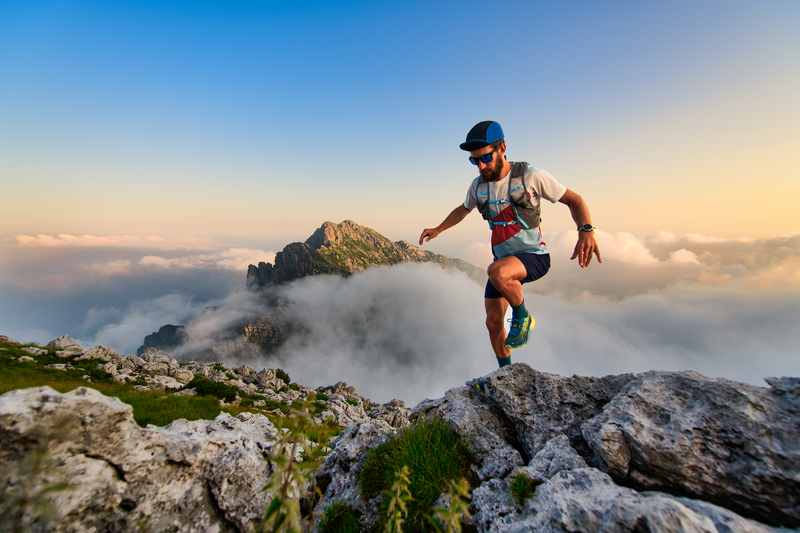
94% of researchers rate our articles as excellent or good
Learn more about the work of our research integrity team to safeguard the quality of each article we publish.
Find out more
ORIGINAL RESEARCH article
Front. Endocrinol. , 15 April 2024
Sec. Clinical Diabetes
Volume 15 - 2024 | https://doi.org/10.3389/fendo.2024.1352829
This article is part of the Research Topic Continuous Glucose Monitoring: Beyond Diabetes Management View all 9 articles
Background: Hypoglycemia is common in individuals with type 1 diabetes, especially during exercise. We investigated the accuracy of two different continuous glucose monitoring systems during exercise-related hypoglycemia in an experimental setting.
Materials and methods: Fifteen individuals with type 1 diabetes participated in two separate euglycemic-hypoglycemic clamp days (Clamp-exercise and Clamp-rest) including five phases: 1) baseline euglycemia, 2) plasma glucose (PG) decline ± exercise, 3) 15-minute hypoglycemia ± exercise, 4) 45-minute hypoglycemia, and 5) recovery euglycemia. Interstitial PG levels were measured every five minutes, using Dexcom G6 (DG6) and FreeStyle Libre 1 (FSL1). Yellow Springs Instruments 2900 was used as PG reference method, enabling mean absolute relative difference (MARD) assessment for each phase and Clarke error grid analysis for each day.
Results: Exercise had a negative effect on FSL1 accuracy in phase 2 and 3 compared to rest (ΔMARD = +5.3 percentage points [(95% CI): 1.6, 9.1] and +13.5 percentage points [6.4, 20.5], respectively). In contrast, exercise had a positive effect on DG6 accuracy during phase 2 and 4 compared to rest (ΔMARD = -6.2 percentage points [-11.2, -1.2] and -8.4 percentage points [-12.4, -4.3], respectively). Clarke error grid analysis showed a decrease in clinically acceptable treatment decisions during Clamp-exercise for FSL1 while a contrary increase was observed for DG6.
Conclusion: Physical exercise had clinically relevant impact on the accuracy of the investigated continuous glucose monitoring systems and their ability to accurately detect hypoglycemia.
Exogenous insulin replacement to obtain glycemic control is a hallmark for type 1 diabetes (T1D) treatment (1). The American Diabetes Association (ADA) recommends physical activity for individuals with type 1 diabetes as it improves glycemic control, decreases insulin requirements, and reduces cardiovascular complications in individuals with type 1 diabetes (2–5). However, exercise in these individuals is associated with hypoglycemia, which may be a barrier for obtaining glycemic control, and as a result many individuals with type 1 diabetes avoid engaging in regular physical activity (6, 7). Individuals with type 1 diabetes may need to consume considerable amounts of carbohydrates prior to physical activity in order to avoid exercise-induced hypoglycemia, which may reduce the potential benefits of vascular health and glycemic control that physical activity brings (8, 9). Continuous glucose monitoring (CGM) systems offer a way to frequently monitor glycemic changes throughout the day and particularly during exercise (10, 11). This provides individuals with type 1 diabetes a level of detail that cannot be achieved using capillary glucose meters, thus assisting in reduction of unnecessary glycemic fluctuations and episodes of hypoglycemia (12, 13).
Studies have indicated reduced accuracy of several CGM sensors during rapid glucose changes and low blood glucose levels, both commonly observed in individuals with type 1 diabetes and especially during exercise (14). CGM performance is clinically important since low sensor precision may lead to undetected events of hypoglycemia or unnecessary meal intake ensuing hyperglycemia. Here, we investigated the performance of two commonly used CGM systems, Dexcom G6 (DG6) and FreeStyle Libre 1 (FSL1), during plasma glucose (PG) decline and hypoglycemia, induced with or without exercise in individuals with type 1 diabetes.
CGM data presented in this study was obtained from a clinical trial investigating cardiovascular effects of exercise-related hypoglycemia in individuals with type 1 diabetes (registration with ClinicalTrials.gov, NCT04650646) (15). The trial was performed at Steno Diabetes Center Copenhagen and Center for Clinical Metabolic Research, Gentofte Hospital, University of Copenhagen, Hellerup, Denmark from September 2020 to June 2021. The study was conducted following the Helsinki Declaration and was approved by the Scientific Ethical Committee of the Capital Region of Denmark (ID No. H-20023688) and the Danish Data Protection Agency (ID No. P-2020-434). Written consent was obtained from all participants before being included in the study.
Fifteen men diagnosed with type 1 diabetes participated in a randomized crossover study including two separate euglycemic-hypoglycemic clamp days. One clamp day included a bout of moderate-intensity cycling exercise performed during declining plasma glucose and hypoglycemia (Clamp-exercise). In the other clamp day, hypoglycemia was induced at rest (Clamp-rest). The participants were recruited from the outpatient clinic at Steno Diabetes Center Copenhagen, Herlev, Denmark. Data reported are a pre-planned secondary analysis from a previously published study. Hence, the sample size was calculated based of the primary aim of that study and has been reported elsewhere (15). The study design is illustrated in Figure 1. The two clamp days were separated by at least four weeks to rule out possible carry-over effects. Inclusion criteria were age ≥18 years, type 1 diabetes diagnosis according to World Health Organization (WHO) classification, C-peptide levels <200 pmol/L, insulin treatment for at least 1 year, and informed and written consent. Further details of inclusion and exclusion criteria have previously been reported (15).
Figure 1 Study design. A randomized crossover euglycemic-hypoglycemic clamp study including Clamp-rest and Clamp-exercise. During Clamp-rest, participants were at bed rest during all phases. Clamp-exercise had an exercise element during plasma glucose decline phase and 15-minute hypoglycemic phase marked with gray (time 45 to 105 minute). Each clamp day was divided into five phases. Two continuous glucose monitoring systems, Dexcom G6 and FreeStyle Libre 1, were active throughout the clamp days. Further details and illustrations on the study design has been reported before (15).
Participants were supplied with and instructed to insert two CGM systems in parallel, Dexcom G6® (Dexcom, Inc., San Diego, CA, USA) and FreeStyle Libre 1® (Abbott Laboratories, Ltd., Alameda, CA, USA). The systems were inserted two days before the clamp days to reduce sensor inaccuracies (16). The participants were admitted in the morning after an overnight 10 hour fast including medicine fasting. Participants receiving insulin pen treatment were instructed to continue their usual basal insulin treatment, regardless of dosing time. Likewise, participants using insulin pump treatment were instructed to solely continue with the basal rate infusion throughout the test days. Sensors were not user-calibrated but relied on the factory calibrations as instructed in the devices’ user guide (17, 18). A peripheral intravenous catheter was inserted in the antecubital fossa of each forearm. One arm was heated throughout the clamp to obtain arterialized blood while the contralateral arm was used for isotonic saline (0.9% NaCl, Fresenius Kabi, Bad Homburg, Germany), insulin (Actrapid®; Novo Nordisk, Bagsværd, Denmark), and glucose (20% solution; Fresenius Kabi, Bad Homburg, Germany) infusion. The isotonic saline solution was administered at a constant infusion rate throughout the clamp to avoid volume depletion due to blood draw and to keep the intravenous cannula working properly. The hyperinsulinemic-euglycemic clamp was initiated at time 0 minutes when the target PG between 5.0 and 8.0 mmol/L was reached. A combination of a fixed insulin infusion rate at 80 mU/m2/min and a variable 200 mg/ml (20%) glucose infusion was initiated to clamp PG. Both clamp days (Clamp-exercise and Clamp-rest) contained the following phases: 1) a baseline euglycemic phase, 2) a PG decline phase induced at bed rest or during exercise, 3) a 15-minute hypoglycemic phase at bed rest or during exercise, continued by 4) a 45-minute hypoglycemic phase at bed rest and finally 5) recovery euglycemia. Exercise was performed at a moderate intensity defined as 64% to 76% of maximum heart rate calculated using the formula [207- (Age) × (0.7)] (19). The participants exercised on a Monark Ergomedic 839E (Monark Exercise AB, Vansbro, Sweden) for a total period of 60 minutes. Target heart rate was reached by adjusting the resistance of the cycle ergometer throughout the exercise period. The participants were instructed to begin exercise at a low-level intensity and gradually increase the intensity until reached target level. The target level of PG during the phases of hypoglycemia was <3.0 mmol/L, representing level 2 hypoglycemia (20). Glucose concentrations were determined every five minutes throughout the clamp days using DG6 and FSL1 in parallel. FSL1 was manually scanned during the clamp days whereas DG6 automatically stored the glucose values. Yellow Springs Instrument (YSI) 2900 biochemistry analyzer (Xylem, Inc., Rye Brook, NY, USA) was used to carry out the clamps as a PG reference method by sampling arterialized venous blood in 0.2 mL NaF tubes centrifuged at 7,400 g for 30 seconds and then analyzed.
For each sensor reading, the absolute relative difference was computed as the absolute difference between the reading and reference PG value divided by reference PG multiplied by 100 (21). For descriptive statistics, the absolute relative differences were summarized as mean ± standard error and plotted against clamp-time in figures. To compare the accuracy of each sensor between Clamp-exercise and Clamp-rest, we applied a linear mixed model with clamp time and clamp day and the interaction between them as fixed effect and with a heterogeneous compound symmetry covariance pattern to account for repeated measurements on each study participant. Results were reported as difference in mean absolute relative difference (ΔMARD) with 95% confidence interval for each clamp phase. Finally, a Clarke error grid analysis was performed for each clamp day to quantify the clinical significance of sensor inaccuracies (22). Paired sensor readings and reference PG values are depicted on a plot with five zones corresponding to varying clinical consequences. P <0.05 was considered statistically significant. SAS Studio version 3.8 (SAS Institute, Inc., Cary, NC, USA) was used to perform the linear mixed model analysis. The Clarke error grid plot was made with ega-package version 2.0.0 in R Statistical software version 4.2.1.
All 15 participants (Table 1) completed both clamp days yielding 551 and 543 DG6 sensor-PG pairs for Clamp-rest and Clamp-exercise, respectively, as well as 491 and 512 FSL1 sensor-PG pairs for Clamp-rest and Clamp-exercise, respectively. All participants placed the sensors according to specified guidelines except one who placed FSL1 on the upper thigh. For both clamp days, mean PG was kept at 6-7 mmol/L during the baseline-euglycemic phase although slightly decreasing towards the decline phase (Figure 2). Target hypoglycemia was reached after 90-minutes and followed by steady-state hypoglycemia of <3.0 mmol/L. Overall, PG levels for both clamp days were comparable.
Figure 2 Glucose readings represented as mean ± SE. The clamp period totals 180 minutes and consists of 37 data points (data collected every five minutes). Clamp-rest (A) was at bed rest throughout the clamp period. Clamp-exercise (B) contained an exercise element on a cycle ergometer during plasma glucose decline phase and 15-minute hypoglycemic phase (time 45 to 105 minute). YSI, Yellow Springs Instrument.
When comparing the clamp days (Table 2), FSL1 had a comparable MARD during phase 1 indicating similar baseline accuracies (Figure 3). Compared to rest, exercise increased MARD during phase 2 which continued during phase 3. There was a comparable MARD during phase 4 post exercise and during phase 5 recovery. DG6 had a comparable MARD between clamp days during phase 1, although this was surprisingly high compared to the expected (Figure 3). Compared to rest, exercise decreased MARD during phase 2 and phase 3, although not significantly. During phase 4, MARD was lower post-exercise, while a comparable MARD was observed between clamp days during phase 5.
Figure 3 MARD% ± SE. * indicates significant difference between Dexcom G6 Clamp-exercise vs Dexcom G6 Clamp-rest. # indicates significant difference between FreeStyle Libre 1 Clamp-exercise vs FreeStyle Libre 1 Clamp-rest. Results at P < 0.05 was considered statistically significant and are shown with a single symbol. Results at P < 0.01 and P < 0.001 are shown with double and triple symbols, respectively. MARD%, mean absolute relative difference in percentages.
Assessing the clinical performance according to Clarke error grid analysis showed a difference between clamp days (Figure 4). FSL1 performance decreased during Clamp-exercise where data points in the combined zones A+B decreased compared to Clamp-rest and accordingly increased in the clinically unacceptable estimates zone D. DG6 performance increased during Clamp-exercise where data points in zones A+B increased indicating an increase in sensor estimates to more clinical acceptable accuracies. No data points were observed in zones C and E for all error grid analyses.
Figure 4 Clarke error grid analysis of Dexcom G6 and FreeStyle Libre 1. (A) Dexcom G6 for Clamp-rest. (B) Dexcom G6 for Clamp-exercise. (C) FreeStyle Libre 1 for Clamp-rest. (D) FreeStyle Libre 1 for Clamp-exercise. Each dot represents a glucose sensor reading paired with corresponding reference plasma glucose. Pairings in zone A and B are defined as clinically acceptable sensor estimates whereas pairings in zones C, D, and E are defined as clinically unacceptable and could lead to errors in diabetes treatment.
We report that exercise can affect the sensor accuracy of the investigated CGM systems by either a decrease in sensor accuracy and the ability to detect hypoglycemia as seen with DG6, or contrary by an increase in sensor accuracy as seen for FSL1 which may be of clinical relevance for physically active individuals with type 1 diabetes when choosing between CGM systems.
The overall MARD of DG6 obtained in this study under baseline euglycemia was substantially higher compared to previous studies (23–25). Shah et al. compared DG6 sensor readings with YSI glucose values in 62 participants and demonstrated a general overall MARD of 9% (23). Generally, the design in the studies were similar e.g., utilizing a YSI analyzer, obtaining arterialized venous blood, and relying on the factory calibration. Furthermore, we followed the manufacturer’s specified guidelines for inserting and initializing the sensor and doing so two days before the clamp days to avoid possible inaccuracy during the sensor warm-up time. Since the overall FSL1 MARD of our study was more comparable with previous studies (26–29), and since our study followed the same principles as previous studies, the deviating MARD values obtained from DG6 may not be explained by the design of our study. The deviation may rather be related to the applied sensors. As the higher-than-expected MARD of DG6 was observed at baseline for both test days, and that they were comparable to each other, the comparison outcomes between clamp days were considered valid.
Our results suggest that exercise has a negative effect on FSL1 performance without affecting the post-exercise sensor performance. Contrary, DG6 had an improvement in performance during exercise which persisted post-exercise. We hypothesize that the exercise-related performance increase of DG6 is caused by increased blood circulation and production of heat during exercise resulting in a subsequent increase in skin blood flow (30). This could potentially lead to a higher interstitial space fluid turnover rate and better equilibrium between plasma and interstitial space fluid, thus more accurate estimates of glucose (31). Conversely, a worsening in performance was seen for FSL1 during exercise. This may be explained by the placement of the sensor in an area of high movement and mechanical activity during exercise as opposed to the abdomen as seen for DG6 (32). Thus, the continual mechanical movement of the sensor may have outweighed the possible performance increase from increased skin blood flow.
Few studies have assessed the sensors’ performance during exercise (33–37), although they mostly relied on capillary blood glucose as a reference method. Guillot et al. showed no notable changes in DG6 sensor accuracy during exercise while wearing the sensors on the abdomen (33). Dyess et al. showed an overall decrease in DG6 sensor performance during exercise, however apart from the abdomen, the participant had the option to wear the sensor on the upper arms, buttocks, and thighs (34). When sub-analyzing for the abdomen only, Dyess et al. found an increase in sensor performance which is in accordance with our study and supports our hypothesis about the role of placement of sensors.
For FSL1, Moser et al. showed higher MARD during exercise in 19 individuals with type 1 diabetes compared to capillary blood glucose (35). Likewise, Fokkert et al. showed an increase in MARD for FSL1 during exercise in 23 individuals with type 1 diabetes compared to capillary blood glucose (36). However, Giani et al. showed no performance difference between rest and exercise in 17 young individuals with type 1 diabetes (37). As FSL1 is placed on the upper arm, an area of high activity during exercise, the decrease in sensor performance may be of this reason. We can only speculate if the performance of FSL1 would remain unchanged or increase if placed on the abdomen during exercise compared to rest. However, Charleer et al. showed a worsening in FSL1 performance when placed on the abdomen (38). Although sensor placement may have a notable role, factors such as varying populations included, different exercise forms and intensities employed, different extent of glycemic excursions and varying PG reference methods utilized may contribute to differing results.
According to Clarke error grid analysis, most of the data points in our study were in the clinically safe zones A+B for both sensors regardless of the clamp day, although the percentage of data points in the upper zone D was surprisingly high for DG6 during Clamp-rest. During Clamp-exercise, DG6 had a shift in data points from the clinically unacceptable zones (solely located in the upper D zone) to the clinically acceptable zones with an increase in zones A+B by almost 10 percentage points compared to Clamp-rest. This indicates that exercise potentially improves sensor accuracy and/or sensor lag time during rapid PG decline and hypoglycemia. In contrast, FSL1 showed a slight decrease of data points in the clinically acceptable zones during Clamp-exercise compared to Clamp-rest. FSL1 had almost a doubling of data points in zone D for Clamp-exercise indicating exercise having a clinically relevant negative effect. Thus, Clarke error grid analysis is consistent with the MARD results and could be explained in the same manner.
The previously mentioned studies also evaluated the clinical safety of DG6 and FSL1 using Clarke Error grid analysis. Guillot et al. showed good clinical reliability for DG6 during exercise with 99% of all values located in the clinically safe zones A+B (33). Dyess et al. found that individuals who wore DG6 on their buttocks during exercise had an increase of values in zone D while an increase of values in zones A+B was seen when wearing the sensor on the abdomen (34). For FSL1, Giani found 97% of sensor readings fell in zones A+B during rest and 98% during exercise indicating a marginal difference between the two settings (37). Moser et al. found 91% of sensor values were in zones A+B during rest while 78% for FSL1 during exercise indicating a decrease in the clinical accuracy of the sensor during exercise (35). Throughout all the studies where exercise negatively impacted the sensor performance, sensor values specifically increased in the upper zone D similarly to our study indicating an increase in failure to detect hypoglycemia.
The strengths of the present study include the cross-over design, the direct comparison of exercise versus rest and the conduction in a controlled clinical research facility. Sensors were initialized two days prior to the clamp day to prevent possible sensor inaccuracies. Unlike other studies that used capillary blood glucose, our study utilized PG measured by YSI 2900 as a glucose reference method which is often cited as the gold standard (39). Furthermore, the sensors were not user-calibrated but relied on the factory calibration mimicking a real-life setting with individuals doing the same.
A limitation to our study is the small number of participants and that the study only included male adults thus limiting the generalizability to females and other age groups. One participant had their FSL1 placed on the upper thigh which could potentially influence on the results, although Charleer et al. showed a minimal difference between the placement of FSL1 on the upper thigh and the upper arm (38). Another limitation is the rather high MARD observed for Dexcom G6 which could influence the results and that MARD does not take sensor errors into account (e.g., consistent higher glucose estimates). To overcome this, an adjunctive analysis called precision absolute relative deviation, which requires the insertion of an identical parallel sensor, could potentially have added value to our study (40). A limitation of Clarke Error grid analysis is the rather stringent limits between the zones which newer error grids seek to mitigate (41). Finally, the physiological effect of physical activity on glucose levels is rather complex and different at differing exercise types, intensities, and durations. Thus, the observed performance of the sensors cannot be generalized to other exercise intensities or durations.
In conclusion, the two commonly used sensors DG6 and FSL1 showed different responses to exercise in relation to PG decline and hypoglycemia in individuals with type 1 diabetes. Exercise negatively impacted FSL1 sensor performance during both declining PG and hypoglycemia, whereas DG6 had more accurate sensor readings during exercise and post-exercise. Individuals with type 1 diabetes and healthcare practitioners should be aware of the potentially negative or positive impacts of exercise on CGM sensor accuracy in detecting clinically relevant episodes of hypoglycemia.
Parts of the following study were presented as an oral presentation at the European Association of the Study of Diabetes (EASD) 58th annual meeting in Stockholm, Sweden, September 2022
The raw data supporting the conclusions of this article will be made available by the authors, without undue reservation.
The studies involving humans were approved by the Scientific Ethical Committee of the Capital Region of Denmark. The studies were conducted in accordance with the local legislation and institutional requirements. The participants provided their written informed consent to participate in this study.
KM: Data curation, Writing – review & editing, Writing – original draft, Visualization, Validation, Software, Project administration, Investigation, Formal analysis. PH: Writing – review & editing, Supervision, Resources, Methodology, Investigation, Funding acquisition, Data curation, Conceptualization. SE: Writing – review & editing, Validation, Resources, Methodology. JF: Formal analysis, Software, Supervision, Validation, Writing – review & editing. UP-b: Writing – review & editing, Methodology, Validation, Supervision, Conceptualization. FK: Conceptualization, Methodology, Resources, Supervision, Validation, Writing – review & editing. TV: Writing – review & editing, Validation, Supervision, Project administration, Funding acquisition, Conceptualization. AA: Conceptualization, Formal analysis, Methodology, Supervision, Validation, Writing – review & editing.
The author(s) declare that financial support was received for the research, authorship, and/or publication of this article. This work was supported by grants from the Grosserer L.F Foghts (grant number 21761) and The Independent Research Fund Denmark (grant number 1030-00256B).
The authors thank the participants for their contribution and commitment. We thank our supportive colleagues for their time and effort in conducting the study.
SE is currently employed by Novo Nordisk and holds stock in Novo Nordisk. UP-b has served on advisory boards for and/or received lecture fees from Novo Nordisk and Sanofi. FK has served on advisory panels at, been part of speaker’s bureaus for, served as a consultant to, and/or received research support from AstraZeneca, Bayer, Boehringer Ingelheim, Eli Lilly, Gubra, MSD/Merck, Novo Nordisk, Sanofi, ShouTi, Zealand Pharma and Zucara. TV has served on scientific advisory panels at, been part of speaker’s bureaus for, served as a consultant to, and/or received research support from Amgen, AstraZeneca, Boehringer Ingelheim, Eli Lilly, Gilead, GSK, Mundipharma, MSD/Merck, Novo Nordisk, Sanofi, and Sun Pharmaceuticals.
The remaining authors declare that the research was conducted in the absence of any commercial or financial relationships that could be construed as a potential conflict of interest.
All claims expressed in this article are solely those of the authors and do not necessarily represent those of their affiliated organizations, or those of the publisher, the editors and the reviewers. Any product that may be evaluated in this article, or claim that may be made by its manufacturer, is not guaranteed or endorsed by the publisher.
ADA, American Diabetes Association; CGM, Continuous glucose monitoring; MARD, Mean absolute relative difference; PG, Plasma glucose; T1D, Type 1 diabetes; YSI, Yellow Springs Instrument.
1. Chiang JL, Maahs DM, Garvey KC, Hood KK, Laffel LM, Weinzimer SA, et al. Type 1 diabetes in children and adolescents: A position statement by the American diabetes association. Diabetes Care. (2018) 41:2026–44. doi: 10.2337/dci18-0023
2. Colberg SR, Sigal RJ, Yardley JE, Riddell MC, Dunstan DW, Dempsey PC, et al. Physical activity/exercise and diabetes: A position statement of the American diabetes association. Diabetes Care. (2016) 39:2065–79. doi: 10.2337/dc16-1728
3. Riddell MC, Gallen IW, Smart CE, Taplin CE, Adolfsson P, Lumb AN, et al. Exercise management in type 1 diabetes: a consensus statement. Lancet Diabetes Endocrinol. (2017) 5:377–90. doi: 10.1016/S2213-8587(17)30014-1
4. ElSayed NA, Aleppo G, Aroda VR, Bannuru RR, Brown FM, Bruemmer D, et al. 5. Facilitating positive health behaviors and well-being to improve health outcomes: Standards of care in diabetes—2023. Diabetes Care. (2023) 46:S68–96. doi: 10.2337/dc23-S005
5. Chimen M, Kennedy A, Nirantharakumar K, Pang TT, Andrews R, Narendran P. What are the health benefits of physical activity in type 1 diabetes mellitus? A literature review. Diabetologia. (2012) 55:542–51. doi: 10.1007/s00125-011-2403-2
6. Brazeau AS, Rabasa-Lhoret R, Strychar I, Mircescu H. Barriers to physical activity among patients with type 1 diabetes. Diabetes Care. (2008) 31:2108–9. doi: 10.2337/dc08-0720
7. Finn M, Sherlock M, Feehan S, Guinan EM, Moore KB. Adherence to physical activity recommendations and barriers to physical activity participation among adults with type 1 diabetes. Ir J Med Sci. (2022) 191(4):1639–46. doi: 10.1007/s11845-021-02741-w
8. Dubé MC, Prud’homme D, Lemieux S, Lavoie C, Weisnagel SJ. Relation between energy intake and glycemic control in physically active young adults with type 1 diabetes. J Sci Med Sport. (2014) 17:47–50. doi: 10.1016/j.jsams.2013.01.009
9. Yardley J, Mollard R, MacIntosh A, MacMillan F, Wicklow B, Berard L, et al. Vigorous intensity exercise for glycemic control in patients with type 1 diabetes. Can J Diabetes. (2013) 37:427–32. doi: 10.1016/j.jcjd.2013.08.269
10. Lin R, Brown F, James S, Jones J, Ekinci E. Continuous glucose monitoring: A review of the evidence in type 1 and 2 diabetes mellitus. Diabetes Med J Br Diabetes Assoc. (2021) 38:e14528. doi: 10.1111/dme.14528
11. Moser O, Riddell MC, Eckstein ML, Adolfsson P, Rabasa-Lhoret R, van den Boom L, et al. Glucose management for exercise using continuous glucose monitoring (CGM) and intermittently scanned CGM (isCGM) systems in type 1 diabetes: position statement of the European Association for the Study of Diabetes (EASD) and of the International Society for Pediatric and Adolescent Diabetes (ISPAD) endorsed by JDRF and supported by the American Diabetes Association (ADA). Diabetologia. (2020) 63:2501–20. doi: 10.1007/s00125-020-05263-9
12. Riddell MC, Milliken J. Preventing exercise-induced hypoglycemia in type 1 diabetes using real-time continuous glucose monitoring and a new carbohydrate intake algorithm: An observational field study. Diabetes Technol Ther. (2011) 13:819–25. doi: 10.1089/dia.2011.0052
13. Burckhardt MA, Chetty T, Smith GJ, Adolfsson P, de Bock M, Jones TW, et al. Use of continuous glucose monitoring trends to facilitate exercise in children with type 1 diabetes. Diabetes Technol Ther. (2019) 21:51–5. doi: 10.1089/dia.2018.0292
14. Stone JY, Bailey TS. Benefits and limitations of continuous glucose monitoring in type 1 diabetes. Expert Rev Endocrinol Metab. (2020) 15:41–9. doi: 10.1080/17446651.2020.1706482
15. Hagelqvist PG, Andersen A, Maytham KB, Andreasen CR, Engberg S, Lindhardt TB, et al. Exercise-related hypoglycaemia induces QTC -interval prolongation in individuals with type 1 diabetes. Diabetes Obes Metab. (2023) 25(5):1186–95. doi: 10.1111/dom.14964
16. Tsoukas M, Rutkowski J, El-Fathi A, Yale JF, Bernier-Twardy S, Bossy A, et al. Accuracy of freeStyle libre in adults with type 1 diabetes: the effect of sensor age. Diabetes Technol Ther. (2020) 22:203–7. doi: 10.1089/dia.2019.0262
17. Dexcom, Inc. Dexcom G6 Continuous Glucose Monitoring Systems User Guide. San Diego, CA: Dexcom, Inc. Available online at: https://s3-us-west-2.amazonaws.com/dexcompdf/G6-CGM-Users-Guide.pdfhttps://dexcompdf.s3.us-west-2.amazonaws.com/en-us/G6-CGM-Users-Guide-new.pdf (Accessed August 27, 2023).
18. Abbott Laboratories, Ltd. FreeStyle Libre Flash Glucose Monitoring System User’s Manual. Alameda, CA: Abbott Laboratories, Ltd. Available online at: https://freestyleserver.com/Payloads/IFU/2023/q1/ART40989-201_rev-A-WEB.pdfhttps://freestyleserver.com/Payloads/IFU/2023/q1/ART40989-201_rev-A-WEB.pdf (Accessed August 27, 2023).
19. Canning KL, Brown RE, Jamnik VK, Salmon A, Ardern CI, Kuk JL. Individuals underestimate moderate and vigorous intensity physical activity. PLoS One. (2014) 9:e97927. doi: 10.1371/journal.pone.0097927
20. International Hypoglycaemia Study Group. Glucose Concentrations of Less Than 3.0 mmol/L (54 mg/dL) Should Be Reported in Clinical Trials: A Joint Position Statement of the American Diabetes Association and the European Association for the Study of Diabetes. Diabetes Care. (2017) 40:155–7. doi: 10.2337/dc16-2215
21. Heinemann L, Schoemaker M, Schmelzeisen-Redecker G, Hinzmann R, Kassab A, Freckmann G, et al. Benefits and limitations of MARD as a performance parameter for continuous glucose monitoring in the interstitial space. J Diabetes Sci Technol. (2020) 14:135–50. doi: 10.1177/1932296819855670
22. Clarke WL, Cox D, Gonder-Frederick LA, Carter W, Pohl SL. Evaluating clinical accuracy of systems for self-monitoring of blood glucose. Diabetes Care. (1987) 10:622–8. doi: 10.2337/diacare.10.5.622
23. Shah VN, Laffel LM, Wadwa RP, Garg SK. Performance of a factory-calibrated real-time continuous glucose monitoring system utilizing an automated sensor applicator. Diabetes Technol Ther. (2018) 20:428–33. doi: 10.1089/dia.2018.0143
24. Castorino K, Polsky S, O’Malley G, Levister C, Nelson K, Farfan C, et al. Performance of the dexcom G6 continuous glucose monitoring system in pregnant women with diabetes. Diabetes Technol Ther. (2020) 22:943–7. doi: 10.1089/dia.2020.0085
25. Davis GM, Spanakis EK, Migdal AL, Singh LG, Albury B, Urrutia MA, et al. Accuracy of dexcom G6 continuous glucose monitoring in non–critically ill hospitalized patients with diabetes. Diabetes Care. (2021) 44:1641–6. doi: 10.2337/dc20-2856
26. Bailey T, Bode BW, Christiansen MP, Klaff LJ, Alva S. The performance and usability of a factory-calibrated flash glucose monitoring system. Diabetes Technol Ther. (2015) 17:787–94. doi: 10.1089/dia.2014.0378
27. Welsh JB, Zhang X, Puhr SA, Johnson TK, Walker TC, Balo AK, et al. Performance of a factory-calibrated, real-time continuous glucose monitoring system in pediatric participants with type 1 diabetes. J Diabetes Sci Technol. (2019) 13:254–8. doi: 10.1177/1932296818798816
28. Ólafsdóttir AF, Attvall S, Sandgren U, Dahlqvist S, Pivodic A, Skrtic S, et al. A clinical trial of the accuracy and treatment experience of the flash glucose monitor freeStyle libre in adults with type 1 diabetes. Diabetes Technol Ther. (2017) 19:164–72. doi: 10.1089/dia.2016.0392
29. Nagl K, Berger G, Aberer F, Ziko H, Weimann K, Bozic I, et al. Performance of three different continuous glucose monitoring systems in children with type 1 diabetes during a diabetes summer camp. Pediatr Diabetes. (2021) 22:271–8. doi: 10.1111/pedi.13160
30. González-Alonso J. Human thermoregulation and the cardiovascular system: Tissue blood flow and temperature regulation. Exp Physiol. (2012) 97:340–6. doi: 10.1113/expphysiol.2011.058701
31. Moser O, Yardley J, Bracken R. Interstitial glucose and physical exercise in type 1 diabetes: Integrative physiology, technology, and the gap in-between. Nutrients. (2018) 10:93. doi: 10.3390/nu10010093
32. Coates AM, Cohen JN, Burr JF. Investigating sensor location on the effectiveness of continuous glucose monitoring during exercise in a non-diabetic population. Eur J Sport Sci. (2023) 23:2109–17. doi: 10.1080/17461391.2023.2174452
33. Guillot FH, Jacobs PG, Wilson LM, Youssef JE, Gabo VB, Branigan DL, et al. Accuracy of the dexcom G6 glucose sensor during aerobic, resistance, and interval exercise in adults with type 1 diabetes. Biosensors. (2020) 10:138. doi: 10.3390/bios10100138
34. Dyess RJ, McKay T, Feygin Y, Wintergerst K, Thrasher BJ. Factory-calibrated continuous glucose monitoring system accuracy during exercise in adolescents with type 1 diabetes mellitus. J Diabetes Sci Technol. (2022) 0(0). doi: 10.1177/19322968221120433
35. Moser O, Eckstein ML, McCarthy O, Deere R, Pitt J, Williams DM, et al. Performance of the Freestyle Libre flash glucose monitoring (flash GM) system in individuals with type 1 diabetes: A secondary outcome analysis of a randomized crossover trial. Diabetes Obes Metab. (2019) 21:2505–12. doi: 10.1111/dom.13835
36. Fokkert M, van Dijk PR, Edens MA, Díez Hernández A, Slingerland R, Gans R, et al. Performance of the Eversense versus the Free Style Libre Flash glucose monitor during exercise and normal daily activities in subjects with type 1 diabetes mellitus. BMJ Open Diabetes Res Care. (2020) 8:e001193. doi: 10.1136/bmjdrc-2020-001193
37. Giani E, Macedoni M, Barilli A, Petitti A, Mameli C, Bosetti A, et al. Performance of the Flash Glucose Monitoring System during exercise in youth with Type 1 diabetes. Diabetes Res Clin Pract. (2018) 146:321–9. doi: 10.1016/j.diabres.2018.10.001
38. Charleer S, Mathieu C, Nobels F, Gillard P. Accuracy and precision of flash glucose monitoring sensors inserted into the abdomen and upper thigh compared with the upper arm. Diabetes Obes Metab. (2018) 20:1503–7. doi: 10.1111/dom.13239
39. Han J, Nichols JH, Rice M, Klonoff DC. The end of the road for the YSI 2300 analyzer: where do we go now? J Diabetes Sci Technol. (2020) 14:595–600. doi: 10.1177/1932296819886603
40. Obermaier K, Schmelzeisen-Redeker G, Schoemaker M, Klötzer HM, Kirchsteiger H, Eikmeier H, et al. Performance evaluations of continuous glucose monitoring systems: precision absolute relative deviation is part of the assessment. J Diabetes Sci Technol. (2013) 7:824–32. doi: 10.1177/193229681300700404
Keywords: accuracy, continuous glucose monitoring, exercise, hypoglycemia, mean absolute relative difference, type 1 diabetes
Citation: Maytham K, Hagelqvist PG, Engberg S, Forman JL, Pedersen-Bjergaard U, Knop FK, Vilsbøll T and Andersen A (2024) Accuracy of continuous glucose monitoring during exercise-related hypoglycemia in individuals with type 1 diabetes. Front. Endocrinol. 15:1352829. doi: 10.3389/fendo.2024.1352829
Received: 09 December 2023; Accepted: 29 March 2024;
Published: 15 April 2024.
Edited by:
Jianzhong Xiao, Tsinghua University, ChinaCopyright © 2024 Maytham, Hagelqvist, Engberg, Forman, Pedersen-Bjergaard, Knop, Vilsbøll and Andersen. This is an open-access article distributed under the terms of the Creative Commons Attribution License (CC BY). The use, distribution or reproduction in other forums is permitted, provided the original author(s) and the copyright owner(s) are credited and that the original publication in this journal is cited, in accordance with accepted academic practice. No use, distribution or reproduction is permitted which does not comply with these terms.
*Correspondence: Andreas Andersen, YW5kcmVhcy5hbmRlcnNlbi4wNEByZWdpb25oLmRr; Per G. Hagelqvist, cGVyLmd1c3Rhdi5oYWdlbHF2aXN0LjAxQHJlZ2lvbmguZGs=
†These authors share first authorship
‡ORCID: Per G. Hagelqvist, orcid.org/0000-0002-9017-9010
Andreas Andersen, orcid.org/0000-0001-8190-5140
Disclaimer: All claims expressed in this article are solely those of the authors and do not necessarily represent those of their affiliated organizations, or those of the publisher, the editors and the reviewers. Any product that may be evaluated in this article or claim that may be made by its manufacturer is not guaranteed or endorsed by the publisher.
Research integrity at Frontiers
Learn more about the work of our research integrity team to safeguard the quality of each article we publish.