- 1Adelaide Medical School and Centre of Research Excellence in Translating Nutritional Science to Good Health, University of Adelaide, Adelaide, SA, Australia
- 2Endocrine and Metabolic Unit, Royal Adelaide Hospital, Adelaide, SA, Australia
It is well established that high-protein diets (i.e. ~25–30% of energy intake from protein) provide benefits for achieving weight loss, and subsequent weight maintenance, in individuals with obesity, and improve glycemic control in type 2 diabetes (T2D). These effects may be attributable to the superior satiating property of protein, at least in part, through stimulation of both gastrointestinal (GI) mechanisms by protein, involving GI hormone release and slowing of gastric emptying, as well as post-absorptive mechanisms facilitated by circulating amino acids. In contrast, there is evidence that the beneficial effects of greater protein intake on body weight and glycemia may only be sustained for 6–12 months. While both suboptimal dietary compliance and metabolic adaptation, as well as substantial limitations in the design of longer-term studies are all likely to contribute to this contradiction, the source of dietary protein (i.e. animal vs. plant) has received inappropriately little attention. This issue has been highlighted by outcomes of recent epidemiological studies indicating that long-term consumption of animal-based protein may have adverse effects in relation to the development of obesity and T2D, while plant-based protein showed either protective or neutral effects. This review examines information relating to the effects of dietary protein on appetite, energy intake and postprandial glycemia, and the relevant GI functions, as reported in acute, intermediate- and long-term studies in humans. We also evaluate knowledge relating to the relevance of the dietary protein source, specifically animal or plant, to the prevention, and management, of obesity and T2D.
1 Introduction
In the last ~20 years, there has been substantial, and increasing, interest in promoting dietary protein intake to improve health outcomes (1–5). We believe that the first official recommendation for daily protein intake, reported in 1936 by the League of Nations (6), was 1.0 g/kg of body weight. This has been subsequently challenged by several joint Food and Agriculture Organization (FAO)/World Health Organization (WHO) expert committees, who made the current recommendation of 0.8 g/kg daily protein intake in healthy adults, accounting for ~10–15% of daily energy intake, in 2007 (7). There is now compelling evidence that high-protein diets, which can entail a protein intake up to 5-fold greater than the recommended daily amount, and are in most cases characterized by ~25–30% of energy intake from protein, facilitate weight loss and attenuate weight (re)gain, in individuals with obesity, and improve glycemic control in type 2 diabetes (T2D), in the intermediate-term, i.e. during 6–12 months’ consumption (8–10). Protein suppresses energy intake (11–16), and reduces postprandial glycemia (17–20). These effects may be attributable to the capacity of protein to stimulate both gastrointestinal (GI) hormones (11–13) and postabsorptive, possibly ‘central’, mechanisms in response to meals (21, 22). Key GI hormones include cholecystokinin (CCK), the so-called ‘incretin’ hormones, glucose-dependent insulinotropic polypeptide (GIP) and glucagon-like peptide 1 (GLP-1), as well as peptide tyrosine-tyrosine (PYY), which are pivotal to the regulation of both energy intake and/or postprandial blood glucose, in some cases, at least in part, through slowing of gastric emptying (23–25) (Figure 1).
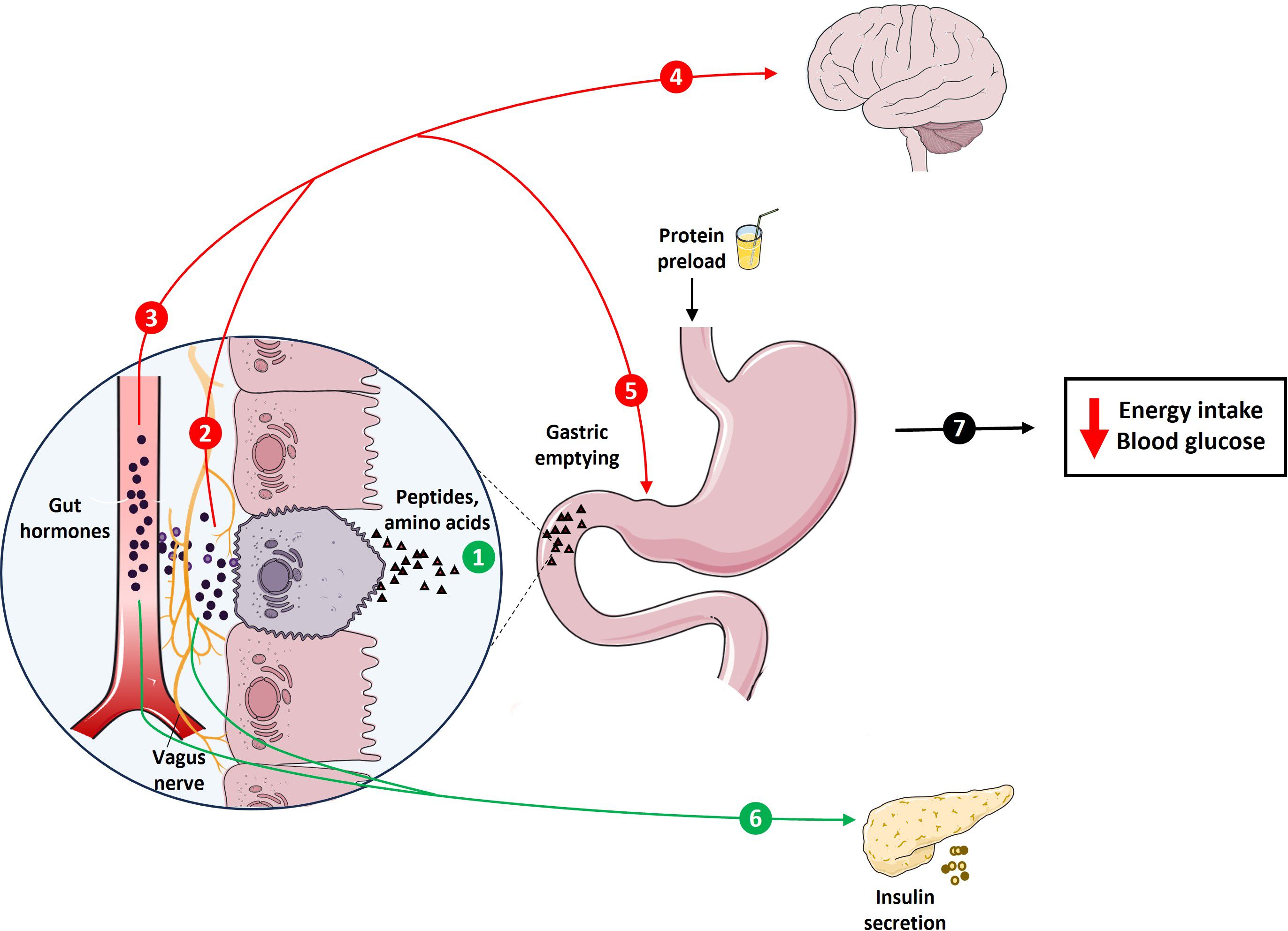
Figure 1 Schematic representation of protein-induced stimulation of gastrointestinal (GI) functions, including GI hormone release and slowing of gastric emptying, which are integral to the regulation of energy intake and glycemia. The presence of protein digestion products, including peptides and amino acids, in the GI lumen stimulates key GI hormones, including CCK, the incretins, GIP and GLP-1, and PYY (1). These hormones exert their effects through various pathways, including activation of hormone-specific receptors on vagal afferent endings (2) or following transport through the bloodstream (3). These inputs, together, are conveyed to higher brain centers to modulate eating behaviors (4), as well as feedback regulation of GI motor functions, particularly stimulation of pyloric pressures, associated with the slowing of gastric emptying (5). GIP and GLP-1, when transported in the bloodstream and/or by activating receptors on vagal afferent endings, also stimulate insulin secretion from pancreas (6). Together, these signals contribute to the effects of protein to reduce energy intake and blood glucose (7).
In contrast to these potent acute/intermediate-term effects of protein, there is evidence that the beneficial effects of greater protein intake on body weight and glycemia may only be sustained for 6–12 months (26–28), which has been attributed to both suboptimal dietary compliance and metabolic adaptation. However, the substantial variations, as well as limitations in the design of longer-term studies, including inconsistencies in the amount and composition of tested foods, and the characteristics of study participants (e.g. age, ethnicity and race) are also likely to be relevant. There are also considerable variations in the source of dietary protein between individuals worldwide (29, 30), which may be derived from animal- and/or plant-based foods. This issue has received less attention despite compelling evidence that animal and plant proteins may have different metabolic effects in the longer-term (31–33). This issue has assumed increasing importance, particularly in view of emerging evidence derived from recent epidemiological studies to indicate an increased risk of T2D with animal, but a protective effect of plant, protein (34–36).
The focus of this review relates to the effects of dietary protein on appetite, energy intake and postprandial glycemia, and the relevant GI functions, including the stimulation of GI hormones and slowing of gastric emptying, as reported in acute, intermediate- and long-term studies in humans. We also evaluate knowledge relating to the relevance of the dietary protein source, specifically animal or plant, to the prevention, and management, of obesity and T2D. While dietary protein is also of importance to other areas, including muscle mass, particularly in elderly and malnourished people, these and other issues are not addressed.
2 Acute effects of protein on appetite, energy intake and postprandial glycemia
A number of studies have shown that acute oral administration of protein preloads, in doses ranging from 20–70 g, dose-dependently reduce hunger, and induce fullness, associated with suppression of energy intake at a subsequent meal, in both healthy lean individuals and those with obesity (11–16, 20, 37). A comprehensive meta-analysis comprising 49 trials, which investigated the acute effects of protein preloads on commonly used markers of appetite, revealed decreases in hunger, desire to eat, prospective food consumption, and an increase in fullness in both lean and obese participants (38). These effects were associated with a reduction in subsequent food intake, when participants were presented with a standardized meal (38). These effects of protein are also often accompanied by reductions in postprandial glycemia. Indeed, a higher protein intake, either as a ‘preload’ before, or as part of, a carbohydrate meal, has been shown to reduce postprandial glycemic excursions, in both lean and obese individuals with and without T2D (10, 13, 17–20, 39–42). Accordingly, the outcomes of these acute studies are consistent, showing that a higher protein intake at a meal has beneficial effects to reduce both energy intake and postprandial glycemia.
The acute appetite- and glucoregulatory effects of protein have been shown to vary between different sources of protein (43–59) (Table 1). For example, when the effects of preloads, containing either milk proteins (whey or casein protein), egg, turkey, tuna, or soy protein, were compared, each suppressed hunger and energy intake, but whey protein had the most profound effects (44, 49, 51, 53, 56). In contrast, a number of studies reported that whey protein was less satiating than some other proteins (46, 47, 55). For example, when the effects of whey protein, pea protein hydrolysate, a combination of whey protein and pea protein hydrolysate, and control milk protein (80% casein and 20% whey) were compared, pea protein hydrolysate was the most effective in suppressing hunger and desire to eat, with no difference in their effects on subsequent energy intake (46). Veldhorst et al. also reported that both alpha-lactalbumin and gelatin are ~40% more satiating than whey protein, inducing a related ~20% reduction in subsequent energy intake (55). However, when compared with casein or soy, whey protein was still more effective in suppressing energy intake (56). Milk proteins have also been found, in some studies, to exert more potent effects to reduce blood glucose than turkey, fish, egg, or pea proteins (52–54, 57). For example, when 45 g of protein, of different sources (either gluten, cod, casein, or whey), was added to a high-fat meal, the postprandial blood glucose response in T2D was less with whey, compared to the other proteins (52). In contrast, in another study in healthy and prediabetic adults, there was no difference in postprandial glycemic excursions between whey and casein, when added to a drink containing maltodextrin (50). Whether variations in the effects of different protein sources to reduce postprandial glycemia are associated with the magnitude of their effect on appetite remains uncertain, with some studies suggesting a strong relationship, particularly for whey (43, 53, 60). The latter is potentially attributable to the rapid digestion of whey protein, due to its high solubility in the acidic environment of the stomach, leading to the stimulation of GI mechanisms more effectively than other proteins (49, 60). However, comprehensive evidence comparing all types of protein sources, particularly different plant-based proteins, is lacking and further investigation is required.
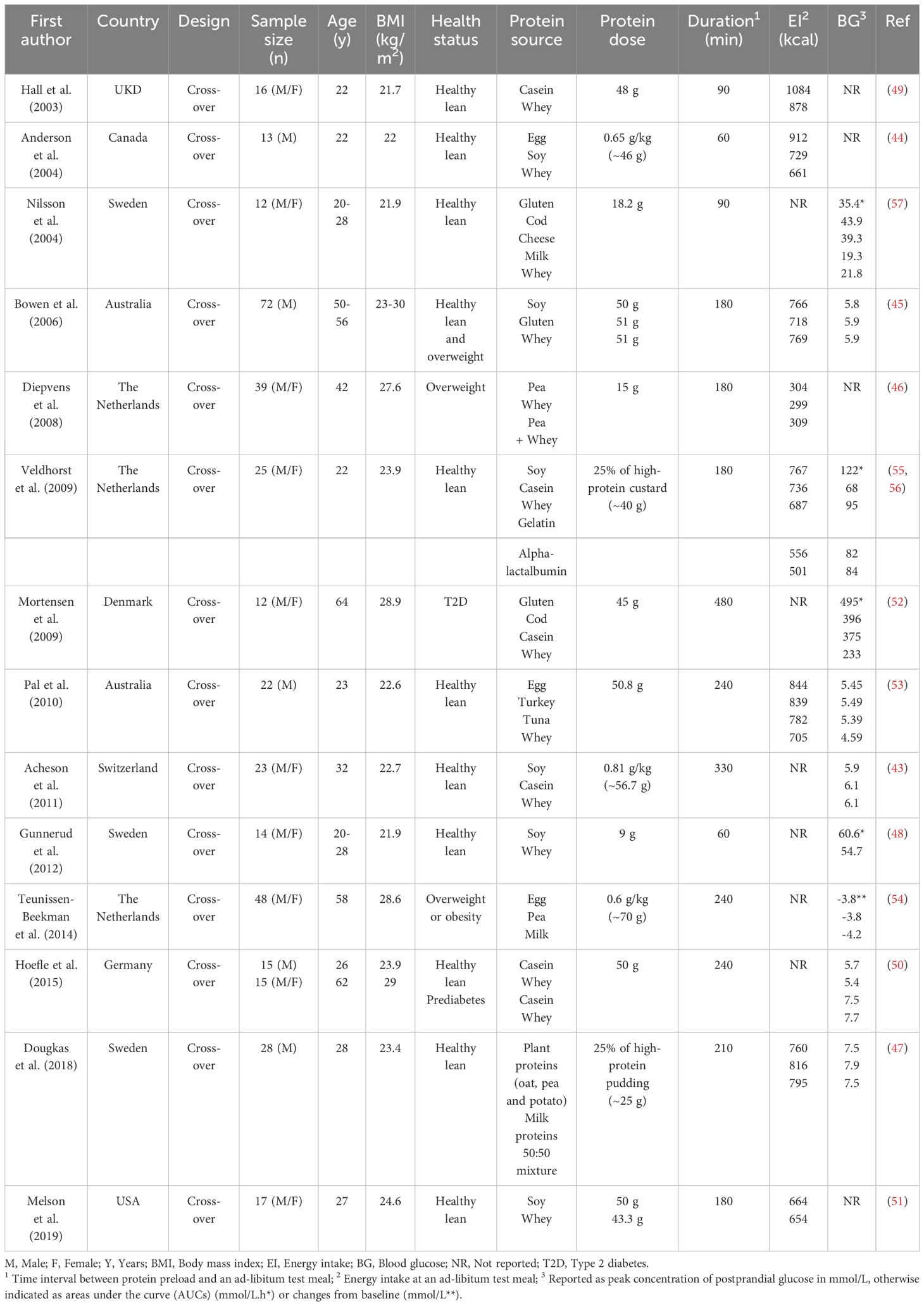
Table 1 Acute effects of different protein preloads (animal vs. plant) on ad-libitum energy intake and postprandial blood glucose levels.
2.1 Mechanisms underlying the effects of protein on energy intake and glycemia
The stimulation of both GI mechanisms, involving GI hormone release and slowing of gastric emptying (23–25), as well as post-absorptive mechanisms facilitated by specific circulating amino acids (21, 22), have been shown to be integral to these effects of protein. Protein, and its digestion products (amino acids), when administered directly into the GI lumen, stimulate key GI hormones, including CCK, the incretins, GIP and GLP-1, and PYY (61–70). In addition to the direct activation of receptors on submucosal vagal afferent and enteric neurons to modulate eating behavior (71), these hormones are transported in the bloodstream to affect peripheral organs, including the stomach, to stimulate pyloric pressures, which are important to the regulation of gastric emptying, and the pancreas, to stimulate insulin secretion (72), overall resulting in a reduction in postprandial glycemia (Figure 1). The rate of gastric emptying plays a key role in determining the postprandial glycemic response, particularly in the first 30–60 min following a meal, accounting for up to 35% of the variance in the initial glycemic response to a meal in healthy participants (73). With progressive impairment in glucose tolerance, this relationship exhibits a ‘shift to the right’, so that the 120-min blood glucose in a 75 g oral glucose tolerance test is inversely related to the rate of gastric emptying in healthy participants, but directly in people with T2D (74). Proteins also stimulate glucagon secretion, which may affect postprandial glycemia adversely (75). Moreover, postprandial glucagon secretion is characteristically exaggerated in individuals with T2D (76).
As alluded to, these acute effects of protein to modulate GI functions are dependent on the type of protein, with evidence that whey protein is more potent than other protein sources, including casein, fish, soy, gluten and pea protein (45, 46, 48, 49, 53, 56, 57). Accordingly, the majority of studies have focused predominantly on whey protein. For example, in healthy men, a 60-min intraduodenal infusion of whey protein, in loads of 0.5, 1.5 and 3 kcal/min, reflecting the physiological range of gastric emptying of ~1–3 kcal/min, has been shown to stimulate plasma CCK and GLP-1 concentrations, and pyloric pressures, all in a dose-dependent manner, associated with suppression of subsequent energy intake in both lean men (61) and those with obesity (62). At the highest load (3 kcal/min), whey protein also reduced blood glucose levels in individuals without T2D (61, 62). Oral preloads of whey protein, in doses of 30 and 70 g, also stimulated plasma CCK, GLP-1, glucagon, and slowed gastric emptying, associated with suppression of energy intake, and improved glycemia, in healthy men (13). In T2D, acute administration of whey protein, ingested as a preload, in a dose of 55 g, 30 min before a mashed potato meal, also stimulated GLP-1, GIP and insulin and slowed gastric emptying, associated with a substantial reduction in peak postprandial glucose of ~3 mmol/L (17). Moreover, these effects were shown to be sustained when whey protein (25 g) was given 30 min before each of three main meals, for 4 weeks (18). Similar acute effects of whey protein were evident when a preload incorporating whey (17 g) together with guar (5 g), a viscous polysaccharide that can itself reduce postprandial glycemic excursions, was given to individuals with T2D or prediabetes (77). 12 weeks’ treatment with this preload, consumed twice daily before breakfast and dinner in individuals with well-controlled T2D, had sustained effects to slow gastric emptying and reduce postprandial blood glucose (78).
There is evidence that the high content of branched-chain amino-acids (BCAAs), particularly leucine and isoleucine, in whey protein, contributes to its efficacy in reducing energy intake and glycemia, through stimulation of GI hormone secretion (57, 79, 80). For example, intraduodenal administration of L-leucine, in a load of 0.45 kcal/min (9.9 g over 90 min), stimulated CCK secretion and suppressed subsequent energy intake (63). Moreover, both L-leucine and L-isoleucine, when administered intragastrically, in a dose of 10 g, 30 min before a mixed-nutrient drink (500 kcal), lowered postprandial blood glucose (67). In contrast, valine was ineffective, potentially reflecting the concurrent stimulation of glucagon (67). L-leucine also stimulated C-peptide, a marker of insulin secretion, and both L-leucine and L-isoleucine slowed gastric emptying of the drink modestly (67). However, these effects of L-leucine and L-isoleucine were not evident in individuals with T2D for uncertain reasons (68). There is also compelling evidence that these effects of amino acids are type-specific, with some, such as L-proline (81), and L‐lysine (82), being less potent, compared to the aromatic amino acids, L-tryptophan (65, 66, 83) and L-phenylalanine (69). In a comparative analysis of the effects of four different amino acids (L-tryptophan, L-phenylalanine, L-leucine and L-glutamine) administered intraduodenally, L-tryptophan and L-leucine were shown to have the most potent effects to reduce energy intake, which was related to greater stimulation of plasma CCK (70). Both L-tryptophan (dose of 3 g) (66) and L-phenylalanine (dose of 10 g) (69), when administered intragastrically before a carbohydrate-containing drink, also lowered the blood glucose response in healthy lean participants (66, 69) and those with obesity (66).
The concept that these amino acids may also mediate the effects of dietary protein, after absorption, via both vagal mechanisms and direct effects on specific brain regions, including the hypothalamus and brainstem (21, 22, 84), was first introduced in 1956, as the so-called ‘aminostatic hypothesis’, which recognized that while amino acids are used primarily for protein synthesis, the amino acids remaining in the circulation might serve as a food intake-regulatory signal (84). BCAAs, particularly L-leucine, were shown to activate the mammalian target of rapamycin complex 1 (mTORC1), to act as a cellular fuel sensor in which hypothalamic activity is tied directly to the regulation of energy intake. In a variety of model systems, mTOR activity has been shown to be highly sensitive to plasma levels of L-leucine (85, 86). There is also emerging preclinical evidence to support a major role for BCAAs, particularly L-leucine and L-isoleucine, in β-cell signaling and metabolism, to acutely stimulate insulin secretion through activation of mTORC1, which is also responsible for increasing β-cell mass and function (87, 88). Elevated plasma concentrations of other amino acids, particularly L-tryptophan, which serves as a precursor for the neurotransmitter serotonin, a key regulator of appetite (89), have also been reported to be associated with reduced energy intake. A lesser number of studies have addressed the role of other amino acids. Both tyrosine and histidine can be converted into anorexigenic neurotransmitters, including dopamine, norepinephrine and histamine, but their contributions to protein-induced food intake suppression remain uncertain (90, 91). Thus, amino acids appear to mediate, at least in part, the effects of protein through distinct physiological pathways. This is likely to be important given that the amino acid composition of different sources of proteins may represent a major factor to account for their diverse metabolic effects in the longer-term.
3 Intermediate-term effects of protein on food intake, body weight and glycemia
The capacity of high-protein diets to induce weight loss has been examined primarily through two approaches; ‘ad-libitum’ diets, in which participants are allowed to consume based on their desire to eat, and ‘energy-restricted’ diets, where the proportion of protein is increased while restricting and then maintaining a constant total energy intake. Irrespective of the type of dietary protocol, in a majority of studies, enriching diets with a relatively high protein content has been shown to facilitate weight loss more than standard-protein diets (~10–15% of energy intake from protein) with intervention durations of up to 6 months (92–96). Ad-libitum high-protein diets, however, have shown more consistent efficacy, while under iso-energetic conditions, strict control of energy intake has invariably been associated with clinically relevant weight loss that compromised assessment of potential metabolic effects of protein. A number of meta-analyses have reported favorable effects of high-protein diets on weight loss (8, 9, 97). For example, a meta-analysis of 24 randomized clinical trials that only compared energy-restricted isocaloric high-protein (27–35% protein) and standard-protein (16–21% protein) diets, with a mean diet duration of 12 weeks, revealed modestly greater reductions in weight (-0.79 kg) and fat mass (-0.87 kg) with a high-protein diet (8). Another meta-analysis of 74 randomized controlled trials, investigating the effects of high-protein diets with or without energy restriction, with a mean duration of 6 months, also found reductions in body weight (-0.36 kg), body mass index (-0.37 kg/m2), and waist circumference (-0.43 cm) in the high-protein (16–45% protein) compared to the standard-protein (5–23% protein) diet group (97).
In contrast to the promising and relatively consistent outcomes of the shorter-term effects (≤6 months duration) of high-protein diets on weight loss in numerous studies, the majority of longer-term studies (at least 12 months in duration), albeit much fewer in number, found no effect of higher protein intake (26–28, 98–102). For example, in a follow-up to an intensive 6-month weight-loss trial, Due et al. reported that, at 12 months, weight loss was no greater in participants assigned to a high-protein diet (30% protein), compared with a medium-protein diet (12% protein) (98). A 2013 meta-analysis, which included 15 trials, in which the intervention period was for a minimum of 12 months, also revealed neither a beneficial, nor detrimental, effect of higher protein intakes on weight loss (28). In contrast, in a 12-month study, McAuley et al. reported modestly improved weight-loss maintenance (-6.6 kg) with a higher-protein diet (30% protein) than either a high-carbohydrate diet (-4.4 kg) or a high-fat diet (-5.5 kg), each containing 15% protein (99). Clifton et al. also found a direct relationship between weight loss and protein intake when comparing high-protein (34% protein) with high-carbohydrate diets (containing 17% protein) for 12 months, however, there was no difference in weight loss effects of the two diets (101). In two trials by Brinkworth et al, one in people with T2D (27), the other in normoglycemic individuals with obesity (26), the effects of a high-protein diet (30% protein) and a standard-protein diet (15% protein), both low in fat, during 8 to 12 weeks of energy restriction and 12 months of energy balance were compared, reporting a net weight loss in both groups, which was slightly greater in the high-protein group (-3.7 to -4.1 kg) compared with the standard-protein group (-2.2 to -2.9 kg). Accordingly, while the majority of evidence indicates that the efficacy of high-protein diets is attenuated in the longer-term, adherence to such diets may still facilitate weight maintenance, for at least up to 12 months (103). In addition, it should be noted that the interpretation of these studies is, in many cases, compromised by poor compliance and high dropout rates, precluding definitive conclusions regarding the long-term effects of high-protein diets on weight loss. However, there is unequivocal evidence that a progressive decrease in adherence is very common with any dietary intervention and, not surprisingly, irrespective of the macronutrient composition, a greater adherence to any energy-restricted diet is associated with a greater weight loss at both one (104) and two years (105). An inherent challenge in longer-term studies is to minimize the impacts of potential cofounders, including the unavoidable lack of blinding, as well as differences in participant characteristics (age, ethnicity and race), which may impact on the GI-induced effects of protein (106, 107).
While there is a lack of definitive evidence regarding the optimal dietary approach for T2D management, in the majority of cases, weight loss represents a primary strategy for improved glycemic control, usually assessed by measurement of glycated hemoglobin (HbA1c). In individuals with prediabetes or newly diagnosed T2D, a modest (5–10%) reduction in body weight improves glycemic control significantly (108). Accordingly, high-protein diets, because of their established weight loss effects, at least in studies of up to 6 months duration, have been advocated as a strategy to improve glycemic control (109). In a recent network meta-analysis of 42 randomized controlled trials, involving 4,809 patients with T2D, comparing the impacts of 10 different dietary approaches on glycemic control, high-protein diets were shown to be among the most effective in reducing both HbA1c and fasting glucose (110). The beneficial effects of high-protein diets to reduce the postprandial blood glucose response, which, in individuals with relatively well-controlled T2D (i.e. baseline HbA1c ≤8.0%), is the major determinant of glycemic control, have been reported in several trials (10, 111–113). However, there are also inconsistent observations, particularly in studies with duration of >6 months (26–28, 114–116). A 2012 meta-analysis, summarizing nine clinical trials with intervention durations between 4 to 24 weeks, revealed a modest, but significant, reduction of 0.52% in HbA1c, but not fasting glucose, in individuals with T2D following a high-protein diet (~25–32% of energy intake) (9). However, no significant effects, on either HbA1c or fasting glucose, were evident in a more recent meta-analysis of 13 trials with intervention durations ranging from 12 weeks to 52 months (116), although, given the large variations in study conditions, this is probably not surprising. Another meta-analysis, which included 15 trials with longer intervention durations (at least 12 months) in individuals with or without T2D, also found no effects on either HbA1c or fasting glucose (28). Therefore, it remains uncertain whether sustained adherence to a high-protein diet improves glycemic control in T2D or prediabetes. It is also not known whether the positive outcomes of shorter-term trials reflect the use of protein per se, the concurrent reduction in weight, or both, particularly since these trials were often based on energy-restricted high-protein diets or incorporated a prior weight-loss period. This issue is, to some extent, semantic given that since 90% of people with T2D are obese in Western countries (117), there is a rationale for high-protein diets as a weight-loss strategy to improve glycemic control. However, whether this represents an effective longer-term approach remains to be established.
A number of studies have investigated the effects of high-protein diets on glycemic variability, which has recently emerged as a target for glycemic control and, potentially, an independent risk factor for the micro- and macrovascular complications of T2D, particularly when glycemic control is ‘reasonable’ (i.e. HbA1c ≤8.0%) (113, 118–122). For example, in 16 well-controlled T2D patients, replacing an isocaloric standard-protein (16% protein) with a high-protein (29% protein), diet, for two separate 48-hour periods, was associated with reductions in indices of glycemic variability by 34 to 45%, supporting the concept that a higher intake of protein should be incorporated in dietary advice for patients with T2D (120). Comparable effects were also observed among 20 insulin-resistant women with obesity, where a high-protein diet was more effective in reducing glycemic variability, compared with a Mediterranean diet, in a 21-day trial (121). Furthermore, in a study by Fabricatore et al., in which 26 participants with obesity and T2D underwent a 3-day continuous glucose monitoring (CGM), a higher protein intake was associated with reduced glycemic variability (122). While these findings are promising, confirmation in longer-term studies is required before recommending changes to clinical practice.
A lesser number of studies have examined the effects of selected animal- and plant-based protein sources on weight and glycemia, again with inconsistent outcomes (123–136) (Table 2). While these studies have focused primarily on weight loss, rather than glycemia, observed glucoregulatory effects of different protein sources were in the majority of these studies found not to differ. For example, when the effects of supplemental whey and soy protein (~56 g/d) were compared to an isoenergetic amount of carbohydrate among free-living overweight and obese participants, slightly, but significantly, greater weight loss was observed with whey (-1.8 kg), compared with soy protein (-0.9 kg), with no differences in their effects on fasting glucose (123). In another study of 48 participants with obesity, the effects of two formulas containing either soy (12 g) and milk proteins (9 g) or only milk protein (22 g), given daily every morning for 20 weeks, were compared, and milk protein (-2.5 kg) had superior effects in inducing weight loss than soy protein (-1.1 kg), and also led to a greater reduction in HbA1c levels (124). Another study reported that milk induced a greater reduction in body weight (-4.43 kg) over a period of 8 weeks compared with calcium-fortified soy milk (-3.46 kg) (126). In contrast, greater weight loss effects were reported after 12 weeks with soy- (-9%), rather than milk-based (-7.9%), meal replacements within an energy-restricted diet (125). Interestingly, reductions in fasting glucose were only evident with the soy-, but not with milk-based, meals in this study (125). Consumption of either 3 soy, or 3 casein, shakes daily as part of a 16-week, energy-restricted diet, in two groups of women with obesity, had comparable effects on weight loss and body composition, as well as fasting insulin, while a greater reduction in fasting glucose was evident in the soy group (127). In another study, no difference was found between the weight-reducing effects of a meat-based (-2.2 kg), and a soy-based (-2.4 kg), diet (~30% of energy from protein), with a significant reduction in fasting glucose observed with both diets (130). Two studies reported that beef and chicken, as the primary sources of protein in an energy-restricted diet, had comparable weight loss effects (128, 129). Abete et al. reported that an energy-restricted diet with a high content of legumes (consumed 4 days per week with 17% protein from energy intake) led to body weight reductions comparable to those achieved with a high-protein diet (30% protein) mainly composed of animal proteins, which was associated with significantly greater reduction in fasting glucose only in legume diet group (131). Altogether, there is, therefore, no compelling evidence that a particular protein source leads to greater weight loss, or improvement in glycemia, than another, indicating that plant-based proteins are likely to be as effective for weight loss as animal-based proteins. Moreover, in some cases, plant-based sources were associated with more potent positive glucoregulatory effects (125, 127, 131). Importantly, it remains uncertain whether the effects of the source of protein are independent of other macro- and micronutrient contents.
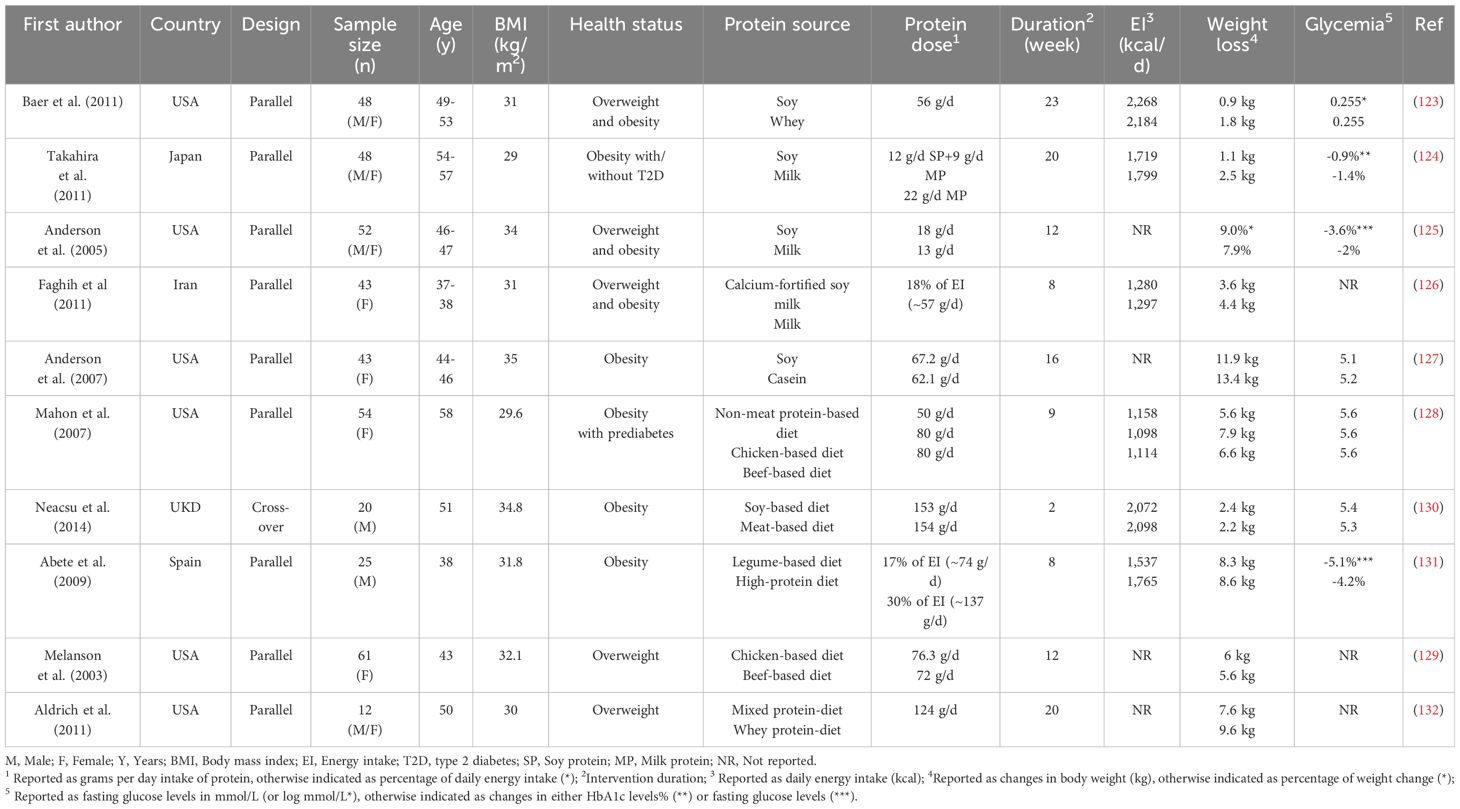
Table 2 Intermediate-term effects of different protein sources (animal vs. plant) on energy intake, body weight and glycemia.
4 Longer-term effects of dietary protein intake in obesity and T2D
While protein has, for many years, represented the cornerstone of dietary approaches for weight management in obesity, associated with improved glycemic control, there is a lack of consensus regarding the maximal amount of dietary protein that can be consumed in the long-term without adverse effects. Interestingly, in contrast to the beneficial acute and intermediate-term effects of protein on weight loss and glycemic control discussed above, outcomes of large prospective studies investigating the association between the long-term consumption of protein with body weight and/or T2D have indicated no overall beneficial effects (137–139). Moreover, there is evidence that the long-term health effects of protein may vary according to the source of protein, thus, long-term consumption of animal-based proteins may have adverse effects in relation to obesity and T2D, while plant-based proteins have either protective or neutral effects (Figure 2).
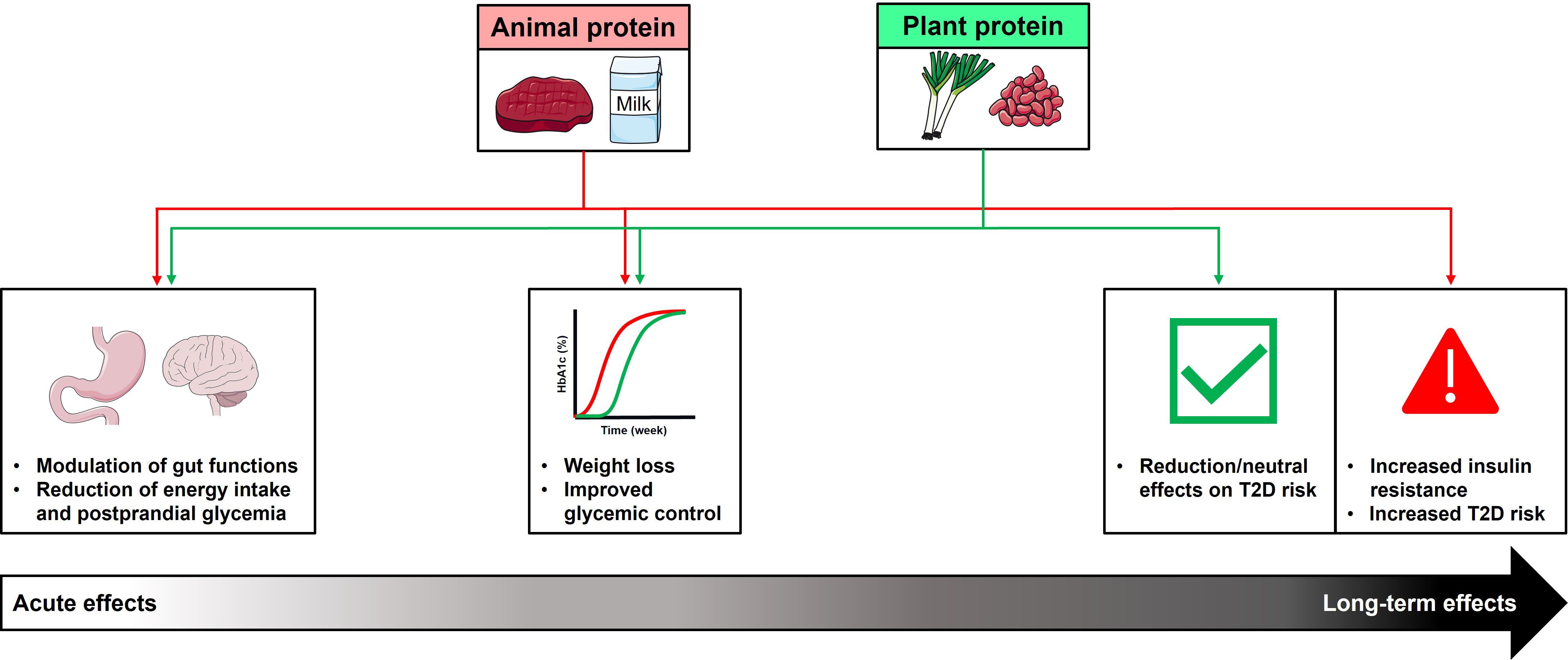
Figure 2 Summary of acute, intermediate-, and longer-term effects of dietary animal and plant protein consumption on metabolic health. Acute intakes of protein (animal or plant-based protein), either in an isolated form as ‘preloads’, or as part of a meal, stimulate GI hormones, associated with reductions in energy intake and postprandial blood glucose. These acute effects are associated with greater weight loss, and improved glycemic control, when consumed as part of a high-protein diet, with comparable outcomes observed with both types of protein. In contrast, the outcomes of longer-term studies suggest that long-term consumption of animal-based protein may have adverse effects in relation to the development of type 2 diabetes (T2D), while plant-based protein have either protective or neutral effects. This may reflect animal protein-specific effects to increase insulin resistance, leading to increased risk of T2D.
Several epidemiological studies, investigating the role of greater protein intake from different sources in the development of obesity in large populations, have consistently reported a direct association between prospective weight gain and higher animal protein intake, and by inference, the risk of obesity (137, 140–142) (Table 3). For example, in the European Prospective Investigation into Cancer and Nutrition (EPIC) study, of 89,432 weight-stable men and women from five countries, overall associations were evident between higher daily intakes of total and animal protein and subsequent weight gain over 6.5 years, which was mainly attributed to protein derived from red and processed meats and chicken, rather than to fish and dairy products (137). In contrast, there were neither protective, nor adverse, associations with plant-based proteins (137). A 2015 analysis, examining the relationships between consumption of different protein sources with long-term weight gain across three separate prospective cohorts of US men and women (the Nurses’ Health Studies (NHS) I and II and the Health Professionals Follow-Up Study (HPFS)) revealed that animal-based protein sources were independently associated with long-term weight gain (i.e. each increased serving/day of red meat, chicken and regular cheese was associated with a 0.13–1.17 kg weight gain), whereas plant-based proteins were independently associated with relative weight loss (i.e. each increased serving/day of peanut butter, walnuts or other nuts was associated with -0.14 to -0.71 kg weight loss) over 4 years (140). Similarly, in a recent analysis of the NHS II study, over a 26-year follow-up, intakes of red meat (both fresh and processed products) and high-fat dairy products, were associated with an increased risk of nonalcoholic fatty liver disease (NAFLD), with obesity found to be the major contributor, while a higher intake of nuts was associated with a reduced risk (143). In another cohort of 1,730 employed men, aged 40 to 55 years from the Chicago Western Electric Study, which were followed from 1958 to 1966, animal protein was positively associated with a 4 times greater risk of obesity, while plant protein reduced the risk by 50% (141). Recent studies have also found that substituting different animal protein sources, particularly processed red meats, with plant protein was associated with reduced risks of coronary heart disease (CHD) and all-cause mortality (144–146).
The majority of studies have also reported that long-term consumption of animal protein increased the risk of T2D, while plant proteins had protective or neutral effects (34–36, 147–154) (Table 4). For example, in two large cohort studies (Women’s Health Initiative and the UK Biobank), with 16,505 incident cases of T2D (out of 143,297 adults without T2D at baseline), during a median follow-up of 15.8 years, replacing consumption of animal protein (5% of energy intake) with plant protein was associated with a 21% lower risk of T2D, attributable to reductions in obesity-related inflammatory factors (36). Moreover, in another study, a higher intake of animal, but not plant, protein was associated with increased risks of both prediabetes and T2D; so that each 5% increment in energy intake from animal protein at the expense of carbohydrate was associated with increased risks of prediabetes of 35% and T2D of 37% (154). This was attributable primarily to increased insulin resistance, as assessed by the indirect homeostatic model (HOMA-IR) (154). A number of recent reviews and meta-analyses have also concluded that higher animal, but not plant, protein intake is associated with an increased risk of T2D (155–160).
The mechanisms through which a high protein intake, from animal vs. plant-based sources, may have differential impacts in the long-term are poorly defined. However, there are several potential explanations, including differences in amino acid composition, glycemic load and potential deleterious effects of the high insulinotropic properties of animal protein, which, in turn, promotes fat storage and impedes fat oxidation (161, 162). Preclinical models also indicate that increased levels of specific amino acids, particularly BCAAs, which are abundant in animal-based proteins, could lead to insulin resistance by activating mTOR, to initiate a detrimental feedback loop toward insulin receptor substrate 1 signaling (163, 164). Indeed, insulin-mediated glucose uptake decreases when body tissues are chronically overexposed to high levels of insulin. Thus, prolonged hyperinsulinemia may lead to insulin resistance and, ultimately, T2D. Elevated postprandial levels of BCAAs have also been shown to inhibit muscle glucose transport and/or glucose phosphorylation directly, to reduce glycogen synthesis, further contributing to insulin resistance (165). Limited human studies also indicate that an increase in protein intake in the longer-term can reduce insulin sensitivity (166, 167). For example, in healthy participants, a higher consumption of protein (~1.87 g/kg of body weight) for six months was associated with greater glucose-stimulated insulin secretion and a modest reduction in insulin sensitivity (166). In another study, in overweight participants, comparing an isoenergetic high-protein diet (~25–30% protein) with a conventional-protein (~15% protein) control diet over 18 weeks, a reduction in insulin sensitivity, as measured by the euglycemic hyper-insulinemic clamp, was observed (167). The differential impacts of animal and plant protein may also be influenced by other dietary nutrients. For example, plant-based foods are rich in dietary fiber, which is known to mitigate T2D risk and may interact additively with plant protein (168). In contrast, a number of dietary components in red and processed meats, as the primary sources of animal protein, such as heme iron, animal fat, and advanced glycation end products, may be, both directly and indirectly, associated with an increased T2D risk. This association may reflect factors including obesity and its related inflammatory markers (leptin and endothelial dysfunction biomarkers) (36, 154, 169).
It is important to also appreciate other potential deleterious effects of high-protein diets, particularly increased risks of osteoporosis and renal diseases (170–174). A potential link with osteoporosis was supported by the observation of increased urinary calcium excretion during a high protein intake (170–172). High-protein diets (>2 g/kg/day) may also increase bone resorption by increasing the acid load in the body, compared with diets of low- to normal-protein content of 0.7-1.0 g/kg/day (172). Indeed, it has been suggested that high consumption of animal-based protein, in particular, leads to an acidification of the blood that may increase carbonate, and subsequently calcium, release from the skeleton to decrease bone mineral density (171). In an epidemiological study of older men (>60 years), a greater dietary acid load due to a chronic high-protein intake was associated with femoral bone loss only under conditions of very low calcium intake <800 mg/d dietary calcium (173). An increased renal acid load, such as the sulfuric acid produced from the oxidation of different amino acids, has also been suggested to increase the risk of kidney stones, and/or increase the glomerular filtration rate, which may lead to renal dysfunction over time (174). While these findings are yet to be confirmed in different populations, they further support that recommendations for a higher protein intake in the long-term should be circumspect.
5 Conclusions and recommendations/priorities for future studies
There is strong evidence from short-term studies (i.e. <6 months in duration) that a higher dietary protein intake facilitates weight loss in obesity and improves glycemic control in T2D. In contrast, the outcomes of longer-term studies, of which there are less, are not convincing, precluding clear-cut recommendations. Suboptimal dietary adherence and metabolic adaptations are likely to contribute to this apparent anomaly, as well as methodological limitations with respect to the type and duration of studies, characteristics of study participants, and how well-controlled the studies are. While acute studies are well-controlled and provide the most reliable findings, these are characteristically performed among a smaller number of participants, who are predominantly young. Accordingly, longer-term studies with larger and more heterogeneous populations are required. An important issue, which has received inappropriately little attention, is the source of dietary protein (i.e. animal vs. plant). The importance of this issue is highlighted by recent epidemiological studies, which strongly support the concept that animal-, but not plant-, based protein intake may have adverse effects in relation to the development of obesity and T2D. Importantly, the longer-term comparative effects of high-protein diets, based on different sources, on body weight and glycemic control remain to be formally evaluated. Despite this limitation, it would be appropriate for current dietary guidelines to consider the source of dietary protein in relation to the use of high-protein diets, and reasonable to advise a reduction in the consumption of animal protein and a relatively increased intake of plant protein. Such a nuanced approach may prove fundamental to longer-term outcomes. Moreover, future studies should focus on the relevance of animal vs. plant-based protein sources, particularly how longer-term consumption of different protein sources may affect GI-related food intake- and gluco-regulatory mechanisms. The outcomes of such studies are likely to lead to more personalized and effective use of protein in the prevention and management of obesity and T2D.
Author contributions
JA-S: Writing – review & editing, Writing – original draft, Conceptualization. CF-B: Writing – review & editing, Conceptualization. MH: Writing – review & editing, Conceptualization.
Funding
The author(s) declare financial support was received for the research, authorship, and/or publication of this article. JA-S was supported by a University of Adelaide Research Scholarship (2021-25).
Acknowledgments
Artwork shown in Figures 1, 2 was adapted from pictures provided by Servier Medical Art, licensed under a Creative Commons Attribution 4.0 Unported License (https://creativecommons.org/licenses/by/4.0/).
Conflict of interest
The authors declare that the research was conducted in the absence of any commercial or financial relationships that could be construed as a potential conflict of interest.
Publisher’s note
All claims expressed in this article are solely those of the authors and do not necessarily represent those of their affiliated organizations, or those of the publisher, the editors and the reviewers. Any product that may be evaluated in this article, or claim that may be made by its manufacturer, is not guaranteed or endorsed by the publisher.
References
1. Leidy HJ, Clifton PM, Astrup A, Wycherley TP, Westerterp-Plantenga MS, Luscombe-Marsh ND, et al. The role of protein in weight loss and maintenance. Am J Clin Nutr. (2015) 101:1320S–9S. doi: 10.3945/ajcn.114.084038
2. Halton TL, Hu FB. The effects of high protein diets on thermogenesis, satiety and weight loss: a critical review. J Am Coll Nutr. (2004) 23:373–85. doi: 10.1080/07315724.2004.10719381
3. Astrup A, Raben A, Geiker N. The role of higher protein diets in weight control and obesity-related comorbidities. Int J Obes (Lond). (2015) 39:721–6. doi: 10.1038/ijo.2014.216
4. Westerterp-Plantenga MS, Nieuwenhuizen A, Tomé D, Soenen S, Westerterp KR. Dietary protein, weight loss, and weight maintenance. Annu Rev Nutr. (2009) 29:21–41. doi: 10.1146/annurev-nutr-080508-141056
5. Te Morenga L, Mann J. The role of high-protein diets in body weight management and health. Br J Nutr. (2012) 108:130–8. doi: 10.1017/S0007114512002437
6. League Of Nations. Report on the physiological bases of nutrition drawn up by the Technical Commission of the Health Committee. Problem Nutr. (1936).
7. Joint WHO/FAO/UNU expert consultation. Protein and amino acid requirements in human nutrition. World Health Organ Tech Rep Ser. (2007) 935:1–265.
8. Wycherley TP, Moran LJ, Clifton PM, Noakes M, Brinkworth GD. Effects of energy-restricted high-protein, low-fat compared with standard-protein, low-fat diets: a meta-analysis of randomized controlled trials. Am J Clin Nutr. (2012) 96:1281–98. doi: 10.3945/ajcn.112.044321
9. Dong J-Y, Zhang Z-L, Wang P-Y, Qin L-Q. Effects of high-protein diets on body weight, glycaemic control, blood lipids and blood pressure in type 2 diabetes: meta-analysis of randomised controlled trials. Br J Nutr. (2013) 110:781–9. doi: 10.1017/S0007114513002055
10. Gannon MC, Nuttall FQ, Saeed A, Jordan K, Hoover H. An increase in dietary protein improves the blood glucose response in persons with type 2 diabetes. Am J Clin Nutr. (2003) 78:734–41. doi: 10.1093/ajcn/78.4.734
11. Bowen J, Noakes M, Trenerry C, Clifton PM. Energy intake, ghrelin, and cholecystokinin after different carbohydrate and protein preloads in overweight men. J Clin Endocrinol Metab. (2006) 91:1477–83. doi: 10.1210/jc.2005-1856
12. Brennan IM, Luscombe-Marsh ND, Seimon RV, Otto B, Horowitz M, Wishart JM, et al. Effects of fat, protein, and carbohydrate and protein load on appetite, plasma cholecystokinin, peptide YY, and ghrelin, and energy intake in lean and obese men. Am J Physiol Gastrointest Liver Physiol. (2012) 303:129–40. doi: 10.1152/ajpgi.00478.2011
13. Hutchison AT, Piscitelli D, Horowitz M, Jones KL, Clifton PM, Standfield S, et al. Acute load-dependent effects of oral whey protein on gastric emptying, gut hormone release, glycemia, appetite, and energy intake in healthy men. Am J Clin Nutr. (2015) 102:1574–84. doi: 10.3945/ajcn.115.117556
14. Akhavan T, Luhovyy BL, Brown PH, Cho CE, Anderson GH. Effect of premeal consumption of whey protein and its hydrolysate on food intake and postmeal glycemia and insulin responses in young adults. Am J Clin Nutr. (2010) 91:966–75. doi: 10.3945/ajcn.2009.28406
15. Astbury NM, Stevenson EJ, Morris P, Taylor MA, Macdonald IA. Dose–response effect of a whey protein preload on within-day energy intake in lean subjects. Br J Nutr. (2010) 104:1858–67. doi: 10.1017/S000711451000293X
16. Bertenshaw EJ, Lluch A, Yeomans MR. Dose-dependent effects of beverage protein content upon short-term intake. Appetite. (2009) 52:580–7. doi: 10.1016/j.appet.2009.01.010
17. Ma J, Stevens JE, Cukier K, Maddox AF, Wishart JM, Jones KL, et al. Effects of a protein preload on gastric emptying, glycemia, and gut hormones after a carbohydrate meal in diet-controlled type 2 diabetes. Diabetes Care. (2009) 32:1600–2. doi: 10.2337/dc09-0723
18. Ma J, Jesudason DR, Stevens JE, Keogh JB, Jones KL, Clifton PM, et al. Sustained effects of a protein 'preload' on glycaemia and gastric emptying over 4 weeks in patients with type 2 diabetes: A randomized clinical trial. Diabetes Res Clin Pract. (2015) 108:31–4. doi: 10.1016/j.diabres.2015.02.019
19. Nuttall FQ, Mooradian AD, Gannon MC, Billington C, Krezowski P. Effect of protein ingestion on the glucose and insulin response to a standardized oral glucose load. Diabetes Care. (1984) 7:465–70. doi: 10.2337/diacare.7.5.465
20. Belza A, Ritz C, Sørensen MQ, Holst JJ, Rehfeld JF, Astrup A. Contribution of gastroenteropancreatic appetite hormones to protein-induced satiety. Am J Clin Nutr. (2013) 97:980–9. doi: 10.3945/ajcn.112.047563
21. Fromentin G, Darcel N, Chaumontet C, Marsset-Baglieri A, Nadkarni N, Tomé D. Peripheral and central mechanisms involved in the control of food intake by dietary amino acids and proteins. Nutr Res Rev. (2012) 25:29–39. doi: 10.1017/S0954422411000175
22. Woods SC, D'Alessio DA. Central control of body weight and appetite. J Clin Endocrinol Metab. (2008) 93:37–50. doi: 10.1210/jc.2008-1630
23. Steinert RE, Feinle-Bisset C, Asarian L, Horowitz M, Beglinger C, Geary N. Ghrelin, CCK, GLP-1, and PYY(3-36): secretory controls and physiological roles in eating and glycemia in health, obesity, and after RYGB. Physiol Rev. (2017) 97:411–63. doi: 10.1152/physrev.00031.2014
24. Camilleri M. Peripheral mechanisms in appetite regulation. Gastroenterology. (2015) 148:1219–33. doi: 10.1053/j.gastro.2014.09.016
25. Marathe CS, Rayner CK, Jones KL, Horowitz M. Relationships between gastric emptying, postprandial glycemia, and incretin hormones. Diabetes Care. (2013) 36:1396–405. doi: 10.2337/dc12-1609
26. Brinkworth G, Noakes M, Keogh J, Luscombe N, Wittert G, Clifton P. Long-term effects of a high-protein, low-carbohydrate diet on weight control and cardiovascular risk markers in obese hyperinsulinemic subjects. Int J Obes Relat Metab Disord. (2004) 28:661–70. doi: 10.1038/sj.ijo.0802617
27. Brinkworth G, Noakes M, Parker B, Foster P, Clifton P. Long-term effects of advice to consume a high-protein, low-fat diet, rather than a conventional weight-loss diet, in obese adults with type 2 diabetes: one-year follow-up of a randomised trial. Diabetologia. (2004) 47:1677–86. doi: 10.1007/s00125-004-1511-7
28. Schwingshackl L, Hoffmann G. Long-term effects of low-fat diets either low or high in protein on cardiovascular and metabolic risk factors: a systematic review and meta-analysis. Nutr J. (2013) 12:48. doi: 10.1186/1475-2891-12-48
29. Pasiakos SM, Agarwal S, Lieberman HR, Fulgoni VL III. Sources and amounts of animal, dairy, and plant protein intake of US adults in 2007–2010. Nutrients. (2015) 7:7058–69. doi: 10.3390/nu7085322
30. Miller V, Reedy J, Cudhea F, Zhang J, Shi P, Erndt-Marino J, et al. Global, regional, and national consumption of animal-source foods between 1990 and 2018: findings from the Global Dietary Database. Lancet Planet Health. (2022) 6:243–56. doi: 10.1016/S2542-5196(21)00352-1
31. Naghshi S, Sadeghi O, Willett WC, Esmaillzadeh A. Dietary intake of total, animal, and plant proteins and risk of all cause, cardiovascular, and cancer mortality: systematic review and dose-response meta-analysis of prospective cohort studies. BMJ. (2020) 370:2412. doi: 10.1136/bmj.m2412
32. Neuenschwander M, Stadelmaier J, Eble J, Grummich K, Szczerba E, Kiesswetter E, et al. Substitution of animal-based with plant-based foods on cardiometabolic health and all-cause mortality: a systematic review and meta-analysis of prospective studies. BMC Med. (2023) 21:404. doi: 10.1186/s12916-023-03093-1
33. Ferrari L, Panaite S-A, Bertazzo A, Visioli F. Animal- and plant-based protein sources: a scoping review of human health outcomes and environmental impact. Nutrients. (2022) 14:5115. doi: 10.3390/nu14235115
34. Malik VS, Li Y, Tobias DK, Pan A, Hu FB. Dietary protein intake and risk of type 2 diabetes in us men and women. Am J Epidemiol. (2016) 183:715–28. doi: 10.1093/aje/kwv268
35. Ericson U, Sonestedt E, Gullberg B, Hellstrand S, Hindy G, Wirfält E, et al. High intakes of protein and processed meat associate with increased incidence of type 2 diabetes. Br J Nutr. (2013) 109:1143–53. doi: 10.1017/S0007114512003017
36. Li J, Glenn AJ, Yang Q, Ding D, Zheng L, Bao W, et al. Dietary protein sources, mediating biomarkers, and incidence of type 2 diabetes: findings from the Women's Health Initiative and the UK Biobank. Diabetes Care. (2022) 45:1742–53. doi: 10.2337/dc22-0368
37. Burton-Freeman BM. Glycomacropeptide (GMP) is not critical to whey-induced satiety, but may have a unique role in energy intake regulation through cholecystokinin (CCK). Physiol Behav. (2008) 93:379–87. doi: 10.1016/j.physbeh.2007.09.010
38. Kohanmoo A, Faghih S, Akhlaghi M. Effect of short- and long-term protein consumption on appetite and appetite-regulating gastrointestinal hormones, a systematic review and meta-analysis of randomized controlled trials. Physiol Behav. (2020) 226:113123. doi: 10.1016/j.physbeh.2020.113123
39. Smedegaard S, Kampmann U, Ovesen PG, Støvring H, Rittig N. Whey protein premeal lowers postprandial glucose concentrations in adults compared with water - the effect of timing, dose, and metabolic status: a systematic review and meta-analysis. Am J Clin Nutr. (2023) 118:391–405. doi: 10.1016/j.ajcnut.2023.05.012
40. Gheldof N, Francey C, Rytz A, Egli L, Delodder F, Bovetto L, et al. Effect of different nutritional supplements on glucose response of complete meals in two crossover studies. Nutrients. (2022) 14:2674. doi: 10.3390/nu14132674
41. Gordon RA, Zumbro EL, Castleberry TJ, Sokoloski ML, Brisebois MF, Irvine CJ, et al. Whey protein improves glycemia during an oral glucose tolerance test compared to vigorous-intensity aerobic exercise in young adult men. BMC Sports Sci Med Rehabil. (2022) 14:147. doi: 10.1186/s13102-022-00540-z
42. Smith HA, Watkins JD, Walhin JP, Gonzalez JT, Thompson D, Betts JA. Whey protein-enriched and carbohydrate-rich breakfasts attenuate insulinemic responses to an ad libitum lunch relative to extended morning fasting: a randomized crossover trial. J Nutr. (2023) 153:2842–53. doi: 10.1016/j.tjnut.2023.08.008
43. Acheson KJ, Blondel-Lubrano A, Oguey-Araymon S, Beaumont M, Emady-Azar S, Ammon-Zufferey C, et al. Protein choices targeting thermogenesis and metabolism. Am J Clin Nutr. (2011) 93:525–34. doi: 10.3945/ajcn.110.005850
44. Anderson GH, Tecimer SN, Shah D, Zafar TA. Protein source, quantity, and time of consumption determine the effect of proteins on short-term food intake in young men. J Nutr. (2004) 134:3011–5. doi: 10.1093/jn/134.11.3011
45. Bowen J, Noakes M, Clifton PM. Appetite regulatory hormone responses to various dietary proteins differ by body mass index status despite similar reductions in ad libitum energy intake. J Clin Endocrinol Metab. (2006) 91:2913–9. doi: 10.1210/jc.2006-0609
46. Diepvens K, Häberer D, Westerterp-Plantenga M. Different proteins and biopeptides differently affect satiety and anorexigenic/orexigenic hormones in healthy humans. Int J Obes (Lond). (2008) 32:510–8. doi: 10.1038/sj.ijo.0803758
47. Dougkas A, Östman E. Comparable effects of breakfast meals varying in protein source on appetite and subsequent energy intake in healthy males. Eur J Nutr. (2018) 57:1097–108. doi: 10.1007/s00394-017-1392-4
48. Gunnerud UJ, Heinzle C, Holst JJ, Östman EM, Björck IM. Effects of pre-meal drinks with protein and amino acids on glycemic and metabolic responses at a subsequent composite meal. PloS One. (2012) 7:44731. doi: 10.1371/journal.pone.0044731
49. Hall W, Millward D, Long S, Morgan L. Casein and whey exert different effects on plasma amino acid profiles, gastrointestinal hormone secretion and appetite. Br J Nutr. (2003) 89:239–48. doi: 10.1079/BJN2002760
50. Hoefle AS, Bangert AM, Stamfort A, Gedrich K, Rist MJ, Lee Y-M, et al. Metabolic responses of healthy or prediabetic adults to bovine whey protein and sodium caseinate do not differ. J Nutr. (2015) 145:467–75. doi: 10.3945/jn.114.199190
51. Melson CE, Nepocatych S, Madzima TA. The effects of whey and soy liquid breakfast on appetite response, energy metabolism, and subsequent energy intake. Nutrition. (2019) 61:179–86. doi: 10.1016/j.nut.2018.11.007
52. Mortensen LS, Hartvigsen ML, Brader LJ, Astrup A, Schrezenmeir J, Holst JJ, et al. Differential effects of protein quality on postprandial lipemia in response to a fat-rich meal in type 2 diabetes: comparison of whey, casein, gluten, and cod protein. Am J Clin Nutr. (2009) 90:41–8. doi: 10.3945/ajcn.2008.27281
53. Pal S, Ellis V. The acute effects of four protein meals on insulin, glucose, appetite and energy intake in lean men. Br J Nutr. (2010) 104:1241–8. doi: 10.1017/S0007114510001911
54. Teunissen-Beekman KF, Dopheide J, Geleijnse JM, Bakker SJ, Brink EJ, de Leeuw PW, et al. Differential effects of proteins and carbohydrates on postprandial blood pressure-related responses. Br J Nutr. (2014) 112:600–8. doi: 10.1017/S0007114514001251
55. Veldhorst MA, Nieuwenhuizen AG, Hochstenbach-Waelen A, Westerterp KR, Engelen MP, Brummer R-JM, et al. A breakfast with alpha-lactalbumin, gelatin, or gelatin+ TRP lowers energy intake at lunch compared with a breakfast with casein, soy, whey, or whey-GMP. Clin Nutr. (2009) 28:147–55. doi: 10.1016/j.clnu.2008.12.003
56. Veldhorst MAB, Nieuwenhuizen AG, Hochstenbach-Waelen A, van Vught AJAH, Westerterp KR, Engelen MPKJ, et al. Dose-dependent satiating effect of whey relative to casein or soy. Physiol Behav. (2009) 96:675–82. doi: 10.1016/j.physbeh.2009.01.004
57. Nilsson M, Stenberg M, Frid AH, Holst JJ, Björck IME. Glycemia and insulinemia in healthy subjects after lactose-equivalent meals of milk and other food proteins: the role of plasma amino acids and incretins. Am J Clin Nutr. (2004) 80:1246–53. doi: 10.1093/ajcn/80.5.1246
58. Pham T, Knowles S, Bermingham E, Brown J, Hannaford R, Cameron-Smith D, et al. Plasma amino acid appearance and status of appetite following a single meal of red meat or a plant-based meat analog: a randomized crossover clinical trial. Curr Dev Nutr. (2022) 6:nzac082. doi: 10.1093/cdn/nzac082
59. Sambashivaiah S, Cope M, Mukherjea R, Selvam S, George N, Kuriyan R, et al. The Effect of soy and whey protein supplementation on glucose homeostasis in healthy normal weight Asian Indians. J Nutr Metab. (2023) 2023:2622057. doi: 10.1155/2023/2622057
60. Boirie Y, Dangin M, Gachon P, Vasson MP, Maubois JL, Beaufrère B. Slow and fast dietary proteins differently modulate postprandial protein accretion. Proc Natl Acad Sci U.S.A. (1997) 94:14930–5. doi: 10.1073/pnas.94.26.14930
61. Ryan AT, Feinle-Bisset C, Kallas A, Wishart JM, Clifton PM, Horowitz M, et al. Intraduodenal protein modulates antropyloroduodenal motility, hormone release, glycemia, appetite, and energy intake in lean men. Am J Clin Nutr. (2012) 96:474–82. doi: 10.3945/ajcn.112.038133
62. Hutchison AT, Feinle-Bisset C, Fitzgerald PC, Standfield S, Horowitz M, Clifton PM, et al. Comparative effects of intraduodenal whey protein hydrolysate on antropyloroduodenal motility, gut hormones, glycemia, appetite, and energy intake in lean and obese men. Am J Clin Nutr. (2015) 102:1323–31. doi: 10.3945/ajcn.115.114538
63. Steinert RE, Landrock MF, Ullrich SS, Standfield S, Otto B, Horowitz M, et al. Effects of intraduodenal infusion of the branched-chain amino acid leucine on ad libitum eating, gut motor and hormone functions, and glycemia in healthy men. Am J Clin Nutr. (2015) 102:820–7. doi: 10.3945/ajcn.115.114488
64. Steinert RE, Luscombe-Marsh ND, Little TJ, Standfield S, Otto B, Horowitz M, et al. Effects of intraduodenal infusion of l-tryptophan on ad libitum eating, antropyloroduodenal motility, glycemia, insulinemia, and gut peptide secretion in healthy men. J Clin Endocrinol Metab. (2014) 99:3275–84. doi: 10.1210/jc.2014-1943
65. Hajishafiee M, Ullrich SS, Fitzgerald PC, Horowitz M, Lange K, Poppitt SD, et al. Suppression of energy intake by intragastric l-tryptophan in lean and obese men: relations with appetite perceptions and circulating cholecystokinin and tryptophan. J Nutr. (2021) 151:2932–41. doi: 10.1093/jn/nxab218
66. Ullrich SS, Fitzgerald PCE, Giesbertz P, Steinert RE, Horowitz M, Feinle-Bisset C. Effects of intragastric administration of tryptophan on the blood glucose response to a nutrient drink and energy intake, in lean and obese men. Nutrients. (2018) 10:463. doi: 10.3390/nu10040463
67. Elovaris RA, Bitarafan V, Agah S, Ullrich SS, Lange K, Horowitz M, et al. Comparative effects of the branched-chain amino acids, leucine, isoleucine and valine, on gastric emptying, plasma glucose, c-peptide and glucagon in healthy men. Nutrients. (2021) 13:1613. doi: 10.3390/nu13051613
68. Elovaris RA, Hajishafiee M, Ullrich SS, Fitzgerald PCE, Lange K, Horowitz M, et al. Intragastric administration of leucine and isoleucine does not reduce the glycaemic response to, or slow gastric emptying of, a carbohydrate-containing drink in type 2 diabetes. Diabetes Res Clin Pract. (2021) 171:108618. doi: 10.1016/j.diabres.2020.108618
69. Fitzgerald PCE, Manoliu B, Herbillon B, Steinert RE, Horowitz M, Feinle-Bisset C. Effects of L-phenylalanine on energy intake and glycaemia-impacts on appetite perceptions, gastrointestinal hormones and gastric emptying in healthy males. Nutrients. (2020) 12:1788. doi: 10.3390/nu12061788
70. Steinert RE, Ullrich SS, Geary N, Asarian L, Bueter M, Horowitz M, et al. Comparative effects of intraduodenal amino acid infusions on food intake and gut hormone release in healthy males. Physiol Rep. (2017) 5:13492. doi: 10.14814/phy2.13492
71. de Lartigue G, Diepenbroek C. Novel developments in vagal afferent nutrient sensing and its role in energy homeostasis. Curr Opin Pharmacol. (2016) 31:38–43. doi: 10.1016/j.coph.2016.08.007
72. Furness JB, Rivera LR, Cho H-J, Bravo DM, Callaghan B. The gut as a sensory organ. Nat Rev Gastroenterol Hepatol. (2013) 10:729–40. doi: 10.1038/nrgastro.2013.180
73. Horowitz M, Edelbroek M, Wishart J, Straathof J. Relationship between oral glucose tolerance and gastric emptying in normal healthy subjects. Diabetologia. (1993) 36:857–62. doi: 10.1007/BF00400362
74. Jalleh RJ, Wu T, Jones KL, Rayner CK, Horowitz M, Marathe CS. Relationships of glucose, GLP-1, and insulin secretion with gastric emptying after a 75-g glucose load in type 2 diabetes. J Clin Endocrinol Metab. (2022) 107:3850–6. doi: 10.1210/clinem/dgac330
75. Young A. Inhibition of glucagon secretion. Adv Pharmacol. (2005) 52:151–71. doi: 10.1016/S1054-3589(05)52008-8
76. Ahmed M, Nuttall FQ, Gannon MC, Lamusga RF. Plasma glucagon and alpha-amino acid nitrogen response to various diets in normal humans. Am J Clin Nutr. (1980) 33:1917–24. doi: 10.1093/ajcn/33.9.1917
77. Watson LE, Phillips LK, Wu T, Bound MJ, Checklin H, Grivell J, et al. Differentiating the effects of whey protein and guar gum preloads on postprandial glycemia in type 2 diabetes. Clin Nutr. (2019) 38:2827–32. doi: 10.1016/j.clnu.2018
78. Watson LE, Phillips LK, Wu T, Bound MJ, Checklin HL, Grivell J, et al. A whey/guar "preload" improves postprandial glycaemia and glycated haemoglobin levels in type 2 diabetes: A 12-week, single-blind, randomized, placebo-controlled trial. Diabetes Obes Metab. (2019) 21:930–8. doi: 10.1111/dom.13604
79. Elovaris RA, Hutchison AT, Lange K, Horowitz M, Feinle-Bisset C, Luscombe-Marsh ND. Plasma free amino acid responses to whey protein and their relationships with gastric emptying, blood glucose- and appetite-regulatory hormones and energy intake in lean healthy men. Nutrients. (2019) 11:2465. doi: 10.3390/nu11102465
80. Luscombe-Marsh ND, Hutchison AT, Soenen S, Steinert RE, Clifton PM, Horowitz M, et al. Plasma free amino acid responses to intraduodenal whey protein, and relationships with insulin, glucagon-like peptide-1 and energy intake in lean healthy men. Nutrients. (2016) 8:4. doi: 10.3390/nu8010004
81. Nuttall FQ, Gannon MC, Jordan K. The metabolic response to ingestion of proline with and without glucose. Metabolism. (2004) 53:241–6. doi: 10.1016/j.metabol.2003.09.013
82. Kalogeropoulou D, LaFave L, Schweim K, Gannon MC, Nuttall FQ. Lysine ingestion markedly attenuates the glucose response to ingested glucose without a change in insulin response. Am J Clin Nutr. (2009) 90:314–20. doi: 10.3945/ajcn.2008.27381
83. Anjom-Shoae J, Fitzgerald PCE, Horowitz M, Mohammadpour Z, Gv H, JJ H, et al. Intraduodenal calcium enhances the effects of L-tryptophan to stimulate gut hormone secretion and suppress energy intake in healthy males: a randomized, cross-over, clinical trial. Am J Clin Nutr. (2024) S0002-9165:00602–6. doi: 10.1016/j.ajcnut.2024.07.006
84. Mellinkoff SM, Frankland M, Boyle D, Greipel M. Relationship between serum amino acid concentration and fluctuations in appetite. 1956. Obes Res. (1997) 5:381–4. doi: 10.1002/j.1550-8528.1997.tb00568.x
85. Takahara T, Amemiya Y, Sugiyama R, Maki M, Shibata H. Amino acid-dependent control of mTORC1 signaling: a variety of regulatory modes. J BioMed Sci. (2020) 27:87. doi: 10.1186/s12929-020-00679-2
86. Meijer AJ, Dubbelhuis PF. Amino acid signalling and the integration of metabolism. Biochem Biophys Res Commun. (2004) 313:397–403. doi: 10.1016/j.bbrc.2003.07.012
87. Blandino-Rosano M, Chen AY, Scheys JO, Alejandro EU, Gould AP, Taranukha T, et al. mTORC1 signaling and regulation of pancreatic β-cell mass. Cell Cycle. (2012) 11:1892–902. doi: 10.4161/cc.20036
88. Yoon MS. The emerging role of branched-chain amino acids in insulin resistance and metabolism. Nutrients. (2016) 8:405. doi: 10.3390/nu8070405
89. Berger M, Gray JA, Roth BL. The expanded biology of serotonin. Annu Rev Med. (2009) 60:355–66. doi: 10.1146/annurev.med.60.042307.110802
90. He W, Wu G. Metabolism of amino acids in the brain and their roles in regulating food intake. Adv Exp Med Biol. (2020) 1265:167–85. doi: 10.1007/978-3-030-45328-2_10
91. Wellman PJ. Modulation of eating by central catecholamine systems. Curr Drug Targets. (2005) 6:191–9. doi: 10.2174/1389450053174532
92. Skov A, Toubro S, Rønn B, Holm L, Astrup A. Randomized trial on protein vs carbohydrate in ad libitum fat reduced diet for the treatment of obesity. Int J Obes Relat Metab Disord. (1999) 23:528–36. doi: 10.1038/sj.ijo.0800867
93. McAuley K, Hopkins C, Smith K, McLay R, Williams S, Taylor R, et al. Comparison of high-fat and high-protein diets with a high-carbohydrate diet in insulin-resistant obese women. Diabetologia. (2005) 48:8–16. doi: 10.1007/s00125-004-1603-4
94. Claessens M, Van Baak M, Monsheimer S, Saris W. The effect of a low-fat, high-protein or high-carbohydrate ad libitum diet on weight loss maintenance and metabolic risk factors. Int J Obes (Lond). (2009) 33:296–304. doi: 10.1038/ijo.2008.278
95. Farnsworth E, Luscombe ND, Noakes M, Wittert G, Argyiou E, Clifton PM. Effect of a high-protein, energy-restricted diet on body composition, glycemic control, and lipid concentrations in overweight and obese hyperinsulinemic men and women. Am J Clin Nutr. (2003) 78:31–9. doi: 10.1093/ajcn/78.1.31
96. Layman DK, Boileau RA, Erickson DJ, Painter JE, Shiue H, Sather C, et al. A reduced ratio of dietary carbohydrate to protein improves body composition and blood lipid profiles during weight loss in adult women. J Nutr. (2003) 133:411–7. doi: 10.1093/jn/133.2.411
97. Santesso N, Akl EA, Bianchi M, Mente A, Mustafa R, Heels-Ansdell D, et al. Effects of higher- versus lower-protein diets on health outcomes: a systematic review and meta-analysis. Eur J Clin Nutr. (2012) 66:780–8. doi: 10.1038/ejcn.2012.37
98. Due A, Toubro S, Skov A, Astrup A. Effect of normal-fat diets, either medium or high in protein, on body weight in overweight subjects: a randomised 1-year trial. Int J Obes Relat Metab Disord. (2004) 28:1283–90. doi: 10.1038/sj.ijo.0802767
99. McAuley K, Smith K, Taylor R, McLay R, Williams S, Mann J. Long-term effects of popular dietary approaches on weight loss and features of insulin resistance. Int J Obes (Lond). (2006) 30:342–9. doi: 10.1038/sj.ijo.0803075
100. Clifton PM, Condo D, Keogh JB. Long term weight maintenance after advice to consume low carbohydrate, higher protein diets – a systematic review and meta analysis. Nutr Metab Cardiovasc Dis. (2014) 24:224–35. doi: 10.1016/j.numecd.2013.11.006
101. Clifton PM, Keogh JB, Noakes M. Long-term effects of a high-protein weight-loss diet. Am J Clin Nutr. (2008) 87:23–9. doi: 10.1093/ajcn/87.1.23
102. Layman DK, Evans EM, Erickson D, Seyler J, Weber J, Bagshaw D, et al. A moderate-protein diet produces sustained weight loss and long-term changes in body composition and blood lipids in obese adults. J Nutr. (2009) 139:514–21. doi: 10.3945/jn.108.099440
103. Magkos F. Protein-rich diets for weight loss maintenance. Curr Obes Rep. (2020) 9:213–8. doi: 10.1007/s13679-020-00391-0
104. Dansinger ML, Gleason JA, Griffith JL, Selker HP, Schaefer EJ. Comparison of the Atkins, Ornish, Weight Watchers, and Zone diets for weight loss and heart disease risk reduction: a randomized trial. JAMA. (2005) 293:43–53. doi: 10.1001/jama.293.1.43
105. Sacks FM, Bray GA, Carey VJ, Smith SR, Ryan DH, Anton SD, et al. Comparison of weight-loss diets with different compositions of fat, protein, and carbohydrates. N Engl J Med. (2009) 360:859–73. doi: 10.1056/NEJMoa0804748
106. Ran A, Ellen W, Ad AMM, Hauke S, Erwin GZ, Daisy J. Age-dependent changes in GI physiology and microbiota: time to reconsider? Gut. (2018) 67:2213–22. doi: 10.1136/gutjnl-2017-315542
107. Walther B, Lett AM, Bordoni A, Tomás-Cobos L, Nieto JA, Dupont D, et al. GutSelf: Interindividual variability in the processing of dietary compounds by the human gastrointestinal tract. Mol Nutr Food Res. (2019) 63:1900677. doi: 10.1002/mnfr.201900677
108. Wilding J. The importance of weight management in type 2 diabetes mellitus. Int J Clin Pract. (2014) 68:682–91. doi: 10.1111/ijcp.12384
109. Abete I, Astrup A, Martínez JA, Thorsdottir I, Zulet MA. Obesity and the metabolic syndrome: role of different dietary macronutrient distribution patterns and specific nutritional components on weight loss and maintenance. Nutr Rev. (2010) 68:214–31. doi: 10.1111/nure.2010.68.issue-4
110. Jing T, Zhang S, Bai M, Chen Z, Gao S, Li S, et al. Effect of dietary approaches on glycemic control in patients with type 2 diabetes: a systematic review with network meta-analysis of randomized trials. Nutrients. (2023) 15:3156. doi: 10.3390/nu15143156
111. Samkani A, Skytte MJ, Kandel D, Kjaer S, Astrup A, Deacon CF, et al. A carbohydrate-reduced high-protein diet acutely decreases postprandial and diurnal glucose excursions in type 2 diabetes patients. Br J Nutr. (2018) 119:910–17. doi: 10.1017/S0007114518000521
112. Jakubowicz D, Wainstein J, Landau Z, Ahren B, Barnea M, Bar-Dayan Y, et al. High-energy breakfast based on whey protein reduces body weight, postprandial glycemia and HbA1C in type 2 diabetes. J Nutr Biochem. (2017) 49:1–7. doi: 10.1016/j.jnutbio.2017.07.005
113. Skytte MJ, Samkani A, Astrup A, Frystyk J, Rehfeld JF, Holst JJ, et al. Effects of carbohydrate restriction on postprandial glucose metabolism, β-cell function, gut hormone secretion, and satiety in patients with type 2 diabetes. Am J Physiol Endocrinol Metab. (2021) 320:7–18. doi: 10.1152/ajpendo.00165.2020
114. Krebs JD, Elley CR, Parry-Strong A, Lunt H, Drury PL, Bell DA, et al. The Diabetes Excess Weight Loss (DEWL) Trial: a randomised controlled trial of high-protein versus high-carbohydrate diets over 2 years in type 2 diabetes. Diabetologia. (2012) 55:905–14. doi: 10.1007/s00125-012-2461-0
115. Parker B, Noakes M, Luscombe N, Clifton P. Effect of a high-protein, high–monounsaturated fat weight loss diet on glycemic control and lipid levels in type 2 diabetes. Diabetes Care. (2002) 25:425–30. doi: 10.2337/diacare.25.3.425
116. Yu Z, Nan F, Wang LY, Jiang H, Chen W, Jiang Y. Effects of high-protein diet on glycemic control, insulin resistance and blood pressure in type 2 diabetes: A systematic review and meta-analysis of randomized controlled trials. Clin Nutr. (2020) 39:1724–34. doi: 10.1016/j.clnu.2019.08.008
117. Bramante CT, Lee CJ, Gudzune KA. Treatment of obesity in patients with diabetes. Diabetes Spectr. (2017) 30:237–43. doi: 10.2337/ds17-0030
118. Monnier L, Lapinski H, Colette C. Contributions of fasting and postprandial plasma glucose increments to the overall diurnal hyperglycemia of type 2 diabetic patients: variations with increasing levels of HbA(1c). Diabetes Care. (2003) 26:881–5. doi: 10.2337/diacare.26.3.881
119. Tay J, Luscombe-Marsh ND, Thompson CH, Noakes M, Buckley JD, Wittert GA, et al. Comparison of low-and high-carbohydrate diets for type 2 diabetes management: a randomized trial. Am J Clin Nutr. (2015) 102:780–90. doi: 10.3945/ajcn.115.112581
120. Thomsen MN, Skytte MJ, Astrup A, Deacon CF, Holst JJ, Madsbad S, et al. The clinical effects of a carbohydrate-reduced high-protein diet on glycaemic variability in metformin-treated patients with type 2 diabetes mellitus: a randomised controlled study. Clin Nutr ESPEN. (2020) 39:46–52. doi: 10.1016/j.clnesp.2020.07.002
121. Tettamanzi F, Bagnardi V, Louca P, Nogal A, Monti GS, Mambrini SP, et al. A high protein diet is more effective in improving insulin resistance and glycemic variability compared to a mediterranean diet - a cross-over controlled inpatient dietary study. Nutrients. (2021) 13:4380. doi: 10.3390/nu13124380
122. Fabricatore AN, Ebbeling CB, Wadden TA, Ludwig DS. Continuous glucose monitoring to assess the ecologic validity of dietary glycemic index and glycemic load. Am J Clin Nutr. (2011) 94:1519–24. doi: 10.3945/ajcn.111.020354
123. Baer DJ, Stote KS, Paul DR, Harris GK, Rumpler WV, Clevidence BA. Whey protein but not soy protein supplementation alters body weight and composition in free-living overweight and obese adults. J Nutr. (2011) 141:1489–94. doi: 10.3945/jn.111.139840
124. Takahira M, Noda K, Fukushima M, Zhang B, Mitsutake R, Uehara Y, et al. Randomized, double-blind, controlled, comparative trial of formula food containing soy protein vs. milk protein in visceral fat obesity - FLAVO study. Circ J. (2011) 75:2235–43. doi: 10.1253/circj.cj-10-1013
125. Anderson JW, Hoie LH. Weight loss and lipid changes with low-energy diets: comparator study of milk-based versus soy-based liquid meal replacement interventions. J Am Coll Nutr. (2005) 24:210–6. doi: 10.1080/07315724.2005.10719467
126. Faghih S, Abadi AR, Hedayati M, Kimiagar SM. Comparison of the effects of cows' milk, fortified soy milk, and calcium supplement on weight and fat loss in premenopausal overweight and obese women. Nutr Metab Cardiovasc Dis. (2011) 21:499–503. doi: 10.1016/j.numecd.2009.11.013
127. Anderson JW, Fuller J, Patterson K, Blair R, Tabor A. Soy compared to casein meal replacement shakes with energy-restricted diets for obese women: randomized controlled trial. Metabolism. (2007) 56:280–8. doi: 10.1016/j.metabol.2006.10.013
128. Mahon AK, Flynn MG, Stewart LK, McFarlin BK, Iglay HB, Mattes RD, et al. Protein intake during energy restriction: effects on body composition and markers of metabolic and cardiovascular health in postmenopausal women. J Am Coll Nutr. (2007) 26:182–9. doi: 10.1080/07315724.2007.10719600
129. Melanson K, Gootman J, Myrdal A, Kline G, Rippe JM. Weight loss and total lipid profile changes in overweight women consuming beef or chicken as the primary protein source. Nutrition. (2003) 19:409–14. doi: 10.1016/s0899-9007(02)01080-8
130. Neacsu M, Fyfe C, Horgan G, Johnstone AM. Appetite control and biomarkers of satiety with vegetarian (soy) and meat-based high-protein diets for weight loss in obese men: a randomized crossover trial. Am J Clin Nutr. (2014) 100:548–58. doi: 10.3945/ajcn.113.077503
131. Abete I, Parra D, Martinez JA. Legume-, fish-, or high-protein-based hypocaloric diets: effects on weight loss and mitochondrial oxidation in obese men. J Med Food. (2009) 12:100–8. doi: 10.1089/jmf.2007.0700
132. Aldrich ND, Reicks MM, Sibley SD, Redmon JB, Thomas W, Raatz SK. Varying protein source and quantity do not significantly improve weight loss, fat loss, or satiety in reduced energy diets among midlife adults. Nutr Res. (2011) 31:104–12. doi: 10.1016/j.nutres.2011.01.004
133. Li J, Armstrong CLH, Campbell WW. Effects of dietary protein source and quantity during weight loss on appetite, energy expenditure, and cardio-metabolic responses. Nutrients. (2016) 8:63. doi: 10.3390/nu8020063
134. Teixeira FJ, Matias CN, Faleiro J, Giro R, Pires J, Figueiredo H, et al. A novel plant-based protein has similar effects compared to whey protein on body composition, strength, power, and aerobic performance in professional and semi-professional futsal players. Front Nutr. (2022) 9:934438. doi: 10.3389/fnut.2022.934438
135. Vasei MH, Hosseinpour-Niazi S, Ainy E, Mirmiran P. Effect of dietary approaches to stop hypertension (DASH) diet, high in animal or plant protein on cardiometabolic risk factors in obese metabolic syndrome patients: a randomized clinical trial. Prim Care Diabetes. (2022) 16:634–9. doi: 10.1016/j.pcd.2022.09.001
136. Toh DWK, Fu AS, Mehta KA, Lam NYL, Haldar S, Henry CJ. Plant-based meat analogs and their effects on cardiometabolic health: an 8-week randomized controlled trial comparing plant-based meat analogs with their corresponding animal-based foods. Am J Clin Nutr. (2024) 119:1405–16. doi: 10.1016/j.ajcnut.2024.04.006
137. Halkjær J, Olsen A, Overvad K, Jakobsen MU, Boeing H, Buijsse B, et al. Intake of total, animal and plant protein and subsequent changes in weight or waist circumference in European men and women: the Diogenes project. Int J Obes (Lond). (2011) 35:1104–13. doi: 10.1038/ijo.2010.254
138. Alhazmi A, Stojanovski E, McEvoy M, Garg ML. Macronutrient intake and type 2 diabetes risk in middle-aged Australian women. Results from the Australian Longitudinal Study on Women's Health. Public Health Nutr. (2014) 17:1587–94. doi: 10.1017/S1368980013001870
139. Mirmiran P, Bahadoran Z, Gaeini Z, Azizi F. Habitual intake of dietary L-arginine in relation to risk of type 2 diabetes: a prospective study. BMC Endocr Disord. (2021) 21:113. doi: 10.1186/s12902-021-00774-x
140. Smith JD, Hou T, Ludwig DS, Rimm EB, Willett W, Hu FB, et al. Changes in intake of protein foods, carbohydrate amount and quality, and long-term weight change: results from 3 prospective cohorts. Am J Clin Nutr. (2015) 101:1216–24. doi: 10.3945/ajcn.114.100867
141. Bujnowski D, Xun P, Daviglus ML, Van Horn L, He K, Stamler J. Longitudinal association between animal and vegetable protein intake and obesity among men in the United States: the Chicago Western Electric Study. J Am Diet Assoc. (2011) 111:1150–5. doi: 10.1016/j.jada.2011.05.002
142. Lin Y, Bolca S, Vandevijvere S, De Vriese S, Mouratidou T, De Neve M, et al. Plant and animal protein intake and its association with overweight and obesity among the Belgian population. Br J Nutr. (2011) 105:1106–16. doi: 10.1017/S0007114510004642
143. Kim MN, Lo C-H, Corey KE, Luo X, Long L, Zhang X, et al. Red meat consumption, obesity, and the risk of nonalcoholic fatty liver disease among women: Evidence from mediation analysis. Clin Nutr. (2022) 41:356–64. doi: 10.1016/j.clnu.2021.12.014
144. Bajracharya R, Katzke V, Mukama T, Kaaks R. Effect of iso-caloric substitution of animal protein for other macro nutrients on risk of overall, cardiovascular and cancer mortality: prospective evaluation in EPIC-Heidelberg cohort and systematic review. Nutrients. (2023) 15:794. doi: 10.3390/nu15030794
145. Das A, Cumming R, Naganathan V, Blyth F, Couteur DGL, Handelsman DJ, et al. Associations between dietary intake of total protein and sources of protein (plant vs. animal) and risk of all-cause and cause-specific mortality in older Australian men: The Concord Health and Ageing in Men Project. J Hum Nutr Diet. (2022) 35:845–60. doi: 10.1111/jhn.12965
146. Hidayat K, Chen J-S, Wang H-P, Wang T-C, Liu Y-J, Zhang X-Y, et al. Is replacing red meat with other protein sources associated with lower risks of coronary heart disease and all-cause mortality? a meta-analysis of prospective studies. Nutr Rev. (2022) 80:1959–73. doi: 10.1093/nutrit/nuac017
147. Sluijs I, Beulens JWJ, van der A DL, Spijkerman AMW, Grobbee DE, van der Schouw YT. Dietary intake of total, animal, and vegetable protein and risk of type 2 diabetes in the European Prospective Investigation into Cancer and Nutrition (EPIC)-NL study. Diabetes Care. (2009) 33:43–8. doi: 10.2337/dc09-1321
148. Zhou C, Liu C, Zhang Z, Liu M, Zhang Y, Li H, et al. Variety and quantity of dietary protein intake from different sources and risk of new-onset diabetes: a Nationwide Cohort Study in China. BMC Med. (2022) 20:6. doi: 10.1186/s12916-021-02199-8
149. Shang X, Scott D, Hodge AM, English DR, Giles GG, Ebeling PR, et al. Dietary protein intake and risk of type 2 diabetes: results from the Melbourne Collaborative Cohort Study and a meta-analysis of prospective studies. Am J Clin Nutr. (2016) 104:1352–65. doi: 10.3945/ajcn.116.140954
150. Song Y, Manson JE, Buring JE, Liu S. A prospective study of red meat consumption and type 2 diabetes in middle-aged and elderly women: The Women’s Health Study. Diabetes Care. (2004) 27:2108–15. doi: 10.2337/diacare.27.9.2108
151. Sugihiro T, Yoneda M, Ohno H, Oki K, Hattori N. Associations of nutrient intakes with obesity and diabetes mellitus in the longitudinal medical surveys of Japanese Americans. J Diabetes Investig. (2019) 10:1229–36. doi: 10.1111/jdi.13010
152. van Nielen M, Feskens EJ, Mensink M, Sluijs I, Molina E, Amiano P, et al. Dietary protein intake and incidence of type 2 diabetes in Europe: the EPIC-InterAct Case-Cohort Study. Diabetes Care. (2014) 37:1854–62. doi: 10.2337/dc13-2627
153. Yuan S, Ming-wei L, Qi-qiang H, Larsson SC. Egg, cholesterol and protein intake and incident type 2 diabetes mellitus: Results of repeated measurements from a prospective cohort study. Clin Nutr. (2021) 40:4180–6. doi: 10.1016/j.clnu.2021.01.041
154. Chen Z, Franco OH, Lamballais S, Ikram MA, Schoufour JD, Muka T, et al. Associations of specific dietary protein with longitudinal insulin resistance, prediabetes and type 2 diabetes: The Rotterdam Study. Clin Nutr. (2020) 39:242–9. doi: 10.1016/j.clnu.2019.01.021
155. Fan M, Li Y, Wang C, Mao Z, Zhou W, Zhang L, et al. Dietary protein consumption and the risk of type 2 diabetes: adose-response meta-analysis of prospective studies. Nutrients. (2019) 11:2783. doi: 10.3390/nu11112783
156. Zhao LG, Zhang QL, Liu XL, Wu H, Zheng JL, Xiang YB. Dietary protein intake and risk of type 2 diabetes: a dose-response meta-analysis of prospective studies. Eur J Nutr. (2019) 58:1351–67. doi: 10.1007/s00394-018-1737-7
157. Ye J, Yu Q, Mai W, Liang P, Liu X, Wang Y. Dietary protein intake and subsequent risk of type 2 diabetes: a dose-response meta-analysis of prospective cohort studies. Acta Diabetol. (2019) 56:851–70. doi: 10.1007/s00592-019-01320-x
158. Schulze MB, Haardt J, Amini AM, Kalotai N, Lehmann A, Schmidt A, et al. Protein intake and type 2 diabetes mellitus: an umbrella review of systematic reviews for the evidence-based guideline for protein intake of the German Nutrition Society. Eur J Nutr. (2024) 63:33–50. doi: 10.1007/s00394-023-03234-5
159. Fotouhi Ardakani A, Anjom-Shoae J, Sadeghi O, Marathe CS, Feinle-Bisset C, Horowitz M. Association between total, animal, and plant protein intake and type 2 diabetes risk in adults: a systematic review and dose-response meta-analysis of prospective cohort studies. Clin Nutr. (2024) 43:1941–55. doi: 10.1016/j.clnu.2024.07.001
160. Lamberg-Allardt C, Bärebring L, Arnesen EK, Nwaru BI, Thorisdottir B, Ramel A, et al. Animal versus plant-based protein and risk of cardiovascular disease and type 2 diabetes: a systematic review of randomized controlled trials and prospective cohort studies. Food Nutr Res. (2023) 67 (In press). doi: 10.29219/fnr.v67.9003
161. Jackman MR, Steig A, Higgins JA, Johnson GC, Fleming-Elder BK, Bessesen DH, et al. Weight regain after sustained weight reduction is accompanied by suppressed oxidation of dietary fat and adipocyte hyperplasia. Am J Physiol Regul Integr Comp Physiol. (2008) 294:1117–29. doi: 10.1152/ajpregu.00808.2007
162. Van Baak MA, Mariman EC. Mechanisms of weight regain after weight loss - the role of adipose tissue. Nat Rev Endocrinol. (2019) 15:274–87. doi: 10.1038/s41574-018-0148-4
163. Tremblay F, Marette A. Amino acid and insulin signaling via the mTOR/p70 S6 kinase pathway. A negative feedback mechanism leading to insulin resistance in skeletal muscle cells. J Biol Chem. (2001) 276:38052–60. doi: 10.1074/jbc.M106703200
164. Khamzina L, Veilleux A, Bergeron S, Marette A. Increased activation of the mammalian target of rapamycin pathway in liver and skeletal muscle of obese rats: possible involvement in obesity-linked insulin resistance. Endocrinology. (2005) 146:1473–81. doi: 10.1210/en.2004-0921
165. Krebs M, Krssak M, Bernroider E, Anderwald C, Brehm A, Meyerspeer M, et al. Mechanism of amino acid-induced skeletal muscle insulin resistance in humans. Diabetes. (2002) 51:599–605. doi: 10.2337/diabetes.51.3.599
166. Linn T, Santosa B, Grönemeyer D, Aygen S, Scholz N, Busch M, et al. Effect of long-term dietary protein intake on glucose metabolism in humans. Diabetologia. (2000) 43:1257–65. doi: 10.1007/s001250051521
167. Weickert MO, Roden M, Isken F, Hoffmann D, Nowotny P, Osterhoff M, et al. Effects of supplemented isoenergetic diets differing in cereal fiber and protein content on insulin sensitivity in overweight humans. Am J Clin Nutr. (2011) 94:459–71. doi: 10.3945/ajcn.110.004374
168. Barnard ND, Katcher HI, Jenkins DJ, Cohen J, Turner-McGrievy G. Vegetarian and vegan diets in type 2 diabetes management. Nutr Rev. (2009) 67:255–63. doi: 10.1111/nure.2009.67.issue-5
169. Pan A, Sun Q, Bernstein AM, Schulze MB, Manson JE, Willett WC, et al. Red meat consumption and risk of type 2 diabetes: 3 cohorts of US adults and an updated meta-analysis. Am J Clin Nutr. (2011) 94:1088–96. doi: 10.3945/ajcn.111.018978
170. Arjmandi BH, Khalil DA, Smith BJ, Lucas EA, Juma S, Payton ME, et al. Soy protein has a greater effect on bone in postmenopausal women not on hormone replacement therapy, as evidenced by reducing bone resorption and urinary calcium excretion. J Clin Endocrinol Metab. (2003) 88:1048–54. doi: 10.1210/jc.2002-020849
171. Kerstetter JE, O'Brien KO, Caseria DM, Wall DE, Insogna KL. The impact of dietary protein on calcium absorption and kinetic measures of bone turnover in women. J Clin Endocrinol Metab. (2005) 90:26–31. doi: 10.1210/jc.2004-0179
172. Kerstetter JE, O'Brien KO, Insogna KL. Dietary protein, calcium metabolism, and skeletal homeostasis revisited. Am J Clin Nutr. (2003) 78:584–92. doi: 10.1093/ajcn/78.3.584S
173. Mangano KM, Walsh SJ, Kenny AM, Insogna KL, Kerstetter JE. Dietary acid load is associated with lower bone mineral density in men with low intake of dietary calcium. J Bone Miner Res. (2014) 29:500–6. doi: 10.1002/jbmr.2053
Keywords: animal protein, appetite, food intake, gastrointestinal function, glycemic control, obesity, plant protein, type 2 diabetes
Citation: Anjom-Shoae J, Feinle-Bisset C and Horowitz M (2024) Impacts of dietary animal and plant protein on weight and glycemic control in health, obesity and type 2 diabetes: friend or foe? Front. Endocrinol. 15:1412182. doi: 10.3389/fendo.2024.1412182
Received: 04 April 2024; Accepted: 17 July 2024;
Published: 31 July 2024.
Edited by:
Hubert Vaudry, Université de Rouen, FranceReviewed by:
Theodore Ciaraldi, University of California, San Diego, United StatesGiovana Punaro, Federal University of São Paulo, Brazil
Copyright © 2024 Anjom-Shoae, Feinle-Bisset and Horowitz. This is an open-access article distributed under the terms of the Creative Commons Attribution License (CC BY). The use, distribution or reproduction in other forums is permitted, provided the original author(s) and the copyright owner(s) are credited and that the original publication in this journal is cited, in accordance with accepted academic practice. No use, distribution or reproduction is permitted which does not comply with these terms.
*Correspondence: Michael Horowitz, bWljaGFlbC5ob3Jvd2l0ekBhZGVsYWlkZS5lZHUuYXU=