- 1Zhang Zhongjing College of Chinese Medicine, Henan Key Laboratory of Zhang Zhongjing’s Formulas for Immunoregulation, Nanyang Institute of Technology, Nanyang, Henan, China
- 2School of Medicine, Zhengzhou University of Industrial Technology, Xinzheng, Henan, China
- 3The First Clinical College, Shandong University of Traditional Chinese Medicine, Jinan, Shandong, China
The expression of BDNF in both neuronal and non-neuronal cells is influenced by various stimuli, including prenatal developmental factors and postnatal conditions such as estrogens, dietary habits, and lifestyle factors like obesity, blood pressure, and aging. Central BDNF plays a crucial role in modulating how target tissues respond to these stimuli, influencing the pathogenesis of hypertension, mitigating obesity, and protecting neurons from aging. Thus, BDNF serves as a dynamic mediator of environmental influences, reflecting an individual's unique history of exposure. Estrogens, on the other hand, regulate various processes to maintain overall physiological well-being. Through nuclear estrogen receptors (ERα, ERβ) and the membrane estrogen receptor (GPER1), estrogens modulate transcriptional processes and signaling events that regulate the expression of target genes, such as ERα, components of the renin-angiotensin system (RAS), and hormone-sensitive lipase. Estrogens are instrumental in maintaining the set point for blood pressure and energy balance. BDNF and estrogens work cooperatively to prevent obesity by favoring lipolysis, and counteractively regulate blood pressure to adapt to the environment. Estrogen deficiency leads to menopause in women with low central BDNF level. This review delves into the complex mechanisms involving BDNF and estrogen, especially in the context of hypertension and obesity, particularly among postmenopausal women. The insights gained aim to inform the development of comprehensive therapeutic strategies for these prevalent syndromes affecting approximately 68% of adults.
1 Introduction
Brain-derived neurotrophic factor (BDNF) is highly expressed in the brain and moderately expressed in the heart, lungs, and kidneys. Numerous investigations confirm that BDNF has extensive roles by binding to its specific receptor, tropomyosin-related kinase receptor B (TrkB). This binding leads to the autophosphorylation of tyrosine residues in TrkB, initiating multiple signaling cascades, including rat sarcoma (RAS)-mitogen-activated protein kinases (MAPK) pathway, the phosphatidylinositol 3-kinase (PI3K)-Akt pathway, and the phospholipase C (PLC)-Ca2+ pathway (1–3). The MAPK pathway promotes neuronal differentiation and growth, the PI3K-Akt pathway is essential for cell survival, and PLCγ activation leads to the production of inositol 1,4,5 trisphosphate (IP3) and diacylglycerol (DAG), which subsequently activate Ca2+/calmodulin-dependent protein kinases and protein kinase C (PKC) pathways respectively (4). Physiologically, BDNF is well-known for its essential role in various neuronal processes during prenatal development, growth, maintenance, and the plasticity of the nervous system. Moreover, it also exerts non-neuronal effects on normal physiology and has been implicated in the pathogenesis of obesity and hypertension (5).
Estrogens, primarily produced in the follicular granulosa cells in premenopausal women and the stromal cells of adipose tissue in postmenopausal women, exert their effects by binding to specific receptors—the nuclear estrogen receptors (ERα, ERβ) and the membrane estrogen receptor (GPER1). These receptors activate transcriptional processes either as coregulators or through signaling pathways involving G protein activation and the cross-activation of MAPK, PI3K-Akt, and PKC, ultimately regulating gene expression and/or enzyme activity (6–8). Genes regulated by estrogen, known as estrogen-responsive genes, include BDNF (9), renin (10), estrogen receptor α (ERα) (11), angiotensinogen (12), hormone-sensitive lipase (HSL), proadipogenic genes such as peroxisome proliferator-activated receptor γ (PPARγ), steroid receptor coactivator-1 (SRC-1), and CREB-binding protein (CBP) (13, 14), as well as adipogenic genes like fatty acid synthase (FASN) (15). Estrogens are vital for both reproductive and non-reproductive functions (16), significantly influencing sexually dimorphic traits and renin expression through ERα (10). Dysregulation or deficiency of estrogen, particularly in postmenopausal women, can lead to conditions such as hypertension and obesity, common symptoms associated with menopause (17, 18).
BDNF and estrogens demonstrate multifaceted interactions that influence a range of physiological processes (4, 9, 19). At the cellular level, estrogens promote BDNF expression through the ERα-mediated classic transcriptional pathway in regions such as the hippocampus, amygdala, frontal cortex, dentate gyrus and hypothalamus, subsequently activating MAPKs, PI3K, and PKC pathways (20–22). However, this induction varies across different areas (21), with some studies reporting a decrease in the hippocampus (22, 23). In adipose tissue, the ratio of Bdnf/TrkB (tropomyosin receptor kinase B) expression is higher in female mice than in male mice (24). In addition, estrogens and BDNF may converge to promote the expression of neuropeptide Y (NPY) in hippocampal neurons through the aforementioned pathways (20). BDNF/TrkB signaling activation is essential for ligand-independent ERα activation (25) and is required for the long-term genomic actions of 17β-estradiol on dendritic spine plasticity (26). At the physiological level, their interdependent relationship is supported by evidence showing the fluctuation of BDNF levels during the ovarian cycle in women (19, 27) and in animal models (28). Conversely, reductions in estrogen and BDNF levels have been reported in patients with Parkinson's disease, Alzheimer's disease (AD) (21), postmenopausal women (19), ovariectomized (OVX) mouse models (29, 30), and ER-deficient mouse models (31). Estrogen deficiency is linked to obesity in over 43% of menopausal women (32), characterized by a central reduction of BDNF levels, while plasma BDNF remains unaffected (33, 34). It is also associated with hypertension in 19% of premenopausal women, 44% of perimenopausal women, and 75% of postmenopausal women aged 65 to 74 (35). Additionally, lower plasma BDNF levels are associated with significantly poorer memory performance (36). Although plasma BDNF is believed to influence blood pressure regulation, studies have produced conflicting results: some report elevated BDNF levels in cases of hypertension (1), while others indicate reduced levels (37).
Physiologically, BDNF is recognized as an anti-obesity molecule, while estrogens promote lipolysis and help prevent obesity. Furthermore, BDNF can contribute to increased blood pressure, whereas estrogens aid in maintaining blood pressure within a healthy range. Both BDNF and estrogens play critical roles in the development of hypertension and obesity, particularly in the postmenopausal context. These conditions are significant global public health concerns, affecting approximately 68% of the adults (1, 17, 38). This review examines recent advances in understanding the interaction between BDNF and estrogen in the context of hypertension and obesity. It aims to identify effective therapeutic strategies that leverage BDNF and estrogen, focusing on the timing and selection of appropriate diets or medications.
2 BDNF is a key mediator in activity-dependent processes, playing a crucial role from embryogenesis through aging
BDNF is expressed in various tissues, including both CNS and non-CNS organs such as the liver, lungs, kidneys, fat pads, and reproductive tissues. As a target-derived factor, BDNF plays diverse roles in numerous physiological processes, influencing blood pressure, body mass, learning, memory, cognitive development (5), and notably, appetite and metabolic control (39–42). It also has significant contributions to the cardiovascular health (1, 18, 39, 43). BDNF expression begins as early as the 11th to 12th day of embryogenesis in rats and mice, coinciding with prenatal programming, and increases with the onset of neurogenesis and heightened neuronal activity during development (44). Additionally, BDNF expression in target organs and tissues can be enhanced by exposure to various substances, including alcohol (45, 46), cocaine (47–49), exercise (50), high-fat diet (51, 52), low-level of ozone (O3) (53), lead (54), cigarette or cannabis smoke (55, 56), and drugs like valproate (57) in rodents or humans. Conversely, prenatal BDNF expression is downregulated by factors such as viral infection (50) or other stressors, including depression and estrogen deficiency (54). Importantly, BDNF plays a critical role in transmitting drug-induced phenotypes to subsequent generations, as observed in women with exposures to alcohol (10, 11), cocaine (12, 13), exercise (14, 15) and high-fat diet (16, 17).
Postnatally, central expression of BDNF significantly increases, influenced by various factors, including estrogens (9), high salt intake (58), angiotensin II or aldosterone (1, 59), exercise (60, 61), intestinal microbial colonization (62), chlorpyrifos (63), cocaine addiction (64), and moderate alcohol consumption (65). This activity-dependent increase in BDNF levels likely results from the stimulation of N-Methyl-D-aspartate ionotropic glutamate receptors (NMDARs), leading to intracellular Ca2+ influx. This influx activates Ca2+/cAMP-responsive element binding protein (CREB), which binds to the BDNF promoter to initiate transcription (66). The cumulative effect of BDNF is observed with stimuli that lead to persistent and specific changes, particularly in the central nervous system. This heightened sensitivity, shaped by dietary habits and life experiences, enhances environmental adaptation, as seen in the appropriate increase in blood pressure (1, 59, 67). These factors influencing BDNF regulation and their underlying expression mechanisms are summarized in Table 1.
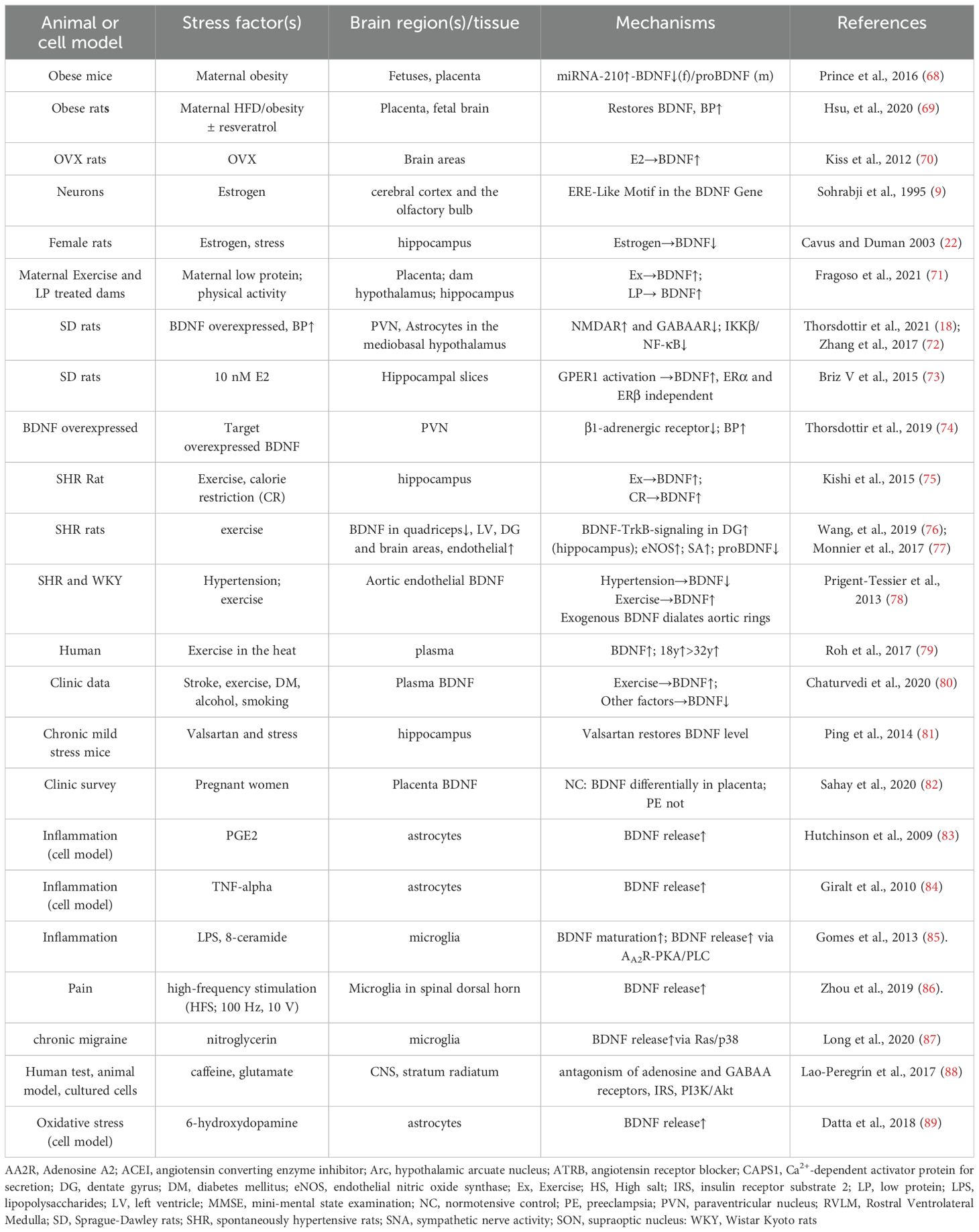
Table 1. Representative references for prenatal and postnatal stimuli influencing BDNF expression and the underlying mechanisms.
The regulatory effect of estrogens on BDNF is particularly evident in case of estrogen deficiency. Both amenorrheic individuals and postmenopausal women exhibit significantly lower plasma BDNF levels compared to fertile females, and hormone therapy effectively restores BDNF levels in these patients (90). Additionally, the administration of estradiol increases BDNF levels in ovariectomized animals across all ages (70). These findings further underscore the interaction between estrogens and BDNF, particularly in postmenopausal women and animal models.
Enhancing central BDNF levels through the peripheral administration of specific drugs offers a promising strategy for delaying age-related neurodegenerative diseases (91) and ameliorating many of the symptoms discussed above. Alternatively, long-term exercise training enhances brain function and helps prevent neurological disorders by stimulating brain plasticity through the induction of BDNF expression (92, 93). This expression is essential for certain forms of hippocampal-dependent information storage and memory (94). The benefits of exercise training can persist for an extended period, as evidenced by spatial learning and memory tests conducted in both rodents and humans (94, 95).
Therefore, plasma or serum BDNF serves as an endocrine molecule and is proposed as a biomarker for various diseases, including hepatic encephalopathy (96), depression (61, 97), Alzheimer's disease (98), mood disorders (99), schizophrenia (100), neuropsychiatric disorders (101), obesity (102), psoriasis (103), cardiometabolic problems (104), and glaucoma (105), among others. Additionally, BDNF may serve as a useful biomarker for assessing impaired memory and general cognitive function in aging women (106), as well as for prenatal hypertensive anxiety and depression in both rats and post-partum women (107).
However, studies have shown that a chronic reduction of BDNF does not exacerbate the development of neurodegenerative diseases like a Alzheimer's in mouse models (108). Interestingly, BDNF levels in the hippocampus of postmortem brain samples from AD patients are significantly higher compared to age-matched non-demented controls (98). These conflicting data may reflect the complexities of the aging brain, which can be both a consequence and a causative factor to pathological development. Furthermore, the original source of circulating BDNF remains largely unclear (109).
3 The role of BDNF and estrogen in body mass regulation
The identification of BDNF as a key gene linked to obesity highlights its crucial role in metabolic regulation (110), affecting both the CNS and peripheral organs (111). This association is particularly evident in individuals with WAGR syndrome (Wilms' tumor, aniridia, genitourinary anomalies, and intellectual disability), where those with heterozygous BDNF deletions exhibit approximately half the serum BDNF levels and a higher incidence of childhood-onset obesity, compared to those with an intact BDNF sequence (112). Additionally, central BDNF knockdown leads to hyperphagia and obesity (39, 113), while the knockout of Trek B in adipocytes reduces HDF-induced obesity in female conditional knockout mice, but this effect is not observed in males (24).
Similarly, global ERα knockout (114) leads to the development of metabolic syndrome characteristics in animal models, including weight gain, increased visceral adiposity, hyperphagia, hyperglycemia, and impaired energy expenditure through the PI3K pathway (115). In contrast to the BDNF’s central effects, estrogens act in the arcuate nucleus (ARC) to suppress food intake via ERα in pro-opiomelanocortin (POMC) neurons and NPY neurons (116, 117). In the ventromedial nucleus of the hypothalamus (VMN), estrogens influence obesity primarily by enhancing energy expenditure, mediated by VMN nitric oxide (NO) and γ-aminobutyric acid (GABA) neurons, involving both ERα and GPER. The role of ERβ, however, varies depending on the experimental model used (114, 118, 119). Additionally, in the nucleus of the NTS, estrogens inhibit food intake by sensitizing satiety signals induced by cholecystokinin (CCK) through the activation of ERα (120). Overall, estrogens contribute to maintaining a healthy lifestyle by promoting balanced nutrition and well-being in both sexes (121–123).
3.1 Maternal HFD induces prenatal central BDNF deficiency and offspring obesity
Maternal eating habits play a significant role in influencing offspring health, highlighting the critical role of BDNF in energy balance (124). An optimal fatty acid profile in a mother's diet is essential for the well-being of both mother and fetus. Clinical and experimental evidence suggests that an over-nutritious maternal HFD environment can lead to extensive molecular and cellular changes in the offspring's brain through epigenetic modifications. These changes may include downregulation of BDNF, mutations in the BDNF gene and/or its receptor, and alterations in downstream signaling pathways in the brain, all of which can contribute to neurodevelopmental disorders in the offspring (52). Additionally, maternal HFD impacts the epigenetic programming of appetite and energy homeostasis in the fetus, playing a crucial role in the development of childhood obesity (125). This evidence aligns with the phenotype associated with central BDNF knockdown (Kd) (39, 113), and reduced hypothalamic BDNF expression has been observed in leptin-receptor-deficient db/db obese mice (126).
HFDs induce the expression of neuropeptide Y (NPY) and agouti-related protein (AgRP) in orexigenic neurons, while downregulating pro-opiomelanocortin (POMC) and cocaine- and a mphetamine-regulated transcript (CART) in anorexigenic neurons. These changes occur in various hypothalamic nuclei, including the ventromedial nucleus (VMN), dorsomedial hypothalamus (DMH), lateral hypothalamus (LH), and paraventricular nucleus (PVN) in adults. BDNF and its receptor TrkB are expressed in these regions, with BDNF being most abundant in the VMN under normal dietary conditions. Maribel Rios (126) has elucidated the feeding circuits within these hypothalamic nuclei, demonstrating that HFD-induced changes in these circuits can disrupt appetite regulation and energy balance, potentially leading to obesity.
3.2 Hypothalamic BDNF decreases food intake and increases energy expenditure
Postnatal animal models demonstrate that hypothalamic BDNF suppresses food intake by acting on both orexigenic and anorexigenic neurons (39, 113). Consistently, genetically engineered rodents with CNS BDNF knockdown develop hyperphagia and obesity (113, 127, 128). Similarly, individuals with Rett syndrome, characterized by a deficiency in central BDNF, are reported to have a higher risk of obesity (129).
The cellular mechanism involves central BDNF activating the sympathetic nervous system via the Ca2+-CREB signaling pathway (1, 59, 130, 131). The cumulative effect of central BDNF activity reduces appetite by increasing the expression of anorexigenic molecules and decreasing the expression of orexigenic molecules in the hypothalamus (39). Additionally, it enhances energy expenditure by boosting sympathetic nerve activity (130), ultimately leading to a reduction in body mass.
3.3 Central estrogens decrease appetite, increase energy expenditure and promote weight loss
Estrogen is primarily produced in the ovaries in females, but it is also produced by the adrenal glands and adipose tissue in both males and females (132). Additionally, the CNS can produce estrogens, as it contains all the necessary enzymes for this process. Forebrain-specific knockout of aromatase, the rate-limiting enzyme for neuronal estrogen production, leads to a significant reduction in synaptic density and related functions in mice (133). As adipocyte enlarge, the expression of aromatase in these cells increases, resulting in elevated estrogen levels (16, 121, 134), particularly in postmenopausal women, where this contribution constitutes a substantial portion of endogenous estrogens (134).
Regardless of their sources, estrogens predominantly exert a catabolic effect by interacting with anorexigenic and orexigenic neurons in the hypothalamic arcuate nucleus (ARC). The arcuate nucleus plays a critical role in long-term energy balance, integrating signals from a variety of hormones, including estrogens and leptin (135, 136). Estrogens activate POMC neurons in the ARC, which in turn inhibit NPY/AgRP neurons, leading to reduced food intake (137). Estrogens modulate POMC neuron activity and inhibit AgRP/NPY neuron activity in the ARC through genomic pathways, Gq-coupled membrane ERα (137), and ERα-independent mechanisms (138). They enhance the phosphorylation of protein kinase B, activating a key neuronal signal pathway (139), including protein kinase C, protein kinase A, phosphatidylinositol 3-kinase, and mitogen-activated protein kinase (117, 140, 141). Additionally, estrogens increase POMC neuronal activity and reprogram synaptic plasticity in the arcuate nucleus via a signal transducer and activator of transcription 3 (STAT3)-dependent mechanism, ultimately reducing feeding. Notably, this signaling pathway operates independently of leptin (142). A comprehensive summary of the neuronal circuit and estrogenic signaling pathways can be found in the work of Mahboobifard et al. (141). The ventromedial nucleus of the hypothalamus (VMH) is a key site where both E2 and BDNF act on energy expenditure, primarily receiving projections from AgRP/NPY and CART/POMC neurons in the ARC (143, 144). Estrogen centrally inhibits AMP-activated protein kinase (AMPK) selectively in the VMH through ERα, enhancing sympathetic nervous system-brown adipose tissue (SNS-BAT) signaling and promoting thermogenesis in brown adipose tissue (BAT) (145). This results in increased glucose transport and uptake, aerobic glycolysis, and mitochondrial function, ultimately boosting ATP product, energy expenditure, and weight loss. Moreover, estrogens can also activate the Gq-coupled membrane estrogen receptor (Gq-mER) in NPY/AgRP neurons, which enhances the GABAergic postsynaptic response, however, ERα activation by E2 attenuates this effect. This highlights a functional dichotomy in the central estrogenic regulation of energy homeostasis, contrasting the rapid membrane-initiated signaling via ERα with that of Gq-mER in CNS neurons. (116). Additionally, estradiol administration has been shown to attenuate skeletal sympathetic nerve activity responses to exercise in postmenopausal women (146), indicating suggesting that estrogen may regulate sympathetic activity in a specific and conditional manner.
In addition, estrogens inhibit food intake by enhancing cholecystokinin (CCK)-induced satiety, which involves increased activity of NTS neurons through binding to ERα. This interaction regulates target gene expression, including the upregulation of c-fos (147–149) and postsynaptic density 95 (PSD-95) (139). Additionally, estrogens amplify other appetite-reducing signals, such as apolipoprotein A-IV (apo A-IV) (150) and glucagon-like peptide 1 (GLP-1) (151) within the NTS to further reduce food intake. Furthermore, BDNF/TrkB signaling in the NTS serves as a downstream mediator of estrogen's effects on energy intake, specific knockdown of BDNF in the NTS diminishes the feeding response to estrogens (152).
3.4 The role of adipocytic BDNF in the peripheral regulation of fat mass
The peripheral effects of BDNF on cellular functions and the associated signaling pathways related to metabolism have been demonstrated (24, 153) and thoroughly reviewed by Iu and Chan (24, 111). In contrast to the lower BDNF levels in the CNS observed in HFD-induced obese mice, these mice exhibit higher levels in inguinal white adipocyte tissue (iWAT) and epididymal white adipose tissue (eWAT) compared to controls, with this increase being macrophage-dependent (154). Adipocyte-specific TrkB knockout mice show resistance to HFD-induced obesity in females (24). Conversely, fat pads in systemic BDNF knockdown mice still respond to HFD stimulation by secreting more leptin than controls (155). Moreover, BDNF knockout leads to obesity (39), indicating that adipocytic BDNF is essential for the central-peripheral BDNF regulatory loop, which integrates central appetite signals and adipokine levels (155). Without adipocytic BDNF, the obese phenotype resulting from central BDNF deficiency cannot manifest, thus, the presence of adipocyte BDNF is necessary for expressing obesity due to central BDNF deficiency.
3.5 Peripheral effects of estrogen on body mass
Fat pads serve as the primary extragonadal sites for estrogen production, acting locally in a paracrine fashion or being released into circulation, particularly in postmenopausal women, men, obese individuals, and other cases (121). Peripherally, estrogens exert various metabolic effects, including increasing mtDNA polymerase Polg1 levels and mitochondrial content in WAT through ERα, thereby enhancing energy expenditure (156). They also improve insulin sensitivity by promoting energy sensing and glucose uptake via the Akt-AMP-activated protein kinase (AMPK) pathway in skeletal muscles (157). Additionally, estrogens reduce the expression of hepatic lipogenic genes, such as FASN, acetyl CoA carboxylase (ACC), and stearoyl CoA desaturase 1(SCD-1), through the STAT3 signaling pathway in the liver (158, 159). Furthermore, estrogens strongly inhibit key adipogenic genes, such as PPARγ, CBP, and adipsin, as well as leptin production, while increasing hormone-sensitive lipase expression and reducing adipocyte size (15, 160). Notably, estrogens significantly influence body fat distribution, favoring the accumulation of metabolically healthy subcutaneous fat in females while promoting visceral fat accumulation in males or OVX females (15, 15, 161, 162). The actions of estrogens in adipose tissue also extend to influence adipocyte differentiation (163) and reducing inflammation (164). Through ERα activation, estrogens provide protection against adiposity, insulin resistance, and type II diabetes while simultaneously increasing energy expenditure (165, 166). In this context, ERβ has a counteractive effect against ERα (166).
Similar to central estrogen, peripheral estrogens can also correct the abnormal appetite and metabolism resulting from central BDNF deficiency. This correction involves the transcriptional regulation of metabolic enzymes, including the downregulation of fatty acid synthase (167) and the upregulation of hormone-sensitive lipase (168). In summary, estrogens integrate brain and body metabolism, encompassing the effects of BDNF on metabolic processes, enabling the peripheral metabolic state to reflect the brain's bioenergetic status (141).
3.6 The collaborative effect of estrogen and BDNF on energy expenditure and body weight
In animal models, estrous rats undergoing sham surgery and ovariectomized rats cyclically treated with estradiol exhibit increased sensitivity to lower doses of centrally administered BDNF, leading to reduced food intake compared to male rats and oil-treated ovariectomized rats (131). This finding suggests a cooperative effect between estrogen and BDNF in regulating food intake. Although a tri-molecular cascade model—estrogen-BDNF-NPY/AgRP—has been established in the hippocampus and dentate gyrus, its direct evidence in the hypothalamus, particularly in the arcuate nucleus (ARC) and ventromedial hypothalamus (VMH), remains limited (20). Given that the estrogenic effect on BDNF expression is highly location-specific, research is needed to elucidate their relationship (21, 22, 73). The following paragraphs will focus on detailing their interaction, supported by direct evidence from the nuclei in the hypothalamus and brainstem, including the ARC, ventromedial hypothalamus (VMH), and nucleus tractus solitarius (NTS).
The ARC is a key site for the actions of steroids, BDNF, and leptin action, mediating leptin's effects through the antagonistic activity of POMC and AgRP/NPY neurons (135, 169, 170). These microcircuits play crucial roles in energy homeostasis: AgRP/NPY neurons signal hunger and stimulates food intake, while POMC neurons signal satiety and reduces food intake (171, 172). leptin acts as a monitor of energy balance within the system (173). Estrogens activate POMC neurons and inhibit AgRP/NPY neuron activity through ERα-dependent genomic and membrane-coupled pathways (137), as well as ERE-independent signaling (117, 138, 140). In contrast, the ARC expresses little to no TrkB in neurons that produce cocaine– and amphetamine–regulated transcript (CART) or NPY, suggesting that BDNF likely serves as a downstream effector of melanocortin-4 receptor (MC4R) signaling to decrease the NPY/AgRP neuron activity (173). MC4R is activated by α-melanocyte-stimulating hormone (α-MSH), a posttranslational product of POMC, which increases BDNF expression through the classic cAMP-Protein kinase A-cAMP responsive element binding protein (CREB) pathway and the ERK-ribosomal p90 S6 kinase (RSK)-cFos pathway in the rat hypothalamus (174). These evidence supports a model of estrogen-BDNF interplay in the ARC, independent of genomic estrogen effects on BDNF expression (9). This model posits that estrogen, acting in concert with POMC/ α-MSH, MC4R, BDNF, and NPY, modulates food intake regulation (173). Additionally, rapid, non-genomic estrogen signaling and acute BDNF signaling have been shown to promote dendritic spine formation and stabilization, supporting synapse and circuit plasticity while synergistically inhibiting appetite (26).
The VMH is crucial for regulating satiety, with BDNF primarily expressed there through its promoters II (175).The VMH-specific expression of BDNF and Trek B is essential for the suppression of appetite (175). Mutation in BDNF promoters II or Trek B deficiency in the VMH produce phenotypes similar those observed in leptin-deficient (Ob/Ob) mice (170), establishing BDNF as an integral component of central mechanisms mediating satiety (113). BDNF neurons in the VMH are activated by ARC POMC neurons (176, 177), which are also activated by estrogens in the ARC. This activation occurs through the inhibition of the small conductance of the calcium-activated potassium (SK) channel (178), as well as through ERα dependent signaling and c-Fos mediating cascades (116, 117). Moreover, both estrogen and BDNF work together to maintain mGluR5 function, regulating the firing rate, intrinsic excitability, and excitatory and inhibitory transmission in VMH neurons, thereby facilitating glycemic control and lipid metabolism (179). Their cooperation may dependent on ERα (180) and GPER1 signaling (181). However, VMH BDNF primarily exerts its anorexigenic effects through Trek B signaling, interacting indirectly with the leptin pathway (182), while estrogens mainly enhance sympathetically driven thermogenesis (118, 180).
In the NTS, estrogens increase BDNF expression by binding to ERα, but not ERβ, thereby initiating estrogen's genomic effect (20). Similarly to the role of BDNF in the ARC, BDNF/TrkB acts downstream of estrogen-ERα signaling; knocking down BDNF or administering a selective TrkB antagonist in the NTS prevents the anorexic effect of estrogen (152). This suggests that estrogens enhance BDNF's satiating potency, involving CCK-CCKR1 in leptin receptor-positive neurons in the NTS (183, 184). Estrogens also increase the expression of apo A-IV, a satiation factor from the gut and brain, through cytosolic ERα (150). They interact with apo A-IV via the cell membrane-bound ERα-PI3K/Akt signaling pathway to reduce food intake (26, 139, 185), while ERβ appears to have no effect on these pathways (186). Currently, there is no evidence indicating that BDNF is involved in the apo A-IV-mERα-PI3K/Akt pathway.
Non-CNS BDNF may increase in response to the loss of central BDNF induced by HFD (154). Similarly, extragonadal estrogen levels rise in enlarged fat pads due to increased 11β-HSD1 activity, which is triggered by central BDNF deficiency (39, 128, 187) and also observed in postmenopausal women (121). This suggests that peripheral estrogen and BDNF may compensate for the lack of central BDNF; however, this compensation may lead to a high equilibrium body weight (188). Notably, leptin exhibits antidepressant-like effects (189). Peripheral estrogen exerts autocrine and paracrine effects that contribute to increased body mass, alongside elevated BDNF and leptin levels, in the context of central BDNF deficiency— an occurrence referred to as "obesity protecting obesity" (132, 188). In contrast, under normal BDNF levels, estrogen finely tunes lipogenesis in various tissues, supporting a healthy metabolism (165).
3.7 Leptin: a key intersection of BDNF and estrogens
Under most circumstances, body energy levels are primarily sensed through circulating leptin levels (190). The presence of ER, leptin receptor, and BDNF/TrkB in POMC neurons within the ARC indicates that leptin significantly influences the interplay between BDNF and estrogens in regulating energy homeostasis (191, 192). Hypothalamic BDNF downregulates leptin production in adipocytes via sympathoneural β-adrenergic signaling (193). In contrast, central BDNF knockdown leads to obesity and elevated leptin expression in adipocyte (39). This increase in fat raises both estrogen levels (121) and adipokine levels, including leptin (128, 194). In normal cycling women, leptin levels positively and strongly correlate with estrogen levels, and increases further with larger fat depots (195).
Leptin reduces appetite by binding to the leptin receptor, particularly ObRb, in the arcuate (ARC), VMH, and DMH nuclei of the hypothalamus, triggering signal pathways like STAT, PI3K, and ERK (196). Centrally, leptin augments POMC neuron activity via BDNF-expressing neurons in the hypothalamic ARC, a process known as the leptin–BDNF pathway, which alters the sympathetic architecture of adipose tissue through a top (ARC)-down (PVN) neural mechanism (141, 170). Estrogens sensitize the anorexigenic effect of leptin by increasing the expression of the leptin receptor through genomic pathways and by potentiating leptin-induced pSTAT3 activation in the hypothalamus (197). Furthermore, leptin promotes local estrogen production in adipocytes by upregulating the aromatase expression and activity via STAT3 and ERK signaling pathways (198).
Estrogen deficiency, seen in ovariectomized (OVX) mice (70) and postmenopausal women (141), along with central BDNF knockdown (39), leads to increased fat accumulation and elevated leptin level. Adipocyte-specific deletion of BDNF/TrkB results in resistance to HFD-induced obesity, particularly in females (24),indicating that adipocytic BDNF is essential for the adipocytic response to central BDNF signaling and the production of adipocytokines, including leptin. Additionally, activation of mERα/mERβ can reduce body weight gain and fat accumulation in ovariectomized (199) and leptin-deficient obese mice through the PI3K pathway (200), suggesting that mER signaling can regulate energy balance independently of leptin signaling. Thus, part of the protective effects of estrogen and BDNF on energy homeostasis involves leptin (201), which may support the effects of their deficiency. It is possible that estrogen facilitates, or mimics some leptin actions (190), indicating that their interplay in regulating energy homeostasis is complex and warrants further combined studies rather than isolated examinations.
The collaborative influence of BDNF and estrogen on body mass regulation is illustrated in Figure 1.
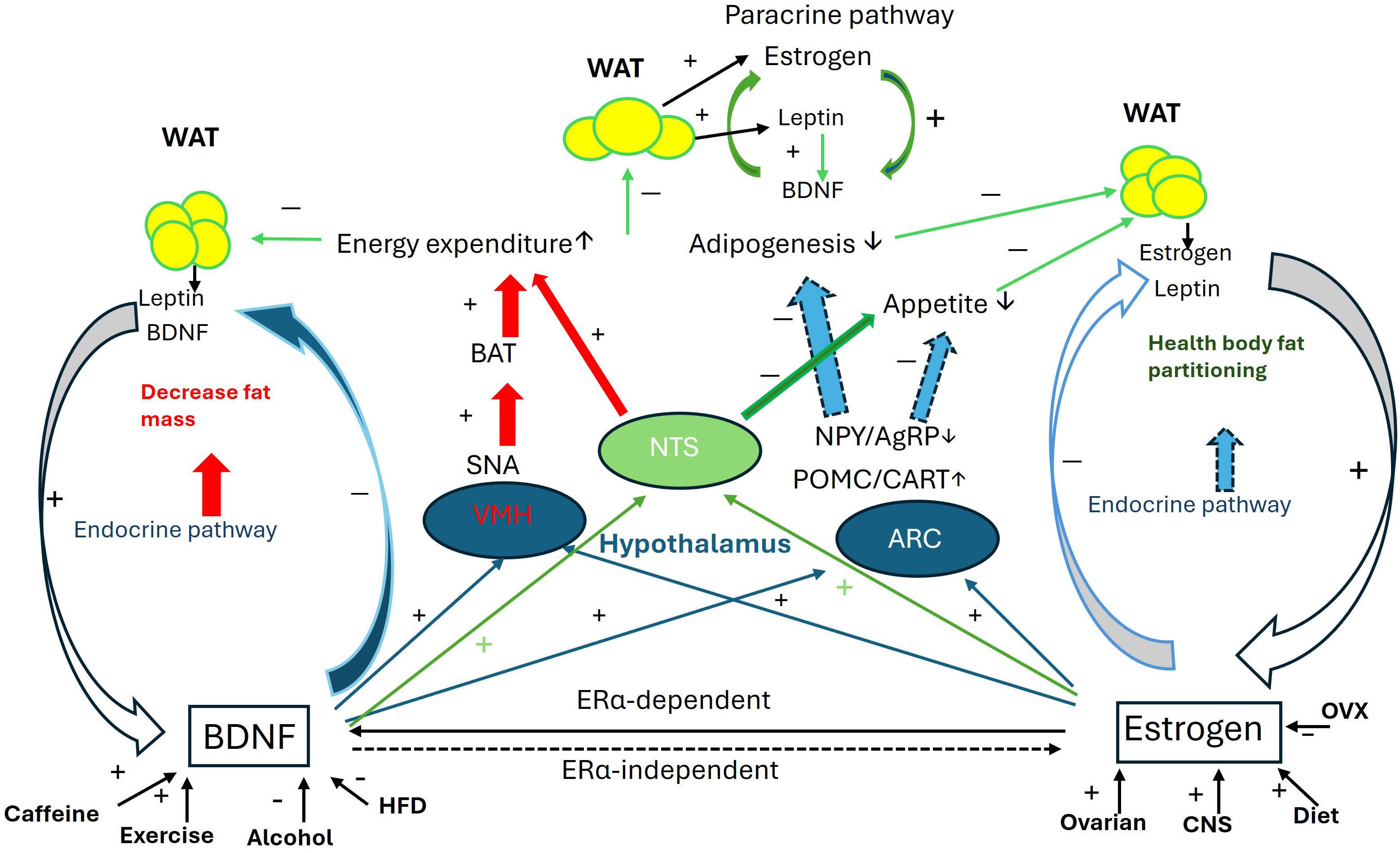
Figure 1. Collaborative interaction between BDNF and estrogen in the regulation of body mass. Schematic of the collaborative interaction between BDNF and estrogen through 1. Mutual activity enhancement: estrogen directly upregulates BDNF expression via the ERα-dependent genomic pathway, while BDNF is essential for estrogen’s effects through ERα-independent non-genomic pathways. 2. Regulation of VMH function: Both BDNF and estrogen enhance energy expenditure through the VMH-SNA-BAT pathway. 3. Regulation of NTS function: With the modulation of leptin released from fat pads, both BDNF and estrogen potentiate anorexic effects and suppress orexic effects to increase energy expenditure and reduce appetite. 4. Regulation of ARC function: BDNF and estrogen collaborate in the ARC to upregulate POMC and CART expression while downregulating NPY and AgRP expression, thus regulating appetite. Additionally, estrogen and BDNF mutually enhance their expression in fat pads. For more details, please refer to the relevant sections in the text. AgRP, agouti-related peptide; ARC, arcuate nucleus; BAT, brown adipocyte tissue; CART, cocaine- and amphetamine-regulated transcript; CNS, central nerve system; HFD, high fat diet; NPY, neuropeptide Y; NTS, Nucleus Solitarius; OVX, ovariectomy; POMC, Pro-opiomelanocortin; SNA, sympathetic nerve activity; VMH, ventromedial nucleus; WAT, white adipocyte tissue. + increase; − decrease.
3.8 Evidence for the interplay Between BDNF and estrogen in the liver and skeleton muscle
Peripheral organs such as muscles and the liver play vital roles in the central-peripheral circulation alongside fat pads. The functions of hepatic and muscle BDNF are extensively reviewed by Lu and Chan (111). Muscle-specific BDNF is essential for the regulatory loop that maintains energy balance; muscle-specific BDNF knockout (MBKO) mice exhibit impaired mitofission and mitophagy, leading to exacerbated body weight gain, reduced energy expenditure, and poor metabolic flexibility (202). Similarly, BDNF deficiency in the liver impairs metabolic regulation, resulting in hepatic steatosis and obesity (127). The deficiencies of BDNF in both liver and skeletal muscles contribute to obesity, highlighting a complex interaction among these organs that extends beyond the scope of this review.
4 The interaction between BDNF and estrogen in the development of hypertension
4.1 BDNF enhances response to recurrent, sustained, or new stressors following blood pressure stimuli
Blood pressure is directly regulated by the renin-angiotensin system (RAS) and its counteracting system. The activity of RAS is influenced by a diverse of plasma signals released from organs or tissues, such as the CNS, kidneys, lungs, liver, and adipose tissue, all of which are enriched with BDNF (1, 203). Changes in the levels of individual RAS components, such as angiotensinogen (AGT) or renin, may not directly correlate with blood pressure (unpublished data). However, the CNS effectively encodes these signals by modulating central BDNF expression, which facilitates neuroplasticity (1). The reconfigured neural network allows the brain to adaptively respond to recurring, sustained, or novel stressors.
The role of BDNF in responding to hypertensive stimuli during prenatal embryogenesis and postnatal adaptation has been thoroughly reviewed by Manti et al. (204) and Johnson et al (1). Table 2 summarizes key animal models and clinical studies that highlight the causative factors of brain’s hypertensive response through BDNF-related pathways.
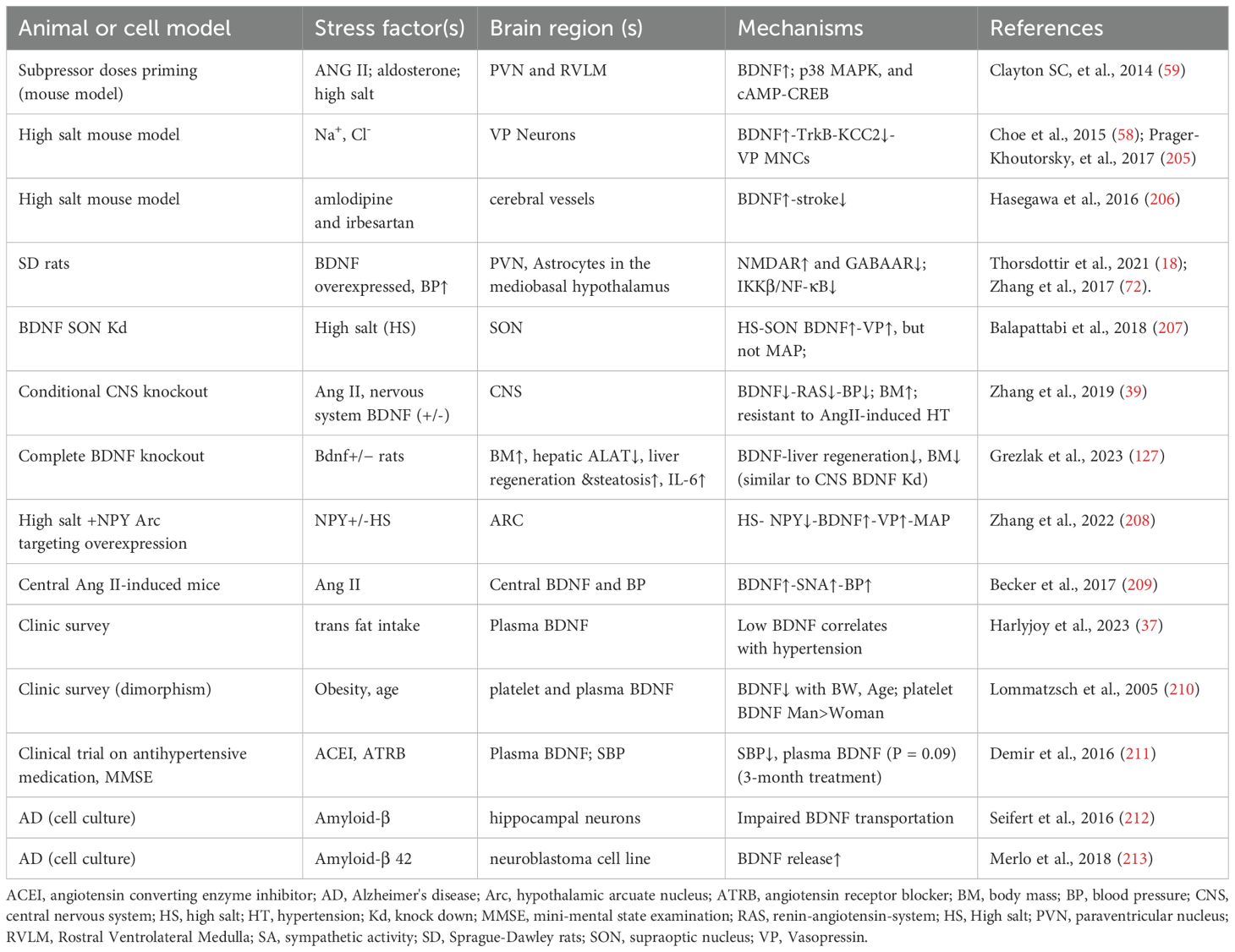
Table 2. Representative references on the adaptive expression of BDNF in response to hypertensive stimuli including high salt, Ang II, and high fat.
4.2 Slow pressor-induced hypertension: animal models demonstrating the gradual cumulation of BDNF effects on enhanced hypertensive responses
Pressor agents, such as high salt/AngII, are commonly used to study hypertension development in animal models. These agents increase BDNF levels in the hypothalamic paraventricular nucleus (PVN), enhancing neuronal activity in this region. This heightened activity stimulates the release of vasopressin (VP), activates downstream signaling pathways that raise BP (59, 207), and increases expression of RAS components (1, 59, 207).
In models employing low-dose salt and AngII induction, a method known as subpressor priming, animals display a progressively heightened hypertensive response (1, 59). This priming, achieved with low dose of salt (59), Ang II (214) or aldosterone (215), sensitizes animals to Ang II-induced hypertension by increasing BDNF levels in the PVN. This suggests that BDNF serves as a critical hub for multiple pathways, enhancing RAS sensitivity and exacerbating the development of hypertension. Johnson et al. (1) illustrate the central circuitry of BDNF, detailing its signaling pathways and physiological effects, including its impact on sympathetic tone and RAS component expression.
However, the roles of BDNF in RAS sensitization by other factors, such as inflammatory agents (216) and predator scent stress (217), remains an enigma. The observed decline in plasma and serum BDNF levels with age in humans (218), along with rising blood pressure, suggests the involvement of additional factors, including sex hormones (219). Notably, microinjection of 1 nmol/L BDNF into the subfornical organ (SFO) of anesthetized rats has been shown to decrease blood pressure (220), indicating that this effect may be context-dependent rather than solely attributable to BDNF.
4.3 Central maintenance of estrogen in regulating blood pressure
The impact of estrogens on hypertension is evident in the observed sex dimorphism, where adult men generally exhibit higher blood pressure. This distinction is further illuminated by examining blood pressure changes in women from adolescence through puberty and into postmenopause, highlighting the role of estrogen. Additionally, fluctuations in blood pressure throughout the menstrual cycle underscore estrogen's regulatory influence (219).
Estrogenic signals are required for the baseline expression of certain RAS components, such as renin (10, 221), angiotensin-converting enzyme 2 (ACE2) (222) and angiotensinogen (12, 221). Furthermore, endogenous estrogens help sustain normal blood pressure in premenopausal women, who typically have lower blood pressure compared to age-matched men (223). One mechanism for this effect is the vasodilation induced by nitric oxide (NO) and hydrogen sulfide (H2S), both produced by estrogens through ERα, ERβ and GPER-dependent pathways (223, 224). This evidence suggests that estrogens are essential for maintaining blood pressure, rather than merely reducing it.
Unlike BDNF, which potentiates the blood pressure response, estrogens act centrally to counteract these stimuli, contributing to stable blood pressure regulation. This stability is partly due to their transcriptional effects, which inhibit RAS components in an ERα-dependent manner (225). For example, low levels of RAS components are observed in female mice compared to their male counterparts (219) and OVX females (203). This subtle difference likely stems from prenatal fetal programming, and is further amplified by estrogen's effects during puberty, leading to the observed sexual dimorphism in blood pressure between males and females (219). This dimorphism tends to diminish with menopause and aging (219, 226).
Estrogen reduces blood pressure centrally by inhibiting RAS components in the subfornical organ (SFO) and other areas of the lamina terminalis (LT), which are vital for long-term blood pressure and hydroelectrolyte balance in the brain (227). Specifically, estrogens reduce the expression of central RAS components, such as the AT1 receptor and ACE1, in the LT (227). Conversely, central knockdown of ERα negates the protective effect on Ang II-induced hypertension, resulting in a significant increase in AT1, ACE1 and renin, along with a decrease in angiotensinogen (225). Additionally, maternal hypertension sensitizes ovariectomized rats to Ang II-induced hypertension in a sex-specific manner, linked to elevated RAS components in the LT and paraventricular nucleus (PVN). Administration of estradiol through the SFO can partially reverse this prenatal sensitization (228). Notably, BDNF knockdown in the SFO also decreases blood pressure by downregulating RAS components (39), indicating that BDNF and estrogen may antagonize each other in the regulation of blood pressure through their impact on RAS components.
In addition to regulating central RAS components, estrogens play a crucial role in modulating neuronal activity in the rostral ventrolateral medulla (RVLM), a key regulatory center for heart rate, blood vessel constriction, and blood pressure. In the Goldblatt two-kidney one-clip (2K-1C) male rat model of renovascular hypertension, microinjection of 17β-estradiol into the RVLM significantly reduced mean arterial pressure and renal sympathetic nerve activity in control rats compared to experimental rats. This effect is primarily mediated by ERα rather than ERβ (229). Furthermore, GPER may also influence blood pressure regulation, as microinjection of the G protein-coupled estrogen receptor (GPER) agonist G-1 into the RVLM resulted in a marked increase in mean arterial pressure and renal sympathetic nerve activity in experimental rats (229). These findings suggest a counteractive relationship between the genomic and non-genomic estrogen effects in the RVLM, mediated through ERα and GPER (229).
In addition to modulating RAS and sympathetic nerve activity, estrogens play a role in regulating various factors related to hypertension, including vasodilation and fluid balance, allowing for adaptation to environmental changes. For a more comprehensive discussion, please refer to the review by Ashraf and Vongpatanasin (230).
4.4 Comparison of BDNF and estrogen in the regulation of RAS activity
Serum BDNF levels influence blood flow and are linked to angiogenesis through TrkB signaling (231). However, it is unclear whether elevated BDNF directly causes increased blood pressure or if changes in blood pressure influence BDNF levels. For example, exogenous BDNF induces vasodilation in aortic rings, while hypertension suppresses BDNF expression in aortic endothelial cells (78).
Estrogens regulate blood pressure by modulating RAS activity through both genomic and non-genomic effects. In contrast to BDNF, which generally increases most RAS components in both the CNS and peripheral organs, except for renin in the kidney (39), estrogens selectively upregulate angiotensinogen levels while downregulating renin levels, angiotensin-converting enzyme (ACE1) activity, AT1 receptor density, and aldosterone production. Consequently, estrogens reduce RAS activity by downregulating most of the RAS components (232). Notably, the transcriptional effects of estrogen on RAS components in peripheral organs are tissue specific. For example, estrogens rapidly and significantly induce angiotensinogen expression in the liver but not in the cardiac atria (221). Additionally, estrogens potentiate vasodilation through eNOS pathway, and attenuate vasoconstriction via GPER signal (233). These patterns suggest that estrogen finely and cooperatively regulates blood pressure by integrating signals from the central nervous system to the peripheral tissues, much like how it shapes fat distribution for a healthy physique.
Overall, central BDNF amplifies the response to hypertensive stimuli by enhancing neuronal plasticity and increasing sympathetic nerve activity. In contrast, estrogens play a key role in establishing the blood pressure setpoint as part of metabolic homeostasis (132) and counteract BDNF-induced deviations through transcriptional regulation of RAS components and by reducing sympathetic nerve activity. The outcome of their interaction can vary significantly depending on factors such as the specific reagents used, cell types involved, and life stages of individuals, such as premenopause, perimenopause and postmenopause.
4.5 Leptin enhances the sensitivity of central RAS activity
Estrogen increases the synthesis and secretion of leptin from adipocytes through ERα signaling, but not ERβ (13). Leptin, in turn, sensitizes the body to hypertensive stimuli by enhancing central RAS activity and promoting the release of proinflammatory cytokines (234), while also innervating BDNF neurons in the paraventricular nucleus of the hypothalamus (170). Thus, instead of acting as a collaborative factor with estrogen in energy homeostasis, leptin functions as a counterpart for estrogens in blood pressure regulation. However, leptin resistance is also reported to contribute to hypertension in Bardet-Biedl syndrome mouse models (235), suggesting that obesity paradox may be at play (236), or leptin's effects can vary depending on different physiological backgrounds.
These pathways (Figure 2) illustrate the communication between peripheral fat pads and the hypothalamus, clarifying the roles of both central and peripheral BDNF in the relationship between obesity and hypertension. This model may also account for the sensitization of angiotensin II-induced hypertension in adult offspring that were primed by maternal high-fat diet (237).
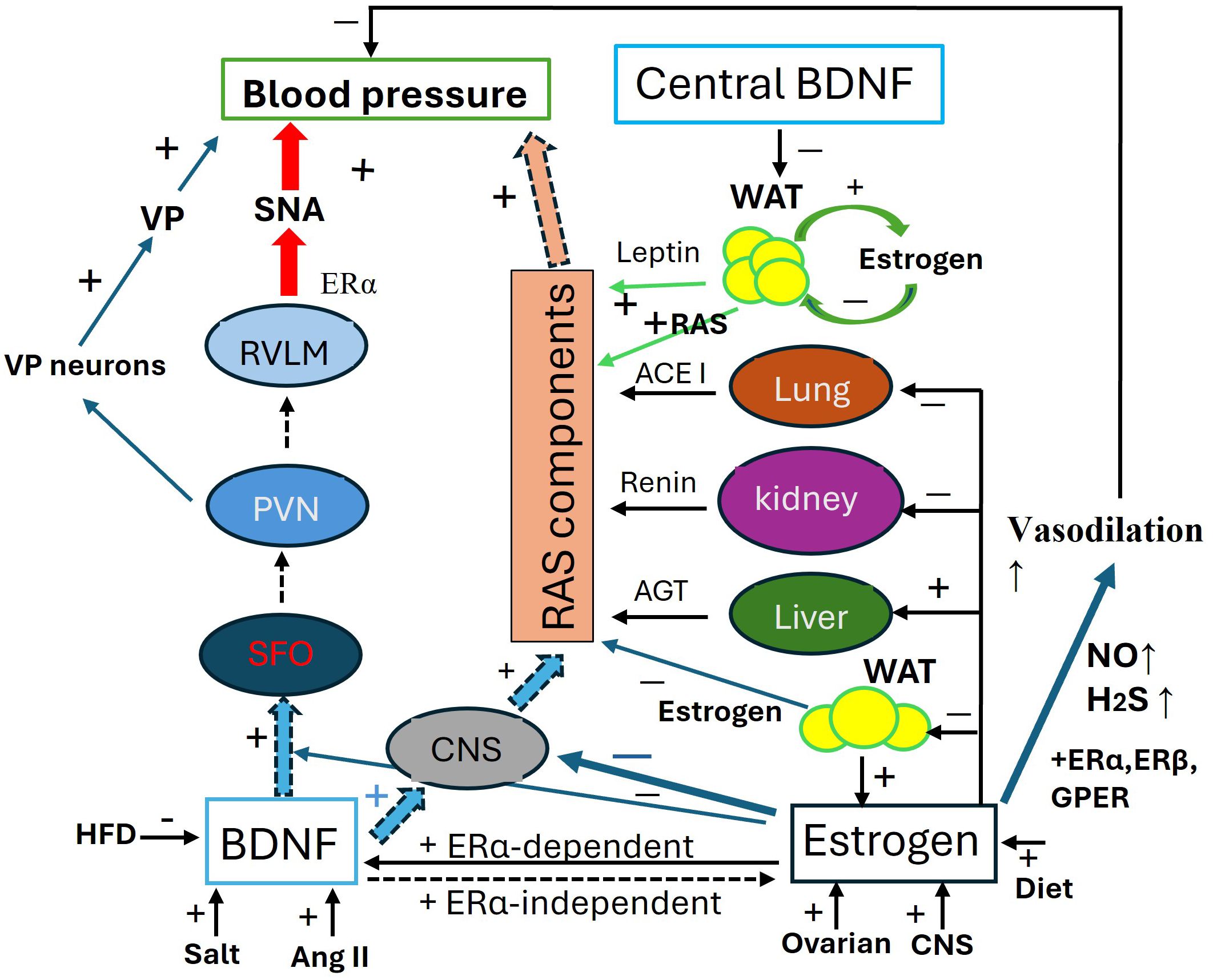
Figure 2. Antagonistic interaction between BDNF and estrogen in the regulation of blood pressure. Schematic of the antagonistic interaction between BDNF and estrogen in the regulation of blood pressure (BP). BDNF increases blood pressure through 1. SFO-PNN-VP Pathway: by activating this pathway, BDNF increases VP release to elevate BP; 2. SFO-PVN-RVLM Pathway, via this pathway, BDNF enhances SNA to increase BP; 3. RAS component expression, BDNF systemically upregulates the RAS components to elevate BP. The expression of BDNF is activity-dependent and influenced by factors such as high salt intake, Ang II, and a high-fat diet. Estrogens maintain baseline blood pressure by transcriptionally regulating RAS components in the liver, kidneys, lungs, CNS, and fat pads. Additionally, Estrogens enhance the generation of H2S and NO for vasodilation through ERα, ERβ, and mGluRs pathways. Estrogens play an integrative role across various organs and tissues, fine-tuning blood pressure regulation, including modulating the effects of BDNF. ACE1, angiotensin converting enzyme 1; AGT, angiotensinogen; AngII, angiotensin II; CNS, central nerve system; ERα/β, estrogen receptor α/β; GPER, G-protein coupled ER; HFD, high fat diet; H2S, hydrogen sulfide; NO, nitric oxide; PVN, paraventricular nucleus; RAS, renin-angiotensin-system; RVLM, Rostral Ventrolateral Medulla; SAN, sympathetic activity; VP, vasopressin.
The interaction between BDNF and estrogen, along with the role of leptin, is illustrated in Figure 2.
4.6 Evidence of BDNF and estrogen interaction in body fluid balance
The amount of body fluids fluctuates during normal reproductive cycles, in sync with varying levels of ovarian hormones. Estrogen treatment enhances fluid retention by lowering the threshold for arginine vasopressin (AVP) release and increasing plasma renin activity (238). Studies have demonstrated that water deprivation for 24 hours, 2 days, and 4 days, as well as salt loading for 7 days, result in a significant increase in BDNF gene transcripts in the SFO in rats (239), suggesting the BDNF’s involvement in the regulation of body fluids. However, there is limited direct information available on the specific interaction between BDNF and estrogen in this context.
5 Postmenopausal syndrome, a clinic model of estrogen and BDNF deficiency
Estrogen deficiency is a key factor in the onset of menopause. Postmenopausal women experience a decline in plasma estrogen levels, leading to menopausal symptoms, including metabolic changes (110). Both postmenopausal women and amenorrheic individuals exhibit significantly lower plasma BDNF levels compared to fertile females (19, 240), underscoring the complex interplay between BDNF and estrogen highlighted in this review.
Postmenopausal women often experience weight gain, particularly in the form of visceral obesity (162, 241). The weight gain and the accumulation of abdominal fat are likely due to estrogen deficiency, as hormone replacement therapy (HRT) can alleviate these symptoms in postmenopausal women (242, 243). Estrogen deficiency results in a reduction in estrogen-dependent BDNF (9) and ERα (11) expression in target tissues, thereby further weakening estrogenic signals. Ultimately, estrogen deficiency results in a loss of fine-tuned fat accumulation, leaving postmenopausal women with more visceral fat, which becomes the primary source of leptin, adipokine (162, 194, 243), RAS components (244, 245) and even estrogen (134) in postmenopausal women.
Increased visceral fat mass leads to higher synthesis of leptin, which upregulates BDNF in hypothalamic neurons (182). Leptin stimulates lipolysis while inhibiting lipogenesis (246) and enhances thermogenesis in BAT (246). Additionally, it restores sympathetic innervation of WAT (194), acting as a substitute for estrogen by suppressing appetite, increasing energy expenditure and reducing body weight and adiposity. Moreover, leptin modulates the neuroendocrine axes, autonomic nervous system, neural plasticity, and memory, thereby partially replicating the effects of estrogen and BDNF (194).
However, alongside aging factors, the elevation of leptin increases sympathetic nerve activity (247) and RAS activity in postmenopausal women and OVX animal models (234, 248), contributing to postmenopausal hypertension. Additionally, elevated levels of adipokines such as IL-1 and IL-6 from enlarged visceral fat pads may promote vascular inflammation, endothelial dysfunction, and increased vascular resistance, further exacerbating hypertension (194, 249, 250). These factors can also trigger the immune system, leaving postmenopausal women vulnerable to chronic inflammatory syndromes (85, 194). These processes are illustrated in Figure 3.
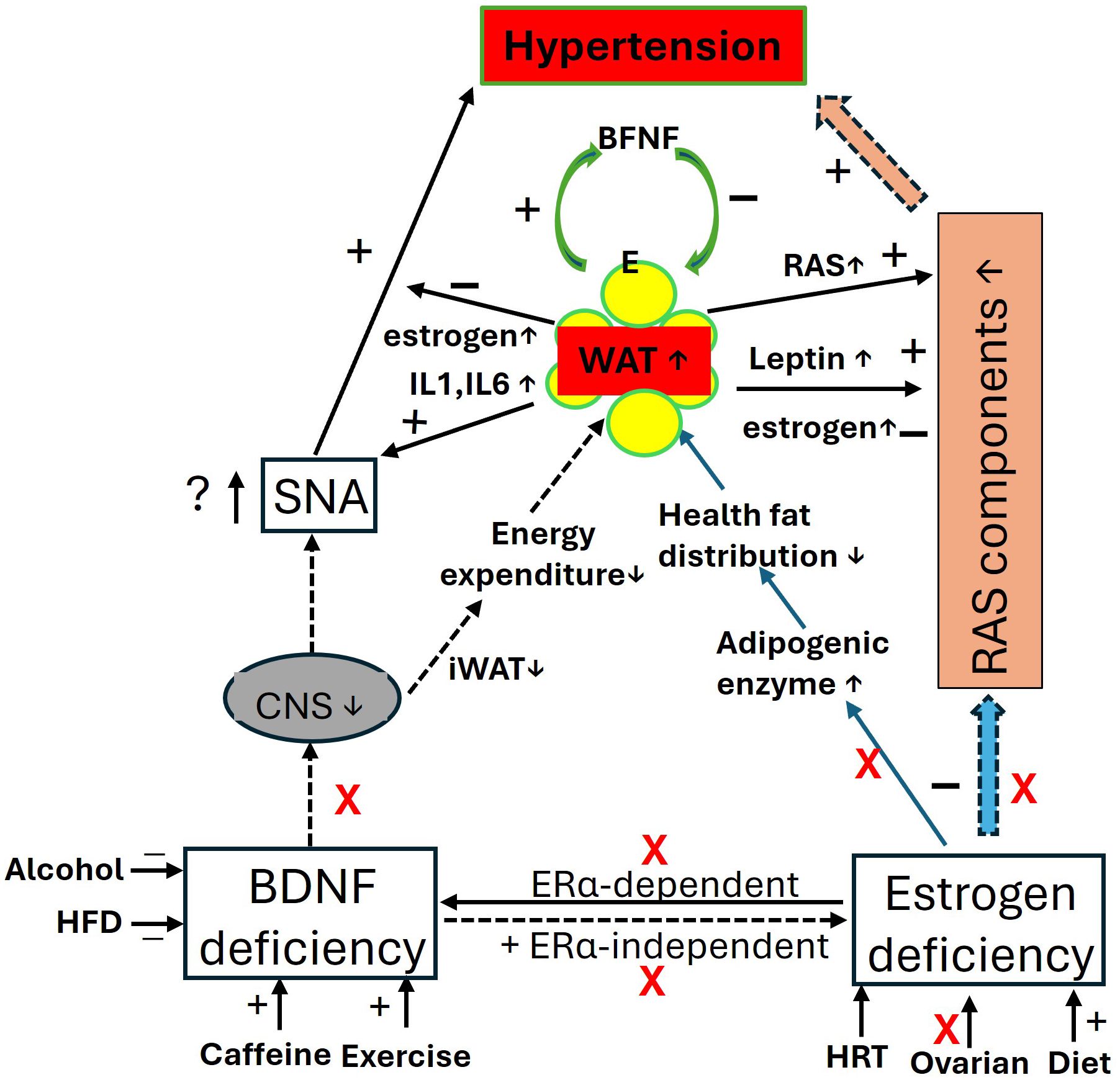
Figure 3. The impact of BDNF and estrogen deficiency in postmenopausal women: focus on blood pressure and obesity. In the context of BDNF and estrogen deficiency, the central effects of BDNF and estrogen, along with their regulation of RAS activity and mutual interactions, are diminished, leading to elevated blood pressure (hypertension) and increased fat mass (obesity). Visceral fat produces more estrogens through the upregulation of estrogen-converting enzymes 17β-hydroxysteroid dehydrogenase (HSD17B7) (134), and synthesizes more leptin with adiposity, which may help inhibit food intake and regulate blood pressure. However, fat pads release proinflammatory agents, contributing to immune and metabolic disorders. iWAT, beige adipose tissue; CNS, central nerve system; E, estrogen; ERα, estrogen receptor α; HFD, high fat diet; HRT, hormone replace treatment; IL, interleukins; RAS, renin-angiotensin-system; RVLM, Rostral Ventrolateral Medulla; SNA, sympathetic activity.
Given that central BDNF knockdown leads to resistance to Ang II-induced hypertension (39), while central ERα knockdown results in heightened sensitivity to Ang II-induced hypertension (225), BDNF and estrogen function as physiological antagonists. Specifically, central BDNF contributes to an elevated blood pressure in response to environmental stimuli (1, 67), whereas estrogen’s central effect is to maintain blood pressure within the set point range (251). Reduced signaling from both BDNF and estrogen can impair an individuals' ability to regulate blood pressure in response to environmental changes, potentially leading to hypertension, especially in postmenopausal women.
Although the role of hormone replacement therapy (HRT) with estrogen or/and progesterone remains debated, it is widely used in clinical practice (176). Additional strategies, such as phytoestrogens, combined estrogen and progesterone treatments, and non-hormonal options, have also been proposed (252). These approaches boost plasma estrogen and BDNF levels (19, 240) provided the individual is suitable for such treatments. For safety reasons, lifestyle modifications like regular exercise (79, 80), coffee consumption (88) and a healthy diet, known to increase plasma BDNF and estrogen levels, are highly recommended. Conversely, high-fat diets (253), smoking, and alcohol, which are known to decrease BDNF levels (80), should be approached with caution as they may exacerbate postmenopausal syndromes.
Author contributions
ZZ: Writing – original draft, Writing – review & editing. ZH: Validation, Writing – review & editing. JP: Validation, Writing – review & editing. MY: Conceptualization, Writing – review & editing. YL: Conceptualization, Methodology, Writing – review & editing. CZ: Supervision, Writing – review & editing. XW: Supervision, Conceptualization, Writing – review & editing.
Funding
The author(s) declare financial support was received for the research, authorship, and/or publication of this article. This work was supported by grants from Henan Natural Science Foundations (242300421302) (to ZZ), Science and Technology Project of Henan Province (172102310218) (to CZ).
Conflict of interest
The authors declare that the research was conducted in the absence of any commercial or financial relationships that could be construed as a potential conflict of interest.
Publisher’s note
All claims expressed in this article are solely those of the authors and do not necessarily represent those of their affiliated organizations, or those of the publisher, the editors and the reviewers. Any product that may be evaluated in this article, or claim that may be made by its manufacturer, is not guaranteed or endorsed by the publisher.
References
1. Johnson AK, Zhang Z, Clayton CS, Beltz TG, Hurley S, Thunhorst and B. Xue R. The roles of sensitization and neuroplasticity in the long-term regulation of blood pressure and hypertension. Am J Physiol Regulatory Integr comparativ. (2015) 309:R1309–1325. doi: 10.1152/ajpregu.00037.2015
2. Kashyap PM, Roberts C, Waseem and P. Tyagi M. Drug targets in neurotrophin signaling in the central and peripheral nervous system. Mol Neurobiol. (2018) 55:6939–55. doi: 10.1007/s12035-018-0885-3
3. Hang ZP, Ge QF, Li FP, Liu J, Zhu and J. Zhao H. The regulatory role of the BDNF/TrkB pathway in organ and tissue fibrosis. Histol Histopathol. (2021) 36:1133–43. doi: 10.14670/HH-18-368
4. Numakawa T, Yokomaku D, Richards M, Hori H, Adachi and H. Kunugi N. Functional interactions between steroid hormones and neurotrophin BDNF. World J Biol Chem. (2010) 1:. 133–143. doi: 10.4331/wjbc.v1.i5.133
5. Colucci-D'Amato L, Speranza L, Volpicelli F. Neurotrophic factor BDNF, physiological functions and therapeutic potential in depression, neurodegeneration and brain cancer. Int J Mol Sci. (2020) 21:7777. doi: 10.3390/ijms21207777
6. Fuentes N, Silveyra P. Estrogen receptor signaling mechanisms. Adv Protein Chem Struct Biol. (2019) 116:135–70. doi: 10.1016/bs.apcsb.2019.01.001
7. Findlay KJ, Liew HS, Simpson RE, Korach SK. Estrogen signaling in the regulation of female reproductive functions. Handb Exp Pharmacol. (2010) 198:29–35. doi: 10.1007/978-3-642-02062-9_2
8. Mannella P, Brinton DR. Estrogen receptor protein interaction with phosphatidylinositol 3-kinase leads to activation of phosphorylated Akt and extracellular signal-regulated kinase 1/2 in the same population of cortical neurons: a unified mechanism of estrogen action. J Neurosci. (2006) 26:. 9439–9447. doi: 10.1523/JNEUROSCI.1443-06.2006
9. Sohrabji F, Miranda CR, Toran-Allerand DC. Identification of a putative estrogen response element in the gene encoding brain-derived neurotrophic factor. Proc Natl Acad Sci USA. (1995) 92:11110–4. doi: 10.1073/pnas.92.24.11110
10. Lu TK, Keen LH, Weatherford TE, Sequeira-Lopez LM, Gomez AR, Sigmund DC. Estrogen receptor α Is required for maintaining baseline renin expression. Hypertension. (2016) 67:992–9. doi: 10.1161/HYPERTENSIONAHA.115.07082
11. Hu P, Kinyamu KH, Wang L, Martin J, Archer and C. Ten KT. Estrogen induces estrogen-related receptor alpha gene expression and chromatin structural changes in estrogen receptor (ER)-positive and ER-negative breast cancer cells. J Biol Chem. (2008) 283:6752–63. doi: 10.1074/jbc.M705937200
12. Feldmer M, Kaling M, Takahashi S, Mullins and D. Ganten JJ. Glucocorticoid- and estrogen-responsive elements in the 5’-flanking region of the rat angiotensinogen gene. J Hypertens. (1991) 9:1005–12. doi: 10.1097/00004872-199111000-00005
13. Kuryłowicz A. Estrogens in adipose tissue physiology and obesity-related dysfunction. Biomedicines. (2023) 11:690. doi: 10.3390/biomedicines11030690
14. Salehpour A, Shidfar F, Hedayati M, Neshatbini Tehrani A, Farshad and S. Mohammadi AA. Bisphenol A enhances adipogenic signaling pathways in human mesenchymal stem cells. Genes Environ. (2020) 42:13. doi: 10.1186/s41021-020-00150-6
15. Stubbins ER, Holcomb BV, Hong J, Núñez PN. Estrogen modulates abdominal adiposity and protects female mice from obesity and impaired glucose tolerance. Eur J Nutr. (2012) 51:. 861–870. doi: 10.1007/s00394-011-0266-4
16. Barakat R, Oakley O, Kim H, Jin J, Ko JC. Extra-gonadal sites of estrogen biosynthesis and function. BMB Rep. (2016) 49:488–96. doi: 10.5483/BMBRep.2016.49.9.141
17. Christakis KM, Hasan H, De Souza and L. Shirreff LR. The effect of menopause on metabolic syndrome: cross-sectional results from the Canadian Longitudinal Study on Aging. Menopause. (2020) 27:999–1009. doi: 10.1097/GME.0000000000001575
18. Thorsdottir D, Einwag Z, Erdos B. BDNF shifts excitatory-inhibitory balance in the paraventricular nucleus of the hypothalamus to elevate blood pressure. J Neurophysiol. (2021) 126:1209–20. doi: 10.1152/jn.00247.2021
19. Cubeddu A, Giannini A, Bucci F, Merlini S, Casarosa E, Pluchino N, et al. Paroxetine increases brain-derived neurotrophic factor in postmenopausal women. Menopause. (2010) 17:338–43. doi: 10.1097/gme.0b013e3181c29e44
20. Scharfman EH, MacLusky JN. Estrogen and brain-derived neurotrophic factor (BDNF) in hippocampus: complexity of steroid hormone-growth factor interactions in the adult CNS. Front Neuroendocrinol. (2006) 27:415–35. doi: 10.1016/j.yfrne.2006.09.004
21. Sohrabji F, Lewis KD. Estrogen-BDNF interactions: implications for neurodegenerative diseases. Front Neuroendocrinol. (2006) 27:404–14404–14. doi: 10.1016/j.yfrne.2006.09.003
22. Cavus I, Duman R. Influence of estradiol, stress, and 5-HT2A agonist treatment on brain-derived neurotrophic factor expression in female rats. Biol Psychiatry. (2003) 54:59–69. doi: 10.1016/S0006-3223(03)00236-1
23. Autry AE. Function of brain-derived neurotrophic factor in the hypothalamus: Implications for depression pathology. Front Mol Neurosci. (2022) 15:1028223. doi: 10.3389/fnmol.2022.1028223
24. Nakagomi A, Okada S, Yokoyama M, Yoshida Y, Shimizu I, Miki T, et al. Role of the central nervous system and adipose tissue BDNF/TrkB axes in metabolic regulation. NPJ Aging Mech Dis. (2015) 1:15009. doi: 10.1038/npjamd.2015.9
25. Zhao Z, Xue F, Gu Y, Han J, Jia Y, Ye and Y. Zhang K. Crosstalk between the muscular estrogen receptor α and BDNF/TrkB signaling alleviates metabolic syndrome via 7,8-dihydroxyflavone in female mice. Mol Metab. (2021) 45:101149. doi: 10.1016/j.molmet.2020.101149
26. Srivastava PD, Woolfrey MK, Evans DP. Mechanisms underlying the interactions between rapid estrogenic and BDNF control of synaptic connectivity. Neuroscience. (2013) 239:17–33. doi: 10.1016/j.neuroscience.2012.12.004
27. Spencer-Segal JL, Waters EM, Bath KG, Chao MV, McEwen BS, Milner TA. Distribution of phosphorylated Trkb receptor in the mouse hippocampal formation depends on sex and estrous cycle stage. J Neurosci. (2011) 31:6780–90. doi: 10.1523/JNEUROSCI.0910-11.2011
28. Liu B, Liu Y, Li S, Chen P, Zhang and L. Feng J. BDNF promotes mouse follicular development and reverses ovarian aging by promoting cell proliferation. J Ovarian Res. (2023) 16:83. doi: 10.1186/s13048-023-01163-9
29. Vieira AD, Medeiros EB, Zabot GC, Pereira NS, do Nascimento NB, Lidio AV, et al. Neuroprotective effects of combined therapy with memantine, donepezil, and vitamin D in ovariectomized female mice subjected to dementia model. Prog Neuropsychopharmacol Biol Psychiatry. (2023) 122:110653. doi: 10.1016/j.pnpbp.2022.110653
30. Farajdokht F, Sadigh-Eteghad S, Vatandoust S, Hosseini L, Morsali S, Feizi H, et al. Sericin improves memory impairment via activation of the PKA-CREB-BDNF signaling pathway and suppression of oxidative stress in ovariectomized mice. Neurochem Res. (2024) 49:1093–104. doi: 10.1007/s11064-023-04094-5
31. Walf AA, Koonce CJ, Frye CA. Adult female wildtype, but not oestrogen receptor beta knockout, mice have decreased depression-like behaviour during pro-oestrus and following administration of oestradiol or diarylpropionitrile. J Psychopharmacol. (2009) 23:442–50. doi: 10.1177/0269881108089598
32. Hales CM, Carroll MD, Fryar CD, Ogden CL. Prevalence of obesity and severe obesity among adults: United States, 2017-2018. NCHS Data Brief. (2020) 360:. 1–8.
33. Sandrini L, Di Minno A, Amadio P, Ieraci A, Tremoli E, Barbieri SS. Association between obesity and circulating brain-derived neurotrophic factor (BDNF) levels: systematic review of literature and meta-analysis. Int J Mol Sci. (2018) 19:2281. doi: 10.3390/ijms19082281
34. Gajewska E, Sobieska M, Łojko D, Wieczorowska-Tobis K, Suwalska A. Obesity itself does not influence BDNF serum levels in adults. Eur Rev Med Pharmacol Sci. (2014) 18:3246–50.
35. Brahmbhatt Y, Gupta M, Hamrahian S. Hypertension in premenopausal and postmenopausal women. Curr Hypertens Rep. (2019) 21:74. doi: 10.1007/s11906-019-0979-y
36. Konishi K, Cherkerzian S, Aroner S, Jacobs EG, Rentz DM, Remington A, et al. Impact of BDNF and sex on maintaining intact memory function in early midlife. Neurobiol Aging. (2020) 88:137–49. doi: 10.1016/j.neurobiolaging.2019.12.014
37. Harlyjoy CB, Sukmawan R, Muliawan SH, Soerarso R, Radi B, Widyantoro B. Plasma brain derived neurotrophic factor level as a modifying factor for trans fat intake and hypertension. Clin Nutr ESPEN. (2023) 55:38–43. doi: 10.1016/j.clnesp.2023.02.005
38. Rus M, Crisan S, Andronie-Cioara FL, Indries M, Marian P, Pobirci OL, et al. Prevalence and risk factors of metabolic syndrome: A prospective study on cardiovascular health. Medicina (Kaunas). (2023) 59:1711. doi: 10.3390/medicina59101711
39. Zhang Z, Zhang Y, Wang Y, Ding S, Wang C, Gao L, et al. Genetic knockdown of brain-derived neurotrophic factor in the nervous system attenuates angiotensin II-induced hypertension in mice. J renin-angiotensin-aldosterone system: JRAAS. (2019) 20:1470320319834406. doi: 10.1177/1470320319834406
40. Xie X, Xu B. Neurotrophic factor control of satiety and body weight. Nat Rev Neurosci. (2016) 17:282–92. doi: 10.1038/nrn.2016.24
41. An J, Liao GY, Kinney CE, Sahibzada N, Xu B. Discrete BDNF neurons in the paraventricular hypothalamus control feeding and energy expenditure. Cell Metab. (2015) 22:175–88. doi: 10.1016/j.cmet.2015.05.008
42. Ribases M, Gratacos M, Fernandez-Aranda F, Bellodi L, Boni C, Anderluh M. Association of BDNF with anorexia, bulimia and age of onset of weight loss in six European populations. Hum Mol Gene. (2004) 13:1205–12. doi: 10.1093/hmg/ddh137
43. Fatma R, Chauhan W, Shahi M, Afzal M. Association of BDNF gene missense polymorphism rs6265 (Val66Met) with three quantitative traits, namely, intelligence quotient, body mass index, and blood pressure: A genetic association analysis from North India. Front Neurol. (2023) 13:1035885. doi: 10.3389/fneur.2022.1035885
44. Maisonpierre P, Belluscio L, Friedman B, Alderson R, Wiegand S, Furth M, et al. NT-3, BDNF, and NGF in the developing rat nervous system: parallel as well as reciprocal patterns of expression. Neuron. (1990) 5:501–9. doi: 10.1016/0896-6273(90)90089-X
45. Carito V, Ceccanti M, Ferraguti and R. Coccurello G. NGF and BDNF alterations by prenatal alcohol exposure. Curr neuropharmacology. (2019) 17:308–17. doi: 10.2174/1570159X15666170825101308
46. Bird C, Baculis B, Mayfield J, Chavez G, Ontiveros T, Paine D, et al. The brain-derived neurotrophic factor VAL68MET polymorphism modulates how developmental ethanol exposure impacts the hippocampus. Genes brain Behav. (2019) 18:e12484. doi: 10.1111/gbb.2019.18.issue-3
47. McCarthy MD, Mueller AK, Cannon NE, Huizenga NM, Darnell BS, Bhide GP, et al. Prenatal cocaine exposure alters BDNF-trkB signaling in the embryonic and adult brain. Dev Neurosci. (2016) 38:365–74. doi: 10.1159/000453609
48. Kabir DZ, Lourenco F, Byrne EM, Katzman and F. Lee A. Brain-derived neurotrophic factor genotype impacts the prenatal cocaine-induced mouse phenotype. Dev Neurosci. (2012) 34:2–3184–97. doi: 10.1159/000337712
49. McCarthy MD, Kabir DZ, Bhide GP, Kosofsky EB. Effects of prenatal exposure to cocaine on brain structure and function. Prog Brain Res. (2014) 211:277–89. doi: 10.1016/B978-0-444-63425-2.00012-X
50. De Sousa R, Peixoto M, Leite RH, Oliveira RL, Freitas AD, Silva-Júnior AF, et al. Neurological consequences of exercise during prenatal Zika virus exposure to mice pups. Int J Neurosci. (2022) 132:1091–101. doi: 10.1080/00207454.2020.1860970
51. Dias TC, Curi TH, Payolla BT, Lemes FS, Betim Pavan CI, Torsoni AM, et al. Maternal high-fat diet stimulates proinflammatory pathway and increases the expression of Tryptophan Hydroxylase 2 (TPH2) and brain-derived neurotrophic factor (BDNF) in adolescent mice hippocampus. Neurochemistry Int. (2020) 139:104781. doi: 10.1016/j.neuint.2020.104781
52. Urbonaite G, Knyzeliene A, Bunn SF, Smalskys and U. Neniskyte A. The impact of maternal high-fat diet on offspring neurodevelopment. Front Neurosci. (2022) 16:909762. doi: 10.3389/fnins.2022.909762
53. Santucci D, Sorace A, Francia N, Aloe and E. Alleva L. Prolonged prenatal exposure to low-level ozone affects aggressive behaviour as well as NGF and BDNF levels in the central nervous system of CD-1 mice. Behav Brain Res. (2006) 166:124–30. doi: 10.1016/j.bbr.2005.07.032
54. Sobolewski M, Abston K, Conrad K, Marvin E, Harvey K, Susiarjo M, et al. Lineage- and sex-dependent behavioral and biochemical transgenerational consequences of developmental exposure to lead, prenatal stress, and combined lead and prenatal stress in mice. Environ Health Perspect. (2020) 128:27001. doi: 10.1289/EHP4977
55. Machaalani R, Thawley M, Huang J, Chen H. Effects of prenatal cigarette smoke exposure on BDNF, PACAP, microglia and gliosis expression in the young male mouse brainstem. Neurotoxicology. (2019) 74:40–6. doi: 10.1016/j.neuro.2019.05.009
56. Benevenuto MS, Domenico DM, Yariwake YV, Dias TC, Mendes-da-Silva C, Alves ON, et al. Prenatal exposure to Cannabis smoke induces early and lasting damage to the brain. Neurochemistry Int. (2022) 160:105406. doi: 10.1016/j.neuint.2022.105406
57. Bath GK, Pimentel T. Effect of early postnatal exposure to valproate on neurobehavioral development and regional BDNF expression in two strains of mice. Epilepsy behavior: E&B. (2017) 70:110–7. doi: 10.1016/j.yebeh.2017.02.026
58. Choe YK, Han YS, Gaub P, Shell B, Voisin LD, Knapp AB, et al. High salt intake increases blood pressure via BDNF-mediated downregulation of KCC2 and impaired baroreflex inhibition of vasopressin neurons. Neuron. (2015) 85:549–60. doi: 10.1016/j.neuron.2014.12.048
59. Clayton CS, Zhang Z, Beltz T, Xue B, Johnson AK. CNS neuroplasticity and salt-sensitive hypertension induced by prior treatment with subpressor doses of ANG II or aldosterone. Am J Physiol Regulatory Integr Comp physioloy. (2014) 306:R908–917. doi: 10.1152/ajpregu.00010.2014
60. Wrann DC, White PJ, Salogiannnis J, Laznik-Bogoslavski D, Wu J, Ma D, et al. Exercise induces hippocampal BDNF through a PGC-1α/FNDC5 pathway. Cell Metab. (2013) 18:649–59. doi: 10.1016/j.cmet.2013.09.008
61. Murawska-Ciałowicz E, Wiatr M, Ciałowicz M, Gomes de Assis G G, Borowicz W, Rocha-Rodrigues S, et al. BDNF impact on biological markers of depression-role of physical exercise and training. Int J Environ Res Public Health. (2021) 18:7553. doi: 10.3390/ijerph18147553
62. Sudo N, Chida Y, Aiba Y, Sonoda J, Oyama N, Yu NX, et al. Postnatal microbial colonization programs the hypothalamic-pituitary-adrenal system for stress response in mice. J Physiol. (2004) 5581:263–75. doi: 10.1113/jphysiol.2004.063388
63. Perez-Fernandez C, Morales-Navas M, Guardia-Escote L, Colomina TM, Giménez E, Sánchez-Santed F. Postnatal exposure to low doses of Chlorpyrifos induces long-term effects on 5C-SRTT learning and performance, cholinergic and GABAergic systems and BDNF expression. Exp Neurol. (2020) 330:113356. doi: 10.1016/j.expneurol.2020.113356
64. Li X, Wolf EM. Multiple faces of BDNF in cocaine addiction. Behav Brain Res. (2015) 279:240–54. doi: 10.1016/j.bbr.2014.11.018
65. Logrip LM, Barak S, Warnault V, Ron D. Corticostriatal BDNF and alcohol addiction. Brain Res. (2015) 1628:60–7. doi: 10.1016/j.brainres.2015.03.025
66. Kowiański P, Lietzau G, Czuba E, Waśkow M, Steliga A, Moryś J. A key factor with multipotent impact on brain signaling and synaptic plasticity. Cell Mol Neurobiol. (2018) 38:579–93. doi: 10.1007/s10571-017-0510-4
67. Young JH. Evolution of blood pressure regulation in humans. Curr Hypertens Rep. (2007) 9:13–8. doi: 10.1007/s11906-007-0004-8
68. Prince SC, Maloyan A, Myatt L. Maternal obesity alters brain derived neurotrophic factor (BDNF) signaling in the placenta in a sexually dimorphic manner. Placenta. (2017) 49:55–63. doi: 10.1016/j.placenta.2016.11.010
69. Hsu HM, Sheen MJ, Lin IC CI, Yu RH, Tiao MM, Tain LY, et al. Effects of maternal resveratrol on maternal high-fat diet/obesity with or without postnatal high-fat diet. Int J Mol Sci. (2020) 21:3428. doi: 10.3390/ijms21103428
70. Kiss A, Delattre MA, Pereira IS, Carolino GR, Szawka ER, Anselmo-Franci AJ, et al. 17β-estradiol replacement in young, adult and middle-aged female ovariectomized rats promotes improvement of spatial reference memory and an antidepressant effect and alters monoamines and BDNF levels in memory- and depression-related brain areas. Behav Brain Res. (2012) 227:100–8. doi: 10.1016/j.bbr.2011.10.047
71. Fragoso J, Carvalho Jurema Santos G, da Silva TH, Loizon E, de Oliveira Nogueira Souza V, Vidal H, et al. Effects of maternal low-protein diet and spontaneous physical activity on the transcription of neurotrophic factors in the placenta and the brains of mothers and offspring rats. J Dev Orig Health Dis. (2021) 12:505–12. doi: 10.1017/S2040174420000756
72. Zhang Y, Reichel MJ, Han C, Zuniga-Hertz PJ, Cai D. Astrocytic process plasticity and IKKβ/NF-κB in central control of blood glucose, blood pressure, and body weight. Cell Metab. (2017) 25:1091–102. doi: 10.1016/j.cmet.2017.04.002
73. Briz V, Liu Y, Zhu G, Bi X, Baudry M. A novel form of synaptic plasticity in field CA3 of hippocampus requires GPER1 activation and BDNF release. J Cell Biol. (2015) 210:1225–37. doi: 10.1083/jcb.201504092
74. Thorsdottir D, Cruickshank CN, Einwag Z, Hennig WG. BDNF downregulates β-adrenergic receptor-mediated hypotensive mechanisms in the paraventricular nucleus of the hypothalamus. Am J Physiol Heart Circ Physiol. (2019) 17:H1258–71. doi: 10.1152/ajpheart.00478.2019
75. Kishi T, Hirooka Y, Nagayama T, Isegawa K, Takesue and K. Sunagawa K. Calorie restriction improves cognitive decline via up-regulation of brain-derived neurotrophic factor: tropomyosin-related kinase B in hippocampus ofobesity-induced hypertensive rats. Int Heart J. (2015) 56:110–5. doi: 10.1536/ihj.14-168
76. Wang T, Maltez TM, Lee WH, Ahmad M, Wang WH, Leenen HF. Effect of exercise training on the FNDC5/BDNF pathway in spontaneously hypertensive rats. Physiol Rep. (2019) 7:e14323. doi: 10.14814/phy2.14323
77. Monnier A, Garnier P, Quirie A, Pernet N, Demougeot C, Marie and A. Prigent-Tessier C. Effect of short-term exercise training on brain-derived neurotrophic factor signaling in spontaneously hypertensive rats. J hypertension. (2017) 35:279–90. doi: 10.1097/HJH.0000000000001164
78. Prigent-Tessier A, Quirié A, Maguin-Gaté K, Szostak J, Mossiat C, Nappey M, et al. Physical training and hypertension have opposite effects on endothelial brain-derived neurotrophic factor expression. Cardiovasc Res. (2013) 100:374–82. doi: 10.1093/cvr/cvt219
79. Roh TH, So YW, Cho YS, Suh HS. Effects of fluid ingestion on brain-derived neurotrophic factor and cognition during exercise in the heat. J Hum Kinet. (2017) 58:73–86. doi: 10.1515/hukin-2017-0074
80. Chaturvedi P, Singh KA, Tiwari V, Thacker KA. Post-stroke BDNF concentration changes following proprioceptive neuromuscular facilitation (PNF) exercises. J Family Med Prim Care. (2020) 9:3361–9. doi: 10.4103/jfmpc.jfmpc_1051_19
81. Ping G, Qian W, Song G, Zhaochun S. Valsartan reverses depressive/anxiety-like behavior and induces hippocampal neurogenesis and expression of BDNF protein in unpredictable chronic mild stress mice. Pharmacol Biochem Behav. (2014) 124:5–12. doi: 10.1016/j.pbb.2014.05.006
82. Sahay SA, Jadhav TA, Sundrani PD, Wagh NG, Joshi RS. Differential expression of nerve growth factor (NGF) and brain derived neurotrophic factor (BDNF) in different regions of normal and preeclampsia placentae. Clin Exp Hypertens. (2020) 42:360–4. doi: 10.1080/10641963.2019.1665677
83. Hutchinson JA, Chou LC, Israel DD, Xu W, Regan WJ. Activation of EP2 prostanoid receptors in human glial cell lines stimulates the secretion of BDNF. Neurochem Int. (2009) 54:439–46. doi: 10.1016/j.neuint.2009.01.018
84. Giralt A, Friedman CH, Caneda-Ferron B, Urban N, Moreno E, Rubio N, et al. BDNF regulation under GFAP promoter provides engineered astrocytes as a new approach for long-term protection in Huntington’s disease. Gene Ther. (2010) 17:1294–308. doi: 10.1038/gt.2010.71
85. Gomes C, Ferreira R, George J, Sanches R, Rodrigues ID, Goncalves N, et al. Activation of microglial cells triggers a release of brain-derived neurotrophic factor (BDNF) inducing their proliferation in an adenosine A2A receptor-dependent manner. A2A receptor blockade prevents BDNF release and proliferation of microglia. Neuroinflammation. (2013) 10:16. doi: 10.1186/1742-2094-10-16
86. Zhou JL, Peng J, Xu NY, Zeng JW, Zhang J, Wei X, et al. Microglia are indispensable for synaptic plasticity in the spinal dorsal horn and chronic pain. Cell Rep. (2019) 27:3844–59. doi: 10.1016/j.celrep.2019.05.087
87. Long T, He W, Pan Q, Zhang S, Zhang D, Qin G, et al. Microglia P2X4R-BDNF signalling contributes to central sensitization in a recurrent nitroglycerin-induced chronic migraine model. J Headache Pain. (2020) 21:4. doi: 10.1186/s10194-019-1070-4
88. Lao-Peregrín C, Ballesteros JJ, Fernández M, Zamora-Moratalla A, Saavedra A, Gómez Lázaro M, et al. Caffeine-mediated BDNF release regulates long-term synaptic plasticity through activation of IRS2 signaling. Addict Biol. (2017) 22:1706–18. doi: 10.1111/adb.12433
89. Datta I, Ganapathy K, Razdan and R. Bhonde R. Location and number of astrocytes determine dopaminergic neuron survival and function under 6-OHDA stress mediated through differential BDNF release. Mol Neurobiol. (2018) 55:5505–25. doi: 10.1007/s12035-017-0767-0
90. Begliuomini S, Casarosa E, Pluchino N, Lenzi E, Centofanti M, Freschi L, et al. Influence of endogenous and exogenous sex hormones on plasma brain-derived neurotrophic factor. Hum Reprod. (2007) 22:995–1002. doi: 10.1093/humrep/del479
91. Shetty KA, Kodali M, Upadhya R, Madhu NL. Emerging anti-aging strategies - scientific basis and efficacy. Aging Dis. (2018) 9:1165–84. doi: 10.14336/AD.2018.1026
92. Walsh JJ, Tschakovsky EM. Exercise and circulating BDNF: Mechanisms of release and implications for the design of exercise interventions. Appl physiology nutrition Metab = Physiologie appliquee Nutr metabolisme. (2018) 43:1095–110. doi: 10.1139/apnm-2018-0192
93. Gilder M, Ramsbottom R, Currie J, Sheridan B, Nevill MA. Effect of fat free mass on serum and plasma BDNF concentrations during exercise and recovery in healthy young men. Neurosci Lett. (2014) 560:137–41. doi: 10.1016/j.neulet.2013.12.034
94. Aarse J, Herlitze S, Manahan-Vaughan D. The requirement of BDNF for hippocampal synaptic plasticity is experience-dependent. Hippocampus. (2016) 26(6):739–51. doi: 10.1002/hipo.22555
95. Alomari AM, Khabour FO, Alzoubi HK, Alzubi AM. Forced and voluntary exercises equally improve spatial learning and memory and hippocampal BDNF levels. Behav Brain Res. (2013) 247:34–9. doi: 10.1016/j.bbr.2013.03.007
96. Stawicka A, Świderska M, Zbrzeźniak J, Sołowianowicz N, Woszczenko A, Flisiak R, et al. Brain-derived neurotrophic factor as a potential diagnostic marker in minimal hepatic encephalopathy. Clin Exp Hepatol. (2021) 7:117–24. doi: 10.5114/ceh.2021.103242
97. Meshkat S, Alnefeesi Y, Jawad YM, Ceban F, Mw Lui L, McIntyre SR, et al. Brain-Derived Neurotrophic Factor (BDNF) as a biomarker of treatment response in patients with Treatment Resistant Depression (TRD): A systematic review & meta-analysis. Psychiatry Res. (2022) 317:114857. doi: 10.1016/j.psychres.2022.114857
98. Ismael S S, Mirzahosseini G, Ahmed AH, Yoo A A, Kassan M, Malik and T. Ishrat UK. Renin-angiotensin system alterations in the human alzheimer’s disease brain. J Alzheimer’s Dis. (2021) 84:1473–84. doi: 10.3233/JAD-215051
99. Polyakova M, Stuke K, Schuemberg K, Mueller K, Schoenknecht P, Schroeter LM. BDNF as a biomarker for successful treatment of mood disorders: a systematic & quantitative meta-analysis. J Affect Disord. (2015) 174:432–40. doi: 10.1016/j.jad.2014.11.044
100. Libman-Sokolowska M, Drozdowicz E, Nasierowski T. BDNF as a biomarker in the course and treatment of schizophrenia. Psychiatria polska. (2015) 49:1149–58. doi: 10.12740/PP/37705
101. Zheleznyakova YG, Cao H, Schiöth HB BH. BDNF DNA methylation changes as a biomarker of psychiatric disorders: literature review and open access database analysis. Behav Brain functions: BBF. (2016) 12:17. doi: 10.1186/s12993-016-0101-4
102. Selvaraju V, Babu RJ, Geetha T. Salivary neurotrophins brain-derived neurotrophic factor and nerve growth factor associated with childhood obesity: A multiplex magnetic luminescence analysis. Diagnostics (Basel Switzerland). (2022) 12:1130. doi: 10.3390/diagnostics12051130
103. Brunoni RA, Lotufo AP, Sabbag C, Goulart CA, Santos SI, Benseñor MI. Decreased brain-derived neurotrophic factor plasma levels in psoriasis patients., Brazilian journal of medical and biological research. Braz J Med Biol Res. (2015) 48(8):711–4. doi: 10.1590/1414-431x20154574
104. Schutte EC, Malan L, Scheepers DJ, Oosthuizen W, Cockeran M, Malan TN. Cortisol:brain-derived neurotrophic factor ratio associated with silent ischaemia in a black male cohort: the SA BPA study. Cardiovasc J Afr. (2016) 27:387–91. doi: 10.5830/CVJA-2016-065
105. Igarashi T, Nakamoto K, Kobayashi M, Suzuki H, Tobita Y, Igarashi T, et al. Serum brain-derived neurotrophic factor in glaucoma patients in Japan: an observational study., journal of nippon medical school. J Nippon Med Sch. (2021) 87(6):339–45. doi: 10.1272/jnms.JNMS.2020_87-605
106. Komulainen P, Pedersen M, Hänninen T, Bruunsgaard H, Lakka AT, Kivipelto M, et al. BDNF is a novel marker of cognitive function in ageing women: the DR’s EXTRA Study. Neurobiol Learn Memory. (2008) 90:596–603. doi: 10.1016/j.nlm.2008.07.014
107. Wallace K, Bowles T, Griffin A, Robinson R, Solis L, Railey T, et al. Evidence of anxiety, depression and learning impairments following prenatal hypertension. Behav Sci (Basel). (2022) 12:53. doi: 10.3390/bs12020053
108. Castello AN, Green NK, LaFerla MF. Genetic knockdown of brain-derived neurotrophic factor in 3xTg-AD mice does not alter Aβ or tau pathology. PloS One. (2012) 7:e39566. doi: 10.1371/journal.pone.0039566
109. Brigadski T, Leßmann V. The physiology of regulated BDNF release. Cell Tissue Res. (2020) 382:15–45. doi: 10.1007/s00441-020-03253-2
110. Mahmoud R, Kimonis V, Butler GM. Genetics of obesity in humans: A clinical review. Int J Mol Sci. (2022) 23:11005. doi: 10.3390/ijms231911005
111. Lu EC, Chan BC. Is brain-derived neurotrophic factor a metabolic hormone in peripheral tissues. Biol (Basel). (2022) 11:1063. doi: 10.3390/biology11071063
112. Han CJ, Liu RQ, Jones M, Levinn LR, Menzie MC, Jefferson-George SK, et al. Brain-derived neurotrophic factor and obesity in the WAGR syndrome. N Engl J Med. (2008) 359:918–27. doi: 10.1056/NEJMoa0801119
113. Unger JT, Calderon AG, Bradley CL, Sena-Esteves M, Rios M. Selective deletion of Bdnf in the ventromedial and dorsomedial hypothalamus of adult mice results in hyperphagic behavior and obesity. J Neurosci. (2007) 2752:14265–1474. doi: 10.1523/JNEUROSCI.3308-07.2007
114. Heine AP, Taylor AJ, Iwamoto AG, Lubahn BD, Cooke SP. Increased adipose tissue in male and female estrogen receptor-alpha knockout mice. Proc Natl Acad Sci U.S.A. (2000) 97:12729–34. doi: 10.1073/pnas.97.23.12729
115. Saito K, He Y, Yang Y, Zhu L, Wang C, Xu P, et al. PI3K in the ventromedial hypothalamic nucleus mediates estrogenic actions on energy expenditure in female mice. Sci Rep. (2016) 6:23459. doi: 10.1038/srep23459
116. Smith AW, Bosch MA, Wagner EJ, Rønnekleiv OK, Kelly MJ. The membrane estrogen receptor ligand STX rapidly enhances GABAergic signaling in NPY/AgRP neurons: role in mediating the anorexigenic effects of 17β-estradiol. Am J Physiol Endocrinol Metab. (2013) 305:E632–40. doi: 10.1152/ajpendo.00281.2013
117. Malyala A, Zhang C, Bryant DN, Kelly MJ, Rønnekleiv OK. PI3K signaling effects in hypothalamic neurons mediated by estrogen. J Comp Neurol. (2008) 506:895–911. doi: 10.1002/cne.v506:6
118. Musatov S, Chen W, Pfaff WD, Mobbs VC, Yang JX, Clegg JD, et al. Silencing of estrogen receptor alpha in the ventromedial nucleus of hypothalamus leads to metabolic syndrome. Proc Natl Acad Sci U S A. (2007) 104:. 2501–2506. doi: 10.1073/pnas.0610787104
119. Mahmood A, Uddin MM, Ibrahim M, Briski KP. Norepinephrine regulation of ventromedial hypothalamic nucleus metabolic-sensory neuron 5’-AMP-activated protein kinase activity: impact of estradiol. Int J Mol Sci. (2020) 21:2013. doi: 10.3390/ijms21062013
120. Mauvais-Jarvis F, Clegg DJ, Hevener AL. The role of estrogens in control of energy balance and glucose homeostasis. Endocr Rev. (2013) 34:309–38. doi: 10.1210/er.2012-1055
121. Mair MK, Gaw R, MacLean RM. Obesity, estrogens and adipose tissue dysfunction - implications for pulmonary arterial hypertension. Pulm Circ. (2020) 10:2045894020952019. doi: 10.1177/2045894020952023
122. Trouillet CA, Ducroq S, Naulé L, Capela D, Parmentier C, Radovick S, et al. Deletion of neural estrogen receptor alpha induces sex differential effects on reproductive behavior in mice. Commun Biol. (2022) 5:383. doi: 10.1038/s42003-022-03324-w
123. Chow DJ, Jones EM, Prelle K, Simpson RE, Boon WC CW. A selective estrogen receptor α agonist ameliorates hepatic steatosis in the male aromatase knockout mouse. J Endocrinol. (2011) 210:323–34. doi: 10.1530/JOE-10-0462
124. Haddad-Tóvolli R, Claret M. Metabolic and feeding adjustments during pregnancy. Nat Rev Endocrinol. (2023) 19:564–80. doi: 10.1038/s41574-023-00871-y
125. Harmancıoğlu B, Kabaran S. Maternal high fat diets: impacts on offspring obesity and epigenetic hypothalamic programming. Front Genet. (2023) 14:1158089. doi: 10.3389/fgene.2023.1158089
126. Rios M. BDNF and the central control of feeding: accidental bystander or essential player? Trends Neurosci. (2013) 36:83–90. doi: 10.1016/j.tins.2012.12.009
127. Grzelak N, Kaczmarek D, Mrówczyński W. Comparison of the effects of BDNF/TRKB signalling on metabolic biomarkers in the liver of sedentary and trained rats with normal and knockout BDNF genotypes. Front Physiol. (2023) 14:1268648. doi: 10.3389/fphys.2023.1268648
128. Hashimoto K, Koizumi H, Nakazato M, Shimizu and M. Iyo E. Role of brain-derived neurotrophic factor in eating disorders: recent findings and its pathophysiological implications, Prog Neuropsychopharmacol Biol. Psychiatry. (2005) 29:499–504. doi: 10.1016/j.pnpbp.2005.01.007
129. Chang Q, Khare G, Dani V, Nelson and R. Jaenisch S. The disease progression of Mecp2 mutant mice is affected by the level of BDNF expression. Neuron. (2006) 49:341–8. doi: 10.1016/j.neuron.2005.12.027
130. Tsuchida A, Nonomura T, Ono-Kishino M, Nakagawa T, Taiji and H. Noguchi M. Acute effects of brain-derived neurotrophic factor on energy expenditure in obese diabetic mice. Int J Obes Relat Metab Disord. (2001) 25:1286–93. doi: 10.1038/sj.ijo.0801678
131. Zhu Z, Liu X, Senthil Kumar PS, Zhang and H. Shi J. Central expression and anorectic effect of brain-derived neurotrophic factor are regulated by circulating estradiol levels. Horm Behav. (2013) 63:533–42. doi: 10.1016/j.yhbeh.2013.01.009
132. Ganipisetti VM, Bollimunta P. Obesity and set-point theory. Treasure Island (FL: StatPearls Publishing (2023).
133. Lu Y, Sareddy RG, Wang J, Wang R, Li Y, Dong Y, et al. Neuron-derived estrogen regulates synaptic plasticity and memory. J Neurosci. (2019) 39:2792–809. doi: 10.1523/JNEUROSCI.1970-18.2019
134. Hetemäki N, Savolainen-Peltonen H, Tikkanen MJ, Wang F, Paatela H, Hämäläinen E, et al. Estrogen metabolism in abdominal subcutaneous and visceral adipose tissue in postmenopausal women. J Clin Endocrinol Metab. (2017) 102:4588–95. doi: 10.1210/jc.2017-01474
135. Cone RD, Cowley MA, Butler AA, Fan W, Marks DL, Low MJ. The arcuate nucleus as a conduit for diverse signals relevant to energy homeostasis. Int J Obes Relat Metab Disord. (2001) Suppl 5:S63–67. doi: 10.1038/sj.ijo.0801913
136. González-García I, García-Clavé E, Cebrian-Serrano A, Le Thuc O, Contreras RE, Xu Y, et al. Estradiol regulates leptin sensitivity to control feeding via hypothalamic Cited1. Cell Metab. (2023) 35:438–55. doi: 10.1016/j.cmet.2023.02.004
137. Stincic TL, Grachev P, Bosch MA, Rønnekleiv OK, Kelly MJ. Estradiol drives the anorexigenic activity of proopiomelanocortin neurons in female mice. eNeuro. (2018) 5:ENEURO.0103–18. doi: 10.1523/ENEURO.0103-18.2018
138. Yasrebi A, Regan D, Roepke TA. The influence of estrogen response element ERα signaling in the control of feeding behaviors in male and female mice. Steroids. (2023) 195:109228. doi: 10.1016/j.steroids.2023.109228
139. Srivastava DP, Waters EM, Mermelstein PG, Kramár EA, Shors and F. Liu TJ. Rapid estrogen signaling in the brain: implications for the fine-tuning of neuronal circuitry. J Neurosci. (2011) 31:16056–63. doi: 10.1523/JNEUROSCI.4097-11.2011
140. Dhillon SS, Belsham DD. Estrogen inhibits NPY secretion through membrane-associated estrogen receptor (ER)-α in clonal, immortalized hypothalamic neurons. Int J Obes (Lond). (2011) 35:198–207. doi: 10.1038/ijo.2010.124
141. Mahboobifard F, Pourgholami HM, Jorjani M, Dargahi L, Amiri M, Sadeghi S, et al. Estrogen as a key regulator of energy homeostasis and metabolic health. BioMed Pharmacother. (2022) 156:113808. doi: 10.1016/j.biopha.2022.113808
142. Gao Q, Mezei G, Nie Y, Rao Y, Choi SC, Bechmann I, et al. Anorectic estrogen mimics leptin’s effect on the rewiring of melanocortin cells and Stat3 signaling in obese animals. Nat Med. (2007) 13:89–94. doi: 10.1038/nm1525
143. Jo HY. Endogenous BDNF regulates inhibitory synaptic transmission in the ventromedial nucleus of the hypothalamus. J Neurophysiol. (2012) 107:42–9. doi: 10.1152/jn.00353.2011
144. Wang C, Bomberg E, Billington JC, Levine SA, Kotz MC. Brain-derived neurotrophic factor (BDNF) in the hypothalamic ventromedial nucleus increases energy expenditure. Brain Res. (2010) 1336:66–77. doi: 10.1016/j.brainres.2010.04.013
145. Martínez de Morentin BP, González-García I, Martins L, Lage R, Fernández-Mallo D, Martínez-Sánchez N, et al. Estradiol regulates brown adipose tissue thermogenesis via hypothalamic AMPK. Cell Metab. (2014) 20:41–53. doi: 10.1016/j.cmet.2014.03.031
146. Wenner MM, Greaney LJ, Matthews LE, McGinty S S, Kaur J, Vongpatanasin W, et al. Influence of age and estradiol on sympathetic nerve activity responses to exercise in women. Med Sci Sports Exerc. (2022) 54:408–16. doi: 10.1249/MSS.0000000000002823
147. Asarian L, Geary N. Estradiol enhances cholecystokinin-dependent lipid-induced satiation and activates estrogen receptor-α-expressing cells in the nucleus tractus solitarius of ovariectomized rats. Endocrinology. (2007) 148:5656–66. doi: 10.1210/en.2007-0341
148. Thammacharoen S, Lutz TA, Geary N, Asarian L. Hindbrain administration of estradiol inhibits feeding and activates estrogen receptor-alpha-expressing cells in the nucleus tractus solitarius of ovariectomized rats. Endocrinology. (2008) 149:1609–17. doi: 10.1210/en.2007-0340
149. Chi JH, Narita K, Ichimaru T, Murata T. Estrogen Increases c-Fos expression in the paraventricular nucleus along with its anorexic effect in developing rats. J Reprod Dev. (2011) 57:. 365–372. doi: 10.1262/jrd.10-189E
150. Shen L, Wang DQ, Lo CM, Tso P, Davidson WS, Woods SC, et al. Estradiol increases the anorectic effect of central apolipoprotein A-IV. Endocrinology. (2010) 151:3163–8. doi: 10.1210/en.2010-0203
151. Maske CB, Jackson CM, Terrill SJ, Eckel L, Williams DL. Estradiol modulates the anorexic response to central glucagon-like peptide 1. Horm Behav. (2017) 93:109–17. doi: 10.1016/j.yhbeh.2017.05.012
152. Shen L, Wang DQ, Xu M, Woods SC, Liu M. BDNF/TrkB signaling mediates the anorectic action of estradiol in the nucleus tractus solitarius. Oncotarget. (2017) 8:84028–38. doi: 10.18632/oncotarget.21062
153. Song DH, Kim NS, Saha A, Ahn YS, Akindehin S, Son Y, et al. Aging-induced brain-derived neurotrophic factor in adipocyte progenitors contributes to adipose tissue dysfunction. Aging Dis. (2020) 11:575–87. doi: 10.14336/AD.2019.0810
154. Sakata K, Kobayashi T, Yokokura S, Fukuchi M. Early macrophage-mediated Bdnf expression in white adipose tissue during high-fat diet feeding. Biochem Biophys Res Commun. (2023) 686:149163. doi: 10.1016/j.bbrc.2023.149163
155. Akça İ, Bodur A, Kahraman C, Abidin İ, Aydın-Abidin and A. Alver S. The regulation of adipokines related to obesity and diabetes is sensitive to BDNF levels and adipose tissue location. Hormones (Athens). (2022) 21:295–303. doi: 10.1007/s42000-022-00364-z
156. Zhou Z, Moore MT, Drew GB, Ribas V, Wanagat J, Civelek M, et al. Estrogen receptor α controls metabolism in white and brown adipocytes by regulating Polg1 and mitochondrial remodeling. Sci Transl Med. (2020) 12:eaax8096. doi: 10.1126/scitranslmed.aax8096
157. Rogers HN, Witczak AC, Hirshman FM, Goodyear JL, Greenberg SA. Estradiol stimulates Akt, AMP-activated protein kinase (AMPK) and TBC1D1/4, but not glucose uptake in rat soleus. Biochem Biophys Res Commun. (2009) 382:646–50. doi: 10.1016/j.bbrc.2009.02.154
158. Gao H, Bryzgalova G, Hedman E, Khan A, Efendic S, Gustafsson and K. Dahlman-Wright AJ. Long-term administration of estradiol decreases expression of hepatic lipogenic genes and improves insulin sensitivity in ob/ob mice: a possible mechanism is through direct regulation of signal transducer and activator of transcription 3. Mol Endocrinol. (2006) 20:1287–99. doi: 10.1210/me.2006-0012
159. Miller CN, Della-Fera MA, Baile CA. The mediation of hepatic lipogenesis through estrogens. Postdoc J. (2013) 1:27–38. doi: 10.14304/SURYA.JPR.V1N5.4
160. Bitirim CV, Ozer ZB, Akcali KC. Estrogen receptor alpha regulates the expression of adipogenic genes genetically and epigenetically in rat bone marrow-derived mesenchymal stem cells. PeerJ. (2021) 9:e12071. doi: 10.7717/peerj.12071
161. Lovejoy CJ, Sainsbury A, Stock Conference 2008 Working Group. Sex differences in obesity and the regulation of energy homeostasis. Obes Rev. (2009) 10:154–67. doi: 10.1111/j.1467-789X.2008.00529.x
162. Steiner MB, Berry CD. The regulation of adipose tissue health by estrogens. Front Endocrinol (Lausanne). (2022) 13:889923. doi: 10.3389/fendo.2022.889923
163. Lizcano F, Guzmán G. Estrogen deficiency and the origin of obesity during menopause. BioMed Res Int. (2014) 2014:757461. doi: 10.1155/2014/757461
164. Patel S, Homaei A, Raju BA, Meher RB. Estrogen: The necessary evil for human health, and ways to tame it. BioMed Pharmacother. (2018) 102:403–11. doi: 10.1016/j.biopha.2018.03.078
165. Rettberg RJ, Yao J, Brinton DR. Estrogen: a master regulator of bioenergetic systems in the brain and body. Front Neuroendocrinol. (2014) 35:8–30. doi: 10.1016/j.yfrne.2013.08.001
166. Naaz A, Zakroczymski M, Heine P, Taylor J, Saunders P, Lubahn D, et al. Effect of ovariectomy on adipose tissue of mice in the absence of estrogen receptor alpha (ERalpha): a potential role for estrogen receptor beta (ERbeta). Horm Metab Res. (2002) 34:758–63. doi: 10.1055/s-2002-38259
167. Cheon PY, Ko C, Lee HK. Assessment of adipocyte differentiation and maturation-related gene expression in the epididymal fat of estrogen receptor α Knockout (ERαKO) mouse during postnatal development period. Dev Reprod. (2020) 24:287–96. doi: 10.12717/DR.2020.24.4.287
168. Palin LS, McTernan GP, Anderson AL, Sturdee WD, Barnett and S. Kumar HA. 17Beta-estradiol and anti-estrogen ICI:compound 182,780 regulate expression of lipoprotein lipase and hormone-sensitive lipase in isolated subcutaneous abdominal adipocytes. Metabolism. (2003) 52:383–8. doi: 10.1053/meta.2003.50088
169. Pinto S, Roseberry AG, Liu H, Diano S, Shanabrough M, Cai X, et al. Rapid rewiring of arcuate nucleus feeding circuits by leptin. Science. (2004) 304:110–5. doi: 10.1126/science.1089459
170. Wang P, Loh HK, Wu M, Morgan AD, Schneeberger M, Yu X, et al. A leptin-BDNF pathway regulating sympathetic innervation of adipose tissue. Nature. (2020) 583:839–44. doi: 10.1038/s41586-020-2527-y
171. Vohra MS, Benchoula K, Serpell CJ, Hwa WE. AgRP/NPY and POMC neurons in the arcuate nucleus and their potential role in treatment of obesity. Eur J Pharmacol. (2022) 915:174611. doi: 10.1016/j.ejphar.2021.174611
172. Sohn JW. JW. Network of hypothalamic neurons that control appetite. BMB Rep. (2015) 48:229–33. doi: 10.5483/BMBRep.2015.48.4.272
173. Xu B, Goulding EH, Zang K, Cepoi D, Cone RD, Jones KR, et al. Brain-derived neurotrophic factor regulates energy balance downstream of melanocortin-4 receptor. Nat Neurosci. (2003) 6:. 736–742. doi: 10.1038/nn1073
174. Ramírez D, Saba J, Carniglia L, Durand D, Lasaga and C. Caruso M. Melanocortin 4 receptor activates ERK-cFos pathway to increase brain-derived neurotrophic factor expression in rat astrocytes and hypothalamus. Mol Cell Endocrinol. (2015) 411:28–37. doi: 10.1016/j.mce.2015.04.008
175. Chu P, Guo W, You and B. Lu H. Regulation of satiety by bdnf-e2-expressing neurons through trkB activation in ventromedial hypothalamus. Biomolecules. (2023) 13:822. doi: 10.3390/biom13050822
176. King BM. The rise, fall, and resurrection of the ventromedial hypothalamus in the regulation of feeding behavior and body weight. Physiol Behav. (2006) 87:221–44. doi: 10.1016/j.physbeh.2005.10.007
177. Toda C, Santoro A, Kim and S. Diano JD. POMC neurons: from birth to death. Annu Rev Physiol. (2017) 79:209–36. doi: 10.1146/annurev-physiol-022516-034110
178. Ye H, Yang X, Feng B, Luo P, Torres IV, Carrillo-Sáenz L, et al. 27-Hydroxycholesterol acts on estrogen receptor α expressed by POMC neurons in the arcuate nucleus to modulate feeding behavior. Sci Adv. (2024) 1028:eadi4746. doi: 10.1126/sciadv.adi4746
179. Fagan MP, Ameroso D, Meng A, Rock A, Maguire and M. Rios J. Essential and sex-specific effects of mGluR5 in ventromedial hypothalamus regulating estrogen signaling and glucose balance. Proc Natl Acad Sci U.S.A. (2020) 117:19566–77. doi: 10.1073/pnas.2011228117
180. Xu Y, Nedungadi TP, Zhu L, Sobhani N, Irani BG, Davis KE, et al. Distinct hypothalamic neurons mediate estrogenic effects on energy homeostasis and reproduction. Cell Metab. (2011) 14:453–65. doi: 10.1016/j.cmet.2011.08.009
181. Wang A, Luo J, Moore W, Alkhalidy H, Wu L, Zhang J, et al. GPR30 regulates diet-induced adiposity in female mice and adipogenesis in vitro. Sci Rep. (2016) 6:34302. doi: 10.1038/srep34302
182. Liao YG, An JJ, Gharami K, Waterhouse EG GE, Vanevski F, Jones and B. Xu RK. Dendritically targeted Bdnf mRNA is essential for energy balance and response to leptin. Nat Med. (2012) 18:564–71. doi: 10.1038/nm.2687
183. Neyens DM, Brenner L, Calkins R, Winzenried ET, Ritter RC, Appleyard SM. CCK-sensitive C fibers activate NTS leptin receptor-expressing neurons via NMDA receptors. Am J Physiol Regul Integr Comp Physiol. (2024) 326:R383–400. doi: 10.1152/ajpregu.00238.2022
184. Geary N. Estradiol, CCK and satiation. Peptides. (2001) 22:1251–63. doi: 10.1016/S0196-9781(01)00449-1
185. Shen L, Liu Y, Wang DQ, Tso P, Woods and M. Liu SC. Estradiol stimulates apolipoprotein A-IV gene expression in the nucleus of the solitary tract through estrogen receptor-α. Endocrinology. (2014) 15510:3882–90. doi: 10.1210/en.2014-1239
186. Liu M, Shen L, Xu M, Wang and P. Tso DQ. Estradiol enhances anorectic effect of apolipoprotein A-IV through ERα-PI3K pathway in the nucleus tractus solitarius. Genes (Basel). (2020) 11:1494. doi: 10.3390/genes11121494
187. Kupczyk D, Bilski R, Kozakiewicz M, Studzińska R, Kędziora-Kornatowska K, Kosmalski T, et al. 11β-HSD as a new target in pharmacotherapy of metabolic diseases. Int J Mol Sci. (23) 16:8984. doi: 10.3390/ijms23168984
188. Garvey WT. Is obesity or adiposity-based chronic disease curable: the set point theory, the environment, and second-generation medications. Endocr Pract. (2022) 28:214–22. doi: 10.1016/j.eprac.2021.11.082
189. Li C, Meng F, Lei Y, Liu J, Liu J, Zhang J, et al. Leptin regulates exon-specific transcription of the Bdnf gene via epigenetic modifications mediated by an AKT/p300 HAT cascade. Mol Psychiatry. (2021) 26:3701–22. doi: 10.1038/s41380-020-00922-0
190. Gao Q, Horvath LT. Cross-talk between estrogen and leptin signaling in the hypothalamus. Am J Physiol Endocrinol Metab. (2008) 294:E817–826. doi: 10.1152/ajpendo.00733.2007
191. Caron A, Dungan LH, Castorena CM, Fujikawa T, Lee S, Lord CC, et al. POMC neurons expressing leptin receptors coordinate metabolic responses to fasting via suppression of leptin levels. Elife. (2018) 7:e33710. doi: 10.7554/eLife.33710.014
192. Zhan C. POMC neurons: feeding, energy metabolism, and beyond. Adv Exp Med Biol. (2018) 1090:17–29. doi: 10.1007/978-981-13-1286-1_2
193. Cao L, Liu X, Lin JE, Wang C, Choi YE, Riban V, et al. Environmental and genetic activation of a brain-adipocyte BDNF/leptin axis causes cancer remission and inhibition. Cell. (2010) 142:52–64. doi: 10.1016/j.cell.2010.05.029
194. Martínez-Sánchez N. There and back again: leptin actions in white adipose tissue. Int J Mol Sci. (2020) 21:6039. doi: 10.3390/ijms21176039
195. Licinio J, Negrão BA, Mantzoros C, Kaklamani V, Wong LM, Bongiorno BP, et al. Synchronicity of frequently sampled, 24-h concentrations of circulating leptin, luteinizing hormone, and estradiol in healthy women. Proc Natl Acad Sci U.S.A. (1998) 95:2541–6. doi: 10.1073/pnas.95.5.2541
196. Barrios-Correa AA, Estrada AJ, Contreras I. Leptin signaling in the control of metabolism and appetite: lessons from animal models. J Mol Neurosci. (2018) 66:390–402. doi: 10.1007/s12031-018-1185-0
197. Brown LM, Clegg DJ. Estrogen and leptin regulation of endocrinological features of anorexia nervosa. Neuropsychopharmacology. (2013) 38:237. doi: 10.1038/npp.2012.176
198. Catalano S, Mauro L, Marsico S, Giordano C, Rizza and V. Rago P. Leptin induces, via ERK1/ERK2 signal, functional activation of estrogen receptor alpha in MCF-7 cells. J Biol Chem. (2004) 279:19908–15. doi: 10.1074/jbc.M313191200
199. Madak-Erdogan Z, Kim SH, Gong P, Zhao YC, Zhang H, Chambliss KL, et al. Design of pathway preferential estrogens that provide beneficial metabolic and vascular effects without stimulating reproductive tissues. Sci Signal. (2016) 9:ra53. doi: 10.1126/scisignal.aad8170
200. Zuo Q, Chen KL, Arredondo Eve A, Liu YJ, Kim SH, Katzenellenbogen BS, et al. Pathway preferential estrogens prevent hepatosteatosis due to ovariectomy and high-fat diets. Nutrients. (2021) 13:3334. doi: 10.3390/nu13103334
201. Raut KP, Choi YD, Kim HS, Hong TJ, Kwon KT, Jeong HJ, et al. Estrogen receptor signaling mediates leptin-induced growth of breast cancer cells via autophagy induction. Oncotarget. (2017) 8:109417–35. doi: 10.18632/oncotarget.22684
202. Ahuja P, Ng FC, Pang SB, Chan SW, Tse LM, Bi X, et al. Muscle-generated BDNF (brain derived neurotrophic factor) maintains mitochondrial quality control in female mice. Autophagy. (2022) 18:1367–84. doi: 10.1080/15548627.2021.1985257
203. Xue B, Zhang Z, Beltz GT, Guo F, Hay M, Johnson KA. Estrogen regulation of the brain renin-angiotensin system in protection against. Am J Physiol Heart Circ Physiol. (2014) 307:H191–198. doi: 10.1152/ajpheart.01012.2013
204. Manti S, Xerra F, Spoto G, Butera A, Gitto E, Di Rosa G, et al. Neurotrophins: expression of brain-lung axis development. Int J Mol Sci. (2023) 24:7089. doi: 10.3390/ijms24087089
205. Prager-Khoutorsky M, Choe YK, Levi ID, Bourque WC. role of vasopressin in rat models of salt-dependent hypertension. Curr Hypertens Rep. (2017) 19:42. doi: 10.1007/s11906-017-0741-2
206. Hasegawa Y, Nakagawa T, Uekawa K, Ma M, Lin B, Kusaka H, et al. Therapy with the combination of amlodipine and irbesartan has persistent preventative effects on stroke onset associated with BDNF preservation on cerebral vessels in hypertensive rats. Transl Stroke Res. (2016) 7:79–87. doi: 10.1007/s12975-014-0383-5
207. Balapattabi K, Little TJ, Farmer EG, Cunningham TJ. High salt loading increases brain derived neurotrophic factor in supraoptic vasopressin neurones. J Neuroendocrinol. (2018) 30:e12639. doi: 10.1111/jne.2018.30.issue-11
208. Zhang LC, Lin ZY, Wu Q, Yan C, Wong WM, Zeng F, et al. Arcuate NPY is involved in salt-induced hypertension via modulation of paraventricular vasopressin and brain-derived neurotrophic factor. J Cell Physiol. (2022) 237:2574–88. doi: 10.1002/jcp.v237.5
209. Becker KB, Wang H, Zucker HI. Central TrkB blockade attenuates ICV angiotensin II-hypertension and sympathetic nerve activity in male Sprague-Dawley rats. Auton Neurosci. (2017) 205:77–86. doi: 10.1016/j.autneu.2017.05.009
210. Lommatzsch M, Zingler D, Schuhbaeck K, Schloetcke K, Zingler C, Schuff-Werner P, et al. The impact of age, weight and gender on BDNF levels in human platelets and plasma. Neurobiol Aging. (2005) 26:115–23. doi: 10.1016/j.neurobiolaging.2004.03.002
211. Demir M, Gürol OA, Özyiğit TR, Üresin YA. effects of blood pressure lowering with different antihypertensive agents on cognitive function and plasma brain-derived neurotrophic factor levels: A comparative study. J Cardiovasc Pharmacol. (2016) 67:538–43. doi: 10.1097/FJC.0000000000000377
212. Seifert B, Eckenstaler R, Ronicke R, Leschik J, Lutz B, Reymann K, et al. Amyloid-beta induced changes in vesicular transport of BDNF in hippocampal neurons. Neural Plast. (2016) 2016:4145708. doi: 10.1155/2016/4145708
213. Merlo S, Spampinato FS, Beneventano M, Sortino AM. The contribution of microglia to early synaptic compensatory responses that precede beta-amyloid-induced neuronal death. Sci Rep. (2018) 8:7297. doi: 10.1038/s41598-018-25453-1
214. Xue B, Zhang Z, Johnson FR, Johnson KA. Sensitization of slow pressor angiotensin II (Ang II)-initiated hypertension: induction of sensitization by prior Ang II treatment. Hypertension. (2012) 59:459–66. doi: 10.1161/HYPERTENSIONAHA.111.185116
215. Xue B, Zhang Z, Roncari FC, Guo F, Johnson KA. Aldosterone acting through the central nervous system sensitizes angiotensin II-induced hypertension. Hypertension. (2012) 60:. 1023–1030. doi: 10.1161/HYPERTENSIONAHA.112.196576
216. Xue B, Zhang Y, Johnson AK KA. interactions of the brain renin-angiotensin-system (RAS) and inflammation in the sensitization of hypertension. Front Neurosci. (2020) 14:650. doi: 10.3389/fnins.2020.00650
217. Xue B, Xue J, Yu Y, Wei GS, Beltz GT, Felder BR, et al. Predator scent-induced sensitization of hypertension and anxiety-like behaviors. Cell Mol Neurobiol. (2022) 42:1141–52. doi: 10.1007/s10571-020-01005-y
218. Ziegenhorn AA, Schulte-Herbrüggen O, Danker-Hopfe H, Malbranc M, Hartung DH, Anders D, et al. Serum neurotrophins–a study on the time course and influencing factors in a large old age sample. Neurobiol Aging. (2007) 289:1436–45. doi: 10.1016/j.neurobiolaging.2006.06.011
219. Yang X, Liu S, Zhang Z. Differences among sexes in blood pressure: A combinatorial consequence of the differences between RAAS components, sex hormones, and time course. Curr Hypertens Rev. (2022) 18:11–6. doi: 10.2174/1573402117666210511011444
220. Black EE, Smith MP, McIsaac W, Ferguson VA. Brain-derived neurotrophic factor acts at neurons of the subfornical organ to influence cardiovascular function. Physiol Rep. (2018) 6:e13704. doi: 10.14814/phy2.13704
221. Kuroski de Bold LM. Estrogen, natriuretic peptides and the renin-angiotensin system. Cardiovasc Res. (1999) 41:524–31. doi: 10.1016/S0008-6363(98)00324-1
222. Gupte M, Thatcher ES, Boustany-Kari MC, Shoemaker R, Yiannikouris F, Zhang and M. Karounos X. Angiotensin converting enzyme 2 contributes to sex differences in the development of obesity hypertension in C57BL/6 mice. Arterioscler Thromb Vasc Biol. (2012) 32:1392–9. doi: 10.1161/ATVBAHA.112.248559
223. Meyer MR, Haas E, Barton M. Gender differences of cardiovascular disease: new perspectives for estrogen receptor signaling. Hypertension. (2006) 47:1019–26. doi: 10.1161/01.HYP.0000223064.62762.0b
224. Bai J, Qi QR, Li Y, Day R, Makhoul J, Magness RR. estrogen receptors and estrogen-induced uterine vasodilation in pregnancy. Int J Mol Sci. (2020) 21:4349. doi: 10.3390/ijms21124349
225. Xue B, Zhang Z, Beltz GT, Guo F, Hay M, Johnson KA. Genetic knockdown of estrogen receptor-alpha in the subfornical organ augments ANG II-induced hypertension in female mice. Am J Physiol Regul Integr Comp Physiol. (2015) 308:R507–516. doi: 10.1152/ajpregu.00406.2014
226. Taddei S. Blood pressure through aging and menopause. Climacteric. (2009) 12:36–40. doi: 10.1080/13697130903004758
227. Xue B, Zhang Z, Beltz GT, Guo F, Hay M, Johnson KA. Estrogen regulation of the brain renin-angiotensin system in protection against angiotensin II-induced sensitization of hypertension. Am J Physiol Heart Circ Physiol. (2014) 307:H191–198. doi: 10.1152/ajpheart.01012.2013
228. Xue B, Beltz GT, Guo F, Johnson KA. Sex differences in maternal gestational hypertension-induced sensitization of angiotensin II hypertension in rat offspring: the protective effect of estrogen. Am J Physiol Regul Integr Comp Physiol. (2018) 314:R274–81. doi: 10.1152/ajpregu.00216.2017
229. Maruyama ON, Estrela FH, Sales OE, Lucas FT, Porto SC, Bergamaschi TC, et al. Differential effects of estrogen receptors in the rostral ventrolateral medulla in Goldblatt hypertension. J Steroid Biochem Mol Biol. (2022) 224:106176. doi: 10.1016/j.jsbmb.2022.106176
230. Ashraf SM, Vongpatanasin W. Estrogen and hypertension. Curr Hypertens Rep. (2006) 8:368–76. doi: 10.1007/s11906-006-0080-1
231. Lemos RJ, Alves RC, de Souza BS, Marsiglia DJ, Silva SM, Pereira CA, et al. Peripheral vascular reactivity and serum BDNF responses to aerobic training are impaired by the BDNF Val66Met polymorphism. Physiol Genomics. (2016) 48:116–23. doi: 10.1152/physiolgenomics.00086.2015
232. Komukai K, Mochizuki S, Yoshimura M. Gender and the renin-angiotensin-aldosterone system. Fundam Clin Pharmacol. (2010) 24:687–98. doi: 10.1111/j.1472-8206.2010.00854.x
233. Prabhushankar R, Krueger C, Manrique C. Membrane estrogen receptors: their role in blood pressure regulation and cardiovascular disease. Curr Hypertens Rep. (2014) 16:408. doi: 10.1007/s11906-013-0408-6
234. Xue B, Yu Y, Zhang Z, Guo F, Beltz GT, Thunhorst LR, et al. Leptin mediates high-fat diet sensitization of angiotensin II-elicited hypertension by upregulating the brain renin-angiotensin system and inflammation. Hypertension. (2016) 67:970–6. doi: 10.1161/HYPERTENSIONAHA.115.06736
235. Rahmouni K, Fath MA, Seo S, Thedens DR, Berry CJ, Weiss R, et al. Leptin resistance contributes to obesity and hypertension in mouse models of Bardet-Biedl syndrome. J Clin Investig. (2008) 118:1458–67. doi: 10.1172/JCI32357
236. Tutor AW, Lavie CJ, Kachur S, Milani RV, Ventura H. Updates on obesity and the obesity paradox in cardiovascular diseases. Prog Cardiovasc Dis. (2023) 78:2–10. doi: 10.1016/j.pcad.2022.11.013
237. Zhang PY, Huo LY, Fang QZ, Wang FX, Li DJ, Wang PH, et al. Maternal high-fat diet acts on the brain to induce baroreflex dysfunction and sensitization of angiotensin II-induced hypertension in adult offspring. Am J Physiol Heart Circ Physiol. (2018) 314:H1061–9. doi: 10.1152/ajpheart.00698.2017
238. Curtis SK. Estrogen and the central control of body fluid balance. Physiol Behav. (2009) 97:180–92. doi: 10.1016/j.physbeh.2009.02.027
239. Saito J, Ozaki Y, Ohnishi H, Nakamura T, Ueta Y. Osmotic stimuli increase brain-derived neurotrophic factor mRNA level in the rat subfornical organ. Neurosci Lett. (2003) 347:65–8. doi: 10.1016/S0304-3940(03)00614-1
240. Pluchino N, Cubeddu A, Begliuomini S, Merlini S, Giannini A, Bucci F, et al. Daily variation of brain-derived neurotrophic factor and cortisol in women with normal menstrual cycles, undergoing oral contraception and in postmenopause. Hum Reprod. (2009) 24:2303–9. doi: 10.1093/humrep/dep119
241. Takahashi A, Anzai Y, Tanji N, Imaizumi H, Fujita M, Hayashi M, et al. Association of equol with obesity in postmenopausal women. Menopause. (2021) 28:807–10. doi: 10.1097/GME.0000000000001761
242. Hirschberg LA. Sex hormones, appetite and eating behaviour in women. Maturitas. (2012) 71:248–56. doi: 10.1016/j.maturitas.2011.12.016
243. Gower AB, Nagy RT, Goran IM, Smith and E. Kent A. Leptin in postmenopausal women: influence of hormone therapy, insulin, and fat distribution. J Clin Endocrinol Metab. (2000) 85:1770–5. doi: 10.1210/jcem.85.5.6602
244. Cassis AL. Fat cell metabolism: insulin, fatty acids, and renin. Curr Hypertens Rep. (2000) 2:132–8. doi: 10.1007/s11906-000-0072-5
245. Thatcher S, Yiannikouris F, Gupte and L. Cassis M. The adipose renin-angiotensin system: role in cardiovascular disease. Mol Cell Endocrinol. (2009) 302:111–7. doi: 10.1016/j.mce.2009.01.019
246. Picó C, Palou M, Pomar AC, Rodríguez and A. Palou MA. Leptin as a key regulator of the adipose organ. Rev Endocr Metab Disord. (2022) 23:. 13–30. doi: 10.1007/s11154-021-09687-5
247. Hogarth JA, Graham NL, Corrigan HJ, Deuchars J, Mary AD, Greenwood PJ. Sympathetic nerve hyperactivity and its effect in postmenopausal women. J Hypertens. (2011) 29:2167–75. doi: 10.1097/HJH.0b013e32834b8014
248. Yanes LL, Romero GD, Iliescu R, Zhang H, Davis D, Reckelhoff FJ. Postmenopausal hypertension: role of the Renin-Angiotensin system. Hypertension. (2010) 56:359–63. doi: 10.1161/HYPERTENSIONAHA.110.152975
249. Yiannikouris F, Gupte M, Putnam and L. Cassis K. Adipokines and blood pressure control. Curr Opin Nephrol Hypertens. (2010) 19:195–200. doi: 10.1097/MNH.0b013e3283366cd0
250. Sabbatini AR, de Faria AP, Modolo and H. Moreno R. Adipokines: another link between obesity and hypertension. J Hum Hypertens. (2015) 29:210. doi: 10.1038/jhh.2014.28
251. Stachenfeld NS. Sex hormone effects on body fluid regulation. Exerc Sport Sci Rev. (2008) 3636:152–9. doi: 10.1097/JES.0b013e31817be928
252. Thaung Zaw JJ, Howe CP, Wong XR. Postmenopausal health interventions: Time to move on from the Women’s Health Initiative? Ageing Res Rev. (2018) 48:79–86. doi: 10.1016/j.arr.2018.10.005
Keywords: BDNF, estrogens, hypertension, obesity, interplay
Citation: Zhang Z, He Z, Pan J, Yuan M, Lang Y, Wei X and Zhang C (2024) The interaction of BDNF with estrogen in the development of hypertension and obesity, particularly during menopause. Front. Endocrinol. 15:1384159. doi: 10.3389/fendo.2024.1384159
Received: 08 February 2024; Accepted: 05 November 2024;
Published: 25 November 2024.
Edited by:
Hubert Vaudry, Université de Rouen, FranceReviewed by:
Carolina Dalmasso, University of Kentucky, United StatesAkira Takamata, Nara Women's University, Japan
Copyright © 2024 Zhang, He, Pan, Yuan, Lang, Wei and Zhang. This is an open-access article distributed under the terms of the Creative Commons Attribution License (CC BY). The use, distribution or reproduction in other forums is permitted, provided the original author(s) and the copyright owner(s) are credited and that the original publication in this journal is cited, in accordance with accepted academic practice. No use, distribution or reproduction is permitted which does not comply with these terms.
*Correspondence: Zhongming Zhang, emhvbmdtaW5nemhhbmdAaG90bWFpbC5jb20=; Chaoyun Zhang, Y2hhb3l1bjIwMTZAMTYzLmNvbQ==