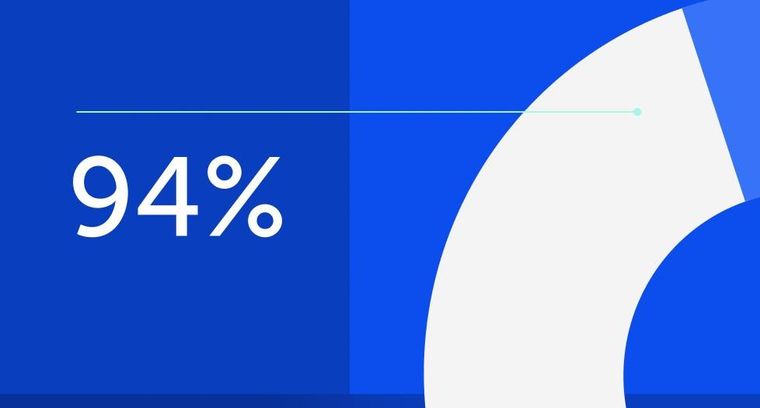
94% of researchers rate our articles as excellent or good
Learn more about the work of our research integrity team to safeguard the quality of each article we publish.
Find out more
ORIGINAL RESEARCH article
Front. Endocrinol., 29 April 2024
Sec. Neuroendocrine Science
Volume 15 - 2024 | https://doi.org/10.3389/fendo.2024.1387964
This article is part of the Research TopicOxytocin and Metabolic Dysregulation: From Pathophysiology to PharmacotherapyView all 6 articles
The high prevalence of obesity has become a pressing global public health problem and there exists a strong association between increased BMI and mortality at a BMI of 25 kg/m2 or higher. The prevalence of obesity is higher among middle-aged adults than among younger groups and the combination of aging and obesity exacerbate systemic inflammation. Increased inflammatory cytokines such as interleukin 6 and tumor necrosis factor alpha (TNFα) are hallmarks of obesity, and promote the secretion of hepatic C-reactive protein (CRP) which further induces systematic inflammation. The neuropeptide oxytocin has been shown to have anti-obesity and anti-inflammation effects, and also suppress sweet-tasting carbohydrate consumption in mammals. Previously, we have shown that the Japanese herbal medicine Kamikihito (KKT), which is used to treat neuropsychological stress disorders in Japan, functions as an oxytocin receptors agonist. In the present study, we further investigated the effect of KKT on body weight (BW), food intake, inflammation, and sweet preferences in middle-aged obese mice. KKT oral administration for 12 days decreased the expression of pro-inflammatory cytokines in the liver, and the plasma CRP and TNFα levels in obese mice. The effect of KKT administration was found to be different between male and female mice. In the absence of sucrose, KKT administration decreased food intake only in male mice. However, while having access to a 30% sucrose solution, both BW and food intake was decreased by KKT administration in male and female mice; but sucrose intake was decreased in female mice alone. In addition, KKT administration decreased sucrose intake in oxytocin deficient lean mice, but not in the WT lean mice. The present study demonstrates that KKT ameliorates chronic inflammation, which is strongly associated with aging and obesity, and decreases food intake in male mice as well as sucrose intake in female mice; in an oxytocin receptor dependent manner.
The high prevalence of overweight and obesity is a global problem (1) and there exists a strong association between increased BMI (over 25 kg/m2) and mortality (2). The prevalence of obesity is higher among middle-aged adults (40-59 yrs) than among younger groups (20-39 yrs) (3). It is known that obesity with an accumulation of visceral fat mass induces chronic inflammation in the brain, adipose tissue, and the liver, ultimately leading to development of further obesity, steatohepatitis, and arteriosclerosis (4). Clinically, high-sensitive C-reactive protein (hs-CRP) is known to be a marker of inflammation and is predictive for myocardial infarction, stroke, and the aggravation of metabolic syndrome (5). C-reactive protein (CRP) is synthesized and secreted in hepatocytes and its transcription is mainly under IL-6 control (6). Increased plasma concentrations of CRP is also associated with aging (7). It has also been reported that both obese and elderly individuals have higher levels of circulating inflammatory markers (8). The combination of obesity and aging is additive leading to chronic, low-grade systemic inflammation (9). Accumulated abdominal adipose tissue secretes pro-inflammatory cytokines, such as IL-1β, TNFα and IL-6 and induces low-grade inflammation (10). Furthermore, this obesity induced pro-inflammatory cytokines lead to liver inflammation, which eventually culminates in non-alcoholic fatty liver disease (NAFLD) (10). NAFLD may lead to further complications such as hepatic steatosis, non-alcoholic steatohepatitis (NASH), fibrosis, cirrhosis, liver failure, and hepatocarcinoma (11).
Oxytocin is a neuropeptide composed of nine amino acids, derived from hypothalamic paraventricular nucleus and supraoptic nucleus. Originally discovered as the hormone that promotes parturition and milk ejection during lactation in females (12), recent work demonstrates a number of new physiological functions for this hormone peptide, such as increasing trust and bonding in humans (13), a decrease of stress and anxiety (14), and an anti-inflammatory effect on microglia (15).
Oxytocin and OXTR mediated signaling also has strong anti-obesity effects. Both OXTR- and oxytocin-deficient mice developed obesity, without changes in food intake and locomotor activity (16, 17), suggesting that the physiological role of oxytocin is to increase energy expenditure (18). On the other hand, oxytocin treatment has been shown to decrease food intake, body weight (BW), abdominal and subcutaneous fat mass, and improve insulin secretion in obese mice (19). The anti-obesity effects were further confirmed in both monkeys and humans (20, 21). Interestingly, oxytocin has been shown to preferentially suppresses the intake of sweet-tasting carbohydrates in mammals (22). This was further confirmed for sucrose intake in rodents and for fructose-sweetened beverage intake in monkeys (20, 23). It has been known that plasma oxytocin levels were decreased by aging (24), as well as obesity (25). Oxytocin plays an important role in muscle regeneration (24) and hepatic regeneration (26) in aged animals.
Obesity is associated with sarcopenia in aged adults, and weight loss induces further muscle mass loss (27). However, nasal oxytocin administration for an 8 week period significantly increased whole lean mass with a trend toward decreasing fat mass in older adults with sarcopenic obesity (27). Therefore, reduction of oxytocin levels may have a significant impact on aging related symptoms, and treatment by oxytocin has potential to improve these metabolic symptoms.
Kamikihito (KKT), a traditional herbal medicine composed of 14 crude drugs, is clinically used in Japan for the treatment of neuropsychological stress disorders including neurosis, amnesia, and insomnia. KKT has been reported to attenuate depressive-like behavior (28), and increase oxytocin secretion in cerebrospinal fluid in rats with acute stress (29). Our previous study clearly demonstrated that KKT activates oxytocin receptors (OXTR) and functions as an agonist (30). It is considered that KKT promotes oxytocin secretion by activating OXTR expressed in oxytocin neurons (30), and may alleviate neuropsychological stress disorders.
Thus, in the present study we examined whether KKT, as an oxytocin agonist, has effects on 1) BW and food intake in a diet-induced obese mouse model, 2) inflammation in adipose and liver tissue in obese mice, 3) sweet preferences in obese mice, and 4) sweet preference in lean oxytocin deficient (Oxt-/-) mice.
It is well established that the combination of obesity and aging accelerate systemic inflammation. Therefore, in this study, we specifically focused on these two factors, “age” and “obesity”, which are also prevalent in the typical patient cohort taking KKT supplements. In order to address both “age” and “obesity”, middle-aged diet-induced obese mice were used in this study. We avoided to use of geriatric mice (generally considered as aged over 96 weeks) due to additional, unrelated complications associated with advanced age. However, middle-aged mature mice (10-15 month) is a relevant model as age related body changes are in progress (31). According to previous work, 50-week-old mice would be the equivalent to 38-year-old humans, generally considered the time when aging related change starts (32). Also, the diet-induced obese mice used in this study corresponds to 46.7 years old in male mice, and 28.4-32.2 years old in female mice, respectively.
The vast majority of research published in the field of metabolism involve mice of a much younger age, typically between 12-25 weeks, and it is well known that the protein expression profiles vary drastically between the different stages of life (33). It is therefore essential that mechanistic studies on the systemic effects of drugs should be undertaken in murine models which recapitulate the typical physical state of patients taking the drug, and the contribution of age and body weight should be taken into account. In this study we report for the first time the effect of KKT administration on body weight, inflammation and sucrose preferences in diet-induced obese middle-aged mice.
Six-week-old male and female C57BL/6J mice were purchased from Japan SLC (Shizuoka, Japan). A high fat diet ([HFD]; HFD32; Clea, Osaka, Japan) was given for 54 weeks for male and 31 weeks for female mice. The HFD feeding protocol was adopted to induce severe obesity and to achieve an equivalent body weight between male and female mice. Initial BW were 55.8 ± 1.6 g and 56.2 ± 0.8 g in male and female mice, respectively with no significant differences (T = 0.21, df = 15, p > 0.05).
Oxytocin-deficient (Oxt-/-), female mice (35–50-week-old) generated by Nishimori et al., were used (34). Mice were fed a standard chow diet [SD] (CE2; Clea, Osaka, Japan). The percentages of kcalories from each ingredient were as follows: SD – protein 20.5%, fat 10.1%; and HFD – protein 20.1%, fat 56.7%.
The animals were kept on a 12-h light/dark cycle (7:00-19:00) with ad libitum access to water in individual cages. All experimental procedures and care of animals were carried out according to relevant guidelines and regulations, and were approved by the ethical committee of Fukushima Medical University for Genetic Experiments (Approval number: 306) and Animal Care and Use (Approval number: 2023004).
Seven days prior to the start of the experiment, animals were moved to individual cages and habituated for oral gavage. For the wild type (WT) obese mice experimental group, the diet was changed from HFD to SD after starting the experiment. The KKT (500 mg/kg/5ml) was dissolved in distilled water for oral administration. The dose of KKT was based on the prior studies of KKT (200–2000 mg/kg oral administration in mice or rats) (30, 35, 36). KKT (Lot No. 341006900) was kindly provided by Tsumura & Co (Tokyo, Japan). KKT is composed of 14 herbal components (Supplementary Table 1); Astragalus Root (3 g, Astragalus mongholicus Bunge), Bupleurum Root (3 g, Bupleurum falcatum L. or Bupleurum scorzonerifolium Willd.), Jujube Seed (3 g, Ziziphus jujuba Mill.), Atractylodes Lancea Rhizome (3 g, Atractylodes lancea (Thunb.) DC.), Ginseng (3 g, Panax ginseng C.A.Mey.), Poria Sclerotium (3 g, Erythrococca ulugurensis Radcl.-Sm.), Longan Aril (3 g, Dimocarpus longan Lour. or Dimocarpus longan subsp. longan), Polygala Root (2 g, Polygala tenuifolia Willd.), Gardenia Fruit (2 g, Gardenia jasminoides J.Ellis), Jujube (2 g, Ziziphus jujuba Mill.), Japanese Angelica Root (2 g, Angelica acutiloba (Siebold & Zucc.) Kitag. or Angelica acutiloba var. acutiloba), Glycyrrhiza (1 g, Glycyrrhiza uralensis Fisch. ex DC. or Glycyrrhiza glabra L.), Ginger (1 g, Panax ginseng C.A.Mey.), and Saussurea Root (1 g, Dolomiaea costus (Falc.) Kasana & A.K.Pandey). The plant names have been checked with “World Flora Online” (www.worldfloraonline.org) or MPNS (http://mpns.kew.org). Plant materials were authenticated by identification of external morphology and marker compounds (saikosaponin b2, geniposide, and glycyrrhizinic acid) for plant specimens according to the methods of the Japanese Pharmacopeia and company standards. This drug was prepared as a spray dried powder from a hot water extract (yield 15.6%). KKT was manufactured under strict scientific and quality control, and approved for ethical clinical use by the Ministry of Health, Labor, and Welfare of Japan.
BW and food intake were measured for six days under KKT administration. Seven days after starting the experiment, water intake and sucrose water (30% sucrose) intake were measured, as well as food intake and BW. The protocol for the WT obese mice is shown in Figure 1A, and that for the Oxt-/-mice is shown in Figure 1B.
Figure 1 The scheme of this study. (A) Six-week-old male and female C57BL/6J mice were fed a high fat diet (HFD); 54 weeks for the male mice and 31 weeks for the female mice. Wild type (WT) obese mice, food was changed from HFD to standard (SD) chow after starting experiment. Kamikihito (KKT: 500 mg/kg/5ml) for oral administration was dissolved in distilled water. Body weight (BW) and food intake were measured for six days under KKT administration. Seven days after starting the experiment, water intake and sucrose water (30% sucrose) were measured, as well as food intake and BW. (B) Oxt-/- female mice were fed SD chow for 35–42 weeks. KKT (500 mg/kg/5ml) for oral administration was dissolved in distilled water. BW and food intake were measured for six days under KKT administration. Seven days after starting the experiment, water intake and sucrose water (30% sucrose) were measured, as well as food intake and BW.
Thirteen days after starting the experiment, the WT obese male mice were anesthetized (10 ml/kg, intraperitoneal injection of a mixture of three anesthetic agents [composition; Medetomidine (0.003%, Domitor, Nippon Zenyaku Kogyo Co., Ltd., Koriyama, Japan), midazolam (0.04%, Dormicum, Astellas Pharma Inc., Tokyo, Japan), and butorphanol tartrate [(0.05%, Vetorphale, Meiji Seika Pharma Co., Ltd., Tokyo, Japan)] and blood, liver and mesenteric fat collected.
WT and Oxt-/- female mice (50 weeks) were anesthetized and brains were harvested. Brain tissues were sectioned (0.14 mm to -2.06 mm from bregma) and the hippocampus, hypothalamus and cerebral cortex containing amygdala were further dissected from sections.
Total RNA was isolated from the collected liver and mesenteric fat and each brain region using the RNeasy Mini Kit (QIAGEN, Hilden, Germany) and Monarch RNA Purification Columns (New England BioLabs Inc., MA). C-DNA synthesis was performed using an M-MLV (Thermo Fisher Scientific, MA), RNaseOUT Recombinant Ribonuclease Inhibitor (Thermo Fisher Scientific, MA), and dNTP (Agilent Technologies, TX). A quantitative RT-PCR assay was performed using the TB Green Premix Ex Taq II (Tli RNaseH Plus, Takara Bio Inc., Shiga, Japan). The cycling protocol was: initial denaturation at 95°C for 30 sec, then 35 cycles of 95°C for 5 sec, 56°C for 10 sec, then 72°C for 15 sec. Product accumulation was measured in real time and the mean cycle thresholds were determined. Expression levels of pro-inflammatory cytokines, anti-inflammatory cytokines and OXTR mRNA were calculated using the 2ΔΔCT method of relative quantification and normalized to the housekeeping gene GAPDH. The PCR primers were as follows: IL1β (NM_008361): Fw (GAAGATGGAAAAACGGTTTG), Rev (GTACCAGTTGGGGAACTCTGC), TNFα (NM_001278601): Fw(GCCTCTTCTCATTCCTGCTTG), Rev(CTGATGAGAGGGAGGCCATT), IL-6(NM_031168): Fw(AGACAAAGCCAGAGTCCTTCA), Rev(GGTCCTTAGCCACTCCTTCTG), TGFβ (NM_009369): Fw(GAGCTGCTTATCCCAGATTCA), Rev(GGCAGTGGAGACGTCAGATT), IL-10 (NM_010548): Fw(CAGAGCCACATGCTCCTAGA), Rev(GTCCAGCTGGTCCTTTGTTT), OXTR (NM_001081147): Fw(GCACGGGTCAGTAGTGTCAA), Rev(AAGCTTCTTTGGGCGCATTG), GAPDH (NM_001289726): Fw(TCCACTCACGGCAAATTCAACG), Rev(TAGACTCCACGACATACTCAGC).
Blood samples were collected in EDTA tubes and centrifuged at 3000 rpm for 10 min at 4°C. Plasma was collected and TNFα concentrations were measured using a mouse TNFα ELISA kit (430907, Biolegend, CA). Intra-assay and inter-assay variations were 3.8–4.2% and 1.7–3.0%, respectively. Plasma CRP concentrations were measured by mouse high-sensitive (hs)-CRP ELISA kit (KT-095, Kamiya Biomedical Company, WA). Intra-assay and inter-assay variations were under 10%.
All data are presented as mean ± SEM. Student’s t-test was used for two-group comparisons. Comparisons of the two groups at each sampling point were analyzed by repeated measures two-way (sampling day×treatment) ANOVA followed by Tukey’s multiple range test. All statistical tests were two-tailed, with P values of 0.05 considered statistically significant.
In order to identify the effect of KKT on standard chow feeding on obese mice, food intake and BW were measured for the first six days under standard chow in HFD induced obese mice as shown in the schematic (Figure 1A). As expected, significant over time changes in BW were observed in male and female mice, respectively (Figures 2A, B) (Male F12, 108 = 86.14, p < 0.01; Female F12, 72 = 69.48, p < 0.01; repeated measures two-way (sampling day × treatment) ANOVA). This is because swapping high preference HFD to low preference standard chow decreased overall energy intake.
Figure 2 KKT suppresses food intake in male, sucrose intake in female mice. (A–D) Body weight change (A, B) and food intake (C, D) during oral KKT administration in obese male (A, C) and obese female (B, D) mice. *P < 0.05, **P < 0.01, Repeated measures two-way ANOVA followed by Tukey’s multiple range test. (E–H) Water intake (E, F) and sucrose solution intake (G, H) during sucrose giving term in obese male (E, G) and obese female (F, H) mice. * P < 0.05, **P < 0.01, Repeated measures two-way ANOVA. followed by Tukey’s multiple range test. (I, J) The ratio of sucrose intake ([sucrose intake]/[water + sucrose intake] × 100) in obese male (I) and obese female (J) mice. *P < 0.05, **P < 0.01, Repeated measures two-way ANOVA followed by Tukey’s multiple range test. (A, C, E, G, I) n = 5, 6. (B, D, F, H, J) n = 4, 4.
KKT administered male mice showed larger decline of BW (Figure 2A) (F1, 108 = 100.56, p < 0.01) and food intake (Figure 2C) (F1, 99 = 38.13, p < 0.01) than those of control on day4 and day5 in the six days period of standard diet administration. However, decreased food intake was not observed for female mice (Figure 2D); therefore, the BW decrease caused by KKT administration under a standard diet was observed only in male mice (Figure 2A).
Next, in order to identify the effect of KKT on sucrose preference, control (water) and 30% sucrose solution were offered for another six days after standard chow administration. Although there were no differences in water intake between the control and KKT groups in both male and female mice (Figures 2E, F) (Male F1, 45 = 0.29, p > 0.05; Female F1, 30 = 5.27, p > 0.05), KKT administration induced a decrease in sucrose intake in female mice only (Figures 2G, H) (Male F1, 45 = 1.08, p > 0.05; Female F1, 30 = 24.57, p < 0.01). During this sucrose offered period, a decline of food intake (Figure 2D) (F1, 66 = 11.31, p < 0.01) and BW (Figure 2B) (F1, 72 = 25.78, p < 0.01) was observed in both male and female mice (Figures 2A, B). Analyses of percentage of sucrose intake ([sucrose intake]/[water + sucrose intake]), showed no changes in male mice (Figure 2I) (F1, 45 = 0.66, p > 0.05), while KKT administration significantly decreased this ratio in female mice (Figure 2J) (F1, 30 = 13.67, p < 0.01).
Previous results showed that oral KKT administration decreased food intake and BW during first 6 days in only male mice. The result indicates that the impacts of KKT on regulation of feeding and BW are larger in male mice on a standard chow diet. Therefore, we examined systemic inflammation and inflammatory cytokines expressions in liver and adipose tissue of male mice.
Consistent with previous reports, the expressions of IL-6 and OXTR mRNA were significantly increased, and TNFα tended to increase in mesenteric adipose tissues of mice after being fed a HFD (Supplementary Figures 1A, B).
Twelve days of oral administration of 500 mg/kg KKT in the HFD-fed mice significantly decreased plasma hs-CRP (Figure 3A; T = 2.26, df = 9, p < 0.05) and TNFα (Figure 3B; T = 2.83, df = 9, p < 0.05), compared with the control group. The hs-CRP were 5.58 ± 0.42 μg/ml in the control group and 4.06 ± 0.50 μg/ml in the KKT administered group. TNFα was 13.20 ± 2.86 pg/ml in the control group and 3.98 ± 1.80 pg/ml in the KKT administered group.
Figure 3 KKT suppresses pro-inflammatory cytokines. (A, B) Plasma high-sensitive C-reactive protein (hs-CRP) (A) and TNFα (B) concentration after KKT oral administration for 12 days in mice with HFD-induced obesity. * P < 0.05, unpaired t-test. n = 5, 6. (C–H) mRNA expression of pro-inflammatory cytokines (C-E), anti-inflammatory cytokines (F, G) and oxytocin receptors (OXTR) (H) in liver after KKT oral administration for 12 days in HFD induced obese mice. *P < 0.05, **P < 0.01, unpaired t-test. n = 4–6. (I–N) mRNA expression of pro-inflammatory cytokines (I-K), anti-inflammatory cytokines (L, M) and oxytocin receptors (OXTR) (N) in adipose tissues from mesenteric fat after KKT oral administration for 12 days in HFD induced obese mice. *P < 0.05, **P < 0.01, unpaired t-test. n = 5–6.
In addition, oral administration of KKT in the HFD-fed mice significantly decreased the expression of mRNA of pro-inflammatory cytokines, TNFα (1.0 ± 0.3 in control, 0.36 ± 0.07 in KKT) Figure 3C; T = 2.76, df = 9, p < 0.05), IL-1β (1.0 ± 0.14 in control, 0.5 ± 0.06 in KKT) (Figure 3D; T = 3.50, df = 9, p < 0.01) and IL-6 (1.0 ± 0.3 in control, 0.49 ± 0.05 in KKT) (Figure 3E; T = 2.80, df = 9, p < 0.05) mRNA expression in the liver. On the other hand, there were no significant differences in anti-inflammatory cytokines, TGFβ (1.0 ± 0.16 in control, 0.73 ± 0.08 in KKT) (Figure 3F; T = 1.66, df = 9, p > 0.05), IL-10 (1.0 ± 0.61 in control, 0.94 ± 0.23 in KKT) (Figure 3G; T = 0.2, df = 8, p > 0.05) and OXTR (1.0 ± 0.18 in control, 1.40 ± 0.41 in KKT) (Figure 3H; T = 1.06, df = 9, p > 0.05) mRNA expression between the control and KKT group liver tissue samples.
In the mesenteric adipose tissue, although mRNA of pro-inflammatory cytokines in the KKT group tended to decrease, there were no significant differences between the control and KKT groups (TNFα, 1.0 ± 0.37 in control, 0.56 ± 0.17 in KKT [Figure 3I; T = 1.20, df = 9, p = 0.29], IL-1β, 1.0 ± 0.13 in control, 0.46 ± 0.15 in KKT [Figure 3J; T = 1.10, df = 9, p = 0.30] and IL-6, 1.0 ± 0.52 in control, 0.33 ± 0.04 in KKT [Figure 3K; T = 1.67, df = 9, p = 0.13]). Similar with the liver tissue samples, there were no significant differences in anti-inflammatory cytokines and OXTR mRNA between the control and KKT groups (TGFβ, 1.0 ± 0.3 in control, 1.90 ± 0.32 in KKT [Figure 3L; T = 1.61, df = 9, p > 0.05], IL-10, 1.0 ± 0.30 in control, 0.71 ± 0.41 in KKT [Figure 3M; T = 0.5, df = 9, p > 0.05] and OXTR, 1.0 ± 0.17 in control, 0.98 ± 0.06 in KKT [Figure 3N; T = 0.10, df = 8, p > 0.05]).
These results indicate that oral administration KKT decreases both systemic inflammation, as is reflected by decreased CRP and TNFα plasma concentrations, and decreased expression of the pro-inflammatory cytokines TNFα, IL-1β and IL-6 in liver.
The results in Figure 2 showed that oral KKT administration decreased sucrose intake only in female mice. This result indicates that the impacts of KKT on regulation of sucrose intake are more robust in female mice, and thus further studies were performed on female mice alone. Previous work from our laboratory showed that KKT exerts its effect through the activation of OXTRs (26). Thus, in order to directly examine the relationship between OXTR signaling, KKT and sucrose preference, a similar experiment (Figure 1B) was performed in lean WT and Oxt-/- middle-aged female mice (35-42wks) on a SD. The initial BWs were 26.33 ± 1.2 g and 24.7 ± 0.34 g, in the WT and Oxt-/-mice, respectively, with no significant differences (T = 1.29, df = 8, p > 0.05).
Similar to the obese female mice, KKT administration did not affect BW in the lean WT and Oxt-/- female mice in the first six days (Figures 4A, B). However, KKT slightly but significantly decreased food intake in the Oxt-/- mice on day 2 and 8 of KKT administration (Figure 4D) (F 1,198 = 6.08, p < 0.05), but showed no significant differences in WT (Figure 4C) (F1, 66 = 2.37, p > 0.05). There were no differences in water intake in the lean WT and Oxt-/-lean mice with or without KKT treatment (Figures 4E, F) (WT F1, 30 = 0.01, p > 0.05; Oxt-/- F1, 90 = 2.01, p > 0.05). However, sucrose intake was decreased in KKT administered lean Oxt-/- mice (Figure 4H) (F1, 90 = 73.29, p < 0.01) while a slight increase was observed on day 1 of KKT administration in WT mice (Figure 4G) (F1,30 = 9.99, p < 0.01). Together with declined food and sucrose intake, BW was also decreased in the KKT-administered Oxt-/-mice (Figure 4B) (F1, 216 = 115.27, p < 0.01). Although there were no differences in % of sucrose intake between the control and KKT groups in the WT mice (Figure 4I) (F1. 30 = 2.06, p > 0.05), KKT significantly decreased % of sucrose intake in the Oxt-/- mice (Figure 4J) (F1, 90 = 19.84, p < 0.01). This result would support the idea that, in the absence of native oxytocin, KKT acts as an agonist of OXTR to exerts its sucrose preference suppressing effect in female.
Figure 4 KKT suppresses sucrose intake in Oxt-/- lean female mice. (A–D) Body weight change (A, B) and food intake (C, D) during oral KKT administration in lean WT (A, C) and lean Oxt-/- (B, D) mice. * P < 0.05, **P < 0.01, Repeated measures two-way ANOVA two-way ANOVA followed by Tukey’s multiple range test. (E–H) Water intake (E, F) and sucrose solution intake (G, H) during sucrose providing term in lean WT (E, G) and lean Oxt-/- female (F, H) mice. * P < 0.05, **P < 0.01, Repeated measures two-way ANOVA two-way ANOVA followed by Tukey’s multiple range test. (I, J) The ratio of sucrose intake ([sucrose intake]/[water + sucrose intake] × 100) in lean WT (I) and lean Oxt-/- female (J) mice. * P < 0.05, **P < 0.01, Repeated measures two-way ANOVA followed by Tukey’s multiple range test. (A, C, E, G, I) n = 4, 4. (B, D, F, H, J) n = 10, 10.
The expression of OXTR in the hypothalamus, hippocampus and cerebral cortex were examined in the WT and Oxt-/- female mice by qRT-PCR. OXTR mRNA expression in the cerebral cortex was significantly increased (T = 5.19, df = 4, p < 0.01) (Supplementary Figure 2C) and tended to increase in the hippocampus (T = 2.27, df = 4, p = 0.08) (Supplementary Figure 2B). There were no significant differences in the hypothalamus (T = 0.85, df = 4, p = 0.44) (Supplementary Figure 2A). These results would further suggest that the mechanism underpinning the observed decline of preference for sucrose induced by KKT administration in Oxt-/-mice is OXTR dependent, and mediated via the limbic system.
The present study demonstrated that KKT ameliorates inflammation induced by obesity, decreases food intake in obese middle-aged males, and sucrose intake in obese middle-aged females. The decline of food intake in males and sucrose intake in females most likely contributes to the reduction of BW observed for both sexes.
Obesity is strongly associated with metabolic syndrome, which includes abdominal obesity, insulin resistance, hypertension, and dyslipidemia (37). Obesity increases the prevalence of metabolic syndrome and raises the risk of cardiovascular diseases and type 2 diabetes (37). Obesity is typically characterized as the simple accumulation of adipose tissue but recent studies highlighted the contribution of chronic inflammation in adipocytes to pathology (10). It is now accepted that accumulated adipose tissue as the primary source of pro-inflammatory cytokines that induce systemic chronic inflammation (10). This obesity-induced systemic chronic inflammation leads to insulin resistance, diabetes and cardiovascular disease (4). This systemic inflammation is reflected in an increase of CRP that is synthesized and secreted in the liver, under the transcriptional control of IL-6 (6). In obese patients, it is not only CRP secretion, but also dietary factors such as the consumption of high calorie foods which contributes to non-alcoholic fatty liver with inflammation, cirrhosis, and ultimately hepatocellular carcinoma (38). Aging itself also leads to the induction of chronic systemic inflammation. The combination of obesity and aging can lead to further increase of inflammation (9) and the prevalence of obesity is typically higher among middle-aged adults (40-59 yrs) than among younger (20-39 yrs) groups (3).
In the present study, we demonstrate that KKT treatment decreased the plasma inflammation markers hs-CRP and TNFα as well as the expression of pro-inflammatory cytokines in the liver in middle-aged mice. The data suggests that KKT may reduce the production of CRP from the liver and suppress the systemic inflammation and development of fatty liver disease.
To the best of our knowledge, this paper is the first to report the anti-inflammatory effect of KKT. How KKT suppresses inflammation in liver and adipose tissue remains unclear. However, considering our previous report, which demonstrated the capability of KKT to activate OXTR (30), at least two mechanisms can be considered for its anti-inflammation mechanism; first, KKT directly affects hepatocytes and adipocytes; second, KKT affects macrophages within the liver and adipose tissues.
As for the first possible mechanism, it is based on previous reports showing that OXTR is expressed in both hepatocytes and adipocytes (26, 39). OXTR in hepatocytes contribute to the rejuvenation of the liver through activation of autophagy and regeneration of hepatocyte (26) while OXTR in adipocytes regulates lipolysis, and its activation reduces the size of adipocytes (39). Further supporting this work, our previous study also confirmed that chronic oxytocin treatment improves fatty liver and reduces the size of adipocytes (19). Considering these reports, KKT may suppress inflammation by rescuing damaged liver and adipose tissue.
As for the second possibility, KKT may have direct effects on macrophages. OXTR is reported to be expressed on macrophages (40) and is OXTR expression is increased following acute inflammation induced by the lipopolysaccharides (LPS). This increase of OXTR expression in macrophages is known to attenuate inflammatory responses (40).
Considering the decline of IL-6 mRNA expression in liver and plasma hs-CRP induced by KKT administration, the two mechanisms discussed above are both plausible. Our previous study (30) showed that KKT administration increased cytosolic Ca2+ in OXTR transfected HEK cells which were abolished in the presence of an OXTR antagonist. Furthermore, we identified 7 chemical components (rutin, ursolic acid, z-butylidenephtalide, sensyunolide-A, P-cymene, [6]-shogaol, [8]-shogaol) from 3 crude drugs (Zizyphi Fructus, Angelicae Acutilobae Radix, Zingiberis Rhizoma) in KKT which act as OXTR agonists. Therefore, these crude drugs may activate OXTR synergistically. However, since KKT is composed of 14 different crude drugs (Supplementary Table 1), different mechanisms independent from OXTR could be involved in attenuating inflammation in the liver and adipose tissue. Further studies, including the use of OXTR knockout mice, are therefore needed to better understand the underlying mechanisms of KKT effect.
As for the food and sucrose intake, there were sex differences in the effect of KKT administration. Male mice showed a decrease in food intake, but not in sucrose intake, while female mice showed a decrease in sucrose intake, but not in food intake. There are two mechanisms for food intake regulation. The first is the basal food intake, which plays a crucial role in matching caloric intake with energy expenditure, and is regulated in the medulla oblongata and the hypothalamus (41). The second mechanism is high palatable feeding, for example sucrose, which is regulated in the reward related brain region including the amygdala (42) and ventral tegmental area (43). Our data therefore would suggest that KKT mainly affects basal feeding regulation in male mice, and reward feeding regulation in female mice.
Because oxytocin preferentially suppresses intake of sweet-tasting carbohydrates in rats, mice, monkeys and humans (20, 23, 44–46), our present result suggests that KKT affect sucrose consumption via oxytocin signaling mediated mechanisms. Therefore, we examined the effect of KKT on food intake and sucrose intake by using lean Oxt-/- middle-aged female mice. Interestingly, KKT showed almost no effect on food intake and sucrose intake in lean WT female mice while it suppressed only sucrose intake in lean Oxt-/- female mice. Since KKT is an established OXTR agonist (30), it is therefore plausible that KKT suppressed sucrose intake via oxytocin induced signaling cascades. Interestingly, sucrose intake was significantly decreased for the first two days of access to sucrose in untreated lean Oxt-/-mice (Figure 3H) when compared to untreated lean WT littermates (Figure 3G), and untreated lean Oxt-/-mice only caught up with WT littermates on the third day of access to sucrose. This would imply that either taste or reward systems are impacted by the loss of oxytocin in these mice, and untreated lean Oxt-/-mice only developed a taste for sucrose over time. This sweet palatable food intake regulation by oxytocin is most likely mediated by OXTR expressed in reward-related brain regions (23). A previous human study showed that intranasally administered oxytocin reduced post-stress sweet snack intake in female participants (46). This mechanism may be explained through the actions of OXTR in dopamine neurons in reward related brain regions, such as the ventral tegmental area, nucleus accumbens by attenuating its excitatory input (23, 47).
It is possible that the sex differences for the effect of KKT may be caused by the different expression levels of OXTR in the brain (48). OXTR expression is reported to be higher in the lateral septum area in male than in female mice, but higher in the amygdala in female than male mice (48). Further studies about sex differences in OXTR expression and the effect of KKT are needed.
One of the main questions raised by the current study is that KKT administration is shown to be effective only in obese mice and its effect observed in lean Oxt-/- mice is surprising. This effect may be explained by the observed increase of OXTR expression levels in the cerebral cortex and hippocampus as a compensatory mechanism in response to the loss of functioning oxytocin. It is well established that obesity increases OXTR mRNA expression level in adipose tissue and the brain (49, 50), an observation further supports by our own finding that HFD feeding increases OXTR mRNA expression in adipose tissue (Supplementary Figure 1D). Similarly, OXTR mRNA was significantly increased in cerebral cortex, and tended to increase in hippocampus (p = 0.085) in middle-aged lean Oxt-/- mice (Supplementary Figures 2B, C). This is in line with the previous article, which reported the deficient of oxytocin lead to OXTR upregulation and/or increased sensitivity (51). Earlier studies reported decreased plasma oxytocin levels in obese, type2 diabetic, and metabolic syndrome patients and aged mice (24, 52, 53). Although we did not measure plasma oxytocin levels in our middle-aged obese model, the decline of plasma oxytocin levels is speculated. Because our middle-aged obese model contains the critical factors, “aging” and “obesity”, that decline of plasma oxytocin levels. Therefore, this phenomenon may be a compensatory mechanism to reflect the decline of oxytocin levels as a result of obesity, aging or complete loss of oxytocin in Oxt-/- mice. Previous reports and work from our laboratory showed that anti-obesity and the anorexigenic effect of oxytocin depended on the severity of obesity. Oxytocin effects on food intake and body weight are directly proportional to mice body weight (54). These results are in agreement with what we have shown here. Also, OXTR mRNA and protein expression are reported to be dramatically upregulated with LPS induced inflammation in macrophages (40). Since KKT moderately activates OXTR (30), the effect of KKT may be amplified when OXTR expression is upregulated in conditions such as inflammation, obesity, aging and under the condition that plasma oxytocin becoming low.
To date, there are no reports showing the effect of KKT on chronic inflammation. However, a recent clinical report showed that KKT attenuated LPS (Lipopolysaccharide) induced depressive behavior, such as loss of object exploration, social interaction deficit, and depressive-like behavior (55). However, it was considered that this effect of KKT was caused via attenuation of neural activity and not by attenuating inflammation (55). However, this paper did not investigate the effect of chronic inflammation. Recent studies highlighted that many diseases, including depression, cardiovascular disease, and cancer are strongly associated with the state of chronic inflammation (38, 56, 57).
An interesting extension of this study would be to test the effect of KKT on younger mice. The current study specifically investigates the effect of KKT administration on middle-aged mice, to reflect the age of the patients typically prescribed this herbal supplement. It would be of interest to see if these effects can also be seen in younger obese mice, which has a different metabolic profile compared to older mice. Since the combination of obesity and aging additively leads to systemic inflammation (9), it can be speculated that in younger obese mice, there could be less inflammation and less decline in plasma oxytocin levels. In this study, it was therefore important to consider the age of mice as a contributing factor as the effects of KKT on BW change and food intake, inflammation and sucrose preferences may be different in younger mice compared to middle-aged mice.
Furthermore, a decline of BW gain in both male and female mice in the KKT treated group was observed. The possible explanation of decreasing BW gain can be considered to be due to the increase in energy expenditure and thermogenesis, as well as decline of food or sucrose intake. Systemic oxytocin treatment increased energy expenditure (19) and oxytocin KO mice show decreased energy expenditure and thermogenesis (16, 58). Thus, the effects of KKT on thermogenesis should be considered as a possible contributing factor in this study.
In summary, the results of the present study show the inhibitory effect of KKT administration on both appetite and chronic inflammation in middle-aged obese mice. Since KKT can regulate inflammatory cytokines secretion, KKT may have a clinical advantage in the treatment of dietary and aging-related chronic inflammation disorders including obesity, steatohepatitis, as well as a treatment of neuropsychological stress disorders.
The original contributions presented in the study are included in the article/Supplementary Material. Further inquiries can be directed to the corresponding authors.
The animal study was approved by Ethical Committee of Fukushima Medical University for Genetic Experiments and Animal Care and Use. The study was conducted in accordance with the local legislation and institutional requirements.
YM: Writing – review & editing, Writing – original draft, Validation, Supervision, Project administration, Methodology, Investigation, Funding acquisition, Formal analysis. SY: Writing – review & editing, Methodology, Investigation, Formal analysis. MY: Writing – review & editing, Methodology, Investigation. SM: Writing – review & editing, Supervision. TO: Writing – review & editing, Supervision, Investigation. HO: Writing – review & editing. KM: Writing – review & editing. SH: Writing – review & editing, Resources. KN: Writing – review & editing, Resources. MA: Writing – review & editing, Supervision, Formal analysis. Hd: Writing – review & editing, Writing – original draft, Supervision. KS: Writing – review & editing, Writing – original draft, Supervision, Funding acquisition.
The author(s) declare financial support was received for the research, authorship, and/or publication of this article. This work was supported by a Grant-Aid for Scientific Research (C) (18K08483, 22K11755 to YM, 26461366 to KS) and research grant from Tsumura & Co. The funders were not involved in the study design, collection, analysis, interpretation of data, the writing of this article or the decision to submit it for publication.
The authors thank Tsumura Co. for kindly providing KKT. The authors also thank Rie Ohashi for her technical support.
HO and KM were employed by Tsumura & Co.
The remaining authors declare that the research was conducted in the absence of any commercial or financial relationships that could be construed as a potential conflict of interest.
The author(s) declared that they were an editorial board member of Frontiers, at the time of submission. This had no impact on the peer review process and the final decision.
All claims expressed in this article are solely those of the authors and do not necessarily represent those of their affiliated organizations, or those of the publisher, the editors and the reviewers. Any product that may be evaluated in this article, or claim that may be made by its manufacturer, is not guaranteed or endorsed by the publisher.
The Supplementary Material for this article can be found online at: https://www.frontiersin.org/articles/10.3389/fendo.2024.1387964/full#supplementary-material
ANOVA, analysis of variance; BW, body weight; CRP, C-reactive protein; hs-CRP, high-sensitive C-reactive protein; IL-1β, interleukin 1β; IL-6, interleukin 6; IL-10, interleukin 10; KKT, kamikihito; LPS, lipopolysaccharide; NAFLD, non-alcoholic fatty liver disease; NASH, non-alcoholic steatohepatitis; OXTR, oxytocin receptor; SEM, standard Error of the Mean; TGFβ, transforming Growth Factor-β; TNFα, tumor necrosis factor α; WT, wild type.
1. Morgen CS, Sørensen TI. Obesity: global trends in the prevalence of overweight and obesity. Nat Rev Endocrinol. (2014) 10:513–4. doi: 10.1038/nrendo.2014.124
2. Bhaskaran K, Dos-Santos-Silva I, Leon DA, Douglas IJ, Smeeth L. Association of BMI with overall and cause-specific mortality: a population-based cohort study of 3·6 million adults in the UK. Lancet Diabetes Endocrinol. (2018) 6:944–53. doi: 10.1016/S2213-8587(18)30288-2
3. Ogden CL, Carroll MD, Kit BK, Flegal KM. Prevalence of obesity among adults: United States, 2011-2012. NCHS Data Brief. (2013) 131:1–8.
4. Wen X, Zhang B, Wu B, Xiao H, Li Z, Li R, et al. Signaling pathways in obesity: mechanisms and therapeutic interventions. Signal Transduct Target Ther. (2022) 7:298. doi: 10.1038/s41392-022-01149-x
5. Bassuk SS, Rifai N, Ridker PM. High-sensitivity C-reactive protein: clinical importance. Curr Probl Cardiol. (2004) 29:439–93. doi: 10.1016/S0146-2806(04)00074-X
6. Zhang S, Liu Q, Wang J, Harnish DC. Suppression of interleukin-6-induced C-reactive protein expression by FXR agonists. Biochem Biophys Res Commun. (2009) 379:476–9. doi: 10.1016/j.bbrc.2008.12.117
7. Gao D, Ni X, Fang S, Wang Z, Jiao J, Liu D, et al. Exploration for the reference interval of C-reactive protein in the Chinese longevity people over 90 years of age. Diabetes Metab Syndr. (2023) 17:102817. doi: 10.1016/j.dsx.2023.102817
8. Oishi Y, Manabe I. Macrophages in age-related chronic inflammatory diseases. NPJ Aging Mech Dis. (2016) 2:16018. doi: 10.1038/npjamd.2016.18
9. Qu L, Matz AJ, Karlinsey K, Cao Z, Vella AT, Zhou B. Macrophages at the crossroad of meta-inflammation and inflammaging. Genes (Basel). (2022) 13:2074. doi: 10.3390/genes13112074
10. Bai Y, Sun Q. Macrophage recruitment in obese adipose tissue. Obes Rev. (2015) 16:127–36. doi: 10.1111/obr.12242
11. Carvalho-Gontijo R, Han C, Zhang L, Zhang V, Hosseini M, Mekeel K, et al. Metabolic injury of hepatocytes promotes progression of NAFLD and AALD. Semin Liver Dis. (2022) 42:233–49. doi: 10.1055/s-0042-1755316
12. Dale HH. On some physiological actions of ergot. J Physiol. (1906) 34:163–206. doi: 10.1113/jphysiol.1906.sp001148
13. Kosfeld M, Heinrichs M, Zak PJ, Fischbacher U, Fehr E. Oxytocin increases trust in humans. Nature. (2005) 435:673–76. doi: 10.1038/nature03701
14. McCall C, Singer T. The animal and human neuroendocrinology of social cognition, motivation and behavior. Nat Neurosci. (2012) 15:681–8. doi: 10.1038/nn.3084
15. Inoue T, Yamakage H, Tanaka M, Kusakabe T, Shimatsu A, Satoh-Asahara N. Oxytocin suppresses inflammatory responses induced by lipopolysaccharide through inhibition of the eIF-2-ATF4 pathway in mouse microglia. Cells. (2019) 8:527. doi: 10.3390/cells8060527
16. Takayanagi Y, Kasahara Y, Onaka T, Takahashi N, Kawada T, Nishimori K. Oxytocin receptor-deficient mice developed late-onset obesity. Neuroreport. (2008) 19:951–5. doi: 10.1097/WNR.0b013e3283021ca9
17. Camerino C. Low sympathetic tone and obese phenotype in oxytocin-deficient mice. Obes (Silver Spring). (2009) 17:980–4. doi: 10.1038/oby.2009.12
18. Camerino C. The new frontier in oxytocin physiology: the oxytonic contraction. Int J Mol Sci. (2020) 21:5144. doi: 10.3390/ijms21145144
19. Maejima Y, Iwasaki Y, Yamahara Y, Kodaira M, Sedbazar U, Yada T. Peripheral oxytocin treatment ameliorates obesity by reducing food intake and visceral fat mass. Aging (Albany NY). (2011) 3:1169–77. doi: 10.18632/aging.100408
20. Blevins JE, Graham JL, Morton GJ, Bales KL, Schwartz MW, Baskin DG, et al. Chronic oxytocin administration inhibits food intake, increases energy expenditure, and produces weight loss in fructose-fed obese rhesus monkeys. Am J Physiol Regul Integr Comp Physiol. (2015) 308:R431–8. doi: 10.1152/ajpregu.00441.2014
21. Zhang H, Wu C, Chen Q, Chen X, Xu Z, Wu J, et al. Treatment of obesity and diabetes using oxytocin or analogs in patients and mouse models. PloS One. (2013) 8:e61477. doi: 10.1371/journal.pone.0061477
22. Leng G, Sabatier N. Oxytocin - The sweet hormone? Trends Endocrinol Metab. (2017) 28:365–76. doi: 10.1016/j.tem.2017.02.007
23. Herisson FM, Waas JR, Fredriksson R, Schiöth HB, Levine AS, Olszewski PK. Oxytocin acting in the nucleus accumbens core decreases food intake. J Neuroendocrinol. (2016) 28. doi: 10.1111/jne.12381
24. Elabd C, Cousin W, Upadhyayula P, Chen RY, Chooljian MS, Li J, et al. Oxytocin is an age-specific circulating hormone that is necessary for muscle maintenance and regeneration. Nat Commun. (2014) 5:4082. doi: 10.1038/ncomms5082
25. Zhang G, Cai D. Circadian intervention of obesity development via resting-stage feeding manipulation or oxytocin treatment. Am J Physiol Endocrinol Metab. (2011) 301:E1004–12. doi: 10.1152/ajpendo.00196.2011
26. Luo D, Jin B, Zhai X, Li J, Liu C, Guo W, et al. Oxytocin promotes hepatic regeneration in elderly mice. iScience. (2021) 24:102125. doi: 10.1016/j.isci.2021.102125
27. Espinoza SE, Lee JL, Wang CP, Ganapathy V, MacCarthy D, Pascucci C, et al. Intranasal oxytocin improves lean muscle mass and lowers LDL cholesterol in older adults with sarcopenic obesity: A pilot randomized controlled trial. J Am Med Dir Assoc. (2021) 22:1877–82.e2. doi: 10.1016/j.jamda.2021.04.015
28. Adachi N, Sakhri FZ, Ikemoto H, Ohashi Y, Kato M, Inoue T, et al. Kamikihito rescued depressive-like behaviors and hippocampus neurogenesis in chronic restraint stress rats. J Tradit Complement Med. (2021) 12:172–9. doi: 10.1016/j.jtcme.2021.08.001
29. Tsukada M, Ikemoto H, Lee XP, Takaki T, Tsuchiya N, Mizuno K, et al. Kamikihito, a traditional Japanese Kampo medicine, increases the secretion of oxytocin in rats with acute stress. J Ethnopharmacol. (2021) 276:114218. doi: 10.1016/j.jep.2021.114218
30. Maejima Y, Horita S, Yokota S, Ono T, Proks P, Yoshida-Komiya H, et al. Identification of oxytocin receptor activating chemical components from traditional Japanese medicines. J Food Drug Anal. (2021) 29:653–75. doi: 10.38212/2224-6614.3381
31. Flurkey K, Currer JM, Harrison DE. The Mouse in Biomedical Research. 2nd ed. New York: Elsevier (2007).
32. Dutta S, Sengupta P. Men and mice: Relating their ages. Life Sci. (2016) 152:244–8. doi: 10.1016/j.lfs.2015.10.025
33. Pann P, de Angelis MH, Prehn C, Adamski J. Mouse age matters: how age affects the murine plasma metabolome. Metabolites. (2020) 10:472. doi: 10.3390/metabo10110472
34. Nishimori K, Young LJ, Guo Q, Wang Z, Insel TR, Matzuk MM. Oxytocin is required for nursing but is not essential for parturition or reproductive behavior. Proc Natl Acad Sci U.S.A. (1996) 93:11699–704. doi: 10.1073/pnas.93.21.11699
35. Tohda C, Nakada R, Urano T, Okonogi A, Kuboyama T. Kamikihi-to (KKT) rescues axonal and synaptic degeneration associated with memory impairment in a mouse model of Alzheimer’s disease, 5XFAD. Int J Neurosci. (2011) 121:641–8. doi: 10.3109/00207454.2011.602809
36. Watari H, Shimada Y, Tohda C. New treatment for Alzheimer’s disease, Kamikihito, reverses amyloid-b-induced progression of tau phosphorylation and axonal atrophy. Evid Based Complement Alternat Med. (2014) 2014:706487. doi: 10.1155/2014/706487
37. Després JP, Lemieux I. Abdominal obesity and metabolic syndrome. Nature. (2006) 444:881–7. doi: 10.1038/nature05488
38. Zheng J, Zhao L, Dong J, Chen H, Li D, Zhang X, et al. The role of dietary factors in nonalcoholic fatty liver disease to hepatocellular carcinoma progression: A systematic review. Clin Nutr. (2022) 41:2295–307. doi: 10.1016/j.clnu.2022.08.018
39. Assinder SJ, Boumelhem BB. Oxytocin stimulates lipolysis, prostaglandin E 2 synthesis, and leptin secretion in 3T3-L1 adipocytes. Mol Cell Endocrinol. (2021) 534:111381. doi: 10.1016/j.mce.2021.111381
40. Szeto A, Sun-Suslow N, Mendez AJ, Hernandez RI, Wagner KV, McCabe PM. Regulation of the macrophage oxytocin receptor in response to inflammation. Am J Physiol Endocrinol Metab. (2017) 312:E183–9. doi: 10.1152/ajpendo.00346.2016
41. Cheng W, Gordian D, Ludwig MQ, Pers TH, Seeley RJ, Myers MG Jr. Hindbrain circuits in the control of eating behavior and energy balance. Nat Metab. (2022) 4:826–35. doi: 10.1038/s42255-022-00606-9
42. Izadi MS, Radahmadi M. Overview of the central amygdala role in feeding behavior. Br J Nutr. (2022) 127:953–60. doi: 10.1017/S0007114521002312
43. Baik JH. Dopaminergic control of the feeding circuit. Endocrinol Metab (Seoul). (2021) 36:229–39. doi: 10.3803/EnM.2021.979
44. Sclafani A, Rinaman L, Vollmer RR, Amico JA. Oxytocin knockout mice demonstrate enhanced intake of sweet and nonsweet carbohydrate solutions. Am J Physiol Regul Integr Comp Physiol. (2007) 292:R1828–33. doi: 10.1152/ajpregu.00826.2006
45. Ott V, Finlayson G, Lehnert H, Heitmann B, Heinrichs M, Born J, et al. Oxytocin reduces reward-driven food intake in humans. Diabetes. (2013) 62:3418–25. doi: 10.2337/db13-0663
46. Burmester V, Gibson EL, Butler G, Bailey A, Terry P. Oxytocin reduces post-stress sweet snack intake in women without attenuating salivary cortisol. Physiol Behav. (2019) 212:112704. doi: 10.1016/j.physbeh.2019.112704
47. Xiao L, Priest MF, Kozorovitskiy Y. Oxytocin functions as a spatiotemporal filter for excitatory synaptic inputs to VTA dopamine neurons. Elife. (2018) 7:e33892. doi: 10.7554/eLife.33892
48. Sharma K, LeBlanc R, Haque M, Nishimori K, Reid MM, Teruyama R. Sexually dimorphic oxytocin receptor-expressing neurons in the preoptic area of the mouse brain. PloS One. (2019) 14:e0219784. doi: 10.1371/journal.pone.0219784
49. Gajdosechova L, Krskova K, Segarra AB, Spolcova A, Suski M, Olszanecki R, et al. Hypooxytocinaemia in obese Zucker rats relates to oxytocin degradation in liver and adipose tissue. J Endocrinol. (2014) 220:333–43. doi: 10.1530/JOE-13-0417
50. Watanabe S, Wei FY, Matsunaga T, Matsunaga N, Kaitsuka T, Tomizawa K. Oxytocin protects against stress-induced cell death in murine pancreatic β-cells. Sci Rep. (2016) 6:25185. doi: 10.1038/srep25185
51. Ragnauth AK, Goodwillie A, Brewer C, Muglia LJ, Pfaff DW, Kow LM. Vasopressin stimulates ventromedial hypothalamic neurons via oxytocin receptors in oxytocin gene knockout male and female mice. Neuroendocrinology. (2004) 80:92–9. doi: 10.1159/000081844
52. Qian W, Zhu T, Tang B, Yu S, Hu H, Sun W, et al. Decreased circulating levels of oxytocin in obesity and newly diagnosed type 2 diabetic patients. J Clin Endocrinol Metab. (2014) 99:4683–9. doi: 10.1210/jc.2014-2206
53. Yuan G, Qian W, Pan R, Jia J, Jiang D, Yang Q, et al. Reduced circulating oxytocin and High-Molecular-Weight adiponectin are risk factors for metabolic syndrome. Endocr J. (2016) 63:655–62. doi: 10.1507/endocrj.EJ16-0078
54. Maejima Y, Aoyama M, Sakamoto K, Jojima T, Aso Y, Takasu K, et al. Impact of sex, fat distribution and initial body weight on oxytocin’s body weight regulation. Sci Rep. (2017) 7:8599. doi: 10.1038/s41598-017-09318-7
55. Araki R, Nishida S, Hiraki Y, Li F, Matsumoto K, Yabe T. Kamikihito ameliorates Lipopolysaccharide-induced sickness behavior via attenuating neural activation, but not Inflammation, in the hypothalamic paraventricular nucleus and central nucleus of the amygdala in mice. Biol Pharm Bull. (2016) 39:289–94. doi: 10.1248/bpb.b15-00707
56. Wang H, He Y, Sun Z, Ren S, Liu M, Wang G, et al. Microglia in depression: an overview of microglia in the pathogenesis and treatment of depression. J Neuroinflamm. (2022) 19:132. doi: 10.1186/s12974-022-02492-0
57. Maierean S, Webb R, Banach M, Mazidi M. The role of inflammation and the possibilities of inflammation reduction to prevent cardiovascular events. Eur Heart J Open. (2022) 2:oeac039. doi: 10.1093/ehjopen/oeac039
Keywords: high fat diet, inflammatory cytokine, Kamikihito, sucrose preference, traditional Japanese medicine, oxytocin receptor, oxytocin
Citation: Maejima Y, Yokota S, Yamachi M, Misaka S, Ono T, Oizumi H, Mizuno K, Hidema S, Nishimori K, Aoyama M, de Wet H and Shimomura K (2024) Traditional Japanese medicine Kamikihito ameliorates sucrose preference, chronic inflammation and obesity induced by a high fat diet in middle-aged mice. Front. Endocrinol. 15:1387964. doi: 10.3389/fendo.2024.1387964
Received: 19 February 2024; Accepted: 09 April 2024;
Published: 29 April 2024.
Edited by:
James Ernest Blevins, United States Department of Veterans Affairs, United StatesReviewed by:
Taka-aki Koshimizu Jichi Medical University, JapanCopyright © 2024 Maejima, Yokota, Yamachi, Misaka, Ono, Oizumi, Mizuno, Hidema, Nishimori, Aoyama, de Wet and Shimomura. This is an open-access article distributed under the terms of the Creative Commons Attribution License (CC BY). The use, distribution or reproduction in other forums is permitted, provided the original author(s) and the copyright owner(s) are credited and that the original publication in this journal is cited, in accordance with accepted academic practice. No use, distribution or reproduction is permitted which does not comply with these terms.
*Correspondence: Yuko Maejima, bWFlamltYXlAZm11LmFjLmpw; Kenju Shimomura, c2hpbW9tdXJAZm11LmFjLmpw
Disclaimer: All claims expressed in this article are solely those of the authors and do not necessarily represent those of their affiliated organizations, or those of the publisher, the editors and the reviewers. Any product that may be evaluated in this article or claim that may be made by its manufacturer is not guaranteed or endorsed by the publisher.
Research integrity at Frontiers
Learn more about the work of our research integrity team to safeguard the quality of each article we publish.