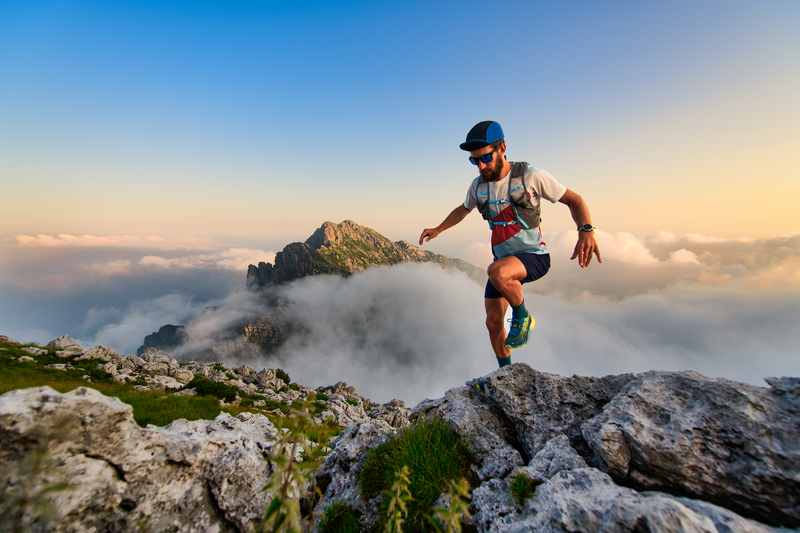
95% of researchers rate our articles as excellent or good
Learn more about the work of our research integrity team to safeguard the quality of each article we publish.
Find out more
REVIEW article
Front. Endocrinol. , 30 May 2024
Sec. Cancer Endocrinology
Volume 15 - 2024 | https://doi.org/10.3389/fendo.2024.1396192
This article is part of the Research Topic New Insights Into Prostate Cancer: New Biomarkers, Molecular Mechanisms, and Therapeutic Approaches View all 12 articles
Prostate cancer is the second most commonly diagnosed cancer in men. The mammalian insulin-like growth factor (IGF) family is made up of three ligands (IGF-I, IGF-II, and insulin), three receptors (IGF-I receptor (IGF-1R), insulin receptor (IR), and IGF-II receptor (IGF-2R)), and six IGF-binding proteins (IGFBPs). IGF-I and IGF-II were identified as potent mitogens and were previously associated with an increased risk of cancer development including prostate cancer. Several reports showed controversy about the expression of the IGF family and their connection to prostate cancer risk due to the high degree of heterogeneity among prostate tumors, sampling bias, and evaluation techniques. Despite that, it is clear that several IGF family members play a role in prostate cancer development, metastasis, and androgen-independent progression. In this review, we aim to expand our understanding of prostate tumorigenesis and regulation through the IGF system. Further understanding of the role of IGF signaling in PCa shows promise and needs to be considered in the context of a comprehensive treatment strategy.
Prostate cancer (PCa) is the fifth leading cause of cancer-related mortality in men worldwide, as well as being the second most commonly diagnosed solid-organ cancer, after lung cancer, in men (1, 2). PCa happens at a rate of 11.3 per 100,000 in developing countries and 37.5 per 100,000 in industrialized countries (3). Similarly, mortality rates in developed and developing nations are 8.1 and 5.9 per 100,000, respectively. According to current estimates, approximately 10 million males are presently diagnosed with PCa, with approximately 400,000 deaths per year, and this figure is expected to rise to over 800,000 by 2040 (3, 4). Although PCa is generally diagnosed at an early stage, the risk-benefit ratio of the treatment remains uncertain. It still represents a global challenge because of the significant morbidity from the current form of therapy and the long disease history and uncertainty in individual patients’ clinical progress (5–7). Approximately 5% of men diagnosed with PCa are diagnosed with distant metastases (often in multiple sites), and 15% are diagnosed with locoregional metastases (8). Such cases have a poor overall survival rate of only 30% for five years (8).
The mammalian insulin-like growth factor (IGF) family is made up of three ligands (IGF-I, IGF-II, and insulin) and three receptors (IGF-I receptor (IGF-1R), insulin receptor (IR), and IGF-II receptor (IGF-2R)) (9). IGF-1R and IR are receptor tyrosine kinases (RTKs) that are structurally similar hetero-tetramers. IR has two alternatively spliced isoforms, IRA and IRB, whose functions are currently unknown. IGF-II, which binds to IRA and IGF-1R and is a more potent mitogen than IGF-I, has recently been demonstrated to control IR isoforms rather than insulin-binding affinities (10). IRB governs metabolic processes in adults, whereas IRA controls prenatal growth and development and mediates the mitogenic effects of insulin. A family of six IGF-binding proteins (IGFBPs) tightly controls the amounts of IGF-I and IGF-II as well as their bioavailability in the circulation and cells (11). IGFBPs are distinct from ligands and receptors and have a greater affinity (pM) for IGFs than their corresponding receptors (nM) (10). IGF-I and IGF-II are produced by a variety of cells, including the liver and muscles, among others. They are secreted constitutively as opposed to being retained in the cells of origin, where they serve as paracrine/autocrine factors (12).
In mammalian cells, the production of IGF-I is mainly induced by growth hormones and transcriptional factors. Then it was shown that IGF-II (67 amino acids) has comparable growth-promoting properties but growth hormone does not regulate its expression (13–15). Additionally, IGF-I and IGF-II are quite similar to insulin in terms of amino acid sequence (16). The three disulfide connections that are shared by these three peptides, allow them to preserve the proper peptide shape. IGFs differ structurally from insulin as they are composed of single chains with a connecting domain (C domain) between the N-terminal B chain and the C-terminal A chain. They result from the elimination of the N- and C-terminal signal peptides from pre-pro-IGF peptides during post-translational processing. The chaperone GRP94 aids in the folding and maturation of the IGF peptides (17, 18).
Studies using knock-out and transgenic mice have revealed additional details about the biological actions of mammalian IGFs. Igf1-null mice had a high postnatal mortality rate and were 30% smaller and lighter than wild-type mice (19). The postnatal growth retardation was also present in those who survived, and it was particularly noticeable during both growth stages (pubertal and post-pubertal). Their bones grew more slowly, and their organs were proportionately smaller. Igf-1r knockout also caused a serious growth deficit and was embryonically fatal in mice (19).
Despite the findings that unraveled the potential role of IGF-I and IGF-II in development, it is still reported that the expression of both IGF-I and IGF-II is tissue-dependent and time-dependent (20). On the other hand, the role of IGF-II in physiology and disease has been the subject of far fewer investigations than that of IGF-I. However, like IGF-I, most tissues in adults produce IGF-II, with the liver producing the majority of the circulating levels. Notably, IGF-II levels in adults are roughly three times higher than IGF-I. Despite this, IGF-II is thought to play crucial roles in fetal growth and development, and it is abundant in the fetal skeletal muscle (12, 21). The stimulation of the IRA to promote stem cell self-renewal is another unique action of IGF-II. Moreover, the expansion of neural progenitor cells and neural stem cell maintenance is supported by IGF-II/IRA signaling (22).
It was evident that the preponderance of circulatory IGFs was much larger than the concentration of peptides in the bloodstream because IGFs were not bound to binding proteins (23). In humans and other mammals, six highly similar high-affinity IGF-binding proteins (IGFBP-1 to IGFBP-6) were identified (24). IGFBPs are pluripotent and used in a variety of metabolic processes. All IGFBPs have conserved three subdomains, including high-affinity IGF-binding terminal domains. Contrarily, one other unstructured domain (known as the central linker domain), is thought to be responsible for the various functions that are unique for each IGFBP (25). Furthermore, it has been reported that the specific function of IGFBP is affected by many post-translational modifications (such as proteolytic cleavage, glycosylation, and phosphorylation). Although this division may not be rigorous, it is possible to roughly divide the functions of IGFBPs into those that rely on their ability to bind and control the activity of IGFs and those that appear to be independent of direct IGF binding (26, 27).
Due to the higher affinity of IGFBPs for IGF-I and IGF-II, the availability of free IGFs decreases and this inhibits them from binding and activation of their receptors. IGFBPs have two key functions that are both critical to the metabolism of IGFs (28). IGFs are produced and swiftly secreted via the constitutive secretory route, as tissues do not contain any intracellular storage of IGFs despite their widespread distribution throughout the body. As soon as they are secreted, IGFs bind to high-affinity IGFBPs, creating binary complexes of about 30–40 kDa. IGFs bound to two IGFBPs, IGFBP-3 and IGFBP-5, can join with the acid-labile subunit (ALS), a third glycoprotein, to form a ternary complex that is about 150 kDa in size (29). Without the ability to store them in tissues, the body can build up enormous IGF reservoirs due to IGFBPs. In humans, the total amount of IGFs in circulation is about 100 nM, with 80–90% of that amount being found in the ternary complex with IGFBP-3 (30). The second important function of IGFBPs is the creation of new pathways for giving IGFs specificity. IGFs are made in most tissues and can regulate the bulk of cell processes. IGFBPs are synthesized in numerous tissues, at different times, in various amounts, and in several combinations to add some specificity to the IGF activity (12).
Furthermore, at the cellular level, IGFBPs can boost IGF activity in several different ways. This can happen by altering the kinetics of IGF ligand/receptor interactions and preventing receptor downregulation, or by changing the interactions between IGFBPs and ECM or cell surfaces thus localizing and increasing the IGF concentrations near to cell receptors (31).
Both IGF-1R and IR are synthesized as polypeptide precursors, which are then modified post-translationally, where α and β subunits are formed upon the cleavage of the precursor molecule. The heterotetramer receptor is made up of two α and two β subunits that are joined together by disulfide bridges. 627 amino acids make up the IGF-1R -subunit, 196 of which are found in the extracellular domain. The intracellular and extracellular domains are joined by a brief transmembrane domain (TM). The juxtamembrane domain (JM), enzymatic tyrosine kinase (TK) domain, and C-terminal domain are the three subdomains of the β-subunit’s intracellular domain. Positions 976 to 981 are occupied by the TK ATP-binding motif (GXGXXG), while position 1003 contains a catalytic lysine that is essential for Mg-ATP binding. The activation loop of the TK domain contains a trio of tyrosines at positions 1131, 1135, and 1136 that are crucial for receptor autophosphorylation. The JM region contains an NPEY motif that, after being phosphorylated, serves as a docking site for Shc and the insulin receptor substrates (IRS), whose recruitment signifies the start of the downstream signaling process. The internalization of receptors, which controls signaling, depends on the NPEY motif (32, 33).
Generally, the IGF-1R, which is expressed on most cells, plays specialized roles in well-differentiated cells such as neurons as well as being involved in cellular proliferation and anti-apoptosis during growth and development. The IGF-pluripotent IR’s roles, however, are still being defined; for instance, a novel function for the IGF-IR in viral entry into cells has just been identified (34).
Several tissue-growth-related transcription factors, including androgen and estrogen receptors, high-mobility group A1 (HMBA1), Krüppel-like factor 6 (KLF6), eukaryotic translation initiation factor 2 (E2F1), and c-Jun, upregulate IGF-IR expression (12, 35). In contrast, several tumor-suppressor genes, including p53, WT1, and BRCA1 downregulate it (36–38).
The binding of IGF-I, IGF-II, or insulin ligand to the IGF-1R initiates a series of events. The binding of IGFs to their receptors activates both MAPK and PI3K-AKT pathways, which results in downstream cellular effects (such as cellular proliferation, anti-apoptosis, and differentiation actions) through other downstream molecules (39). Furthermore, IGF-1R signaling could be mediated through several proteins such as JNK, Jak1, Jak2, focal adhesion kinase, and TIMP2 (40). Moreover, crosstalk between IGF-1R and G-protein coupled receptors (GPCRs) has been reported which may indicate a potential role of IGF-1R in cancer.
IRS 1-4 and other signaling proteins, such as Shc, bind to the IGF-1R at the extracellular subunit as a result of the binding of IGF-I and IGF-II (41). This autophosphorylation of the subunit residues prepares it to serve as a docking site for these proteins. PI3(p110) kinase’s subunit catalyzes the recruitment of protein kinase B (Akt) to the cell membrane (42), causing its phosphorylation and activation, then PI3 regulatory kinase’s component, p85, is recruited by IRS-1. Bcl2 antagonist of cell death (Bad), glycogen synthase kinase 3 (GSK3), forkhead transcription factors (FOXO1), and Akt substrate of 160 kDa are only a few of the many substrates for activated Akt (AS160). These elements have a major role in controlling cell metabolism and apoptosis (42, 43). By phosphorylating the protein Tuberous Sclerosis Protein (TSC2), Akt controls protein synthesis by loosening its inhibition on Rheb and activating the mammalian target of rapamycin (mTORC1). A set of mitogen-activated protein kinase (MAPKKK, MAPKK, and MAPK) pathways can be initiated by the phosphorylation of IRS-1 and Shc, which can also result in the recruitment of Grb2, SOS, and Ras. These pathways can then be activated, which promotes cell growth, migration, and survival (44, 45).
Recent research has shown that the IGF-1R (and IR) can migrate to the nucleus in both healthy and cancerous cells through mechanisms that are still being fully elucidated, although sumoylation is thought to be one method. The IGF-1R can bind to DNA and control the transcription of its own receptor gene as well as genes involved in apoptosis and the cell cycle. A more thorough analysis of the whole range of IGF-1R effects within the cell was completed (46).
The internalization of the IGF-1R is a complicated process that includes subcellular transport, intracellular signaling, and recycling of the receptor to the surface in addition to partial destruction of the receptor. The ligand binding process starts with the IGF-increased IR’s internalization (endocytosis). Through substrates that specifically bind to tyrosine residues 1250 and 1251 in the C-terminus, internalization, and degradation play a part. Internalization separates the ligand from the receptor via the acidic endosomal route, where caveolin- or clathrin-dependent mechanisms may be used for internalization (47). The lysosomal or proteasomal routes are both options for the receptor’s degradation. The process of receptor ubiquitination, which is brought on by ligand interaction, results in the receptor’s destruction by the proteasome. The receptor concentration on the surface may therefore be downregulated as a result of ligand binding and internalization, even though the levels may be adjusted by increased IGF-1R gene expression. A collection of proteins called adhesion-associated proteins may regulate subcellular transport. These include the discoidin domain receptor 1 (DDR1), non-receptor tyrosine adhesion kinase FES-related (FER), and non-integrin collagen RTK. The IGF-1R can translocate to the nucleus or the Golgi apparatus’ internal membrane compartments for destruction (28, 47). Intracellular signaling, which appears to be important in the migratory behavior of cancer cells, can be started by the IGF-1R in the Golgi. IGF-I induces the translocation of IGF-1R to the nucleus, where it may bind with DNA to increase transcription, with consequences that seem to support an aggressive cancer phenotype (28, 47).
The IGF family plays a critical role in various cellular processes such as proliferation, differentiation, and apoptosis (Figure 1). In particular, IGFBPs protect IGFs from degradation and regulate their interactions with the receptors. High circulating levels of the potent mitogen, IGF-I, were previously associated with an increased risk for breast, prostate, lung and colorectal cancers (48–51). Both mitogens, IGF-I and IGF-II, were identified to be overexpressed in various cancer types such as sarcoma, leukemia, breast, lung, colon, stomach, esophagus, liver, pancreas, kidney, thyroid, brain, ovary, cervical, endometrial and prostate cancers (52–57). High IGF-IR expression was positively associated with worse disease outcomes for several cancer types, including prostate and gastric cancer, as well as renal cell carcinoma (58–60).
Figure 1 Structure and role of insulin growth factor (IGF) family, including binding proteins, ligands, and receptors, in multiple processes involved in carcinogenesis.
IGF-I, the crucial peptide hormone involved in controlling human growth and development, functions by promoting cell growth and preventing apoptosis (54, 61, 62). Such actions have a significant impact on the tumor development (63). Other members of the IGF family directly affect cancer-related cellular activities and interact with a wide range of molecules that are crucial for cancer initiation and progression. IGF-I has been associated with the activation of the MAPK and PI3K signaling pathways (64). Additionally, diet and exercise have an impact on the expression and production of IGF-I (65). IGF-I overexpression was previously identified to induce tumor development (66, 67), while high expression of IGF-1R and IGF-II triggered cancer metastasis (68). Additionally, IGF-1R was reported to be essential for cell transformation triggered by oncogenes and tumor-virus proteins (53). Several approaches were implemented to inhibit the mitogenic effect of IGF signaling including eliminating IGF-1R from the cell membrane, blocking the interaction of IGFs with IGF-1R, or interrupting the signal transduction pathway (53, 69–71). On the other hand, IGF-2R is known to antagonize the effect of IGF-II, where tumors that had low expression of IGF-2R or possessed IGF-2R mutations and could not hence degrade IGF-II, had much higher growth rates (72, 73). Thus, restoring IGF-2R expression induced apoptosis and reduced the growth of cancer cells (74).
IGFBPs regulate the interaction between IGF-I and IGF-1R and affect the mitogenic activity of IGF-I (75–84). One of the most studied IGFBPs is IGFBP-3 which prolongs the half-life of the IGFs and regulates their availability to the cell surface receptors (85, 86). Besides, IGFBP-3 has anti-proliferative actions affecting cancer growth (87, 88). It was previously reported that serum levels of IGFBP-3 were inversely associated with cancer risk in those patients. Also, IGFBP-3 was found to inhibit breast and prostate cancer growth and induce apoptosis (89–91). Moreover, vitamin D and its synthetic analogs could increase the expression of IGFBPs and reduce IGF-1R and IGF-II in breast and prostate cancer (92–95). Another factor affecting the expression of IGF family members is the expression of tumor suppressor genes. For example, wild-type p53 protein induces IGFBP-3 expression, represses the transcription of IGF-II, and suppresses IGF-1R expression (96–101).
During the transition from benign to malignant state in prostate cancer, several IGF family members change. For instance, IGF-I, IGFBP-2 and IGFBP-5 levels rise, while IGF-IR and IGFBP-3 levels lessen (82, 102–104). The controversial reports about the expression of the IGF family and its connection to prostate cancer risk and development may be the result of the high degree of heterogeneity among prostate tumors, sampling bias, evaluation techniques, or the experimental design (105).
Some proteases produced in the prostate cancer microenvironment were previously identified to enhance IGF-I/IGF-1R signaling. These include prostate-specific antigen (PSA), human kallikrein 2, trypsin, and cathepsin D, which were identified to degrade IGFBPs, thus releasing free mitogenic IGF-I (106, 107). High IGF-I expression and low IGFBP-3 in prostate cancer were identified as contributors to cancer initiation and progression by affecting cellular transformation, apoptosis, and metastasis (108). IGF-I and IGFBP-3 expression in prostate cancer patients was strongly associated with more advanced or aggressive disease, indicating a critical role in cancer progression (49). Further studies highlighted the potential of IGF-I as a strong predictor of advanced rather than early-stage prostate cancer (109). On the contrary, a study by Ma et al. reported no significant associations between free IGF-I and other IGF-I biomarkers with lethal and non-lethal prostate cancer (110). However, blood levels of IGFBP-3 showed contradictory results in prostate cancer (111). Serum levels of IGF-I, IGF-II, IGFBP-2, IGFBP-4, and IGFBP-5 were found to be higher in prostate cancer patients (82, 103, 112–116). Moreover, IGFBP-2 expression was found to be higher in the prostate cancer tissue compared to the normal tissue counterpart (102). Neither IGF-II nor IGFBP-2 concentrations were associated with prostate cancer risk (117). On the other hand, low levels of IGFBP-3 were reported in the serum and prostate tumor tissue of cancer patients (102). Moreover, IGF-I was found to be significantly elevated while IGFBP-3 was reported to be reduced in prostate cancer tissues (118). Also, such an expression was found to be linked to tumor size and hyperplasia (118, 119). IGF-I and IGFBP-3 plasma levels were suggested to be indicators of prostate cancer risk (112). Moreover, the lower IGFBP-3 levels could lead to enhanced IGF-I bioavailability (85). In addition, the plasma levels of IGFBP-3 were considerably lower in African-American men compared to white men (120, 121). This could support the findings that African-American men have a higher incidence of prostate cancer than white men (122). Additionally, high IGF-I levels in the blood/serum of healthy men were associated with a high risk of developing prostate cancer (123, 124). This suggests that prolonged exposure to high concentrations of IGF-I could trigger carcinogenesis of prostate epithelial cells (125). Therefore, targeting IGF-I could be a potential therapeutic approach in prostate cancer.
When compared to the benign prostate epithelium, primary prostate cancer had higher levels of IGF-1R expression, which is further escalated in metastasis (126). This was further supported by studies using human prostate xenografts where higher IGF-1R expression was found in metastatic and androgen-independent tumors (127, 128). Also, transgenic mice with a prostate-specific deletion of IGF-1R and the tumor suppressor gene p53 had more aggressive prostate cancer than their wild-type counterparts (129).
In vitro, studies indicated that IGF-I stimulated the proliferation of various prostate cancer cell lines (22Rv1 and DU145) by the activation of the AKT/ERK/MAPK pathway (130). Also, IGF-I regulated the invasion potential of DU145 prostate cancer cells by controlling the activity of MMP-2 and MMP-9 as well as secreted TIMP-2 levels, that are transduced via the PI3K and MAPK pathways (131). Further, IGF-I was described to regulate the expression of miR-143, leading to an increase in IGFR expression in PC-3 and DU145 prostate cancer cell lines. Such an effect was found to lead to resistance to docetaxel treatment (132). Additionally, it has been demonstrated that IGF-I activated androgen receptor signaling in prostate cancer cells via the IGF-1R-forkhead box protein O1 (FOXO1) signaling axis, which is also implicated in castration-resistant prostate cancer (133–135). In vivo, PC-3 tumors proliferate at a considerably slower rate in IGF-I-deficient hosts than in IGF-I-expressing hosts (136). Mice injected with the androgen-sensitive and PSA-producing LNCaP cell line were found to develop tumors and their serum PSA levels were correlated with tumor volume (137). Also, mice fed a low-fat diet with xenografts of LAPC-4 showed a decrease in tumor size along with a reduction in the IGF-I expression (138). Other in vivo studies reported that the blocking of IGF-1R in combination with castration inhibited prostate cancer growth (139, 140). However, the most thoroughly studied IGF-1R inhibitor, limsitinib, was explored in a phase II study, where it did not significantly alleviate prostate-specific antigen levels after 12 weeks of treatment or increase the overall survival in men with metastatic castrate-resistant prostate cancer (141).
IGFBP-3 is the most prevalent form of the IGFBPs, which has been linked with prostatic growth. The majority of serum and prostatic IGF-I binds to IGFBP-3, thus regulating its concentrations (142). IGFBP-3 was identified to have anti-tumor effects by regulating multiple processes such as adhesion, motility, proliferation, and invasion of prostate cancer cells (143). Additionally, in vitro studies using PC3 or DU145 cell lines showed that IGF-I regulates cell adhesion and motility that is needed for the formation of a pre-metastatic niche (144). This was reported to be mediated through integrin expression especially α3, α5, and β1 expression pattern and distribution (145).
The second most prevalent IGFBP is IGFBP-2, which has a growth inhibitory effect on healthy prostate epithelial cells but a strong stimulatory effect on prostate cancer cells via the activation of MAPK and PI3K pathways (146). Also, high IGFBP-2 levels in prostate cancer patients not receiving neoadjuvant hormonal therapy had worse survival compared to patients with low IGFBP-2 levels, thus indicating an androgen effect on the IGFBP-2 action (147).
Proteases targeting IGFBPs are known to degrade IGFBPs into small fragments, thus reducing the affinity of IGFBPs to IGFs. Prostate-specific antigen (PSA) is known to be an IGFBP protease while γ-nerve growth factor (NGF) is also known to degrade IGFBPs 3, 4, 5, and 6, thereby boosting IGF action (11). Furthermore, other proteases such as human kallikrein 2 (hK2), trypsin, MMPs, and cathepsin D, present in the prostate tumor microenvironment were identified to affect IGFBP-3 and release free IGF-I (107). Protease-resistant IGFBPs could be a potential therapeutic agent in cancer therapy by preventing the mitogenic activity of IGF. Also, it was interesting to find that inhibiting the signaling pathway of epidermal growth factor (EGF), had a suppression effect on IGF-I in prostate cancer cells (148). The protease PAPP-A is responsible for the cleavage of IGFBPs 2, 4, and 5 (149), which was previously linked to cancer development in various types including prostate cancer (150–155). A possible explanation for the role of PAPP-A is through increasing the levels of IGFs and their downstream signaling pathway (156). Studies have reported that PAPP-A levels were elevated in prostate cancer patients, especially those with metastasis (157). On the other hand, several studies reported that the protease-resistant IGFBP-4 led to an inhibition of cell growth and angiogenesis (155, 158). Other in vitro and in vivo studies indicated that the mutant IGFBP-2 was able to inhibit tumor growth possibly by inhibition of angiogenesis (159).
A subgroup of IGFBPs is called IGFBP-related proteins (IGFBPs-rP) also termed the CNN family (124). These IGFBP-rPs could be critical for the regulation of stromal and epithelial cell growth in the prostate. An interesting protein is insulin-like growth factor binding protein-related protein 1 (IGFBP-rP1)/IGFBP-7. It was found to possess tumor suppressor effects through inhibiting cell growth, and triggering apoptosis and senescence (160). In contrast, another study indicated that it could promote glioma cell growth and migration (161). IGFBP-7 resulted in the activation of the translational repressor 4E-binding protein 1 (4E-BP1) and triggered apoptosis in IGF-1R+ cells. It suppressed IGF-1R downstream signaling, hence hindering protein synthesis, cell growth, and survival (162). In vitro and in vivo studies revealed a downregulation of IGFBP-5 in prostate cancer cells that inhibited IGF cell growth. In benign prostatic hyperplasia (BPH), IGF-II and IGF-1R were found to be overexpressed by stromal prostate cells. Also, these cells expressed IGFBP-5 which is identified to potentiate the IGF actions (163). Reduced expression of IGFBP-rP1 is associated with carcinogenesis, especially in highly tumorigenic and metastatic prostatic cells (164). IGFBP-rP1 functions as a tumor suppressor in prostate cancer cells, as its overexpression slowed down growth and proliferation rates (165). Also, prostatic cell growth is regulated by IGFBP-rP2 and IGFBP-rP3. Several factors can affect the expression of IGFBP-rP2. For instance, TGF-β boosts the expression of IGFBP-rP2 in both normal and cancerous prostate cells while IGF-I could decrease its expression. IGFBP-rP3 may act as a growth stimulator for prostate cancer cells given that it is preferentially expressed in malignant cells (166). CyrH61/IGFBPs-rP4 is localized in the mesenchyme of the benign prostatic tissue and is downregulated in prostate cancer tissues as well as in cancer cell lines (124, 167).
Our understanding of prostate tumorigenesis and regulation has been expanded especially due to our understanding of the IGF system. A high IGF-I to IGFBP-3 ratio was linked with an increased risk of prostate cancer and could be used as a predictor for prostate cancer development (168). On the other hand, another study by Saleh SAK et al. reported that changes in the serum levels of IGF-I and IGFBP-3 were not considered pre-diagnostic risk factors for prostate cancer development (142). Besides, serum levels of total PSA and free/total PSA ratio were found to be higher in prostate cancer patients (142). Also, PSA and IGF-II showed the power of prognosis and differentiation between prostate cancer and BPH (169).
Bones are the most frequent metastatic site for prostate cancer, with 70–80% of patients experiencing skeletal metastases. Prostate cancer can metastasize to numerous organs, including the liver, lymph nodes, lung, and bone. The vertebral column, ribs, skull, and proximal ends of the long bones are among the well-vascularized parts of the skeleton where prostate cancer cells spread frequently. IGF-I and IGF-II, as well as type I and type II IGF receptors, are expressed by bone cells (170). High levels of IGF-I in the primary tumor environment seem to encourage cancer cells to spread to the bone in vivo. Moreover, prostate cancer cell lines that highly expressed IGF-1R were likely to develop larger bone mass (171–173). Notably, IGFs influence cell-cell adhesion and motility of cancer cells through the integrin system such as E-cadherin (174–176). The likelihood of metastasis is influenced by such interactions (177–179). Prostate cancer cells can release mediators that change the balance between osteoblast and osteoclast activities, leading to osteoblastic metastases. IGF-I plays a critical role in bone formation and bone resorption through the receptor activator of the nuclear factor-B ligand (RANKL) system in bone (180). IGF-I upregulates the expression of RANKL by bone marrow stromal cells, and osteoblasts, which can bind to RANK on the surface of osteoclast precursors. This ligand-receptor interaction activates NF-κB, which stimulates the differentiation of osteoclast precursors to osteoclasts (180–183). Also, metastatic prostate cancer cells release a urokinase-type plasminogen activator that binds to the corresponding receptor on the surface of osteoblasts. This could trigger the proteolysis of IGFBPs and increase in the bioavailability of IGFs, thus stimulating the proliferation of osteoblasts and cancer cells (Figure 2). A study by Goya M. et al. reported the potential of KM1468, a monoclonal antibody against IGF-I and IGF-II that inhibited the development of new bone formation and the progression of existing bone tumors (184). Also, IGF-I is secreted by the liver, which attracts circulating cancer cells drain and promotes colonization, proliferation, and the establishment of metastasis (185).
Figure 2 Involvement of IGF ligands, receptors, and binding proteins in prostate cancer. The left panel illustrates the interplay of IGF-I in cancer initiation and progression of prostate cancer, while the right panel highlights the IGF-I effect in metastasis of prostate cancer cells to bone tissue.
IGF-1R was found to control lymphatic metastasis of cancer by inducing the production of VEGF, thus promoting angiogenesis and lymphangiogenesis (186). This was supported by high levels of VEGF in prostate cancer patients with lymph node metastases (187). Androgen deprivation activated FOXO-1 and upregulated VEGF production by inhibiting the IGF-1R pathway (188). By activation of the IGF-1R/Akt/NF-κB pathway, bone-derived IGF-I is the link between metastasized cancer cells during bone metastasis (189).
Prostate cancer could progress to be castration-resistant. Several mechanisms have been put forth to explain the progression including: 1) constitutive activation of the androgen receptor due to alternative splicing of the androgen receptor; 2) epigenetic mutations of the androgen receptor; 3) transactivation of the androgen receptor by growth factors and cytokines; and 4) intracrine steroidogenic pathways within the prostate tumor (190–193).
Another critical aspect of the IGF family is that they play a role in the progression of androgen-independent prostate cancer by promoting the growth and survival of prostate tumor cells. Alternative stimulatory pathways that were activated after androgen withdrawal, were found to trigger intracellular signal transduction pathways by completely avoiding or activating the androgen receptor. For example, non-ligand activation of androgen receptors could be initiated by IGF-I, MAPK, and AKT contributing to cell proliferation in an androgen-depleted environment (194). Also, prostate cancer mouse models showed progression from androgen-dependence to androgen-independence to be associated with an increase in IGF-I and decrease in IGFBP-3, further suggesting their role in cancer aggressiveness (128). In mice, castration induced upregulation of IGFBP-5 that enhanced the development of androgen independence (195).
The effective treatment for patients with advanced prostate cancer is androgen ablation, leading to tumor regression (195). This is because proliferation of prostate cancer cells is dependent on androgens. The androgen receptor is known to bind to testosterone and dihydrotestosterone, after which a conformational change and interaction with specific DNA elements occur. The development of androgen-independent prostate cancer takes place as a consequence of the lack of apoptosis that triggers tumor cell survival (196). Furthermore, other mechanisms are associated with the development of androgen-independent prostate cancer including an increase in the sensitivity of the androgen receptor by coactivators as well as enhancement of the signaling pathways by other growth factors (197, 198). Unlike androgens, IGF-I activates tyrosine kinase-associated surface receptors, leading to the proliferation of prostate cancer cells. This is besides the effect of IGF-I on enhancing the androgen receptor transcriptional activity and its sensitivity to suboptimal stimulation by low androgen levels (199). Metastatic and androgen-independent prostate cancer specimens exhibited an increase in IGF-1R expression (126–128). On the other hand, other studies indicated that reduced IGF-1R expression is required to induce androgen independence, proliferation, and metastasis (200, 201). It was suggested that dysregulation of IGF-1R expression through KLF6 loss-of-function may be an intrinsic mechanism for prostate cancer progression to hormone independence. Also, the tumor suppressor BRCA1 was identified to interact with the androgen receptor in prostate cancer cells and regulate IGF-1R production (202). Furthermore, BRCA1 can suppress IGF-1R promoter activity in androgen receptor-negative prostate cancer cell lines but enhance IGF-1R expression at the transcriptional level in androgen receptor-positive prostate cancer cell lines (203).
Also, long-term androgen suppression may boost the resistance of prostate cancer cells to apoptosis through the inhibition of PI3K/AKT pathway (204). IGF-1R expression can be enhanced by androgens via the activation of the ERK pathway (205). Neuropeptides, including endothelin-1, vasoactive intestinal peptide, and neurotensin, were previously identified to boost IGF signaling and stimulate androgen-independent prostate cancer (111, 206–208). Moreover, castration or anti-androgen treatment induces the expression of IGFBP-2,3,4 and 5 (209, 210). This could be due to an adaptative mechanism to enhance IGF-I mediated mitogenesis, thus leading to androgen independence progression (195, 195, 211). Also, prostate cancer samples and cell lines exhibited growth inhibition when treated with diethylstilbestrol (DES) (212). The expression of the growth-promoting IGFBP-6 was reported to be induced by DES treatment, thus, highlighting its contribution to the effect of DES in androgen-independent prostate cancer (213). Pre-clinical evidence supported the utilization of insulin/IGF-targeting agents in combination with androgen deprivation therapy for prostate cancer. This is attributed to the effect of insulin on the production of androgens by prostate cancer cells, which accelerated the emergence of castration-resistant prostate cancer (214).
For many years now, the gold standard of treatment for advanced or metastatic prostatic cancer has been androgen deprivation therapy (ADT) and second-generation androgen receptors signaling inhibitors (41). Unexpected resistance to castration and recurrence in the form of castration-resistant prostate cancer, for which there are few therapy choices, are regrettably major events contributing to the poor longevity of patients with prostatic cancer, even though these therapies initially demonstrated several pros (105). A lot of research has been done to design agents that target the IGF family and its signaling pathway. For instance, some studies aimed at reducing IGF-I levels or inhibiting the activity of IGF-IR and the downstream signaling pathways. Some of these drugs exhibited an anti-neoplastic activity (184, 215, 216). Antagonists of the growth hormone-releasing hormone, or growth hormone receptor (e.g., pegvisomant), and analogs of somatostatin (e.g. octreotide) resulted in a reduction of IGF-I levels (217). For instance, combinatorial therapy using octreotide and complete androgen blockage had beneficial results in patients with prostate cancer (218). Also, pegvisomant treatment halted the proliferation of prostatic cancer cells (219). As such, anti-IGF-1R monoclonal antibodies (mAbs), human neutralizing IGF antibodies, and tyrosine kinase inhibitors (ligand-gated IGF-R) have been developed. Part of these drugs have been evaluated in clinical studies regarding prostatic cancer either alone or in conjunction with traditional therapy (220).
The primary approach entails employing anti-IGF-1R mAbs to impede ligand-receptor interactions, thereby inducing the internalization and subsequent degradation of IGF-1R. For the treatment of malignant tumors, a number of therapeutic mAbs targeting IGF-1R have been developed, including ganitumab (IgG1) (139), cixutumumab (IgG1) (221–223), and figitumumab (IgG2) (222, 224, 225). The antibodies that have undergone evaluation in clinical trials have demonstrated anti-tumor growth effects to a certain degree and have been well tolerated by the majority of participants.
Cixutumumab and figitumumab have shown mixed results in clinical trials, with some benefits in PSA levels but inconsistent overall survival improvements (221, 226, 227). A12, another mAb, demonstrated promise in preclinical studies by inhibiting cancer cell growth, but requires further research (228, 229). Ganitumab, a unique mAb that avoids interfering with insulin signaling, showed good tolerability but lacks clinical trials in prostate cancer patients (139, 222). Other mAbs like BIIB022 are being explored in other cancers with positive safety profiles (230). Overall, IGF-1R mAbs hold promise for prostatic cancer treatment, but further research is needed to optimize their efficacy and safety, particularly in combination therapies, while exploring new mAbs with improved targeting strategies.
Monoclonal antibodies that neutralize IGF ligands inhibit proliferative and pro-survival signaling, which are initiated by IGF ligands. IGF-I/II neutralizing monoclonal antibodies simultaneously inhibit multiple IGF signaling pathways but do not affect insulin receptor-β (INSR), which regulates glucose homeostasis (231). As a result, they do not increase the risk of hyperglycemia compared to IGF-1R/INSR tyrosine kinase inhibitors. Few neutralizing antibodies for IGF have been developed, including xentuzumab (IgG1) and dusigitumab (IgG2). Human antibody xentuzumab (BI 836845) specifically targeted IGF-I and IGF-II. It exhibited potential in the treatment of breast cancer and in the mitigation of resistance in prostate cancer. It mainly functions by inhibiting IGF-I levels and impeding its effects. The initial trials were met with acclaim (232–234). Dusigitumab (MEDI-573) is an additional IGF signaling-targeting antibody, whose metabolic impact appeared to be comparatively lesser in nature when compared to alternative IGF-targeting therapies. Although it exhibited anti-tumor properties, its efficacy might be constrained by its relatively low binding affinity (235).
Linsitinib targets the insulin and IGF-1R receptors and demonstrated promise in preclinical research but had poor efficacy in clinical trials for prostate cancer among other malignancies. Though it was well tolerated, neither the tumor response nor PSA levels were improved. Though further research is needed, linsitinib may be helpful in breaking through chemotherapy resistance (141, 236, 237). An alternative tyrosine kinase inhibitor, BMS-754807, has shown promise in preclinical research by reducing the proliferation and causing cell death in cancer cells (238). It also affected insulin receptors, though, which could have an effect on blood sugar regulation (239). Its safety and effectiveness in prostate cancer patients have not yet been evaluated in clinical trials.
PCa remains a public health burden. The relationship between the IGF family and prostate cancer is intricate as IGF signaling plays a significant role in the development and progression of prostate cancer. The activation of its downstream signaling pathways promotes cell proliferation, migration, and invasion. Targeting IGF signaling has been considered as a potential therapeutic strategy for PCa. Researchers have explored various approaches to inhibit IGF signaling using antibodies or small molecule inhibitors against various components of the IGF signaling pathway. Therefore, further understanding of the role of IGF signaling in PCa shows promise and needs to be considered in the context of a comprehensive treatment strategy.
NE: Writing – original draft, Writing – review & editing. HH: Writing – original draft, Writing – review & editing. MS: Writing – original draft, Writing – review & editing. WE: Writing – original draft, Writing – review & editing. IT: Writing – original draft, Writing – review & editing.
The author(s) declare that no financial support was received for the research, authorship, and/or publication of this article.
The authors declare that the research was conducted in the absence of any commercial or financial relationships that could be construed as a potential conflict of interest.
All claims expressed in this article are solely those of the authors and do not necessarily represent those of their affiliated organizations, or those of the publisher, the editors and the reviewers. Any product that may be evaluated in this article, or claim that may be made by its manufacturer, is not guaranteed or endorsed by the publisher.
1. Bray F, Ferlay J, Soerjomataram I, Siegel RL, Torre LA, Jemal A. Global cancer statistics 2018: GLOBOCAN estimates of incidence and mortality worldwide for 36 cancers in 185 countries. CA: Cancer J Clin. (2018) 68:394–424. doi: 10.3322/caac.21492
2. Ferlay J, Soerjomataram I, Dikshit R, Eser S, Mathers C, Rebelo M, et al. Cancer incidence and mortality worldwide: sources, methods and major patterns in GLOBOCAN 2012. Int J Cancer. (2015) 136:E359–86. doi: 10.1002/ijc.29210
3. Sung H, Ferlay J, Siegel RL, Laversanne M, Soerjomataram I, Jemal A, et al. Global cancer statistics 2020: GLOBOCAN estimates of incidence and mortality worldwide for 36 cancers in 185 countries. CA: Cancer J Clin. (2021) 71:209–49. doi: 10.3322/caac.21660
4. He Y, Xu W, Xiao Y-T, Huang H, Gu D, Ren S. Targeting signaling pathways in prostate cancer: mechanisms and clinical trials. Signal Transduction Targeted Ther. (2022) 7:198. doi: 10.1038/s41392-022-01042-7
5. Cuzick J, Thorat MA, Andriole G, Brawley OW, Brown PH, Culig Z, et al. Prevention and early detection of prostate cancer. Lancet Oncol. (2014) 15:e484–92. doi: 10.1016/s1470-2045(14)70211-6
6. Desai MM, Cacciamani GE, Gill K, Zhang J, Liu L, Abreu A, et al. Trends in incidence of metastatic prostate cancer in the US. JAMA Netw Open. (2022) 5:e222246. doi: 10.1001/jamanetworkopen.2022.2246
7. Prostate cancer: a tale of two sides. Nat Rev Urol. (2019) 16:141. doi: 10.1038/s41585-019-0152-z
8. Siegel RL, Miller KD, Jemal A. Cancer statistics, 2018. CA: Cancer J Clin. (2018) 68:7–30. doi: 10.3322/caac.21442
9. Simpson A, Petnga W, Macaulay VM, Weyer-Czernilofsky U, Bogenrieder T. Insulin-like growth factor (IGF) pathway targeting in cancer: role of the IGF axis and opportunities for future combination studies. Targeted Oncol. (2017) 12:571–97. doi: 10.1007/s11523-017-0514-5
10. Rosenzweig SA. The continuing evolution of insulin-like growth factor signaling. F1000Research. (2020) 9(F1000 Faculty Rev):205. doi: 10.12688/f1000research.22198.1
11. Brahmkhatri VP, Prasanna C, Atreya HS. Insulin-like growth factor system in cancer: novel targeted therapies. BioMed Res Int. (2015) 2015:538019. doi: 10.1155/2015/538019
12. LeRoith D, Holly JMP, Forbes BE. Insulin-like growth factors: Ligands, binding proteins, and receptors. Mol Metab. (2021) 52:101245. doi: 10.1016/j.molmet.2021.101245
13. Yoshida T, Delafontaine P. Mechanisms of IGF-1-mediated regulation of skeletal muscle hypertrophy and atrophy. Cells. (2020) 9:1970. doi: 10.3390/cells9091970
14. Cohick WS, Clemmons DR. The insulin-like growth factors. Annu Rev Physiol. (1993) 55:131–53. doi: 10.1146/annurev.ph.55.030193.001023
15. Bach LA. The insulin-like growth factor system: towards clinical applications. Clin Biochemist Rev. (2004) 25:155.
16. Miller BS, Rogol AD, Rosenfeld RG. The history of the insulin-like growth factor system. Hormone Res Paediatrics. (2022) 95:619–30. doi: 10.1159/000527123
17. Denley A, Cosgrove LJ, Booker GW, Wallace JC, Forbes BE. Molecular interactions of the IGF system. Cytokine Growth factor Rev. (2005) 16:421–39. doi: 10.1016/j.cytogfr.2005.04.004
18. Vigneri R, Squatrito S, Sciacca L. Insulin and its analogs: actions via insulin and IGF receptors. Acta diabetologica. (2010) 47:271–8. doi: 10.1007/s00592-010-0215-3
19. Stratikopoulos E, Szabolcs M, Dragatsis I, Klinakis A, Efstratiadis A. The hormonal action of IGF1 in postnatal mouse growth. Proc Natl Acad Sci. (2008) 105:19378–83. doi: 10.1073/pnas.0809223105
20. Frasca F, Pandini G, Scalia P, Sciacca L, Mineo R, Costantino A, et al. Insulin receptor isoform A, a newly recognized, high-affinity insulin-like growth factor II receptor in fetal and cancer cells. Mol Cell Biol. (1999) 19:3278–88. doi: 10.1128/MCB.19.5.3278
21. Puche JE, Castilla-Cortázar I. Human conditions of insulin-like growth factor-I (IGF-I) deficiency. J Trans Med. (2012) 10:1–29. doi: 10.1186/1479-5876-10-224
22. Blyth AJ, Kirk NS, Forbes BE. Understanding IGF-II action through insights into receptor binding and activation. Cells. (2020) 9:2276. doi: 10.3390/cells9102276
23. Allard JB, Duan C. IGF-binding proteins: why do they exist and why are there so many? Front Endocrinol. (2018) 9:117. doi: 10.3389/fendo.2018.00117
24. Daza DO, Sundström G, Bergqvist CA, Duan C, Larhammar D. Evolution of the insulin-like growth factor binding protein (IGFBP) family. Endocrinology. (2011) 152:2278–89. doi: 10.1210/en.2011-0047
25. Hwa V, Oh Y, Rosenfeld RG. The insulin-like growth factor-binding protein (IGFBP) superfamily. Endocrine Rev. (1999) 20:761–87. doi: 10.1210/edrv.20.6.0382
26. Clemmons DR. Role of post translational modifications in modifying the biologic activity of insulin like growth factor binding proteins. Curr Dir insulin-like Growth factor Res. (1993) 343:245–53. doi: 10.1007/978-1-4615-2988-0_24
27. Weinzimer SA, Cohen P. Biological significance of insulin-like growth factor binding proteins. NeuroImmune Biol. (2002) 2:37–65. doi: 10.1016/S1567-7443(02)80007-2
28. Baxter RC. Signaling pathways of the insulin-like growth factor binding proteins. Endocrine Rev. (2023) 44:bnad008. doi: 10.1210/endrev/bnad008
29. Poreba E, Durzynska J. Nuclear localization and actions of the insulin-like growth factor 1 (IGF-1) system components: Transcriptional regulation and DNA damage response. Mutat Research/Reviews Mutat Res. (2020) 784:108307. doi: 10.1016/j.mrrev.2020.108307
30. Scharf J, Ramadori G, Braulke T, Hartmann H. Synthesis of insulinlike growth factor binding proteins and of the acid-labile subunit in primary cultures of rat hepatocytes, of Kupffer cells, and in cocultures: Regulation by insulin, insulinlike growth factor, and growth hormone. Hepatology. (1996) 23:818–27. doi: 10.1002/(ISSN)1527-3350
31. Firth SM, Baxter RC. Cellular actions of the insulin-like growth factor binding proteins. Endocrine Rev. (2002) 23:824–54. doi: 10.1210/er.2001-0033
32. Weis F, Menting JG, Margetts MB, Chan SJ, Xu Y, Tennagels N, et al. The signalling conformation of the insulin receptor ectodomain. Nat Commun. (2018) 9:4420. doi: 10.1038/s41467-018-06826-6
33. De Meyts P. Insulin/receptor binding: the last piece of the puzzle? What recent progress on the structure of the insulin/receptor complex tells us (or not) about negative cooperativity and activation. Bioessays. (2015) 37:389–97. doi: 10.1002/bies.201400190
34. Ngo M-HT, Jeng H-Y, Kuo Y-C, Nanda JD, Brahmadhi A, Ling T-Y, et al. The role of IGF/IGF-1R signaling in hepatocellular carcinomas: stemness-related properties and drug resistance. Int J Mol Sci. (2021) 22:1931. doi: 10.3390/ijms22041931
35. Wu JD, Haugk K, Woodke L, Nelson P, Coleman I, Plymate SR. Interaction of IGF signaling and the androgen receptor in prostate cancer progression. J Cell Biochem. (2006) 99:392–401. doi: 10.1002/jcb.20929
36. Werner H. Tumor suppressors govern insulin-like growth factor signaling pathways: implications in metabolism and cancer. Oncogene. (2012) 31:2703–14. doi: 10.1038/onc.2011.447
37. Wang P, Mak VCY, Cheung LWT. Drugging IGF-1R in cancer: New insights and emerging opportunities. Genes Dis. (2023) 10:199–211. doi: 10.1016/j.gendis.2022.03.002
38. Mancarella C, Morrione A, Scotlandi K. Unraveling the IGF system interactome in sarcomas exploits novel therapeutic options. Cells. (2021) 10:2075. doi: 10.3390/cells10082075
39. Moonesi M, Zaka Khosravi S, Molaei Ramshe S, Allahbakhshian Farsani M, Solali S, Mohammadi MH, et al. IGF family effects on development, stability, and treatment of hematological Malignancies. J Cell Physiol. (2021) 236:4097–105. doi: 10.1002/jcp.30156
40. Novosyadlyy R, Kurshan N, Lann D, Vijayakumar A, Yakar S, LeRoith D. Insulin-like growth factor-I protects cells from ER stress-induced apoptosis via enhancement of the adaptive capacity of endoplasmic reticulum. Cell Death Differentiation. (2008) 15:1304–17. doi: 10.1038/cdd.2008.52
41. Liu G, Zhu M, Zhang M, Pan F. Emerging role of IGF-1 in prostate cancer: A promising biomarker and therapeutic target. Cancers. (2023) 15:1287. doi: 10.3390/cancers15041287
42. Hua H, Kong Q, Yin J, Zhang J, Jiang Y. Insulin-like growth factor receptor signaling in tumorigenesis and drug resistance: a challenge for cancer therapy. J Hematol Oncol. (2020) 13:1–17. doi: 10.1186/s13045-020-00904-3
43. Nitulescu GM, Van De Venter M, Nitulescu G, Ungurianu A, Juzenas P, Peng Q, et al. The Akt pathway in oncology therapy and beyond. Int J Oncol. (2018) 53:2319–31. doi: 10.3892/ijo
44. Revathidevi S, Munirajan AK. Akt in cancer: mediator and more. Semin Cancer Biol. (2019) 59:80–91. doi: 10.1016/j.semcancer.2019.06.002
45. Janssen JA. New insights from IGF-IR stimulating activity analyses: Pathological considerations. Cells. (2020) 9:862. doi: 10.3390/cells9040862
46. Chughtai S. The nuclear translocation of insulin-like growth factor receptor and its significance in cancer cell survival. Cell Biochem Funct. (2020) 38:347–51. doi: 10.1002/cbf.3479
47. Rieger L, O’Connor R. Controlled signaling—insulin-like growth factor receptor endocytosis and presence at intracellular compartments. Front Endocrinol. (2021) 11:620013. doi: 10.3389/fendo.2020.620013
48. Hankinson SE, Willett WC, Colditz GA, Hunter DJ, Michaud DS, Deroo B, et al. Circulating concentrations of insulin-like growth factor-I and risk of breast cancer. Lancet (London England). (1998) 351:1393–6. doi: 10.1016/s0140-6736(97)10384-1
49. Chan JM, Stampfer MJ, Giovannucci E, Gann PH, Ma J, Wilkinson P, et al. Plasma insulin-like growth factor-I and prostate cancer risk: a prospective study. Sci (New York NY). (1998) 279:563–6. doi: 10.1126/science.279.5350.563
50. Yu H, Spitz MR, Mistry J, Gu J, Hong WK, Wu X. Plasma levels of insulin-like growth factor-I and lung cancer risk: a case-control analysis. J Natl Cancer Institute. (1999) 91:151–6. doi: 10.1093/jnci/91.2.151
51. Ma J, Pollak MN, Giovannucci E, Chan JM, Tao Y, Hennekens CH, et al. Prospective study of colorectal cancer risk in men and plasma levels of insulin-like growth factor (IGF)-I and IGF-binding protein-3. J Natl Cancer Institute. (1999) 91:620–5. doi: 10.1093/jnci/91.7.620
52. Frostad S, Bruserud O. In vitro effects of insulin-like growth factor-1 (IGF-1) on proliferation and constitutive cytokine secretion by acute myelogenous leukemia blasts. Eur J Haematol. (1999) 62:191–8. doi: 10.1111/j.1600-0609.1999.tb01743.x
53. LeRoith D, Baserga R, Helman L, Roberts CT Jr. Insulin-like growth factors and cancer. Ann Intern Med. (1995) 122:54–9. doi: 10.7326/0003-4819-122-1-199501010-00009
54. Macaulay VM. Insulin-like growth factors and cancer. Br J Cancer. (1992) 65:311–20. doi: 10.1038/bjc.1992.65
55. Oku K, Tanaka A, Yamanishi H, Nishizawa Y, Matsumoto K, Shiozaki H, et al. Effects of various growth factors on growth of a cloned human esophageal squamous cancer cell line in a protein-free medium. Anticancer Res. (1991) 11:1591–5.
56. Singh P, Dai B, Yallampalli U, Lu X, Schroy PC. Proliferation and differentiation of a human colon cancer cell line (CaCo2) is associated with significant changes in the expression and secretion of insulin-like growth factor (IGF) IGF-II and IGF binding protein-4: role of IGF-II. Endocrinology. (1996) 137:1764–74. doi: 10.1210/endo.137.5.8612513
57. Yaginuma Y, Nishiwaki K, Kitamura S, Hayashi H, Sengoku K, Ishikawa M. Relaxation of insulin-like growth factor-II gene imprinting in human gynecologic tumors. Oncology. (1997) 54:502–7. doi: 10.1159/000227610
58. Zu K, Martin NE, Fiorentino M, Flavin R, Lis RT, Sinnott JA, et al. Protein expression of PTEN, insulin-like growth factor I receptor (IGF-IR), and lethal prostate cancer: A prospective study. Cancer Epidemiology Biomarkers Prev. (2013) 22:1984–93. doi: 10.1158/1055-9965.EPI-13-0349
59. Sichani MM, Yazdi FS, Moghaddam NA, Chehrei A, Kabiri M, Naeimi A, et al. Prognostic value of insulin- like growth factor-I receptor expression in renal cell carcinoma. Saudi J Kidney Dis Transplant. (2010) 21:69–74.
60. Matsubara J, Yamada Y, Hirashima Y, Takahari D, Okita NT, Kato K, et al. Impact of insulin-like growth factor type 1 receptor, epidermal growth factor receptor, and HER2 expressions on outcomes of patients with gastric cancer. Clin Cancer Res. (2008) 14:3022–9. doi: 10.1158/1078-0432.CCR-07-1898
61. Qu BH, Karas M, Koval A, LeRoith D. Insulin receptor substrate-4 enhances insulin-like growth factor-I-induced cell proliferation. J Biol Chem. (1999) 274:31179–84. doi: 10.1074/jbc.274.44.31179
62. Párrizas M, Saltiel AR, LeRoith D. Insulin-like growth factor 1 inhibits apoptosis using the phosphatidylinositol 3’-kinase and mitogen-activated protein kinase pathways. J Biol Chem. (1997) 272:154–61. doi: 10.1074/jbc.272.1.154
63. Cory S, Vaux DL, Strasser A, Harris AW, Adams JM. Insights from Bcl-2 and Myc: Malignancy involves abrogation of apoptosis as well as sustained proliferation. Cancer Res. (1999) 59:1685s–92s.
64. Hakuno F, Takahashi S-I. 40 YEARS OF IGF1: IGF1 receptor signaling pathways. J Mol Endocrinol. (2018) 61:T69–86. doi: 10.1530/JME-17-0311
65. Yu H, Rohan T. Role of the insulin-like growth factor family in cancer development and progression. J Natl Cancer Institute. (2000) 92:1472–89. doi: 10.1093/jnci/92.18.1472
66. Rogler CE, Yang D, Rossetti L, Donohoe J, Alt E, Chang CJ, et al. Altered body composition and increased frequency of diverse Malignancies in insulin-like growth factor-II transgenic mice. J Biol Chem. (1994) 269:13779–84. doi: 10.1016/S0021-9258(17)36715-7
67. Bates P, Fisher R, Ward A, Richardson L, Hill DJ, Graham CF. Mammary cancer in transgenic mice expressing insulin-like growth factor II (IGF-II). Br J Cancer. (1995) 72:1189–93. doi: 10.1038/bjc.1995.484
68. Guerra FK, Eijan AM, Puricelli L, Alonso DF, de Kier Joffé EB, Kornblihtt AR, et al. Varying patterns of expression of insulin-like growth factors I and II and their receptors in murine mammary adenocarcinomas of different metastasizing ability. Int J Cancer. (1996) 65:812–20. doi: 10.1002/(ISSN)1097-0215
69. Pietrzkowski Z, Mulholland G, Gomella L, Jameson BA, Wernicke D, Baserga R. Inhibition of growth of prostatic cancer cell lines by peptide analogues of insulin-like growth factor 1. Cancer Res. (1993) 53:1102–6.
70. Neuenschwander S, Roberts CT Jr., LeRoith D. Growth inhibition of MCF-7 breast cancer cells by stable expression of an insulin-like growth factor I receptor antisense ribonucleic acid. Endocrinology. (1995) 136:4298–303. doi: 10.1210/endo.136.10.7664648
71. Jiang Y, Rom WN, Yie TA, Chi CX, Tchou-Wong KM. Induction of tumor suppression and glandular differentiation of A549 lung carcinoma cells by dominant-negative IGF-I receptor. Oncogene. (1999) 18:6071–7. doi: 10.1038/sj.onc.1202984
72. Byrd JC, Devi GR, de Souza AT, Jirtle RL, MacDonald RG. Disruption of ligand binding to the insulin-like growth factor II/mannose 6-phosphate receptor by cancer-associated missense mutations. J Biol Chem. (1999) 274:24408–16. doi: 10.1074/jbc.274.34.24408
73. O’Gorman DB, Costello M, Weiss J, Firth SM, Scott CD. Decreased insulin-like growth factor-II/mannose 6-phosphate receptor expression enhances tumorigenicity in JEG-3 cells. Cancer Res. (1999) 59:5692–4.
74. Souza RF, Wang S, Thakar M, Smolinski KN, Yin J, Zou TT, et al. Expression of the wild-type insulin-like growth factor II receptor gene suppresses growth and causes death in colorectal carcinoma cells. Oncogene. (1999) 18:4063–8. doi: 10.1038/sj.onc.1202768
75. Pollak M. Insulin and insulin-like growth factor signalling in neoplasia. Nat Rev Cancer. (2008) 8:915–28. doi: 10.1038/nrc2536
76. Bachrach LK, Nanto-Salonen K, Tapanainen P, Rosenfeld RG, Gargosky SE. Insulin-like growth factor binding protein production in human follicular thyroid carcinoma cells. Growth Regul. (1995) 5:109–18.
77. Kimura G, Kasuya J, Giannini S, Honda Y, Mohan S, Kawachi M, et al. Insulin-like growth factor (IGF) system components in human prostatic cancer cell-lines: LNCaP, DU145, and PC-3 cells. Int J Urol. (1996) 3:39–46. doi: 10.1111/j.1442-2042.1996.tb00628.x
78. Pekonen F, Nyman T, Ilvesmaki V, Partanen S. Insulin-like growth factor binding proteins in human breast cancer tissue. Cancer Res. (1992) 52:5204–7.
79. Perks CM, Bowen S, Gill ZP, Newcomb PV, Holly JM. Differential IGF-independent effects of insulin-like growth factor binding proteins (1-6) on apoptosis of breast epithelial cells. J Cell Biochem. (1999) 75:652–64. doi: 10.1002/(ISSN)1097-4644
80. Reeve JG, Morgan J, Schwander J, Bleehen NM. Role for membrane and secreted insulin-like growth factor-binding protein-2 in the regulation of insulin-like growth factor action in lung tumors. Cancer Res. (1993) 53:4680–5.
81. Sheikh MS, Shao ZM, Clemmons DR, LeRoith D, Roberts CT Jr., Fontana JA. Identification of the insulin-like growth factor binding proteins 5 and 6 (IGFBP-5 and 6) in human breast cancer cells. Biochem Biophys Res Commun. (1992) 183:1003–10. doi: 10.1016/s0006-291x(05)80290-6
82. Tennant MK, Thrasher JB, Twomey PA, Birnbaum RS, Plymate SR. Insulin-like growth factor-binding proteins (IGFBP)-4, -5, and -6 in the benign and Malignant human prostate: IGFBP-5 messenger ribonucleic acid localization differs from IGFBP-5 protein localization. J Clin Endocrinol Metab. (1996) 81:3783–92. doi: 10.1210/jcem.81.10.8855838
83. Yee D, Favoni RE, Lippman ME, Powell DR. Identification of insulin-like growth factor binding proteins in breast cancer cells. Breast Cancer Res Treat. (1991) 18:3–10. doi: 10.1007/BF01975437
84. Yee D, Jackson JG, Kozelsky TW, Figueroa JA. Insulin-like growth factor binding protein 1 expression inhibits insulin-like growth factor I action in MCF-7 breast cancer cells. Cell Growth Differ. (1994) 5:73–7.
85. Jones JI, Clemmons DR. Insulin-like growth factors and their binding proteins: biological actions. Endocrine Rev. (1995) 16:3–34. doi: 10.1210/edrv-16-1-3
86. Baxter RC, Butt AJ, Schedlich LJ, Martin JL. Antiproliferative and pro-apoptotic activities of insulin-like growth factor-binding protein-3. Growth hormone IGF research: Off J Growth Hormone Res Soc Int IGF Res Soc. (2000) 10 Suppl A:S10–1. doi: 10.1016/s1096-6374(00)90004-2
87. Butt AJ, Firth SM, Baxter RC. The IGF axis and programmed cell death. Immunol Cell Biol. (1999) 77:256–62. doi: 10.1046/j.1440-1711.1999.00822.x
88. Hong J, Zhang G, Dong F, Rechler MM. Insulin-like growth factor (IGF)-binding protein-3 mutants that do not bind IGF-I or IGF-II stimulate apoptosis in human prostate cancer cells. J Biol Chem. (2002) 277:10489–97. doi: 10.1074/jbc.M109604200
89. Oh Y, Müller HL, Lamson G, Rosenfeld RG. Insulin-like growth factor (IGF)-independent action of IGF-binding protein-3 in Hs578T human breast cancer cells. Cell surface binding and growth inhibition. J Biol Chem. (1993) 268:14964–71. doi: 10.1016/S0021-9258(18)82426-7
90. Gill ZP, Perks CM, Newcomb PV, Holly JM. Insulin-like growth factor-binding protein (IGFBP-3) predisposes breast cancer cells to programmed cell death in a non-IGF-dependent manner. J Biol Chem. (1997) 272:25602–7. doi: 10.1074/jbc.272.41.25602
91. Rajah R, Valentinis B, Cohen P. Insulin-like growth factor (IGF)-binding protein-3 induces apoptosis and mediates the effects of transforming growth factor-beta1 on programmed cell death through a p53- and IGF-independent mechanism. J Biol Chem. (1997) 272:12181–8. doi: 10.1074/jbc.272.18.12181
92. Rozen F, Yang XF, Huynh H, Pollak M. Antiproliferative action of vitamin D-related compounds and insulin-like growth factor-binding protein 5 accumulation. J Natl Cancer Institute. (1997) 89:652–6. doi: 10.1093/jnci/89.9.652
93. Colston KW, Perks CM, Xie SP, Holly JM. Growth inhibition of both MCF-7 and Hs578T human breast cancer cell lines by vitamin D analogues is associated with increased expression of insulin-like growth factor binding protein-3. J Mol Endocrinol. (1998) 20:157–62. doi: 10.1677/jme.0.0200157
94. Xie SP, James SY, Colston KW. Vitamin D derivatives inhibit the mitogenic effects of IGF-I on MCF-7 human breast cancer cells. J Endocrinol. (1997) 154:495–504. doi: 10.1677/joe.0.1540495
95. Huynh H, Pollak M, Zhang JC. Regulation of insulin-like growth factor (IGF) II and IGF binding protein 3 autocrine loop in human PC-3 prostate cancer cells by vitamin D metabolite 1,25(OH)2D3 and its analog EB1089. Int J Oncol. (1998) 13:137–43. doi: 10.3892/ijo.13.1.137
96. Ohlsson C, Kley N, Werner H, LeRoith D. p53 regulates insulin-like growth factor-I (IGF-I) receptor expression and IGF-I-induced tyrosine phosphorylation in an osteosarcoma cell line: interaction between p53 and Sp1. Endocrinology. (1998) 139:1101–7. doi: 10.1210/endo.139.3.5832
97. Webster NJ, Resnik JL, Reichart DB, Strauss B, Haas M, Seely BL. Repression of the insulin receptor promoter by the tumor suppressor gene product p53: a possible mechanism for receptor overexpression in breast cancer. Cancer Res. (1996) 56:2781–8.
98. Werner H, Karnieli E, Rauscher FJ, LeRoith D. Wild-type and mutant p53 differentially regulate transcription of the insulin-like growth factor I receptor gene. Proc Natl Acad Sci U.S.A. (1996) 93:8318–23. doi: 10.1073/pnas.93.16.8318
99. Zhang L, Kashanchi F, Zhan Q, Zhan S, Brady JN, Fornace AJ, et al. Regulation of insulin-like growth factor II P3 promotor by p53: a potential mechanism for tumorigenesis. Cancer Res. (1996) 56:1367–73.
100. Zhang L, Zhan Q, Zhan S, Kashanchi F, Fornace AJ Jr., Seth P, et al. p53 regulates human insulin-like growth factor II gene expression through active P4 promoter in rhabdomyosarcoma cells. DNA Cell Biol. (1998) 17:125–31. doi: 10.1089/dna.1998.17.125
101. Buckbinder L, Talbott R, Velasco-Miguel S, Takenaka I, Faha B, Seizinger BR, et al. Induction of the growth inhibitor IGF-binding protein 3 by p53. Nature. (1995) 377:646–9. doi: 10.1038/377646a0
102. Tennant MK, Thrasher JB, Twomey PA, Birnbaum RS, Plymate SR. Insulin-like growth factor-binding protein-2 and -3 expression in benign human prostate epithelium, prostate intraepithelial neoplasia, and adenocarcinoma of the prostate. J Clin Endocrinol Metab. (1996) 81:411–20. doi: 10.1210/jcem.81.1.8550786
103. Tennant MK, Thrasher JB, Twomey PA, Drivdahl RH, Birnbaum RS, Plymate SR. Protein and messenger ribonucleic acid (mRNA) for the type 1 insulin-like growth factor (IGF) receptor is decreased and IGF-II mRNA is increased in human prostate carcinoma compared to benign prostate epithelium. J Clin Endocrinol Metab. (1996) 81:3774–82. doi: 10.1210/jcem.81.10.8855837
104. Plymate SR, Tennant M, Birnbaum RS, Thrasher JB, Chatta G, Ware JL. The effect on the insulin-like growth factor system in human prostate epithelial cells of immortalization and transformation by simian virus-40 T antigen. J Clin Endocrinol Metab. (1996) 81:3709–16. doi: 10.1210/jcem.81.10.8855827
105. Wu J, Yu E. Insulin-like growth factor receptor-1 (IGF-IR) as a target for prostate cancer therapy. Cancer metastasis Rev. (2014) 33:607–17. doi: 10.1007/s10555-013-9482-0
106. Cohen P, Graves HC, Peehl DM, Kamarei M, Giudice LC, Rosenfeld RG. Prostate-specific antigen (PSA) is an insulin-like growth factor binding protein-3 protease found in seminal plasma. J Clin Endocrinol Metab. (1992) 75:1046–53. doi: 10.1210/jcem.75.4.1383255
107. Koistinen H, Paju A, Koistinen R, Finne P, Lövgren J, Wu P, et al. Prostate-specific antigen and other prostate-derived proteases cleave IGFBP-3, but prostate cancer is not associated with proteolytically cleaved circulating IGFBP-3. Prostate. (2002) 50:112–8. doi: 10.1002/pros.10039
108. Pollak M. Insulin-like growth factors and prostate cancer. Epidemiologic Rev. (2001) 23:59–66. doi: 10.1093/oxfordjournals.epirev.a000796
109. Chan JM, Stampfer MJ, Ma J, Gann P, Gaziano JM, Pollak M, et al. Insulin-like growth factor-I (IGF-I) and IGF binding protein-3 as predictors of advanced-stage prostate cancer. J Natl Cancer Institute. (2002) 94:1099–106. doi: 10.1093/jnci/94.14.1099
110. Ma C, Wang Y, Wilson KM, Mucci LA, Stampfer MJ, Pollak M, et al. Circulating insulin-like growth factor 1-related biomarkers and risk of lethal prostate cancer. JNCI Cancer Spectr. (2022) 6:pkab091. doi: 10.1093/jncics/pkab091
111. Renehan AG, Zwahlen M, Minder C, O’Dwyer ST, Shalet SM, Egger M. Insulin-like growth factor (IGF)-I, IGF binding protein-3, and cancer risk: systematic review and meta-regression analysis. Lancet (London England). (2004) 363:1346–53. doi: 10.1016/s0140-6736(04)16044-3
112. Mantzoros CS, Tzonou A, Signorello LB, Stampfer M, Trichopoulos D, Adami HO. Insulin-like growth factor 1 in relation to prostate cancer and benign prostatic hyperplasia. Br J Cancer. (1997) 76:1115–8. doi: 10.1038/bjc.1997.520
113. Djavan B, Bursa B, Seitz C, Soeregi G, Remzi M, Basharkhah A, et al. Insulin-like growth factor 1 (IGF-1), IGF-1 density, and IGF-1/PSA ratio for prostate cancer detection. Urology. (1999) 54:603–6. doi: 10.1016/s0090-4295(99)00280-0
114. Ho PJ, Baxter RC. Insulin-like growth factor-binding protein-2 in patients with prostate carcinoma and benign prostatic hyperplasia. Clin Endocrinol. (1997) 46:333–42.
115. Cohen P, Peehl DM, Stamey TA, Wilson KF, Clemmons DR, Rosenfeld RG. Elevated levels of insulin-like growth factor-binding protein-2 in the serum of prostate cancer patients. J Clin Endocrinol Metab. (1993) 76:1031–5. doi: 10.1210/jcem.76.4.7682560
116. Kanety H, Madjar Y, Dagan Y, Levi J, Papa MZ, Pariente C, et al. Serum insulin-like growth factor-binding protein-2 (IGFBP-2) is increased and IGFBP-3 is decreased in patients with prostate cancer: correlation with serum prostate-specific antigen. J Clin Endocrinol Metab. (1993) 77:229–33. doi: 10.1210/jcem.77.1.7686915
117. Roddam AW, Allen NE, Appleby P, Key TJ, Ferrucci L, Carter HB, et al. Insulin-like growth factors, their binding proteins, and prostate cancer risk: analysis of individual patient data from 12 prospective studies. Ann Intern Med. (2008) 149:461–71. doi: 10.7326/0003-4819-149-7-200810070-00006
118. Sreenivasulu K, Nandeesha H, Dorairajan LN, Rajappa M, Vinayagam V, Cherupanakkal C. Gene expression of insulin receptor, insulin-like growth factor increases and insulin-like growth factor-binding protein-3 reduces with increase in prostate size in benign prostatic hyperplasia. Aging Male. (2018) 21:138–44. doi: 10.1080/13685538.2017.1401994
119. Qian Q, He W, Liu D, Yin J, Ye L, Chen P, et al. M2a macrophage can rescue proliferation and gene expression of benign prostate hyperplasia epithelial and stroma cells from insulin-like growth factor 1 knockdown. Prostate. (2021) 81:530–42. doi: 10.1002/pros.24131
120. Tricoli JV, Winter DL, Hanlon AL, Raysor SL, Watkins-Bruner D, Pinover WH, et al. Racial differences in insulin-like growth factor binding protein-3 in men at increased risk of prostate cancer. Urology. (1999) 54:178–82. doi: 10.1016/s0090-4295(99)00129-6
121. Platz EA, Pollak MN, Rimm EB, Majeed N, Tao Y, Willett WC, et al. Racial variation in insulin-like growth factor-1 and binding protein-3 concentrations in middle-aged men. Cancer epidemiology Biomarkers prevention: Publ Am Assoc Cancer Research cosponsored by Am Soc Prev Oncol. (1999) 8:1107–10.
122. Greenlee RT, Murray T, Bolden S, Wingo PA. Cancer statistics, 2000. CA: Cancer J Clin. (2000) 50:7–33. doi: 10.3322/canjclin.50.1.7
123. Qian F, Huo D. Circulating insulin-like growth factor-1 and risk of total and 19 site-specific cancers: cohort study analyses from the UK biobank. Cancer Epidemiology Biomarkers Prev. (2020) 29:2332–42. doi: 10.1158/1055-9965.EPI-20-0743
124. Tatoud R. Insulin-like growth factor (IGF) network and prostate pathologies. Prostate Cancer prostatic Dis. (1999) 2:66–9. doi: 10.1038/sj.pcan.4500295
125. Matsushita M, Fujita K, Hatano K, De Velasco MA, Uemura H, Nonomura N. Connecting the dots between the gut–IGF-1–prostate axis: A role of IGF-1 in prostate carcinogenesis. Front Endocrinol. (2022) 13. doi: 10.3389/fendo.2022.852382
126. Hellawell GO, Turner GD, Davies DR, Poulsom R, Brewster SF, Macaulay VM. Expression of the type 1 insulin-like growth factor receptor is up-regulated in primary prostate cancer and commonly persists in metastatic disease. Cancer Res. (2002) 62:2942–50. doi: 10.1016/S1569-9056(02)80120-8
127. Krueckl SL, Sikes RA, Edlund NM, Bell RH, Hurtado-Coll A, Fazli L, et al. Increased insulin-like growth factor I receptor expression and signaling are components of androgen-independent progression in a lineage-derived prostate cancer progression model. Cancer Res. (2004) 64:8620–9. doi: 10.1158/0008-5472.Can-04-2446
128. Nickerson T, Chang F, Lorimer D, Smeekens SP, Sawyers CL, Pollak M. In vivo progression of LAPC-9 and LNCaP prostate cancer models to androgen independence is associated with increased expression of insulin-like growth factor I (IGF-I) and IGF-I receptor (IGF-IR). Cancer Res. (2001) 61:6276–80.
129. Sutherland BW, Knoblaugh SE, Kaplan-Lefko PJ, Wang F, Holzenberger M, Greenberg NM. Conditional deletion of insulin-like growth factor-I receptor in prostate epithelium. Cancer Res. (2008) 68:3495–504. doi: 10.1158/0008-5472.Can-07-6531
130. Matsushita M, Fujita K, Hayashi T, Kayama H, Motooka D, Hase H, et al. Gut microbiota–derived short-chain fatty acids promote prostate cancer growth via IGF1 signaling. Cancer Res. (2021) 81:4014–26. doi: 10.1158/0008-5472.CAN-20-4090
131. Saikali Z, Setya H, Singh G, Persad S. Role of IGF-1/IGF-1R in regulation of invasion in DU145 prostate cancer cells. Cancer Cell Int. (2008) 8:10. doi: 10.1186/1475-2867-8-10
132. Niu X-B, Fu G-B, Wang L, Ge X, Liu W-T, Wen Y-Y, et al. Insulin-like growth factor-I induces chemoresistence to docetaxel by inhibiting miR-143 in human prostate cancer. Oncotarget;. (2017) 8:107157. doi: 10.18632/oncotarget.v8i63
133. Plymate SR, Haugk K, Coleman I, Woodke L, Vessella R, Nelson P, et al. An antibody targeting the type I insulin-like growth factor receptor enhances the castration-induced response in androgen-dependent prostate cancer. Clin Cancer Res. (2007) 13:6429–39. doi: 10.1158/1078-0432.CCR-07-0648
134. Fan W, Yanase T, Morinaga H, Okabe T, Nomura M, Daitoku H, et al. Insulin-like growth factor 1/insulin signaling activates androgen signaling through direct interactions of foxo1 with androgen receptor *. J Biol Chem. (2007) 282:7329–38. doi: 10.1074/jbc.M610447200
135. Weyer-Czernilofsky U, Hofmann MH, Friedbichler K, Baumgartinger R, Adam PJ, Solca F, et al. Antitumor activity of the IGF-1/IGF-2–neutralizing antibody xentuzumab (BI 836845) in combination with enzalutamide in prostate cancer models. Mol Cancer Ther. (2020) 19:1059–69. doi: 10.1158/1535-7163.MCT-19-0378
136. Stattin P, Bylund A, Rinaldi S, Biessy C, Déchaud H, Stenman UH, et al. Plasma insulin-like growth factor-I, insulin-like growth factor-binding proteins, and prostate cancer risk: a prospective study. J Natl Cancer Institute. (2000) 92:1910–7. doi: 10.1093/jnci/92.23.1910
137. Horoszewicz JS, Leong SS, Kawinski E, Karr JP, Rosenthal H, Chu TM, et al. LNCaP model of human prostatic carcinoma. Cancer Res. (1983) 43:1809–18.
138. Ngo TH, Barnard RJ, Cohen P, Freedland S, Tran C, deGregorio F, et al. Effect of isocaloric low-fat diet on human LAPC-4 prostate cancer xenografts in severe combined immunodeficient mice and the insulin-like growth factor axis1. Clin Cancer Res. (2003) 9:2734–43.
139. Fahrenholtz CD, Beltran PJ, Burnstein KL. Targeting IGF-IR with ganitumab inhibits tumorigenesis and increases durability of response to androgen-deprivation therapy in VCaP prostate cancer xenografts. Mol Cancer Ther. (2013) 12:394–404. doi: 10.1158/1535-7163.MCT-12-0648
140. Nordstrand A, Bergstrom SH, Thysell E, Bovinder-Ylitalo E, Lerner UH, Widmark A, et al. Inhibition of the insulin-like growth factor-1 receptor potentiates acute effects of castration in a rat model for prostate cancer growth in bone. Clin Exp Metastasis. (2017) 34:261–71. doi: 10.1007/s10585-017-9848-8
141. Barata P, Cooney M, Tyler A, Wright J, Dreicer R, Garcia JA. A phase 2 study of OSI-906 (linsitinib, an insulin-like growth factor receptor-1 inhibitor) in patients with asymptomatic or mildly symptomatic (non-opioid requiring) metastatic castrate resistant prostate cancer (CRPC). Investigational New Drugs. (2018) 36:451–7. doi: 10.1007/s10637-018-0574-0
142. Saleh SAK, Adly HM, Nassir AM. Combination of insulin-like growth factor-1, IGF binding protein-3, chromogranin A and prostate specific antigen can improve the detection of prostate cancer. J Cancer Metastasis Treat. (2017) 3:82–9. doi: 10.20517/2394-4722.2017.20
143. Massoner P, Colleselli D, Matscheski A, Pircher H, Geley S, Jansen Dürr P, et al. Novel mechanism of IGF-binding protein-3 action on prostate cancer cells: inhibition of proliferation, adhesion, and motility. Endocrine-Related Cancer. (2009) 16:795–808. doi: 10.1677/ERC-08-0175
144. Kolb AD, Bussard KM. The bone extracellular matrix as an ideal milieu for cancer cell metastases. Cancers. (2019) 11:1020. doi: 10.3390/cancers11071020
145. Siech C, Rutz J, Maxeiner S, Grein T, Sonnenburg M, Tsaur I, et al. Insulin-like growth factor-1 influences prostate cancer cell growth and invasion through an integrin α3, α5, αV, and β1 dependent mechanism. Cancers. (2022) 14:363. doi: 10.3390/cancers14020363
146. Moore MG, Wetterau LA, Francis MJ, Peehl DM, Cohen P. Novel stimulatory role for insulin-like growth factor binding protein-2 in prostate cancer cells. Int J Cancer. (2003) 105:14–9. doi: 10.1002/ijc.11015
147. Inman BA, Harel F, Audet JF, Meyer F, Douville P, Fradet Y, et al. Insulin-like growth factor binding protein 2: an androgen-dependent predictor of prostate cancer survival. Eur Urol. (2005) 47:695–702. doi: 10.1016/j.eururo.2004.12.015
148. Putz T, Culig Z, Eder IE, Nessler-Menardi C, Bartsch G, Grunicke H, et al. Epidermal growth factor (EGF) receptor blockade inhibits the action of EGF, insulin-like growth factor I, and a protein kinase A activator on the mitogen-activated protein kinase pathway in prostate cancer cell lines. Cancer Res. (1999) 59:227–33.
149. Monget P, Oxvig C. PAPP-A and the IGF system. Annales d’endocrinologie. (2016) 77:90–6. doi: 10.1016/j.ando.2016.04.015
150. Bischof P, Megevand M. Pregnancy-associated plasma protein-A concentrations in men with testicular and prostatic tumors. Arch Androl. (1986) 16:155–60. doi: 10.3109/01485018608986936
151. Boldt HB, Conover CA. Overexpression of pregnancy-associated plasma protein-A in ovarian cancer cells promotes tumor growth in vivo. Endocrinology. (2011) 152:1470–8. doi: 10.1210/en.2010-1095
152. Bulut I, Coskun A, Ciftci A, Cetinkaya E, Altiay G, Caglar T, et al. Relationship between pregnancy-associated plasma protein-A and lung cancer. Am J Med Sci. (2009) 337:241–4. doi: 10.1097/MAJ.0b013e31818967a3
153. Heitzeneder S, Sotillo E, Shern JF, Sindiri S, Xu P, Jones R, et al. Pregnancy-associated plasma protein-A (PAPP-A) in ewing sarcoma: role in tumor growth and immune evasion. J Natl Cancer Institute. (2019) 111:970–82. doi: 10.1093/jnci/djy209
154. Prithviraj P, Anaka M, McKeown SJ, Permezel M, Walkiewicz M, Cebon J, et al. Pregnancy associated plasma protein-A links pregnancy and melanoma progression by promoting cellular migration and invasion. Oncotarget. (2015) 6:15953–65. doi: 10.18632/oncotarget.3643
155. Ryan AJ, Napoletano S, Fitzpatrick PA, Currid CA, O’Sullivan NC, Harmey JH. Expression of a protease-resistant insulin-like growth factor-binding protein-4 inhibits tumour growth in a murine model of breast cancer. Br J Cancer. (2009) 101:278–86. doi: 10.1038/sj.bjc.6605141
156. Pollak M. The insulin and insulin-like growth factor receptor family in neoplasia: an update. Nat Rev Cancer. (2012) 12:159–69. doi: 10.1038/nrc3215
157. Tomlins SA, Mehra R, Rhodes DR, Cao X, Wang L, Dhanasekaran SM, et al. Integrative molecular concept modeling of prostate cancer progression. Nat Genet. (2007) 39:41–51. doi: 10.1038/ng1935
158. Zhang M, Smith EP, Kuroda H, Banach W, Chernausek SD, Fagin JA. Targeted expression of a protease-resistant IGFBP-4 mutant in smooth muscle of transgenic mice results in IGFBP-4 stabilization and smooth muscle hypotrophy*. J Biol Chem. (2002) 277:21285–90. doi: 10.1074/jbc.M112082200
159. Soh CL, McNeil K, Owczarek CM, Hardy MP, Fabri LJ, Pearse M, et al. Exogenous administration of protease-resistant, non-matrix-binding IGFBP-2 inhibits tumour growth in a murine model of breast cancer. Br J Cancer. (2014) 110:2855–64. doi: 10.1038/bjc.2014.232
160. Zhu S, Xu F, Zhang J, Ruan W, Lai M. Insulin-like growth factor binding protein-related protein 1 and cancer. Clinica Chimica Acta. (2014) 431:23–32. doi: 10.1016/j.cca.2014.01.037
161. Jiang W, Xiang C, Cazacu S, Brodie C, Mikkelsen T. Insulin-like growth factor binding protein 7 mediates glioma cell growth and migration. Neoplasia. (2008) 10:1335–42. doi: 10.1593/neo.08694
162. Evdokimova V, Tognon CE, Benatar T, Yang W, Krutikov K, Pollak M, et al. IGFBP7 binds to the IGF-1 receptor and blocks its activation by insulin-like growth factors. Sci Signaling. (2012) 5:ra92–ra. doi: 10.1126/scisignal.2003184
163. Cohen P, Peehl DM, Baker B, Liu F, Hintz RL, Rosenfeld RG. Insulin-like growth factor axis abnormalities in prostatic stromal cells from patients with benign prostatic hyperplasia. J Clin Endocrinol Metab. (1994) 79:1410–5. doi: 10.1210/jcem.79.5.7525636
164. Hwa V, Tomasini-Sprenger C, Bermejo AL, Rosenfeld RG, Plymate SR. Characterization of insulin-like growth factor-binding protein-related protein-1 in prostate cells. J Clin Endocrinol Metab. (1998) 83:4355–62. doi: 10.1210/jcem.83.12.5341
165. Mutaguchi K, Yasumoto H, Mita K, Matsubara A, Shiina H, Igawa M, et al. Restoration of insulin-like growth factor binding protein-related protein 1 has a tumor-suppressive activity through induction of apoptosis in human prostate cancer. Cancer Res. (2003) 63:7717–23.
166. López-Bermejo A, Buckway CK, Devi GR, Hwa V, Plymate SR, Oh Y, et al. Characterization of insulin-like growth factor-binding protein-related proteins (IGFBP-rPs) 1, 2, and 3 in human prostate epithelial cells: potential roles for IGFBP-rP1 and 2 in senescence of the prostatic epithelium. Endocrinology. (2000) 141:4072–80. doi: 10.1210/endo.141.11.7783
167. Pilarsky CP, Schmidt U, Eissrich C, Stade J, Froschermaier SE, Haase M, et al. Expression of the extracellular matrix signaling molecule Cyr61 is downregulated in prostate cancer. Prostate. (1998) 36:85–91. doi: 10.1002/(SICI)1097-0045(19980701)36:2<85::AID-PROS3>3.3.CO;2-X
168. Li L, Yu H, Schumacher F, Casey G, Witte JS. Relation of serum insulin-like growth factor-I (IGF-I) and IGF binding protein-3 to risk of prostate cancer (United States). Cancer Causes Control. (2003) 14:721–6. doi: 10.1023/A:1026383824791
169. Trojan L, Bode C, Weiss C, Mayer D, Grobholz R, Alken P, et al. IGF-II serum levels increase discrimination between benign prostatic hyperplasia and prostate cancer and improve the predictive value of PSA in clinical staging. Eur Urol. (2006) 49:286–92;discussion 92. doi: 10.1016/j.eururo.2005.08.022
170. Gori F, Hofbauer LC, Conover CA, Khosla S. Effects of androgens on the insulin-like growth factor system in an androgen-responsive human osteoblastic cell line. Endocrinology. (1999) 140:5579–86. doi: 10.1210/endo.140.12.7213
171. Zhang XH, Jin X, Malladi S, Zou Y, Wen YH, Brogi E, et al. Selection of bone metastasis seeds by mesenchymal signals in the primary tumor stroma. Cell. (2013) 154:1060–73. doi: 10.1016/j.cell.2013.07.036
172. van Golen CM, Schwab TS, Kim B, Soules ME, Su Oh S, Fung K, et al. Insulin-like growth factor-I receptor expression regulates neuroblastoma metastasis to bone. Cancer Res. (2006) 66:6570–8. doi: 10.1158/0008-5472.Can-05-1448
173. Heidegger I, Massoner P, Sampson N, Klocker H. The insulin-like growth factor (IGF) axis as an anticancer target in prostate cancer. Cancer Lett. (2015) 367:113–21. doi: 10.1016/j.canlet.2015.07.026
174. Goel HL, Fornaro M, Moro L, Teider N, Rhim JS, King M, et al. Selective modulation of type 1 insulin-like growth factor receptor signaling and functions by beta1 integrins. J Cell Biol. (2004) 166:407–18. doi: 10.1083/jcb.200403003
175. Damsky CH, Ilić D. Integrin signaling: it’s where the action is. Curr Opin Cell Biol. (2002) 14:594–602. doi: 10.1016/S0955-0674(02)00368-X
176. Fornaro M, Manes T, Languino LR. Integrins and prostate cancer metastases. Cancer metastasis Rev. (2001) 20:321–31. doi: 10.1023/a:1015547830323
177. Mauro L, Surmacz E. IGF-I receptor, cell-cell adhesion, tumour development and progression. J Mol Histol. (2004) 35:247–53. doi: 10.1023/b:hijo.0000032356.98363.a2
178. Maile LA, Badley-Clarke J, Clemmons DR. Structural analysis of the role of the beta 3 subunit of the alpha V beta 3 integrin in IGF-I signaling. J Cell Sci. (2001) 114:1417–25. doi: 10.1242/jcs.114.7.1417
179. Playford MP, Bicknell D, Bodmer WF, Macaulay VM. Insulin-like growth factor 1 regulates the location, stability, and transcriptional activity of beta-catenin. Proc Natl Acad Sci U.S.A. (2000) 97:12103–8. doi: 10.1073/pnas.210394297
180. Rubin J, Ackert-Bicknell CL, Zhu L, Fan X, Murphy TC, Nanes MS, et al. IGF-I regulates osteoprotegerin (OPG) and receptor activator of nuclear factor-kappaB ligand in vitro and OPG in vivo. J Clin Endocrinol Metab. (2002) 87:4273–9. doi: 10.1210/jc.2002-020656
181. Fizazi K, Yang J, Peleg S, Sikes CR, Kreimann EL, Daliani D, et al. Prostate cancer cells-osteoblast interaction shifts expression of growth/survival-related genes in prostate cancer and reduces expression of osteoprotegerin in osteoblasts. Clin Cancer research: an Off J Am Assoc Cancer Res. (2003) 9:2587–97.
182. Rubin J, Chung LW, Fan X, Zhu L, Murphy TC, Nanes MS, et al. Prostate carcinoma cells that have resided in bone have an upregulated IGF-I axis. Prostate. (2004) 58:41–9. doi: 10.1002/pros.10299
183. Simonet WS, Lacey DL, Dunstan CR, Kelley M, Chang MS, Lüthy R, et al. Osteoprotegerin: a novel secreted protein involved in the regulation of bone density. Cell. (1997) 89:309–19. doi: 10.1016/s0092-8674(00)80209-3
184. Goya M, Miyamoto S, Nagai K, Ohki Y, Nakamura K, Shitara K, et al. Growth inhibition of human prostate cancer cells in human adult bone implanted into nonobese diabetic/severe combined immunodeficient mice by a ligand-specific antibody to human insulin-like growth factors. Cancer Res. (2004) 64:6252–8. doi: 10.1158/0008-5472.Can-04-0919
185. Li S, Wang N, Brodt P. Metastatic cells can escape the proapoptotic effects of TNF-α through increased autocrine IL-6/STAT3 signaling. Cancer Res. (2012) 72:865–75. doi: 10.1158/0008-5472.Can-11-1357
186. Tang Y, Zhang D, Fallavollita L, Brodt P. Vascular endothelial growth factor C expression and lymph node metastasis are regulated by the type I insulin-like growth factor receptor. Cancer Res. (2003) 63:1166–71.
187. Tsurusaki T, Kanda S, Sakai H, Kanetake H, Saito Y, Alitalo K, et al. Vascular endothelial growth factor-C expression in human prostatic carcinoma and its relationship to lymph node metastasis. Br J Cancer. (1999) 80:309–13. doi: 10.1038/sj.bjc.6690356
188. Li J, Wang E, Rinaldo F, Datta K. Upregulation of VEGF-C by androgen depletion: the involvement of IGF-IR-FOXO pathway. Oncogene. (2005) 24:5510–20. doi: 10.1038/sj.onc.1208693
189. Hiraga T, Myoui A, Hashimoto N, Sasaki A, Hata K, Morita Y, et al. Bone-derived IGF mediates crosstalk between bone and breast cancer cells in bony metastases. Cancer Res. (2012) 72:4238–49. doi: 10.1158/0008-5472.Can-11-3061
190. Shafi AA, Yen AE, Weigel NL. Androgen receptors in hormone-dependent and castration-resistant prostate cancer. Pharmacol Ther. (2013) 140:223–38. doi: 10.1016/j.pharmthera.2013.07.003
191. Yuan X, Cai C, Chen S, Chen S, Yu Z, Balk SP. Androgen receptor functions in castration-resistant prostate cancer and mechanisms of resistance to new agents targeting the androgen axis. Oncogene. (2014) 33:2815–25. doi: 10.1038/onc.2013.235
192. Titus MA, Schell MJ, Lih FB, Tomer KB, Mohler JL. Testosterone and dihydrotestosterone tissue levels in recurrent prostate cancer. Clin Cancer research: an Off J Am Assoc Cancer Res. (2005) 11:4653–7. doi: 10.1158/1078-0432.Ccr-05-0525
193. Montgomery RB, Mostaghel EA, Vessella R, Hess DL, Kalhorn TF, Higano CS, et al. Maintenance of intratumoral androgens in metastatic prostate cancer: a mechanism for castration-resistant tumor growth. Cancer Res. (2008) 68:4447–54. doi: 10.1158/0008-5472.Can-08-0249
194. So A, Gleave M, Hurtado-Col A, Nelson C. Mechanisms of the development of androgen independence in prostate cancer. World J Urol. (2005) 23:1–9. doi: 10.1007/s00345-004-0473-1
195. Miyake H, Pollak M, Gleave ME. Castration-induced up-regulation of insulin-like growth factor binding protein-5 potentiates insulin-like growth factor-I activity and accelerates progression to androgen independence in prostate cancer models. Cancer Res. (2000) 60:3058–64.
196. Gurumurthy S, Vasudevan KM, Rangnekar VM. Regulation of apoptosis in prostate cancer. Cancer metastasis Rev. (2001) 20:225–43. doi: 10.1023/a:1015583310759
197. Grossmann ME, Huang H, Tindall DJ. Androgen receptor signaling in androgen-refractory prostate cancer. J Natl Cancer Institute. (2001) 93:1687–97. doi: 10.1093/jnci/93.22.1687
198. Feldman BJ, Feldman D. The development of androgen-independent prostate cancer. Nat Rev Cancer. (2001) 1:34–45. doi: 10.1038/35094009
199. Orio F Jr., Térouanne B, Georget V, Lumbroso S, Avances C, Siatka C, et al. Potential action of IGF-1 and EGF on androgen receptor nuclear transfer and transactivation in normal and cancer human prostate cell lines. Mol Cell Endocrinol. (2002) 198:105–14. doi: 10.1016/S0303-7207(02)00374-X
200. Plymate SR, Bae VL, Maddison L, Quinn LS, Ware JL. Reexpression of the type 1 insulin-like growth factor receptor inhibits the Malignant phenotype of simian virus 40 T antigen immortalized human prostate epithelial cells. Endocrinology. (1997) 138:1728–35. doi: 10.1210/endo.138.4.5071
201. Kaplan PJ, Mohan S, Cohen P, Foster BA, Greenberg NM. The insulin-like growth factor axis and prostate cancer: lessons from the transgenic adenocarcinoma of mouse prostate (TRAMP) model. Cancer Res. (1999) 59:2203–9.
202. Cooke DW, Bankert LA, Roberts CT Jr., LeRoith D, Casella SJ. Analysis of the human type I insulin-like growth factor receptor promoter region. Biochem Biophys Res Commun. (1991) 177:1113–20. doi: 10.1016/0006-291X(91)90654-P
203. Schayek H, Haugk K, Sun S, True LD, Plymate SR, Werner H. Tumor suppressor BRCA1 is expressed in prostate cancer and controls insulin-like growth factor I receptor (IGF-IR) gene transcription in an androgen receptor-dependent manner. Clin Cancer research: an Off J Am Assoc Cancer Res. (2009) 15:1558–65. doi: 10.1158/1078-0432.Ccr-08-1440
204. Moretti RM, Marelli MM, Dondi D, Poletti A, Martini L, Motta M, et al. Luteinizing hormone-releasing hormone agonists interfere with the stimulatory actions of epidermal growth factor in human prostatic cancer cell lines, LNCaP and DU 145. J Clin Endocrinol Metab. (1996) 81:3930–7. doi: 10.1210/jcem.81.11.8923840
205. Pandini G, Mineo R, Frasca F, Roberts CT Jr., Marcelli M, Vigneri R, et al. Androgens up-regulate the insulin-like growth factor-I receptor in prostate cancer cells. Cancer Res. (2005) 65:1849–57. doi: 10.1158/0008-5472.Can-04-1837
206. Nelson JB, Chan-Tack K, Hedican SP, Magnuson SR, Opgenorth TJ, Bova GS, et al. Endothelin-1 production and decreased endothelin B receptor expression in advanced prostate cancer. Cancer Res. (1996) 56:663–8.
207. Sehgal I, Powers S, Huntley B, Powis G, Pittelkow M, Maihle NJ. Neurotensin is an autocrine trophic factor stimulated by androgen withdrawal in human prostate cancer. Proc Natl Acad Sci U.S.A. (1994) 91:4673–7. doi: 10.1073/pnas.91.11.4673
208. Markwalder R, Reubi JC. Gastrin-releasing peptide receptors in the human prostate: relation to neoplastic transformation. Cancer Res. (1999) 59:1152–9.
209. Nickerson T, Pollak M, Huynh H. Castration-induced apoptosis in the rat ventral prostate is associated with increased expression of genes encoding insulin-like growth factor binding proteins 2,3,4 and 5. Endocrinology. (1998) 139:807–10. doi: 10.1210/endo.139.2.5912
210. Thomas LN, Cohen P, Douglas RC, Lazier C, Rittmaster RS. Insulin-like growth factor binding protein 5 is associated with involution of the ventral prostate in castrated and finasteride-treated rats. Prostate. (1998) 35:273–8. doi: 10.1002/(SICI)1097-0045(19980601)35:4<273::AID-PROS6>3.3.CO;2-J
211. Kiyama S, Morrison K, Zellweger T, Akbari M, Cox M, Yu D, et al. Castration-induced increases in insulin-like growth factor-binding protein 2 promotes proliferation of androgen-independent human prostate LNCaP tumors. Cancer Res. (2003) 63:3575–84.
212. Schulz P, Link TA, Chaudhuri L, Fittler F. Role of the mitochondrial bc1-complex in the cytotoxic action of diethylstilbestrol-diphosphate toward prostatic carcinoma cells. Cancer Res. (1990) 50:5008–12.
213. Koike H, Ito K, Takezawa Y, Oyama T, Yamanaka H, Suzuki K. Insulin-like growth factor binding protein-6 inhibits prostate cancer cell proliferation: implication for anticancer effect of diethylstilbestrol in hormone refractory prostate cancer. Br J Cancer. (2005) 92:1538–44. doi: 10.1038/sj.bjc.6602520
214. Lubik AA, Gunter JH, Hendy SC, Locke JA, Adomat HH, Thompson V, et al. Insulin increases de novo steroidogenesis in prostate cancer cells. Cancer Res. (2011) 71:5754–64. doi: 10.1158/0008-5472.CAN-10-2470
215. Haluska P, Carboni JM, Loegering DA, Lee FY, Wittman M, Saulnier MG, et al. In vitro and in vivo antitumor effects of the dual insulin-like growth factor-I/insulin receptor inhibitor, BMS-554417. Cancer Res. (2006) 66:362–71. doi: 10.1158/0008-5472.Can-05-1107
216. Ji QS, Mulvihill MJ, Rosenfeld-Franklin M, Cooke A, Feng L, Mak G, et al. A novel, potent, and selective insulin-like growth factor-I receptor kinase inhibitor blocks insulin-like growth factor-I receptor signaling in vitro and inhibits insulin-like growth factor-I receptor dependent tumor growth in vivo. Mol Cancer Ther. (2007) 6:2158–67. doi: 10.1158/1535-7163.Mct-07-0070
217. Lima GA, Corrêa LL, Gabrich R, Miranda LC, Gadelha MR. IGF-I, insulin and prostate cancer. Arquivos brasileiros endocrinologia e metabologia. (2009) 53:969–75. doi: 10.1590/s0004-27302009000800010
218. Sciarra A, Panebianco V, Ciccariello M, Salciccia S, Lisi D, Osimani M, et al. Magnetic resonance spectroscopic imaging (1H-MRSI) and dynamic contrast-enhanced magnetic resonance (DCE-MRI): pattern changes from inflammation to prostate cancer. Cancer Invest. (2010) 28:424–32. doi: 10.3109/07357900903287048
219. Schally AV, Varga JL. Antagonistic analogs of growth hormone-releasing hormone: new potential antitumor agents. Trends Endocrinol metabolism: TEM. (1999) 10:383–91. doi: 10.1016/S1043-2760(99)00209-X
220. Lin SL, Lin CY, Lee W, Teng CF, Shyu WC, Jeng LB. Mini review: molecular interpretation of the IGF/IGF-1R axis in cancer treatment and stem cells-based therapy in regenerative medicine. Int J Mol Sci. (2022) 23:11781. doi: 10.3390/ijms231911781
221. McHugh DJ, Chudow J, DeNunzio M, Slovin SF, Danila DC, Morris MJ, et al. A phase I trial of IGF-1R inhibitor cixutumumab and mTOR inhibitor temsirolimus in metastatic castration-resistant prostate cancer. Clin Genitourin Cancer. (2020) 18:171–8.e2. doi: 10.1016/j.clgc.2019.10.013
222. Qu X, Wu Z, Dong W, Zhang T, Wang L, Pang Z, et al. Update of IGF-1 receptor inhibitor (ganitumab, dalotuzumab, cixutumumab, teprotumumab and figitumumab) effects on cancer therapy. Oncotarget. (2017) 8:29501–18. doi: 10.18632/oncotarget.15704
223. Dean JP, Sprenger CC, Wan J, Haugk K, Ellis WJ, Lin DW, et al. Response of the insulin-like growth factor (IGF) system to IGF-IR inhibition and androgen deprivation in a neoadjuvant prostate cancer trial: effects of obesity and androgen deprivation. J Clin Endocrinol Metab. (2013) 98:E820–8. doi: 10.1210/jc.2012-3856
224. de Bono JS, Piulats JM, Pandha HS, Petrylak DP, Saad F, Aparicio LM, et al. Phase II randomized study of figitumumab plus docetaxel and docetaxel alone with crossover for metastatic castration-resistant prostate cancer. Clin Cancer research: an Off J Am Assoc Cancer Res. (2014) 20:1925–34. doi: 10.1158/1078-0432.CCR-13-1869
225. Molife LR, Fong PC, Paccagnella L, Reid AH, Shaw HM, Vidal L, et al. The insulin-like growth factor-I receptor inhibitor figitumumab (CP-751,871) in combination with docetaxel in patients with advanced solid tumours: results of a phase Ib dose-escalation, open-label study. Br J Cancer. (2010) 103:332–9. doi: 10.1038/sj.bjc.6605767
226. Wong RL, Duong MT, Tangen CM, Agarwal N, Cheng HH, Vogelzang NJ, et al. Survival outcomes and risk group validation from SWOG S0925: a randomized phase II study of cixutumumab in new metastatic hormone-sensitive prostate cancer. Prostate Cancer prostatic Dis. (2020) 23:486–93. doi: 10.1038/s41391-020-0210-x
227. Yu EY, Li H, Higano CS, Agarwal N, Pal SK, Alva A, et al. SWOG S0925: A randomized phase II study of androgen deprivation combined with cixutumumab versus androgen deprivation alone in patients with new metastatic hormone-sensitive prostate cancer. J Clin Oncol. (2015) 33:1601–8. doi: 10.1200/JCO.2014.59.4127
228. Hussain M, Rathkopf D, Liu G, Armstrong A, Kelly WK, Ferrari A, et al. A randomised non-comparative phase II trial of cixutumumab (IMC-A12) or ramucirumab (IMC-1121B) plus mitoxantrone and prednisone in men with metastatic docetaxel-pretreated castration-resistant prostate cancer. Eur J Cancer. (2015) 51:1714–24. doi: 10.1016/j.ejca.2015.05.019
229. Wu JD, Haugk K, Coleman I, Woodke L, Vessella R, Nelson P, et al. Combined in vivo effect of A12, a type 1 insulin-like growth factor receptor antibody, and docetaxel against prostate cancer tumors. Clin Cancer research: an Off J Am Assoc Cancer Res. (2006) 12:6153–60. doi: 10.1158/1078-0432.CCR-06-0443
230. Hewish M, Chau I, Cunningham D. Insulin-like growth factor 1 receptor targeted therapeutics: novel compounds and novel treatment strategies for cancer medicine. Recent patents anti-cancer Drug Discovery. (2009) 4:54–72. doi: 10.2174/157489209787002515
231. de Bono J, Lin CC, Chen LT, Corral J, Michalarea V, Rihawi K, et al. Two first-in-human studies of xentuzumab, a humanised insulin-like growth factor (IGF)-neutralising antibody, in patients with advanced solid tumours. Br J Cancer. (2020) 122:1324–32. doi: 10.1038/s41416-020-0774-1
232. Macaulay VM, Lord S, Hussain S, Maroto JP, Jones RH, Climent MA, et al. A Phase Ib/II study of IGF-neutralising antibody xentuzumab with enzalutamide in metastatic castration-resistant prostate cancer. Br J Cancer. (2023) 129:965–73. doi: 10.1038/s41416-023-02380-1
233. Weyer-Czernilofsky U, Hofmann MH, Friedbichler K, Baumgartinger R, Adam PJ, Solca F, et al. Antitumor activity of the IGF-1/IGF-2-neutralizing antibody xentuzumab (BI 836845) in combination with enzalutamide in prostate cancer models. Mol Cancer Ther. (2020) 19:1059–69. doi: 10.1158/1535-7163.MCT-19-0378
234. Aleksic T, Gray N, Wu X, Rieunier G, Osher E, Mills J, et al. Nuclear IGF1R interacts with regulatory regions of chromatin to promote RNA polymerase II recruitment and gene expression associated with advanced tumor stage. Cancer Res. (2018) 78:3497–509. doi: 10.1158/0008-5472.CAN-17-3498
235. Zhong H, Fazenbaker C, Breen S, Chen C, Huang J, Morehouse C, et al. MEDI-573, alone or in combination with mammalian target of rapamycin inhibitors, targets the insulin-like growth factor pathway in sarcomas. Mol Cancer Ther. (2014) 13:2662–73. doi: 10.1158/1535-7163.MCT-14-0144
236. Lyles RDZ, Martinez MJ, Sherman B, Schurer S, Burnstein KL. Automation, live-cell imaging, and endpoint cell viability for prostate cancer drug screens. PloS One. (2023) 18:e0287126. doi: 10.1371/journal.pone.0287126
237. Li W, Wang Z, Wang L, He X, Wang G, Liu H, et al. Effectiveness of inhibitor rapamycin, saracatinib, linsitinib and JNJ-38877605 against human prostate cancer cells. Int J Clin Exp Med. (2015) 8:6563–7.
238. Kubat Oktem E, Demir U, Yazar M, Arga KY. Three candidate anticancer drugs were repositioned by integrative analysis of the transcriptomes of species with different regenerative abilities after injury. Comput Biol Chem. (2023) 106:107934. doi: 10.1016/j.compbiolchem.2023.107934
Keywords: prostate cancer, IGF-I, IGF-II, IGF-1 receptor, IGF-2 receptor
Citation: Elemam NM, Hotait HY, Saleh MA, El-Huneidi W and Talaat IM (2024) Insulin-like growth factor family and prostate cancer: new insights and emerging opportunities. Front. Endocrinol. 15:1396192. doi: 10.3389/fendo.2024.1396192
Received: 05 March 2024; Accepted: 14 May 2024;
Published: 30 May 2024.
Edited by:
Vittoria Rago, University of Calabria, ItalyReviewed by:
Xiaoqiang Wang, City of Hope, United StatesCopyright © 2024 Elemam, Hotait, Saleh, El-Huneidi and Talaat. This is an open-access article distributed under the terms of the Creative Commons Attribution License (CC BY). The use, distribution or reproduction in other forums is permitted, provided the original author(s) and the copyright owner(s) are credited and that the original publication in this journal is cited, in accordance with accepted academic practice. No use, distribution or reproduction is permitted which does not comply with these terms.
*Correspondence: Iman M. Talaat, aXRhbGFhdEBzaGFyamFoLmFjLmFl; Waseem El-Huneidi, d2VsaHVuZWlkaUBzaGFyamFoLmFjLmFl
Disclaimer: All claims expressed in this article are solely those of the authors and do not necessarily represent those of their affiliated organizations, or those of the publisher, the editors and the reviewers. Any product that may be evaluated in this article or claim that may be made by its manufacturer is not guaranteed or endorsed by the publisher.
Research integrity at Frontiers
Learn more about the work of our research integrity team to safeguard the quality of each article we publish.