia and dyslipidemia, so a link between IR and microvascular dysfunction is not surprising. Insulin has been known to play a direct role in microvascular physiology. In endothelial cells, insulin-mediated Akt activation exerts antiapoptotic effects via phosphorylation of caspase-9 (108). Insulin is a potent vasoactive hormone, which is demonstrated to stimulate the expression and activation of endothelial NO synthase via PI3K pathway (50). Endothelial cell-mediated autoregulation of blood flow is sustained by a balance between NO-dependent vasodilation and the activation of vasoconstrictor mechanisms, including endothelin-1 (ET-1), sympathetic activity, and the renin-angiotensin system (21). In IR conditions, impairments of the PI3K and AMPK-dependent signaling may cause an imbalance between NO and ET-1 and lead to endothelial dysfunction and ischemic injury (109). Moreover, retinal leukostasis, an important contributor to capillary occlusion in ischemic retinopathies, is correlated to endothelial dysfunction and could be enhanced by IR independently (110).
5.4 Neurodegenerative impairments
Insulin and insulin-like growth factor have been reported to play a role in the survival of RGCs, which are responsible for transmitting visual information together with their axons from the eye to the brain (5). That is, functional insulin and downstream elements in insulin signaling pathways are required in neurodegenerative diseases. In addition to vascular theory, neurodegenerative impairments have been increasingly recognized among the diseases listed above, no matter whether primary or secondary to primary disorders. With regard to neurodegenerative diseases, the term “neurovascular unit” was put forward and first applied to blood-brain barrier and then to retina, which refers to the functional coupling and interdependency of neurons, glia, and vasculature (111). Based on this concept, retinal neurodegenerative diseases not only include neural-cell apoptosis but also glial activation and neural function impairments. It is suggested that neuroinflammation and microglial activation play a central role in the pathophysiology of neurodegenerative diseases (112). Consistent with that, microglial cells are shown to be the main source of ROS, TNF-α, and glutamate, all of which are neurotoxic when released at a high level after glial activation induced by the stimulus of toll-like receptors (TLRs) and NF-κB (113, 114). As mentioned above, PI3K and Akt are identified as central components mediating downstream insulin-induced neuronal survival, neuroinflammation, and dendritic regeneration (50). In an experimental study of type 2 diabetic rats, insulin receptors and downstream Akt signaling were found to regulate neural cell survival (115). In addition, although the exact mechanism has not yet been elucidated, IR is suggested to be associated with elevated intraocular pressure (IOP) (116, 117), subsequently causing mechanical impairments for RGCs. Therefore, targeting activation of insulin signaling and improve IR could serve as effective neuro-protective therapy.
6 Potential treatment target of IR in retinal diseases
In the CNS, restoring insulin signaling has significantly prevented cognitive decline in Alzheimer’s disease (AD) through the administration of intranasal insulin without altering blood insulin and glucose levels (118). IR is shown to be directly correlated with AD pathology, including the production and clearance of Aβ peptide, tau hyperphosphorylation, immune function, and inflammatory markers (119, 120). As listed above, though the occurrence of DR, AMD, and glaucoma is multifactorial with distinct clinical features, IR may be a common risk factor causing retinal impairments through pathophysiological mechanisms involving oxidative stress, chronic inflammation, vascular endothelial dysfunction, and retinal neural loss, etc. Moreover, in addition to above retinal diseases we have reviewed, retinal vein occlusion has also been proposed to be inextricably linked to IR (49). Thus, it is conceivable that the actions of insulin signaling pathway exert an important effect on the viability of retinal cells through the activation of downstream pro-survival kinases; thereby, the restoration of insulin signaling transduction could be an underlying therapeutic target in certain retinal diseases. This point is of vital importance for the early management of patients with retinal diseases, given that current available treatments in retinal diseases could not achieve desired results. For example, either surgical intervention or retinal photocoagulation is largely destructive, both of which only delay disease progression. There is, however, a small percentage of eyes (15–40%) that fail to respond or only partially respond to intravitreal injections of vascular endothelial growth factor antagonists (121).
In fact, IR is not equal to hypoinsulinemia, but a decreased sensitivity of insulin receptors and impaired activation of specific downstream pathways. As stated in previous studies, in insulin-responsive tissues such as the heart, liver, and adipose tissue, IR is an adaptive defense mechanism that, to some extent, could be able to protect the tissues from nutrient-induced damage (122). Insulin-induced metabolic stress is likely to occur with systemic high-dose insulin therapy without nutrient off-loading in refractory patients (123). Therefore, no matter whether it is systemic treatment or topical retinal administration, improving IR not only means intensive exogenous insulin usage, but also improves the sensitivity of insulin receptors and the transduction of certain insulin pathways. Undoubtedly, insulin, a primary anabolic peptide, could play a key role in the regulation of retinal functions. Nevertheless, it should be emphasized that insulin signaling pathways are complex, in which selective activation or specific mutation may cause different phenotypes. Many detailed mechanisms of insulin signaling molecules remain undiscovered currently and deserve further investigation. Another alternative approach to improve IR may be lifestyle modification aimed at losing weight through restricted calorie intake and increased physical activity (71, 124). Future prospective longitudinal studies are also needed to validate whether lifestyle intervention would be effective to reduce the incidence and delay the progression of retinal diseases.
7 Conclusion
IR could be defined as reduced insulin sensitivity in target organs, which represents the comorbidity of a sequence of metabolic abnormalities, such as obesity, hyperglycemia, and dyslipidemia. In addition to common target organs (liver, skeletal muscle, etc.), the retina has also been demonstrated to be insulin-sensitive, in which insulin participates in many cellular processes and plays a key role in retinal cell survival. Previous studies have provided evidence that IR is closely associated with several retinal diseases, especially DR, AMD, and glaucoma. Possible explanations for these associations include oxidative stress, chronic low-grade inflammation, endothelial dysfunction and vasoconstriction, and neurodegenerative impairments. Thus, it may be important to monitor the IR conditions in populations and identify those at high IR levels. Subsequently, improving IR may provide new insights for the prevention and treatment of certain retinal diseases. Nevertheless, it should be noted that, on the one hand, the insulin signaling pathways are complex, and on the other hand, current prospective studies regarding the role of improving IR in the management of certain retinal diseases are relatively lacking. Consequently, more clinical and experimental studies are warranted to validate the mutual relationships between IR and certain retinal diseases, which would be beneficial for the management of certain ocular diseases.
Author contributions
ZZ: Conceptualization, Investigation, Writing – original draft. XY: Supervision, Validation, Writing – review & editing.
Funding
The author(s) declare financial support was received for the research, authorship, and/or publication of this article. This work was supported by the National High Level Hospital Clinical Research Funding [grant number: BJ-2022-104].
Conflict of interest
The authors declare that the research was conducted in the absence of any commercial or financial relationships that could be construed as a potential conflict of interest.
Publisher’s note
All claims expressed in this article are solely those of the authors and do not necessarily represent those of their affiliated organizations, or those of the publisher, the editors and the reviewers. Any product that may be evaluated in this article, or claim that may be made by its manufacturer, is not guaranteed or endorsed by the publisher.
References
1. Brown AE, Walker M. Genetics of insulin resistance and the metabolic syndrome. Curr Cardiol Rep. (2016) 18:75. doi: 10.1007/s11886-016-0755-4
2. Chen J, Wu K, Lin Y, Huang M, Xie S. Association of triglyceride glucose index with all-cause and cardiovascular mortality in the general population. Cardiovasc diabetol. (2023) 22:320. doi: 10.1186/s12933-023-02054-5
3. Tan L, Liu Y, Liu J, Zhang G, Liu Z, Shi R. Association between insulin resistance and uncontrolled hypertension and arterial stiffness among US adults: a population-based study. Cardiovasc diabetol. (2023) 22:311. doi: 10.1186/s12933-023-02038-5
4. Karaca C, Karaca Z. Beyond hyperglycemia, evidence for retinal neurodegeneration in metabolic syndrome. Invest Ophthalmol Visual science. (2018) 59:1360–7. doi: 10.1167/iovs.17-23376
5. Song BJ, Aiello LP, Pasquale LR. Presence and risk factors for glaucoma in patients with diabetes. Curr Diabetes Rep. (2016) 16:124. doi: 10.1007/s11892-016-0815-6
6. Yaribeygi H, Farrokhi FR, Butler AE, Sahebkar A. Insulin resistance: Review of the underlying molecular mechanisms. J Cell Physiol. (2019) 234:8152–61. doi: 10.1002/jcp.27603
7. Öztürk H, Özen B, Çatlı G, Dündar BN. Macular variability in children and adolescents with metabolic syndrome: A cross-sectional study examining the associations with anthropometric measurements, metabolic parameters and inflammatory markers. J Clin Res Pediatr endocrinol. (2020) 12:63–70. doi: 10.4274/jcrpe
8. Wang J, Li L, Zhang Z, Zhang X, Zhu Y, Zhang C, et al. Extracellular vesicles mediate the communication of adipose tissue with brain and promote cognitive impairment associated with insulin resistance. Cell Metab. (2022) 34:1264–79.e8. doi: 10.1016/j.cmet.2022.08.004
9. Zhang B, Hu L, Zhang J, Wu H, Li W, Gou L, et al. Insulin growth factor-1 enhances proliferation and inhibits apoptosis of neural progenitor cells by phosphorylation of Akt/mTOR/p70S6K molecules and triggering intrinsic apoptosis signaling pathway. Cell Tissue banking. (2022) 23:459–72. doi: 10.1007/s10561-021-09956-2
10. Faiq MA, Sengupta T, Nath M, Velpandian T, Saluja D, Dada R, et al. Ocular manifestations of central insulin resistance. Neural regeneration Res. (2023) 18:1139–46. doi: 10.4103/1673-5374.355765
11. Roddy GW. Metabolic syndrome and the aging retina. Curr Opin ophthalmol. (2021) 32:280–7. doi: 10.1097/ICU.0000000000000747
12. Castro EF, Mostarda CT, Rodrigues B, Moraes-Silva IC, Feriani DJ, De Angelis K, et al. Exercise training prevents increased intraocular pressure and sympathetic vascular modulation in an experimental model of metabolic syndrome. Braz J Med Biol Res = Rev Bras pesquisas medicas e biologicas. (2015) 48:332–8. doi: 10.1590/1414-431x20144217
13. Færch K, Vistisen D, Pacini G, Torekov SS, Johansen NB, Witte DR, et al. Insulin resistance is accompanied by increased fasting glucagon and delayed glucagon suppression in individuals with normal and impaired glucose regulation. Diabetes. (2016) 65:3473–81. doi: 10.2337/db16-0240
14. Kiselyov VV, Versteyhe S, Gauguin L, De Meyts P. Harmonic oscillator model of the insulin and IGF1 receptors' allosteric binding and activation. Mol Syst Biol. (2009) 5:243. doi: 10.1038/msb.2008.78
15. Aguirre V, Werner ED, Giraud J, Lee YH, Shoelson SE, White MF. Phosphorylation of Ser307 in insulin receptor substrate-1 blocks interactions with the insulin receptor and inhibits insulin action. J Biol Chem. (2002) 277:1531–7. doi: 10.1074/jbc.M101521200
16. Hirosumi J, Tuncman G, Chang L, Görgün CZ, Uysal KT, Maeda K, et al. Author Correction: A central role for JNK in obesity and insulin resistance. Nature. (2023) 619:E25. doi: 10.1038/s41586-023-06285-0
17. Gosbell AD, Favilla I, Jablonski P. The location of insulin receptors in bovine retina and isolated retinal cells. Clin Exp ophthalmol. (2002) 30:124–30. doi: 10.1046/j.1442-6404.2002.00499.x
18. Reiter CE, Gardner TW. Functions of insulin and insulin receptor signaling in retina: possible implications for diabetic retinopathy. Prog retinal eye Res. (2003) 22:545–62. doi: 10.1016/S1350-9462(03)00035-1
19. Gray SM, Meijer RI, Barrett EJ. Insulin regulates brain function, but how does it get there? Diabetes. (2014) 63:3992–7. doi: 10.2337/db14-0340
20. Liu X, Mameza MG, Lee YS, Eseonu CI, Yu CR, Kang Derwent JJ, et al. Suppressors of cytokine-signaling proteins induce insulin resistance in the retina and promote survival of retinal cells. Diabetes. (2008) 57:1651–8. doi: 10.2337/db07-1761
21. Groop PH, Forsblom C, Thomas MC. Mechanisms of disease: Pathway-selective insulin resistance and microvascular complications of diabetes. Nat Clin Pract Endocrinol Metab. (2005) 1:100–10. doi: 10.1038/ncpendmet0046
22. Spoto B, Pisano A, Zoccali C. Insulin resistance in chronic kidney disease: a systematic review. Am J Physiol Renal Physiol. (2016) 311:F1087–f108. doi: 10.1152/ajprenal.00340.2016
23. Matthews DR, Hosker JP, Rudenski AS, Naylor BA, Treacher DF, Turner RC. Homeostasis model assessment: insulin resistance and beta-cell function from fasting plasma glucose and insulin concentrations in man. Diabetologia. (1985) 28:412–9. doi: 10.1007/BF00280883
24. Choi EY, Park SE, Lee SC, Koh HJ, Kim SS, Byeon SH, et al. Association between clinical biomarkers and optical coherence tomography angiography parameters in type 2 diabetes mellitus. Invest Ophthalmol Visual science. (2020) 61:4. doi: 10.1167/iovs.61.3.4
25. Wild S, Roglic G, Green A, Sicree R, King H. Global prevalence of diabetes: estimates for the year 2000 and projections for 2030. Diabetes Care. (2004) 27:1047–53. doi: 10.2337/diacare.27.5.1047
26. Prentki M, Nolan CJ. Islet beta cell failure in type 2 diabetes. J Clin Invest. (2006) 116:1802–12. doi: 10.1172/JCI29103
27. Ji L, Tian H, Webster KA, Li W. Neurovascular regulation in diabetic retinopathy and emerging therapies. Cell Mol Life Sci CMLS. (2021) 78:5977–85. doi: 10.1007/s00018-021-03893-9
28. Fort PE, Losiewicz MK, Reiter CE, Singh RS, Nakamura M, Abcouwer SF, et al. Differential roles of hyperglycemia and hypoinsulinemia in diabetes induced retinal cell death: evidence for retinal insulin resistance. PLoS One. (2011) 6:e26498. doi: 10.1371/journal.pone.0026498
29. Genuth S. Insights from the diabetes control and complications trial/epidemiology of diabetes interventions and complications study on the use of intensive glycemic treatment to reduce the risk of complications of type 1 diabetes. Endocrine Pract. (2006) 12 Suppl 1:34–41. doi: 10.4158/EP.12.S1.34
30. Ismail-Beigi F, Craven T, Banerji MA, Basile J, Calles J, Cohen RM, et al. Effect of intensive treatment of hyperglycaemia on microvascular outcomes in type 2 diabetes: an analysis of the ACCORD randomised trial. Lancet (London England). (2010) 376:419–30. doi: 10.1016/S0140-6736(10)60576-4
31. Duckworth W, Abraira C, Moritz T, Reda D, Emanuele N, Reaven PD, et al. Glucose control and vascular complications in veterans with type 2 diabetes. New Engl J Med. (2009) 360:129–39. doi: 10.1056/NEJMoa0808431
32. Bronson-Castain KW, Bearse MA Jr., Neuville J, Jonasdottir S, King-Hooper B, Barez S, et al. Early neural and vascular changes in the adolescent type 1 and type 2 diabetic retina. Retina (Philadelphia Pa). (2012) 32:92–102. doi: 10.1097/IAE.0b013e318219deac
33. Tung TH, Shih HC, Tsai ST, Chou P, Chen SJ, Lee FL, et al. A community-based study of the relationship between insulin resistance/beta-cell dysfunction and diabetic retinopathy among type II diabetics in Kinmen, Taiwan. Ophthalmic Epidemiol. (2007) 14:148–54. doi: 10.1080/09286580601139220
34. Bao YK, Yan Y, Wilson B, Gordon MO, Semenkovich CF, Rajagopal R. Association of retinopathy and insulin resistance: NHANES 2005-2008. Curr eye Res. (2020) 45:173–6. doi: 10.1080/02713683.2019.1659977
35. Gao L, Xin Z, Yuan MX, Cao X, Feng JP, Shi J, et al. High prevalence of diabetic retinopathy in diabetic patients concomitant with metabolic syndrome. PLoS One. (2016) 11:e0145293. doi: 10.1371/journal.pone.0145293
36. Zheng Z, Yan M, Zhang D, Li L, Zhang L. Quantitatively evaluating the relationships between insulin resistance and retinal neurodegeneration with optical coherence tomography in early type 2 diabetes mellitus. Ophthalmic Res. (2023) 66:968–77. doi: 10.1159/000530904
37. Zapata MA, Badal J, Fonollosa A, Boixadera A, García-Arumí J. Insulin resistance and diabetic macular oedema in type 2 diabetes mellitus. Br J ophthalmol. (2010) 94:1230–2. doi: 10.1136/bjo.2009.171702
38. Rolev KD, Shu XS, Ying Y. Targeted pharmacotherapy against neurodegeneration and neuroinflammation in early diabetic retinopathy. Neuropharmacology. (2021) 187:108498. doi: 10.1016/j.neuropharm.2021.108498
39. Jiang Y, Pagadala J, Miller D, Steinle JJ. Reduced insulin receptor signaling in retinal Müller cells cultured in high glucose. Mol vision. (2013) 19:804–11.
40. Walker RJ, Anderson NM, Bahouth S, Steinle JJ. Silencing of insulin receptor substrate-1 increases cell death in retinal Müller cells. Mol vision. (2012) 18:271–9.
41. Jiang Y, Liu L, Li H, Wang JM, Steinle JJ. Insulin signal transduction is impaired in the type 2 diabetic retina. J Diabetes Clin Res. (2020) 2:12–5. doi: 10.33696/diabetes
42. Jiang Y, Biswas SK, Steinle JJ. Serine 307 on insulin receptor substrate 1 is required for SOCS3 and TNF-α signaling in the rMC-1 cell line. Mol vision. (2014) 20:1463–70.
43. Laville V, Kang JH, Cousins CC, Iglesias AI, Nagy R, Cooke Bailey JN, et al. Genetic correlations between diabetes and glaucoma: an analysis of continuous and dichotomous phenotypes. Am J ophthalmol. (2019) 206:245–55. doi: 10.1016/j.ajo.2019.05.015
44. Lavaju P, Shah S, Sharma S, Maskey R. Diabetes Mellitus and the risk of Primary open angle glaucoma. Nepalese J Ophthalmol. (2017) 9:17–23. doi: 10.3126/nepjoph.v9i1.17526
45. Tan GS, Wong TY, Fong CW, Aung T. Diabetes, metabolic abnormalities, and glaucoma. Arch Ophthalmol (Chicago Ill 1960). (2009) 127:1354–61. doi: 10.1001/archophthalmol.2009.268
46. Zhao D, Cho J, Kim MH, Friedman D, Guallar E. Diabetes, glucose metabolism, and glaucoma: the 2005-2008 National Health and Nutrition Examination Survey. PloS One. (2014) 9:e112460. doi: 10.1371/journal.pone.0112460
47. Vergroesen JE, Thee EF, Ahmadizar F, van Duijn CM, Stricker BH, Kavousi M, et al. Association of diabetes medication with open-angle glaucoma, age-related macular degeneration, and cataract in the Rotterdam study. JAMA ophthalmol. (2022) 140:674–81. doi: 10.1001/jamaophthalmol.2022.1435
48. Zhao D, Cho J, Kim MH, Friedman DS, Guallar E. Diabetes, fasting glucose, and the risk of glaucoma: a meta-analysis. Ophthalmology. (2015) 122:72–8. doi: 10.1016/j.ophtha.2014.07.051
49. Stewart RM, Clearkin LG. Insulin resistance and autoregulatory dysfunction in glaucoma and retinal vein occlusion. Am J ophthalmol. (2008) 145:394–6. doi: 10.1016/j.ajo.2007.11.005
50. Al Hussein Al Awamlh S, Wareham LK, Risner ML, Calkins DJ. Insulin signaling as a therapeutic target in glaucomatous neurodegeneration. Int J Mol Sci. (2021) 22. doi: 10.3390/ijms22094672
51. Hu Z, Zhou F, Kaminga AC, Xu H. Type 2 diabetes, fasting glucose, hemoglobin A1c levels and risk of primary open-angle glaucoma: A Mendelian randomization study. Invest Ophthalmol Visual science. (2022) 63:37. doi: 10.1167/iovs.63.5.37
52. Jung Y, Han K, Ohn K, Kim DR, Moon JI. Association between diabetes status and subsequent onset of glaucoma in postmenopausal women. Sci Rep. (2021) 11:18272. doi: 10.1038/s41598-021-97740-3
53. Sim YS, Kwon JW, Jee D, Choi JA, Ko SH, Park CK. Increased prelaminar tissue thickness in patients with open-angle glaucoma and type 2 diabetes. PLoS One. (2019) 14:e0211641. doi: 10.1371/journal.pone.0211641
54. Wong VH, Bui BV, Vingrys AJ. Clinical and experimental links between diabetes and glaucoma. Clin Exp optometry. (2011) 94:4–23. doi: 10.1111/j.1444-0938.2010.00546.x
55. Yamaguchi A, Tamatani M, Matsuzaki H, Namikawa K, Kiyama H, Vitek MP, et al. Akt activation protects hippocampal neurons from apoptosis by inhibiting transcriptional activity of p53. J Biol Chem. (2001) 276:5256–64. doi: 10.1074/jbc.M008552200
56. Agostinone J, Di Polo A. Retinal ganglion cell dendrite pathology and synapse loss: Implications for glaucoma. Prog Brain Res. (2015) 220:199–216. doi: 10.1016/bs.pbr.2015.04.012
57. Agostinone J, Alarcon-Martinez L, Gamlin C, Yu WQ, Wong ROL, Di Polo A. Insulin signalling promotes dendrite and synapse regeneration and restores circuit function after axonal injury. Brain. (2018) 141:1963–80. doi: 10.1093/brain/awy142
58. Muniyappa R, Sowers JR. Role of insulin resistance in endothelial dysfunction. Rev endocrine Metab Disord. (2013) 14:5–12. doi: 10.1007/s11154-012-9229-1
59. Ferreira ST, Clarke JR, Bomfim TR, De Felice FG. Inflammation, defective insulin signaling, and neuronal dysfunction in Alzheimer's disease. Alzheimer's dementia J Alzheimer's Assoc. (2014) 10:S76–83. doi: 10.1016/j.jalz.2013.12.010
60. García-Bermúdez MY, Freude KK, Mouhammad ZA, van Wijngaarden P, Martin KK, Kolko M. Glial cells in glaucoma: friends, foes, and potential therapeutic targets. Front neurology. (2021) 12:624983. doi: 10.3389/fneur.2021.624983
61. Eastlake K, Luis J, Limb GA. Potential of Müller glia for retina neuroprotection. Curr eye Res. (2020) 45:339–48. doi: 10.1080/02713683.2019.1648831
62. Huster D, Reichenbach A, Reichelt W. The glutathione content of retinal Müller (glial) cells: effect of pathological conditions. Neurochemistry Int. (2000) 36:461–9. doi: 10.1016/S0197-0186(99)00149-7
63. Bringmann A, Grosche A, Pannicke T, Reichenbach A. GABA and glutamate uptake and metabolism in retinal glial (Müller) cells. Front endocrinol. (2013) 4:48. doi: 10.3389/fendo.2013.00048
64. González-García I, Gruber T, García-Cáceres C. Insulin action on astrocytes: From energy homeostasis to behaviour. J neuroendocrinol. (2021) 33:e12953. doi: 10.1111/jne.12953
65. Sánchez-Chávez G, Peña-Rangel MT, Riesgo-Escovar JR, Martínez-Martínez A, Salceda R. Insulin stimulated-glucose transporter Glut 4 is expressed in the retina. PLoS One. (2012) 7:e52959. doi: 10.1371/journal.pone.0052959
66. Cooper ML, Pasini S, Lambert WS, D'Alessandro KB, Yao V, Risner ML, et al. Redistribution of metabolic resources through astrocyte networks mitigates neurodegenerative stress. Proc Natl Acad Sci U S A. (2020) 117:18810–21. doi: 10.1073/pnas.2009425117
67. Kawasaki R, Yasuda M, Song SJ, Chen SJ, Jonas JB, Wang JJ, et al. The prevalence of age-related macular degeneration in Asians: a systematic review and meta-analysis. Ophthalmology. (2010) 117:921–7. doi: 10.1016/j.ophtha.2009.10.007
68. Wong WL, Su X, Li X, Cheung CM, Klein R, Cheng CY, et al. Global prevalence of age-related macular degeneration and disease burden projection for 2020 and 2040: a systematic review and meta-analysis. Lancet Global Health. (2014) 2:e106–16. doi: 10.1016/S2214-109X(13)70145-1
69. Mitchell P, Liew G, Gopinath B, Wong TY. Age-related macular degeneration. Lancet (London England). (2018) 392:1147–59. doi: 10.1016/S0140-6736(18)31550-2
70. Zimmet P, Magliano D, Matsuzawa Y, Alberti G, Shaw J. The metabolic syndrome: a global public health problem and a new definition. J Atheroscl thrombosis. (2005) 12:295–300. doi: 10.5551/jat.12.295
71. Roddy GW, Rosa RH, Viker KB, Holman BH, Hann CR, Krishnan A, et al. Diet mimicking "Fast food" Causes structural changes to the retina relevant to age-related macular degeneration. Curr eye Res. (2020) 45:726–32. doi: 10.1080/02713683.2019.1694156
72. Ghaem Maralani H, Tai BC, Wong TY, Tai ES, Li J, Wang JJ, et al. Metabolic syndrome and risk of age-related macular degeneration. Retina (Philadelphia Pa). (2015) 35:459–66. doi: 10.1097/IAE.0000000000000338
73. Yongpeng Z, Yaxing W, Jinqiong Z, Qian W, Yanni Y, Xuan Y, et al. The association between diabetic retinopathy and the prevalence of age-related macular degeneration-the Kailuan eye study. Front Public Health. (2022) 10:922289. doi: 10.3389/fpubh.2022.922289
74. Hwang S, Kang SW, Kim SJ, Lee KN, Han K, Lim DH. Diabetes-related risk factors for exudative age-related macular degeneration: A nationwide cohort study of a diabetic population. Invest Ophthalmol Visual science. (2023) 64:10. doi: 10.1167/iovs.64.10.10
75. He MS, Chang FL, Lin HZ, Wu JL, Hsieh TC, Lee YC. The association between diabetes and age-related macular degeneration among the elderly in Taiwan. Diabetes Care. (2018) 41:2202–11. doi: 10.2337/dc18-0707
76. Hahn P, Acquah K, Cousins SW, Lee PP, Sloan FA. Ten-year incidence of age-related macular degeneration according to diabetic retinopathy classification among medicare beneficiaries. Retina (Philadelphia Pa). (2013) 33:911–9. doi: 10.1097/IAE.0b013e3182831248
77. Topouzis F, Anastasopoulos E, Augood C, Bentham GC, Chakravarthy U, de Jong PT, et al. Association of diabetes with age-related macular degeneration in the EUREYE study. Br J ophthalmol. (2009) 93:1037–41. doi: 10.1136/bjo.2008.146316
78. Zhang W, Liu H, Al-Shabrawey M, Caldwell RW, Caldwell RB. Inflammation and diabetic retinal microvascular complications. J Cardiovasc Dis Res. (2011) 2:96–103. doi: 10.4103/0975-3583.83035
79. Chen X, Rong SS, Xu Q, Tang FY, Liu Y, Gu H, et al. Diabetes mellitus and risk of age-related macular degeneration: a systematic review and meta-analysis. PloS One. (2014) 9:e108196. doi: 10.1371/journal.pone.0108196
80. Venza I, Visalli M, Cucinotta M, Teti D, Venza M. Association between oxidative stress and macromolecular damage in elderly patients with age-related macular degeneration. Aging Clin Exp Res. (2012) 24:21–7. doi: 10.1007/BF03654791
81. McFarlane S, Glenn JV, Lichanska AM, Simpson DA, Stitt AW. Characterisation of the advanced glycation endproduct receptor complex in the retinal pigment epithelium. Br J ophthalmol. (2005) 89:107–12. doi: 10.1136/bjo.2004.045914
82. Blitzer AL, Ham SA, Colby KA, Skondra D. Association of metformin use with age-related macular degeneration: A case-control study. JAMA ophthalmol. (2021) 139:302–9. doi: 10.1001/jamaophthalmol.2020.6331
83. Brown EE, Ball JD, Chen Z, Khurshid GS, Prosperi M, Ash JD. The common antidiabetic drug metformin reduces odds of developing age-related macular degeneration. Invest Ophthalmol Visual science. (2019) 60:1470–7. doi: 10.1167/iovs.18-26422
84. Kothary PC, Del Monte MA. A possible impaired signaling mechanism in human retinal pigment epithelial cells from patients with macular degeneration. Adv Exp Med Biol. (2008) 613:269–75. doi: 10.1007/978-0-387-74904-4_31
85. Campochiaro PA, Hackett SF, Conway BP. Retinoic acid promotes density-dependent growth arrest in human retinal pigment epithelial cells. Invest Ophthalmol Visual science. (1991) 32:65–72.
86. Singh SR, Parameswarappa DC, Govindahari V, Lupidi M, Chhablani J. Clinical and angiographic characterization of choroidal neovascularization in diabetic retinopathy. Eur J ophthalmol. (2021) 31:584–91. doi: 10.1177/1120672120902027
87. Cackett P, Yeo I, Cheung CM, Vithana EN, Wong D, Tay WT, et al. Relationship of smoking and cardiovascular risk factors with polypoidal choroidal vasculopathy and age-related macular degeneration in Chinese persons. Ophthalmology. (2011) 118:846–52. doi: 10.1016/j.ophtha.2010.09.026
88. Chakravarthy U, Wong TY, Fletcher A, Piault E, Evans C, Zlateva G, et al. Clinical risk factors for age-related macular degeneration: a systematic review and meta-analysis. BMC ophthalmol. (2010) 10:31. doi: 10.1186/1471-2415-10-31
89. Maciejczyk M, Żebrowska E, Chabowski A. Insulin resistance and oxidative stress in the brain: what's new? Int J Mol Sci. (2019) 20. doi: 10.3390/ijms20040874
90. Mima A, Qi W, Hiraoka-Yamomoto J, Park K, Matsumoto M, Kitada M, et al. Retinal not systemic oxidative and inflammatory stress correlated with VEGF expression in rodent models of insulin resistance and diabetes. Invest Ophthalmol Visual science. (2012) 53:8424–32. doi: 10.1167/iovs.12-10207
91. Fernandes R, Bento CF, Matafome P, Sena CM, Seiça RM, Pereira P. Atorvastatin-mediated protection of the retina in a model of diabetes with hyperlipidemia. Can J Physiol Pharmacol. (2014) 92:1037–43. doi: 10.1139/cjpp-2014-0212
92. Lee JJ, Wang PW, Yang IH, Huang HM, Chang CS, Wu CL, et al. High-fat diet induces toll-like receptor 4-dependent macrophage/microglial cell activation and retinal impairment. Invest Ophthalmol Visual science. (2015) 56:3041–50. doi: 10.1167/iovs.15-16504
93. Lushchak VI. Free radicals, reactive oxygen species, oxidative stress and its classification. Chemico-biological interactions. (2014) 224:164–75. doi: 10.1016/j.cbi.2014.10.016
94. Scanlon G, Loughman J, Farrell D, McCartney D. A review of the putative causal mechanisms associated with lower macular pigment in diabetes mellitus. Nutr Res Rev. (2019) 32:247–64. doi: 10.1017/S095442241900012X
95. Borys J, Maciejczyk M, Antonowicz B, Krętowski A, Sidun J, Domel E, et al. Glutathione metabolism, mitochondria activity, and nitrosative stress in patients treated for mandible fractures. J Clin Med. (2019) 8. doi: 10.3390/jcm8010127
96. Valko M, Leibfritz D, Moncol J, Cronin MT, Mazur M, Telser J. Free radicals and antioxidants in normal physiological functions and human disease. Int J Biochem Cell Biol. (2007) 39:44–84. doi: 10.1016/j.biocel.2006.07.001
97. Yang Y, Hayden MR, Sowers S, Bagree SV, Sowers JR. Retinal redox stress and remodeling in cardiometabolic syndrome and diabetes. Oxid Med Cell longevity. (2010) 3:392–403. doi: 10.4161/oxim.3.6.14786
98. Chen J, Ding X, Wu R, Tong B, Zhao L, Lv H, et al. Novel sesquiterpene glycoside from loquat leaf alleviates type 2 diabetes mellitus combined with nonalcoholic fatty liver disease by improving insulin resistance, oxidative stress, inflammation, and gut microbiota composition. J Agric Food Chem. (2021) 69:14176–91. doi: 10.1021/acs.jafc.1c05596
99. Moriggi M, Belloli S, Barbacini P, Murtaj V, Torretta E, Chaabane L, et al. Skeletal muscle proteomic profile revealed gender-related metabolic responses in a diet-induced obesity animal model. Int J Mol Sci. (2021) 22. doi: 10.3390/ijms22094680
100. Barone E, Di Domenico F, Perluigi M, Butterfield DA. The interplay among oxidative stress, brain insulin resistance and AMPK dysfunction contribute to neurodegeneration in type 2 diabetes and Alzheimer disease. Free Radical Biol Med. (2021) 176:16–33. doi: 10.1016/j.freeradbiomed.2021.09.006
101. Algvere PV, Marshall J, Seregard S. Age-related maculopathy and the impact of blue light hazard. Acta ophthalmologica Scandinavica. (2006) 84:4–15. doi: 10.1111/j.1600-0420.2005.00627.x
102. Esser N, Legrand-Poels S, Piette J, Scheen AJ, Paquot N. Inflammation as a link between obesity, metabolic syndrome and type 2 diabetes. Diabetes Res Clin practice. (2014) 105:141–50. doi: 10.1016/j.diabres.2014.04.006
103. Syauqy A, Hsu CY, Rau HH, Chao JC. Association of dietary patterns, anthropometric measurements, and metabolic parameters with C-reactive protein and neutrophil-to-lymphocyte ratio in middle-aged and older adults with metabolic syndrome in Taiwan: a cross-sectional study. Nutr J. (2018) 17:106. doi: 10.1186/s12937-018-0417-z
104. Stepanczuk C, Williams N, Morrison K, Kemmerer C. Factors influencing patients' receptiveness to evidence-based recommendations during the clinical encounter. J Comp effectiveness Res. (2017) 6:347–61. doi: 10.2217/cer-2016-0077
105. Donath MY, Shoelson SE. Type 2 diabetes as an inflammatory disease. Nat Rev Immunol. (2011) 11:98–107. doi: 10.1038/nri2925
106. Boucher J, Kleinridders A, Kahn CR. Insulin receptor signaling in normal and insulin-resistant states. Cold Spring Harbor Perspect Biol. (2014) 6. doi: 10.1101/cshperspect.a009191
107. Cleland SJ, Petrie JR, Small M, Elliott HL, Connell JM. Insulin action is associated with endothelial function in hypertension and type 2 diabetes. Hypertension (Dallas Tex 1979). (2000) 35:507–11. doi: 10.1161/01.HYP.35.1.507
108. Hermann C, Assmus B, Urbich C, Zeiher AM, Dimmeler S. Insulin-mediated stimulation of protein kinase Akt: A potent survival signaling cascade for endothelial cells. Arteriosclerosis thrombosis Vasc Biol. (2000) 20:402–9. doi: 10.1161/01.ATV.20.2.402
109. Kim JA, Montagnani M, Koh KK, Quon MJ. Reciprocal relationships between insulin resistance and endothelial dysfunction: molecular and pathophysiological mechanisms. Circulation. (2006) 113:1888–904. doi: 10.1161/CIRCULATIONAHA.105.563213
110. Abiko T, Abiko A, Clermont AC, Shoelson B, Horio N, Takahashi J, et al. Characterization of retinal leukostasis and hemodynamics in insulin resistance and diabetes: role of oxidants and protein kinase-C activation. Diabetes. (2003) 52:829–37. doi: 10.2337/diabetes.52.3.829
111. Gardner TW, Davila JR. The neurovascular unit and the pathophysiologic basis of diabetic retinopathy. Graefe's Arch Clin Exp Ophthalmol = Albrecht von Graefes Archiv fur klinische und experimentelle Ophthalmologie. (2017) 255:1–6. doi: 10.1007/s00417-016-3548-y
112. Chen WW, Zhang X, Huang WJ. Role of neuroinflammation in neurodegenerative diseases (Review). Mol Med Rep. (2016) 13:3391–6. doi: 10.3892/mmr.2016.4948
113. Soni D, Sagar P, Takkar B. Diabetic retinal neurodegeneration as a form of diabetic retinopathy. Int ophthalmol. (2021) 41:3223–48. doi: 10.1007/s10792-021-01864-4
114. González H, Elgueta D, Montoya A, Pacheco R. Neuroimmune regulation of microglial activity involved in neuroinflammation and neurodegenerative diseases. J neuroimmunol. (2014) 274:1–13. doi: 10.1016/j.jneuroim.2014.07.012
115. Kim YH, Kim YS, Park CH, Chung IY, Yoo JM, Kim JG, et al. Protein kinase C-delta mediates neuronal apoptosis in the retinas of diabetic rats via the Akt signaling pathway. Diabetes. (2008) 57:2181–90. doi: 10.2337/db07-1431
116. Chun YH, Han K, Park SH, Park KM, Yim HW, Lee WC, et al. Insulin resistance is associated with intraocular pressure elevation in a non-obese Korean population. PLoS One. (2015) 10:e112929. doi: 10.1371/journal.pone.0112929
117. Fujiwara K, Yasuda M, Ninomiya T, Hata J, Hashimoto S, Yoshitomi T, et al. Insulin resistance is a risk factor for increased intraocular pressure: the Hisayama study. Invest Ophthalmol Visual science. (2015) 56:7983–7. doi: 10.1167/iovs.15-16766
118. Kellar D, Craft S. Brain insulin resistance in Alzheimer's disease and related disorders: mechanisms and therapeutic approaches. Lancet Neurol. (2020) 19:758–66. doi: 10.1016/S1474-4422(20)30231-3
119. Kellar D, Register T, Lockhart SN, Aisen P, Raman R, Rissman RA, et al. Intranasal insulin modulates cerebrospinal fluid markers of neuroinflammation in mild cognitive impairment and Alzheimer's disease: a randomized trial. Sci Rep. (2022) 12:1346. doi: 10.1038/s41598-022-05165-3
120. Arnold SE, Arvanitakis Z, Macauley-Rambach SL, Koenig AM, Wang HY, Ahima RS, et al. Brain insulin resistance in type 2 diabetes and Alzheimer disease: concepts and conundrums. Nat Rev Neurol. (2018) 14:168–81. doi: 10.1038/nrneurol.2017.185
121. Wallsh JO, Gallemore RP. Anti-VEGF-resistant retinal diseases: A review of the latest treatment options. Cells. (2021) 10. doi: 10.3390/cells10051049
122. Nolan CJ, Ruderman NB, Kahn SE, Pedersen O, Prentki M. Insulin resistance as a physiological defense against metabolic stress: implications for the management of subsets of type 2 diabetes. Diabetes. (2015) 64:673–86. doi: 10.2337/db14-0694
123. Shimizu I, Minamino T, Toko H, Okada S, Ikeda H, Yasuda N, et al. Excessive cardiac insulin signaling exacerbates systolic dysfunction induced by pressure overload in rodents. J Clin Invest. (2010) 120:1506–14. doi: 10.1172/JCI40096
Keywords: insulin resistance, retina, diabetic retinopathy, glaucoma, age related macular degeneration
Citation: Zheng Z and Yu X (2024) Insulin resistance in the retina: possible implications for certain ocular diseases. Front. Endocrinol. 15:1415521. doi: 10.3389/fendo.2024.1415521
Received: 10 April 2024; Accepted: 05 June 2024;
Published: 17 June 2024.
Edited by:
Johnny Di Pierdomenico, University of Murcia, SpainReviewed by:
Maria Norte-Muñoz, University of Murcia, SpainManuel Vidal-Sanz, University of Murcia, Spain
Copyright © 2024 Zheng and Yu. This is an open-access article distributed under the terms of the Creative Commons Attribution License (CC BY). The use, distribution or reproduction in other forums is permitted, provided the original author(s) and the copyright owner(s) are credited and that the original publication in this journal is cited, in accordance with accepted academic practice. No use, distribution or reproduction is permitted which does not comply with these terms.
*Correspondence: Xiaobing Yu, yuxiaobing1214@163.com