- 1Department of Endocrinology, Diabetes and Metabolism, University Hospital Essen, University of Duisburg-Essen, Essen, Germany
- 2Cardiovascular Pharmacology, Leibniz-Institut für Analytische Wissenschaften-ISAS-e.V., Dortmund, Germany
- 3Institute of Endocrinology and Diabetes and Center for Brain, Behavior and Metabolism, University Hospital Schleswig-Holstein (UKSH), University of Lübeck, Lübeck, Germany
- 4Institute of Human Genetics, University Hospital Essen, University of Duisburg-Essen, Essen, Germany
- 5Institute of Pharmacology and Toxicology, University of Würzburg, Würzburg, Germany
Introduction: Thyroid hormones (THs) are known to have various effects on the cardiovascular system. However, the impact of TH levels on preexisting cardiac diseases is still unclear. Pressure overload due to arterial hypertension or aortic stenosis and aging are major risk factors for the development of structural and functional abnormalities and subsequent heart failure. Here, we assessed the sensitivity to altered TH levels in aged mice with maladaptive cardiac hypertrophy and cardiac dysfunction induced by transverse aortic constriction (TAC).
Methods: Mice at the age of 12 months underwent TAC and received T4 or anti-thyroid medication in drinking water over the course of 4 weeks after induction of left ventricular pressure overload.
Results: T4 excess or deprivation in older mice had no or only very little impact on cardiac function (fractional shortening), cardiac remodeling (cardiac wall thickness, heart weight, cardiomyocyte size, apoptosis, and interstitial fibrosis), and mortality. This is surprising because T4 excess or deprivation had significantly changed the outcome after TAC in young 8-week-old mice. Comparing the gene expression of deiodinases (Dio) 2 and 3 and TH receptor alpha (TRα) 1 and the dominant-negative acting isoform TRα2 between young and aged mice revealed that aged mice exhibited a higher expression of TRα2 and Dio3, while expression of Dio2 was reduced compared with young mice. These changes in Dio2 and 3 expressions might lead to reduced TH availability in the hearts of 12-month-old mice accompanied by reduced TRα action due to higher TRα2.
Discussion: In summary, our study shows that low and high TH availability have little impact on cardiac function and remodeling in older mice with preexisting pressure-induced cardiac damage. This observation seems to be the result of an altered expression of deiodinases and TRα isoforms, thus suggesting that even though cardiovascular risk is increasing with age, the response to TH stress may be dampened in certain conditions.
1 Introduction
Cardiovascular diseases are still the leading cause of death (1). Several clinical and preclinical studies suggest a close association of thyroid dysfunction with cardiovascular diseases (2–7). In this context, systemic hyper- and hypothyroidism have been revealed as risk factors for heart failure (8). Aging acts as an important further modifier, as the prevalence of both thyroid dysfunction and cardiac diseases increases with age (9–11). However, the relevance and interplay of altered thyroid hormone (TH) availability, age, and their impact on the progression or recovery of cardiac diseases are still not fully understood.
THs increase the heart rate and cardiac inotropy and decrease the arterial vascular smooth muscle tone leading to reduced cardiac afterload (3, 12–14). These TH effects involve the regulation of sarcomeric proteins, e.g., myosin heavy chain alpha and beta, proteins involved in the regulation of calcium homeostasis, e.g., the sarcoplasmic/endoplasmic reticulum Ca(2+)ATPase 2a2 and the ryanodine receptor 2 (15–17). Administered for a certain duration, THs have shown to reduce cardiac afterload, improve cardiac output, and lead to a so-called physiological, compensatory cardiac hypertrophy (3, 18–24).
In our previous study in 8-week-old mice, we found a low TH state to be beneficial under conditions of left ventricular pressure overload induced by transverse aortic constriction (TAC). Reduced cardiac hypertrophy and improved cardiac function were evident after 4 weeks of TH deprivation. In contrast, TH excess was not beneficial in this situation as it did not prevent cardiac hypertrophy, increased apoptosis, and had a negative impact on cardiac function (25). Under these conditions, TH excess led to a so-called pathological cardiac hypertrophy accompanied by increased interstitial fibrosis and cardiomyocyte apoptosis (26, 27).
Here, we aimed to investigate whether or to what extent older mice at the age of 12 months develop changes in heart function and morphology after TAC in response to TH deprivation or high-dose TH availability. In line with Kerp et al. (25), modulation of thyroid status was started after 1 week of TAC when cardiac hypertrophy and the first signs of cardiac depression have developed. In contrast to our previous study in young mice, low and high TH availability showed little impact on cardiac hypertrophy, apoptosis, fibrosis, or cardiac function. Our results suggest that these age-related differences are associated with altered gene expression levels of TRα isoforms and deiodinases 2 and 3 in 12-month-old mice compared with 8-week-old mice. Therefore, we suggest that even though cardiovascular risk is increasing with age, the response to TH stress may be dampened.
2 Materials and methods
2.1 Animals
Male wild-type C57BL/6JRj mice (Janvier Labs, France) were studied at the age of 12 months as previously described (25). Briefly, all mice were single-housed under standard conditions (room temperature 23°C ± 1°C; humidity 55% ± 10%; 12:12 h light–dark cycle). Standard chow diet and water were provided ad libitum. All animal experiments were performed in accordance with the German Regulations for Laboratory Animal Science (GV-SOLAS) and the European Health Law of the Federation of Laboratory Animal Science Associations (FELASA). The experimental protocols were approved by the Landesamt für Natur, Umwelt und Verbraucherschutz Nordrhein-Westfalen, Germany (LANUV-NRW, AZ 84-02.04.2016.A261).
2.2 Transverse aortic constriction
Mice were subjected to TAC using a 25-gauge needle to induce chronic left ventricular pressure overload as previously described (28, 29). One day prior to TAC and 1, 3, and 5 weeks after TAC, echocardiography was performed (Figure 1A). Mice with an aortic pressure gradient below 60 mmHg at 1 week after TAC were excluded from the study.
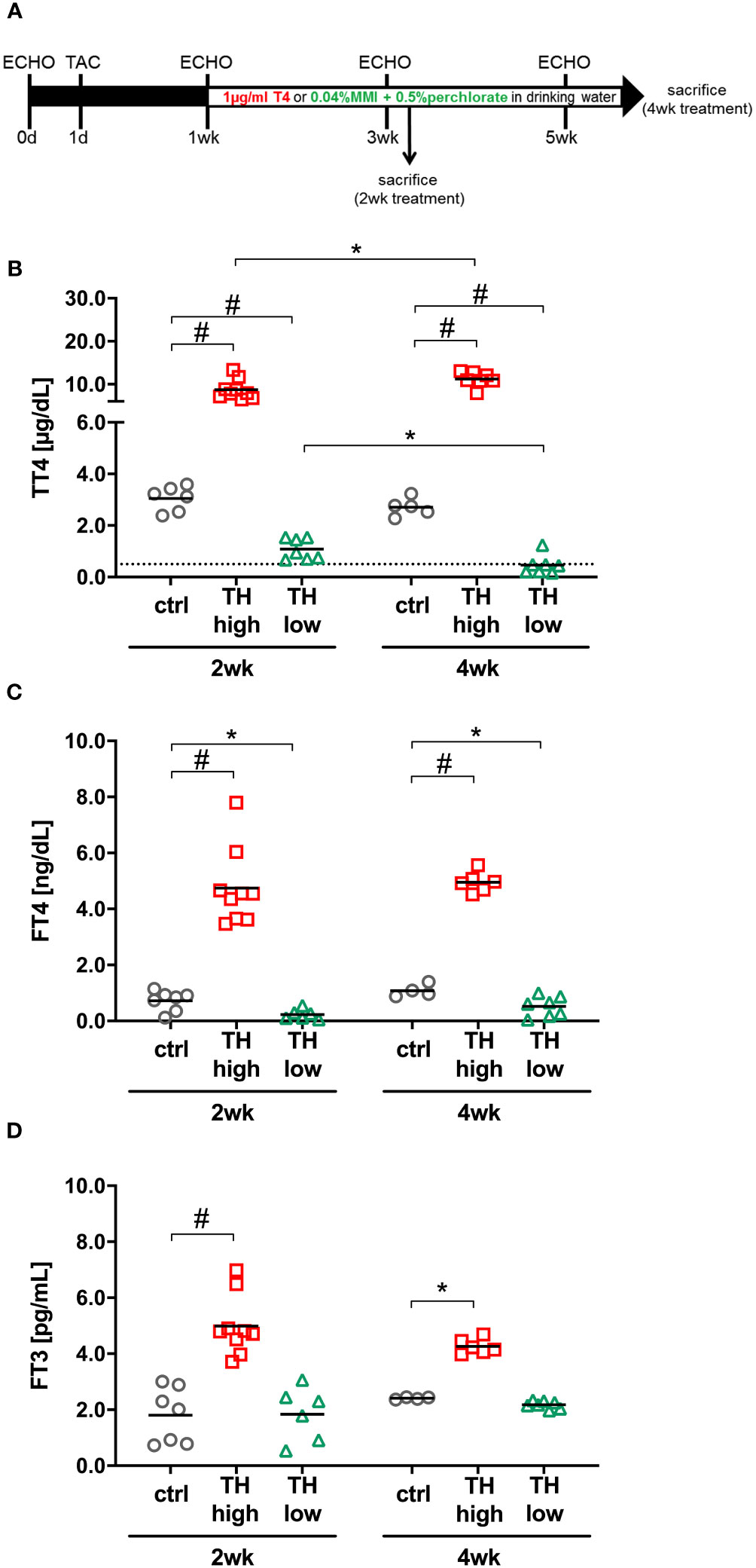
Figure 1 Study design and serum thyroid hormone (TH) status. Twelve-month-old male C57Bl/6 mice were subjected to transaortic constriction (TAC) at experimental day 1. Echocardiographic measurements (ECHO) were conducted 1 day prior to TAC (day 0) and after 1, 3, and 5 weeks. One week after TAC, oral T4 treatment (TH high) and TH deprivation [MMI/ClO4− in combination with low iodine diet (TH low)] were started. Controls (ctrl) received drinking water without supplements. Mice were sacrificed 2 or 4 weeks after induction of TH dysfunction (A). TT4 (B), FT4 (C), and FT3 (D) serum concentrations confirmed TH excess and TH deprivation at both time points in the two treatment groups compared with controls (C). The dotted line represents the detection limit of the assay and the values below were calculated from the standard curve. *p < 0.05, #p < 0.0001 by two-way ANOVA and Tukey’s post-hoc analysis; ctrl, control; wk, weeks.
2.3 Echocardiography
Transthoracic echocardiograms were performed in a blinded fashion using the Vevo 3100 high-resolution imaging system (FUJIFILM VisualSonics, Amsterdam, The Netherlands) and the MX550D transducer with pentobarbital (20–35 mg/kg body weight, i.p.) as an anesthetic. Two-dimensional M-mode images in the short axis view at the proximal level of the papillary muscles were used to evaluate end-diastolic and end-systolic intraventricular septal (IVSd/IVSs), left ventricular posterior wall thickness (LVPWd/LVPWs), and end-diastolic and end-systolic left ventricular internal diameters (LVIDd/LVIDs). Pulsed-wave Doppler measurements were performed to evaluate the peak blood flow velocities at the site of constriction (Vmax (mm/s)). Fractional shortening (FS) and aortic pressure gradients (mmHg) were calculated using the VisualSonics Cardiac Measurements software. The data represent the average of at least six cardiac cycles. The investigators were blinded regarding treatment groups during measurements and data analysis. Measurements at heart rates below 450 bpm were excluded.
2.4 Treatment
Mice were subjected to two different treatment protocols: 1) to increase TH availability (TH high), mice received thyroxine (T4) in drinking water [1 μg/ml, Sigma-Aldrich (T2376), USA; stock solution: 100 µg/ml of T4 solved in 40 mM of NaOH and 2 g/L of bovine serum albumin] (30). 2) For TH deprivation (TH low), mice received a low-iodine diet (LoI; MD.1571, Envigo, USA) and drinking water supplemented with 0.04% methimazole [MMI, Sigma-Aldrich (301507), USA], 0.5% sodium perchlorate [ClO4−, Sigma-Aldrich (310514), USA], and 0.3% saccharine as sweetener [Sigma-Aldrich (240931), USA) (LoI/MMI/ClO4−]. In the control group (ctrl), animals were fed a normal diet (MD.1572, Envigo, USA) and received drinking water without supplements. Using the above-described treatment protocol, we have extensively studied male mice without TAC surgery under chronic high or low TH availability and, therefore, decided to investigate male mice in order to focus on the impact of age and TH sensitivity in pathological cardiac hypertrophy (31–33). Furthermore, TAC sham operation has previously been shown not to affect parameters of cardiac function or morphology (28, 29, 34). For these reasons and in line with the “3R concept” in animal research, the present study was done without including an additional sham-operated control group. Except for the low iodine content in the TH deprivation group, the caloric and nutritional composition of the diet was comparable for all mice (MD.1572, Envigo, USA, for the control and T4-treated mice). The indicated treatment started 1 week after TAC surgery and was continued for 2 or 4 weeks (Figure 1A).
2.5 Organ isolation and serum measurements
For organ extraction, mice were anesthetized by i.p. injection of 200 µl of ketamine/xylazine mixture [150 µl of 100 mg/ml ketamine (Betapharm, Germany) and 50 µl of 20 mg/ml xylazine (Ceva, Germany)], and blood was withdrawn by cardiac puncture. Mice were perfused with heparinized saline by transcardial perfusion. Hearts were shock-frozen in liquid N2 and stored at −80°C or fixed in 4% buffered formalin (Formafix, Germany). Blood samples were placed on ice for 30 min and centrifuged, and the concentrations of free triiodothyronine (FT3), free T4 (FT4), and total T4 (TT4) in mouse sera were measured according to the manufacturer’s instructions of commercial ELISA kits (DRG Instruments GmbH, Marburg, Germany) as previously described (25, 31, 35). The detection limit was 0.5 µg/dl, 0.05 ng/dl, and 0.05 pg/ml for TT4, FT4, and FT3, respectively. Values were calculated from the standard curve.
2.6 RNA isolation and qRT-PCR
Total cardiac RNA was isolated and reverse-transcribed to cDNA as previously described (35). In compliance with the guidelines for RT-PCR of MIQE, we used three reference genes to assure correct normalization and calculation: Gapdh (glyceraldehyde-3-phosphate dehydrogenase), Rn18s (18S ribosomal RNA), and Polr2a (polymerase RNA II). The primer sequences are listed in Supplementary Table 1. Analysis and calculation of the fold changes of gene expression were applied to Ct values ≤35. In addition to the 12-month-old mice, RNA samples of the analogously treated 8-week-old mice (generated and published in Kerp et al. (25)) were used for some gene expression analyses as indicated.
2.7 Histological staining
Formalin-fixed hearts were embedded in paraffin. Five-micrometer sections were used for staining. Hematoxylin and eosin (H&E) staining was used for the determination of cardiomyocyte size, and Sirius Red was used for the analysis of fibrosis as described previously (28). For quantification, stained sections were imaged using an Olympus BX51 microscope (Olympus Life Science, Tokyo, Japan). Cross-sectional areas of cardiomyocytes were determined (n = 37–88 cells per animal) using ImageJ. Only cells with a central nucleus were included in the analysis. Fibrosis was calculated as the ratio of red-stained/myocardial area via Adobe Photoshop. Analysis and quantification were performed by blinded researchers.
2.8 TUNEL assay
After dewaxing and rehydration, cardiac sections were permeabilized using proteinase K. For TUNEL (terminal deoxynucleotidyl transferase dUTP nick-end labeling) staining, an in-situ detection kit was used according to the manufacturer’s protocol (Sigma-Aldrich, USA). Sample pretreatment with DNase I served as positive control and TUNEL reaction mixture without terminal transferase (TdT) served as negative control. DAPI (D1306, Thermo Fisher Scientific, USA) and wheat germ agglutinin (W11261, Thermo Fisher Scientific, USA) were used as counterstains for cell nuclei and membranes. Olympus BX51 upright microscope was used for the imaging, and quantification was conducted in a blinded manner.
2.9 Statistical analysis
GraphPad Prism 6 software was used for statistical analysis. Data were checked for normality prior to statistical testing. Two-way ANOVA considering the treatment group (ctrl, TH high, TH low) and time points (regarding weeks after TAC or TH modulation, respectively) followed by Tukey’s post-hoc analysis was used. p-values of *p < 0.05, **p < 0.01, ***p < 0.001, and #p < 0.0001 were considered significant. Outliers were identified using the GraphPad outlier test (excluded if p < 0.05).
3 Results
TH availability may alter the progression of heart disease and the development of heart failure (2, 5, 36). To investigate the impact of aging on this process, we subjected 12-month-old male mice to chronic left ventricular pressure overload induced by TAC. This intervention leads to pathological growth of the heart, i.e., maladaptive cardiac hypertrophy accompanied by interstitial fibrosis and cardiomyocyte death and subsequently to decreased fractional shortening (FS) and the development of congestive heart failure (34).
Changes in TH availability were induced by T4 administration or anti-thyroidal drug treatment (LoI/MMI/ClO4−), respectively, initiated 1 week after TAC surgery and continued over 4 weeks as depicted in the study design (Figure 1A). As expected, 2 and 4 weeks of T4 treatment resulted in increased TT4, FT4, and FT3 serum concentrations (Figures 1B–D). LoI/MMI/ClO4− treatment significantly decreased TT4 and FT4 but not FT3 serum concentrations (Figures 1B–D).
3.1 TH effects on maladaptive cardiac hypertrophy are reduced in 12-month-old mice
Serial echocardiographic measurements were performed to monitor the progression of cardiac hypertrophy and cardiac dysfunction in response to TAC. Aortic pressure gradients induced by TAC were comparable in the different treatment groups and were stable throughout the experiment (Figure 2A). At 1 week of TAC and before manipulation of TH status commenced, a significantly decreased FS was confirmed. Of note, the subsequent alteration of TH levels, i.e., gradual TH increase and TH deprivation, did not alter FS over 4 weeks of treatment (Figure 2B; Table 1). The thickness of the left ventricular wall was significantly increased after 1 week of TAC as depicted by both posterior wall and septum diameters compared with baseline values and hardly changed during the following 4 weeks of observation in the euthyroid and T4-treated groups. Only in the TH low group, a significant decrease of the left ventricular posterior wall thickness (LVPWd; Figure 2C) and of the interventricular septum thickness (IVSd; Figure 2D) was observed after 4 weeks of TH deprivation. However, the left ventricular inner diameter was unaltered at all time points and in all treatment and control groups (Supplementary Figure 1A).
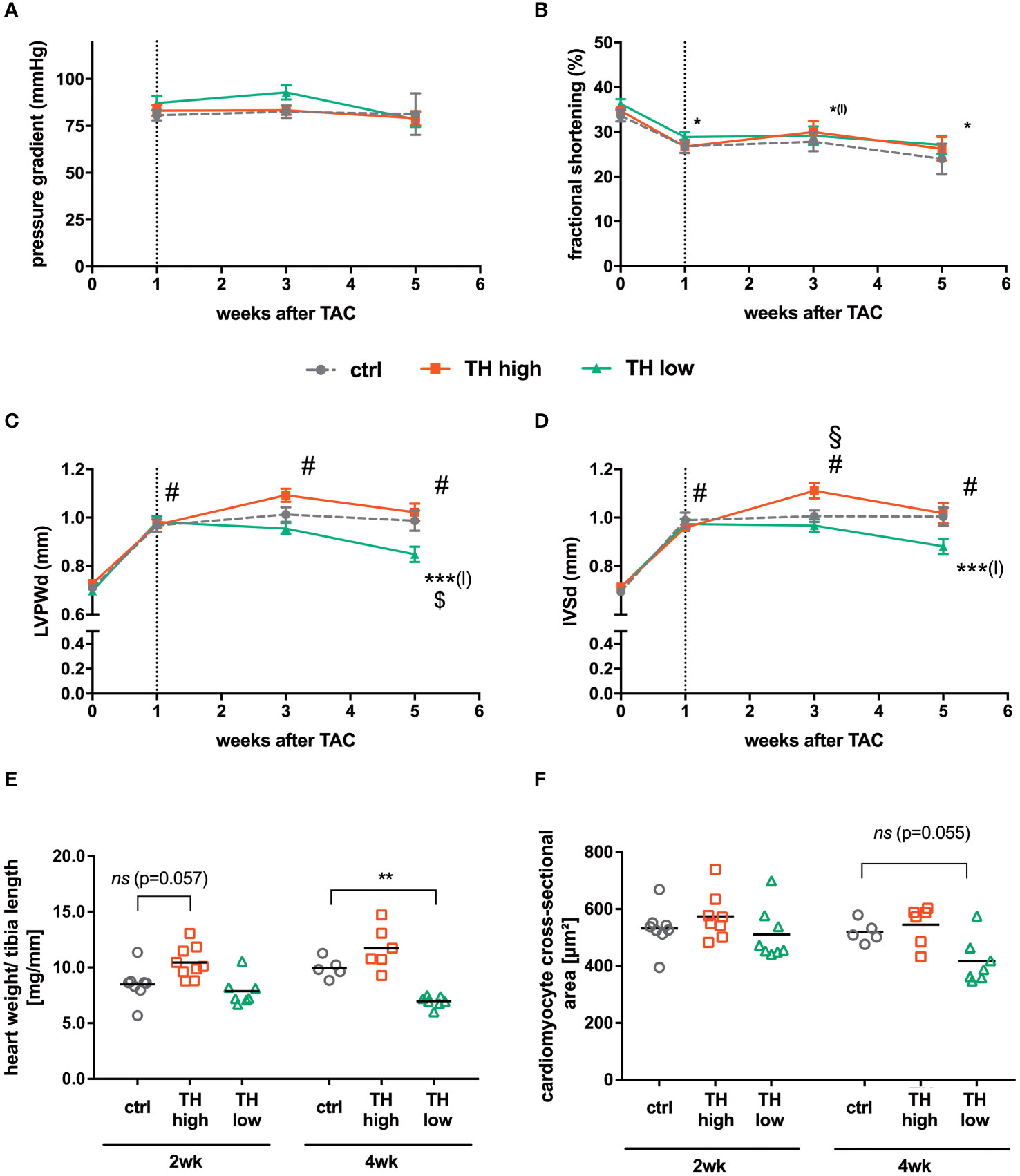
Figure 2 Cardiac function and hypertrophy characteristics. Echocardiography was conducted before TAC and 1, 3, and 5 weeks after surgery. After 1 week, pressure gradients confirmed successful TAC surgery (A) and fractional shortening decreased in all indicated groups compared with baseline values without an apparent impact on thyroid status (B). For cardiac hypertrophy analysis, diastolic left ventricular posterior wall (LVPWd; C) and interventricular septum (IVSd; D) thickness were calculated. In addition, heart weight to tibia length ratios (E) and cardiomyocyte cross-sectional areas (F) were assessed 3 or 5 weeks after TAC [i.e., after 2 or 4 weeks of subsequent modulation of TH status by T4 treatment (TH high) or TH deprivation (TH low)]. TH deprivation reduced cardiac hypertrophy parameters (LVPWd, IVSd, and heart weight to tibia length). Values are indicated as mean ± SEM; for n, see Supplementary Table 1A. #p < 0.0001 (for echocardiographic parameters all groups vs. basal), *(l)p < 0.05 (for TH high vs. basal), ***(l)p < 0.001 (TH low vs. baseline after TAC), $p < 0.05 (TH low vs. ctrl at 5 weeks after TAC), and §p < 0.05 (TH high vs. ctrl at 3 weeks after TAC) by two-way ANOVA and Tukey’s post-hoc analysis; ctrl, control; wk, weeks. The dotted line represents the start of T4 or LoI/MMI/ClO4− treatment.
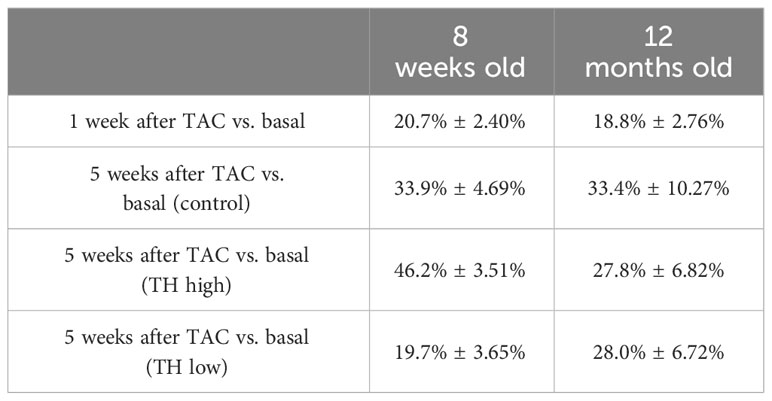
Table 1 Reduction of FS over time in 12-month-old compared with 8-week-old groups (23), receiving the same experimental conditions of TAC surgery and modulation of TH status (TH high: T4 treatment and TH low: TH deprivation).
These echocardiographic data on cardiac hypertrophy were validated by heart weight to tibia length ratios and cardiomyocyte cross-sectional area. Here, a significantly reduced heart weight to tibia length ratio was found under TH deprivation (Figure 2E), and a similar trend was observed for the cardiomyocyte cross-sectional area (Figure 2F). No significant differences were detected after 2 and 4 weeks of T4 treatment (Figures 2E, F). Thus, elevated TH serum concentrations did not affect the development of cardiac hypertrophy, whereas low TH serum concentrations had a mild impact on the progression of cardiac hypertrophy. Neither cardiac function nor lung weight as a measure of cardiac congestion was influenced by altered TH levels during the 4-week treatment interval after induction of left ventricular pressure overload (Supplementary Figure 1B).
3.2 TH-dependent development of fibrosis and apoptosis is attenuated after TAC in 12-month-old mice
Apart from cardiac hypertrophy, deposition of collagen contributing to fibrosis and cardiomyocyte apoptosis are key features of pathological cardiac remodeling, which leads to cardiac dysfunction, altered electrophysiological dynamics, and cardiac tissue stiffness (37). Interstitial fibrosis was histologically analyzed by Sirius Red staining and apoptotic cell death by TUNEL staining (29). No significant alterations in the extent of fibrosis (Figures 3A, B) and apoptosis (Figure 3C) were evident between the treatment groups after 2 and 4 weeks. Furthermore, cardiac expression of atrial natriuretic peptide (Anp) and brain natriuretic peptide (Bnp), markers that correlate with the development of heart failure and pathological cardiac hypertrophy, was analyzed by qRT-PCR (38, 39). Anp expression levels did not change in response to altered TH status, while cardiac Bnp expression was significantly lowered after 4 weeks of TH deprivation compared with controls (Figures 3D, E).
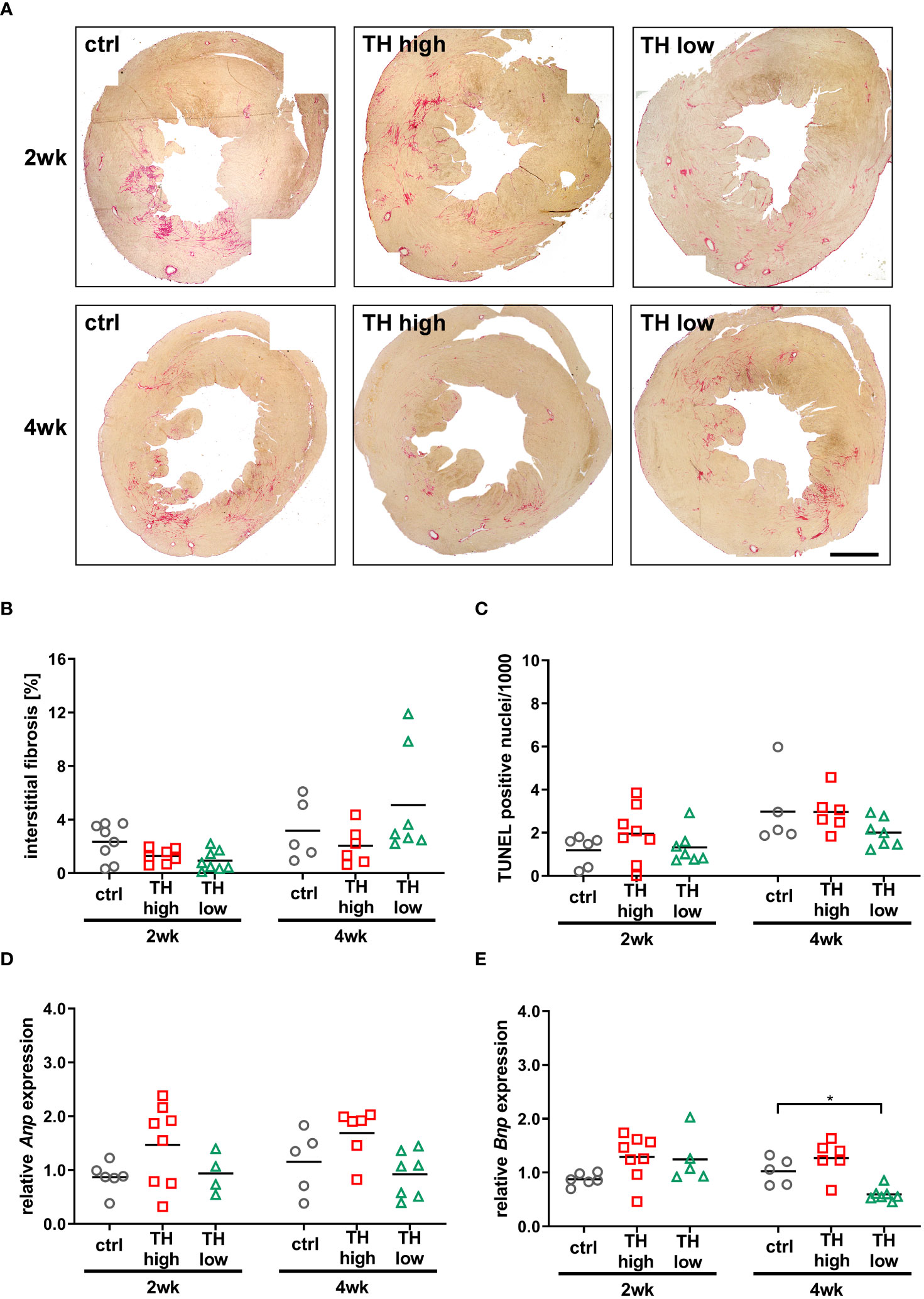
Figure 3 Analysis of fibrosis, apoptosis, and cardiac stress markers at 3 or 5 weeks after TAC [i.e., after 2 or 4 weeks of subsequent modulation of TH status by T4 treatment (TH high) or TH deprivation (TH low)]. Representative pictures of Sirius Red-stained sections (A, scale bar = 1,000 µm) and relative quantification of interstitial fibrosis (B) are shown. TUNEL-positive nuclei were counted to assess apoptotic rate (C) and Anp and Bnp expression as cardiac stress markers (D, E). TH deprivation reduced cardiac Bnp expression in 12-month-old mouse hearts at 5 weeks after TAC. Scatter dot plot and mean in all panels, *p < 0.05 by two-way ANOVA and Tukey’s post-hoc analysis; ctrl, control; wk, weeks.
Moreover, despite the absence of apparent features of heart failure, three death events occurred in the control group and two in the T4 treatment group in comparison to none in TH-deprived mice within 4 weeks of modulation of TH status.
3.3 TH responsiveness of TAC-subjected hearts reflects low and high TH tissue states
To answer whether the higher or lower TH availability in mice translated into altered expression of genes associated with hypo- or hyperthyroidism (13) and TH target gene expression of myosin heavy chain alpha (Myh6) and beta (Myh7), ATPase sarcoplasmic/endoplasmic reticulum Ca2+ transporting 2 (Serca2a2) and ryanodine receptor 2 (Ryr2) was analyzed in mouse hearts, sacrificed after 2 and 4 weeks of treatment. As observed upon TH deprivation in previous studies (25), Myh6 levels were significantly lowered and Myh7 expression increased after 2 and 4 weeks of low TH treatment. High TH treatment did not affect Myh6 expression but resulted in reduced expression of Myh7 (Figures 4A, B). Expression of Ryr2 was decreased in the low TH group only after 2 weeks of treatment, whereas expression of Serca2a2 was significantly reduced after 2 and 4 weeks under TH deprivation (Figures 4C, D). Thus, both treatments leading to high or low TH serum concentration, respectively, caused the expected gene alteration in cardiac muscle. However, the effect on cardiac TH target gene expression was greater under TH deprivation than under T4 treatment.
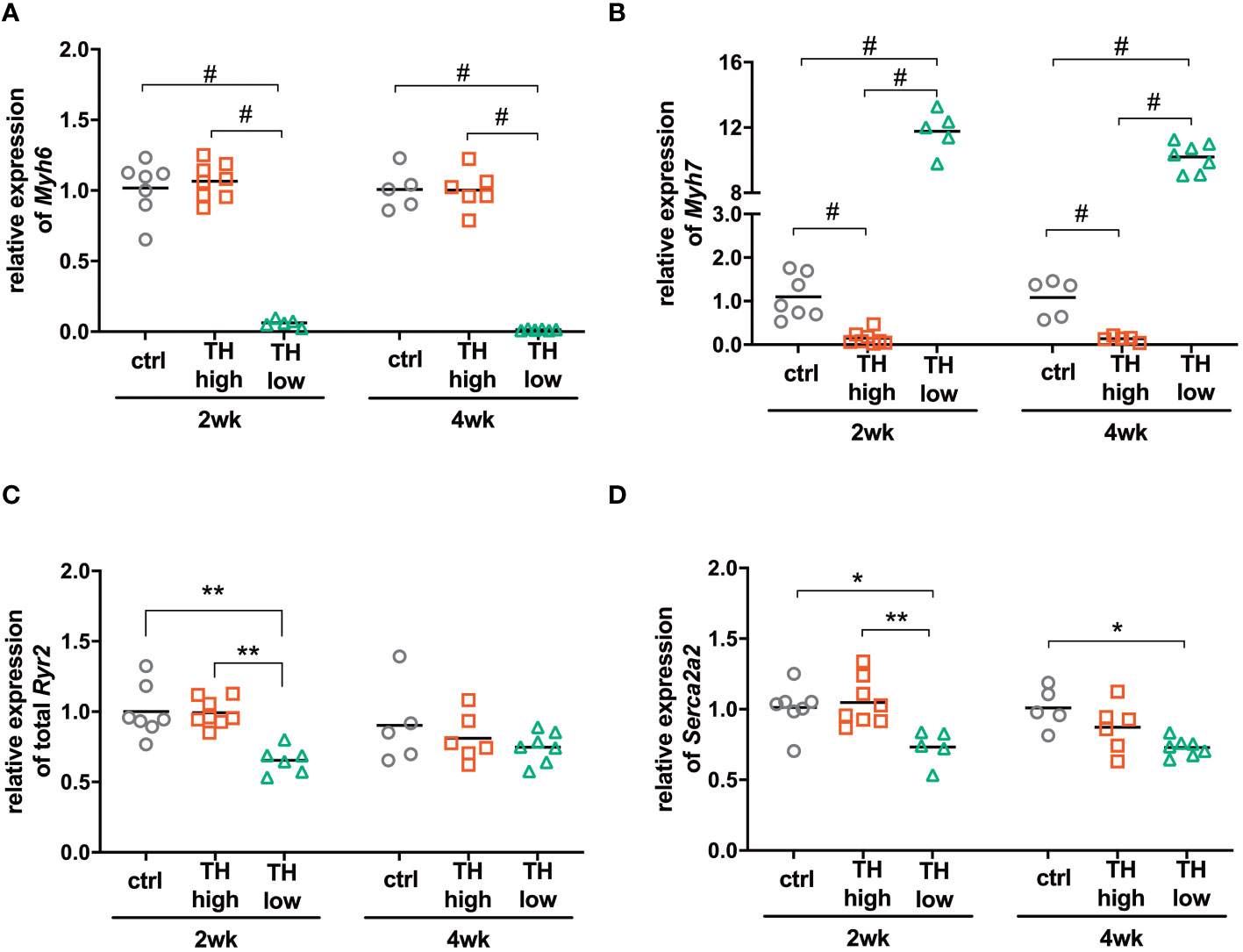
Figure 4 Expression of TH-responsive genes in the hearts of mice subjected to TAC and subsequent modulation of TH status by T4 treatment (TH high) or TH deprivation (TH low). The amount of Myh6 (A), Myh7 (B), Ryr2 (C), and Atp2a2 (D) transcripts was determined in mouse hearts by qRT-PCR after 2 and 4 weeks of treatment. Scatter dot plot and mean in all panels, one-way ANOVA with Tukey’s post-hoc test, *p < 0.05, **p < 0.01, #p < 0.0001; ctrl, control; wk, weeks.
We also measured the expression of the TAC-associated genes (Col1a1, Atp5g1, Ndufa5, Uqcrq, Mmp2, and Pdk4) according to Li et al. (40). There were no considerable differences detectable except for the expression of Pdk4 that showed an expression pattern that correlated with TH treatment (TH high and low, respectively) in the 4-week groups (Supplementary Figure 2).
3.4 Attenuated TH response in 12-month-old mice is associated with altered TRα isoform and deiodinase expression
Compared with young TAC mice (8-week-old), as previously published (25), we noticed reduced TH effects on TAC-related parameters in this aged cohort. To identify the underlying molecular causes, we decided to analyze TH-signaling features, i.e., gene expression of deiodinases and TR isoforms. Gene expression analyses of Thra and Thrb encoding for TH receptor alpha (TRα) and beta (TRβ), respectively, confirmed the predominant expression of TRα over TRβ in the heart (Supplementary Figure 3). Expression of TRα was decreased under high TH and increased under low TH, while TRβ was unaffected (Supplementary Figure 3). Interestingly, in older mice, the analysis of TRα isoform expression revealed that the proportion of TRα2, the isoform that is not able to bind TH, was increased compared with young mice. The expression of TRα2 further increased after 4 weeks of treatment, irrespective of the treatment regime (Figure 5A). This increase of the TRα2 isoform was less pronounced in young mice (Figure 5B). Moreover, the older mice had in general a higher proportion of TRα2 (2 weeks, 12.0% ± 1.3% vs. 7.7% ± 1.3% and 4 weeks 16.3% ± 1.7% vs. 10% ± 1.3%, old vs. young, respectively). A closer look at the TRα isoform distribution revealed that the expression of both TRα1 and TRα2 is higher in older mice compared with young mice (Figure 5C). Next, we measured the gene expression levels of deiodinases 2 and 3 encoded by Dio2 and Dio3, respectively. After 2 weeks of treatment, the expression of Dio2 was significantly higher in euthyroid and T4-treated young mice compared with the same groups of 12-month-old mice (Figure 5D, left panel). This difference disappeared after 4 weeks of treatment (Figure 5D, right panel). Interestingly, contrary to Dio2, the expression of Dio3 was higher in euthyroid and hypothyroid older mice compared with young mice, reaching a significant difference under TH deprivation (Figure 5E).
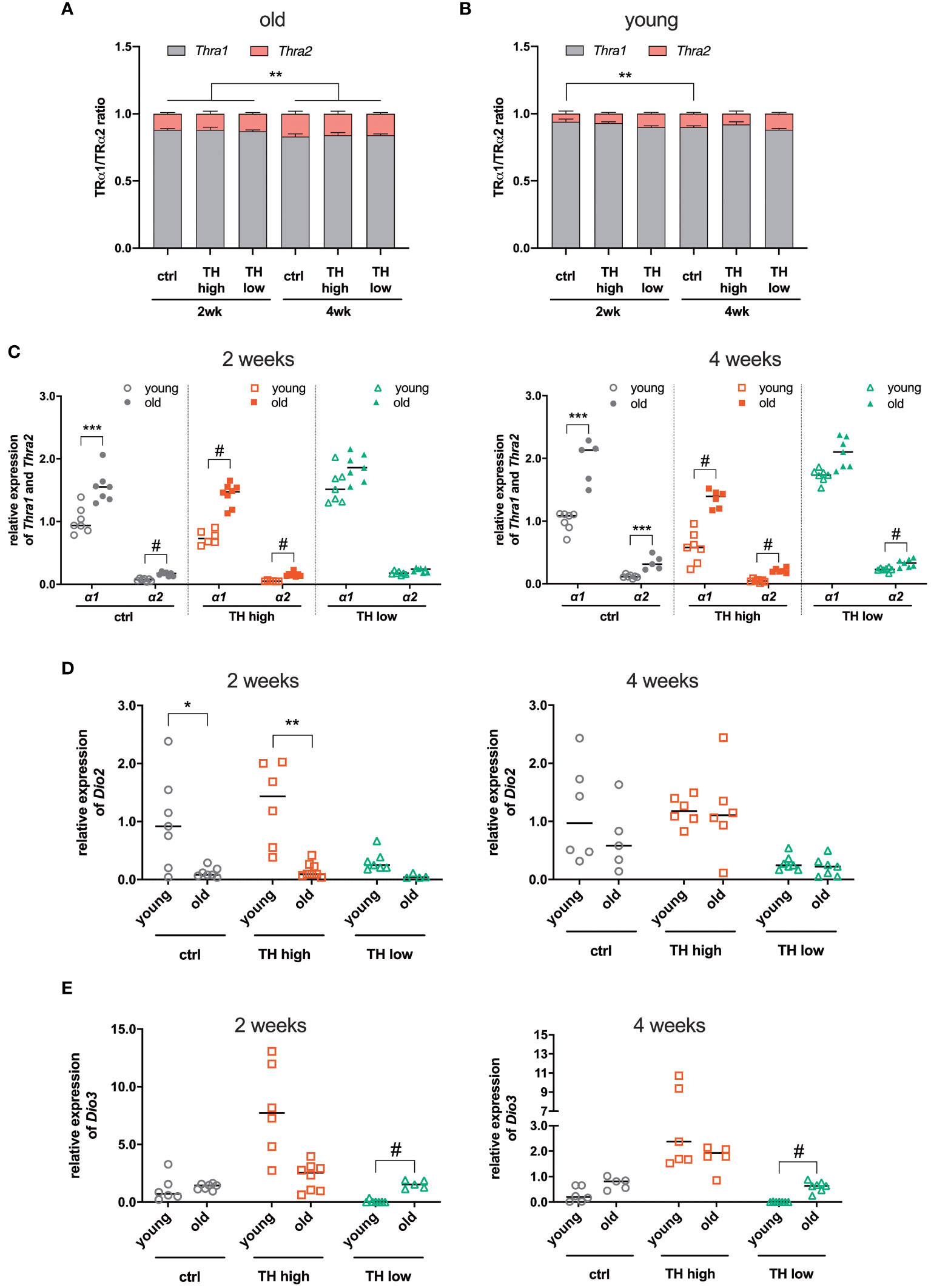
Figure 5 Comparison of gene expression between young and old mice after TAC. The expression ratio of TRα isoforms 1 and 2, encoded by Thra1 and Thra2 transcripts, respectively, in the hearts from (A) old (12-month-old) and (B) young mice (8-week-old) was assessed by qRT-PCR. (C) Relative gene expression of TRα1 and 2 in the hearts from young and old mice, 2 and 4 weeks after treatment, as well as Dio2 (D) and Dio3 (E). Scatter dot plot and mean in all (C, D), one-way ANOVA with Tukey’s post-hoc test, *p < 0.05, **p < 0.01, ***p < 0.001, #p < 0.0001; ctrl, control; wk, weeks.
4 Discussion
In our study, we investigated the impact of high and low TH availability after pressure overload-induced cardiac dysfunction in male 12-month-old mice. The aim of the study was to delineate the sensitivity to altered TH levels in aged mice in the context of “predamaged” hearts with left ventricular pressure overload.
Chronic pressure overload is a pathological condition that triggers the development of heart failure. Left ventricular pressure overload can be caused by arterial hypertension or aortic stenosis and TAC as a mouse model mimicking such a condition is widely used. TAC induces the development of cardiac hypertrophy, fibrosis, an increase in apoptosis, and eventually cardiac dilatation and congestive heart failure, characteristic features of heart disease induced by chronic pressure overload (28, 41).
Previously, we showed that modulation of the TH status 1 week after TAC surgery in young 8-week-old mice impressively impacted the outcome of cardiac recovery. In contrast to controls, TH deprivation in young mice stopped the progression of cardiac hypertrophy and cardiac dysfunction with mild improvement of FS, whereas elevated TH availability increased apoptosis, did not prevent hypertrophy, and decreased FS (25).
These results prompted us to add aging as another important modifier to this experimental setup since the prevalence of cardiac and TH-related diseases increases with age (10, 11) and sensitivity to TH and cardiovascular outcome changes with aging. TH action in aged mice has been well-documented and findings from our previous studies suggested decreased systemic effects in aged animals (31, 35). The heart as one of the main TH target organs was more likely to have a preserved TH action compared with other organs (35, 42). However, chronic TH excess in old mice led to a similar extent of cardiac hypertrophy, tachycardia, and regulation of TH-responsive gene expression as in young mice, whereas chronic TH deprivation resulted in an attenuated cardiac phenotype with less pronounced bradycardia and degree of gene regulation (31).
The effects of aging on cardiac hypertrophy and cardiac disease in animal models have been occasionally reported. Hypertrophic response of the left ventricle 4 weeks after ascending aortic banding in rats of 9 and 18 months of age showed a diminished degree of myocardial hypertrophy in old rats in terms of muscle weight, size of myocytes, and protein content (43–45). Mouse studies reported contradictory results of either worse response to chronic pressure overload and lower survival of old animals (46) or less left ventricular remodeling and lower mortality than young mice after TAC (47). However, so far, the impact of TH on TAC-induced heart disease has not been studied in the context of aging.
Due to the limited availability of aged TAC mouse models, our first aim was to identify similar conditions in 12-month-old as compared with 8-week-old mice for our setup so that aortic pressure gradients would not differ in older mice. This was achieved by using a bigger needle size (25G) during TAC, which resulted in a similar decline in FS within 1 week after surgery in aged compared with our previous study in young mice (25) and confirmed our approach to achieve age-independent conditions for TAC within the experimental time prior to TH alterations. Four weeks of T4 or LoI/MMI/ClO4− treatment resulted in distinct effects on fractional shortening in both age groups, i.e., fractional shortening worsened in young mice with high TH levels and improved with low TH levels, whereas fractional shortening was hardly affected by low or high TH levels in old mice (Table 1).
Thus, TH alterations had no obvious detrimental or beneficial effects within the studied time frame in older mice. As no age effect was noted in the control group, we consider these findings unlikely to be a result of an inappropriate experimental setting.
Another influencing aspect might be that treatment time and dosage have to be age-adjusted to achieve comparable effects in younger and older individuals. Of note, the modulation of TH status in the present study led to similar changes in TH serum concentrations and cardiac gene expression as observed in young mice, further supporting comparable experimental conditions between the study in young mice and the herein-reported study in older mice (25). However, FT3 was not significantly reduced by LoI/MMI/ClO4− treatment as seen in young mice. We previously showed that aging is associated with a low thyroid state resulting in lower basal FT3 concentrations in old mice compared with young mice (35). Therefore, the missing drop in FT3 could be a consequence of lower basal FT3 concentrations attenuating the effect of LoI/MMI/ClO4− treatment.
Age has an effect on the progression of TH-induced physiological cardiac hypertrophy as early studies described a delayed development of cardiac hypertrophy in senescent mice upon thyroxine treatment. However, already after 9 days of treatment, no age differences were apparent anymore. In addition, different dosages were tested, and even with the lowest (1.7 µg/g BW), no age dependency of cardiac hypertrophy was noted (35, 48). In our present study and in response to TAC, however, the responsiveness of the heart to high or low serum TH levels was diminished compared with young mice (25). Aging per se reduces basal TH tissue content (35) and alters TH-dependent gene expression in the heart (42). Here, we show that there is also a different expression of TRα isoforms and deiodinases 2 and 3 in 12-month-old mice compared with young mice that have undergone TAC surgery. Noteworthy, we detected a shift in the TRα isoform ratio toward more TRα2 expression. TRα2 is incapable of binding TH and, thus, has a dominant-negative effect on TRα1 action (49). This increase was not affected by TH modulation, i.e., TH deprivation and TH treatment. However, as there was more TRα2 in the hearts of all groups after 4 weeks of treatment, we suggest that the amount of TRα2 correlates with the progression of cardiac hypertrophy. This is supported by previous studies that showed a shift from TRα1 to TRα2 expression in the human heart during heart failure (50, 51). Moreover, elevated TRα2 expression was found to attenuate TRα1-mediated hypertrophy in this condition (52). These findings suggest that the higher expression of TRα2 reduces TH response in 12-month-old mouse hypertrophied hearts. Interestingly, in addition to higher levels of TRα2, we found a reduced expression of Dio2 in aged mice. Reduced expression of Dio2 leads to less conversion of T4 to T3, consequently resulting in lower local T3 tissue content. This is further accompanied by higher cardiac expression of the TH-inactivating Dio3 in aged compared with young mice and could further reduce TH availability in aged hearts. This is in line with previous findings reporting lower T3 content in cardiac tissue of old mice (35). Briefly, the combination of a reduced TH availability caused by a reduction in Dio2 and higher expression of Dio3 together with an increased expression of the dominant-negative TRα2 together might provide a molecular explanation for the diminished TH response in aged TAC mice. Therefore, these factors might contribute to the outcome of TH modulation in the pathophysiology of heart disease.
TH deprivation has been studied less frequently. Thus, it remains speculative whether treatment time or dosage would affect the outcome of TAC in older mice. In addition, other studies suggested that cardiac hypertrophy significantly depends on indirect TH action in the central nervous system rather than direct TH effects on heart tissue (53, 54). Thus, the observed age difference could also arise from altered central TH rather than cardiac TH signaling in young vs. old mice with induced pressure overload (33).
Most clinical studies include patients with differing ages, sex, comorbidities, and different causes of cardiac dysfunction as well as common endpoints such as all-cause mortality or cardiovascular death in correlation with the thyroid status so that conclusions for therapeutic TH modulation are difficult (8, 55, 56). TH modulation may have different impacts in different subgroups of heart failure patients, e.g., in heart failure patients with or without atrial fibrillation (56) and in patients with congestive or dilative heart failure, with or without preexisting thyroid dysfunction. In line with these diverse factors that may impact the outcome of TH modulation, e.g., via altered Dio2/3 and TRα1/2 ratios and thus the availability of TH, also the timing and extent of TH modulation may be relevant (57–60).
The data of our current mouse study are focused and limited to TH sensitivity in 12-month-old mice after pressure overload-induced hypertrophy and cardiac dysfunction but suggest that TH modulation (high and low levels) at an older age—at least within a certain time frame and in the herein investigated male mice—has less impact to foster key risk factors of heart failure.
Taken together, certain subgroups of patients with preexisting cardiovascular disease may respond to manipulation of TH status with a beneficial or detrimental outcome. Our study suggests a different outcome to TH modulation based on age; thus, the recognition of differences due to, e.g., age, sex, cardiovascular diseases, and risk factors, needs to be included in future TH studies.
Data availability statement
The original contributions presented in the study are included in the article/Supplementary Material, further inquiries can be directed to the corresponding author.
Ethics statement
The animal study was approved by Landesamt für Natur, Umwelt und Verbraucherschutz Nordrhein-Westfalen, Germany. The study was conducted in accordance with the local legislation and institutional requirements.
Author contributions
HK: Writing – original draft, Investigation, Formal analysis. JG: Writing – review & editing, Investigation, Formal analysis. SG: Writing – review & editing, Formal analysis. GSH: Writing – review & editing, Investigation, Formal analysis. SD: Writing – review & editing, Formal analysis. JM: Writing – review & editing, Validation. NH: Writing – review & editing, Formal analysis. FK: Writing – review & editing, Validation. LCM: Writing – review & editing, Validation. KL: Writing – original draft, Conceptualization, Investigation. DF: Writing – original draft, Conceptualization.
Funding
The author(s) declare financial support was received for the research, authorship, and/or publication of this article. This work was supported by the Deutsche Forschungsgemeinschaft in the framework of SFB/TR 296 LOCOTACT funding ID 424957847 (DF, LCM, JM, FK and KL) and SFB1525 funding ID B03/453989101 (KL), the German Ministry of Research and Education (BMBF; ERK-Casting), the Ministry for Innovation, Science and Research of the Federal State of North Rhine Westphalia.
Acknowledgments
The authors thank the Imaging Core Facility Essen (IMCES) for expert technical assistance. We are also grateful to K. Schättel, S. Rehn, and A. Jaeger for their dedicated technical support. We acknowledge the support by the Open Access Publication Fund of the University of Duisburg-Essen.
Conflict of interest
The authors declare that the research was conducted in the absence of any commercial or financial relationships that could be construed as a potential conflict of interest.
The author(s) declared that they were an editorial board member of Frontiers, at the time of submission. This had no impact on the peer review process and the final decision.
Publisher’s note
All claims expressed in this article are solely those of the authors and do not necessarily represent those of their affiliated organizations, or those of the publisher, the editors and the reviewers. Any product that may be evaluated in this article, or claim that may be made by its manufacturer, is not guaranteed or endorsed by the publisher.
Supplementary material
The Supplementary Material for this article can be found online at: https://www.frontiersin.org/articles/10.3389/fendo.2024.1339741/full#supplementary-material
References
1. Bragazzi NL, Zhong W, Shu J, Abu Much A, Lotan D, Grupper A, et al. Burden of heart failure and underlying causes in 195 countries and territories from 1990 to 2017. Eur J Prev Cardiol. (2021) 28:1682–90. doi: 10.1093/eurjpc/zwaa147
2. Dillmann W. Cardiac hypertrophy and thyroid hormone signaling. Review Heart failure Rev. (2010) 15:125–32. doi: 10.1007/s10741-008-9125-7
3. Pantos C, Mourouzis I, Cokkinos DV. New insights into the role of thyroid hormone in cardiac remodeling: time to reconsider? Heart Fail Rev. (2011) 16:79–96. doi: 10.1007/s10741-010-9185-3
4. Jabbar A, Pingitore A, Pearce SH, Zaman A, Iervasi G, Razvi S. Thyroid hormones and cardiovascular disease. Nat Rev Cardiol. (2017) 14:39–55. doi: 10.1038/nrcardio.2016.174
5. Razvi S, Jabbar A, Pingitore A, Danzi S, Biondi B, Klein I, et al. Thyroid hormones and cardiovascular function and diseases. J Am Coll Cardiol. (2018) 71:1781–96. doi: 10.1016/j.jacc.2018.02.045
6. Debmalya S, Saumitra R, Singh MH. Interplay between cardiovascular and thyroid dysfunctions: A review of clinical implications and management strategies. Endocr Regul. (2022) 56:311–28. doi: 10.2478/enr-2022-0033
7. Paschou SA, Bletsa E, Stampouloglou PK, Tsigkou V, Valatsou A, Stefanaki K, et al. Thyroid disorders and cardiovascular manifestations: an update. Endocrine. (2022) 75:672–83. doi: 10.1007/s12020-022-02982-4
8. Cappola AR, Desai AS, Medici M, Cooper LS, Egan D, Sopko G, et al. Thyroid and cardiovascular disease: research agenda for enhancing knowledge, prevention, and treatment. Circulation. (2019) 139:2892–909. doi: 10.1161/CIRCULATIONAHA.118.036859
9. Barbesino G. Thyroid function changes in the elderly and their relationship to cardiovascular health: A mini-review. Gerontology. (2019) 65:1–8. doi: 10.1159/000490911
10. Waring AC, Arnold AM, Newman AB, Buzkova P, Hirsch C, Cappola AR. Longitudinal changes in thyroid function in the oldest old and survival: the cardiovascular health study all-stars study. J Clin Endocrinol Metab. (2012) 97:3944–50. doi: 10.1210/jc.2012-2481
11. Mozaffarian D, Benjamin EJ, Go AS, Arnett DK, Blaha MJ, Cushman M, et al. Executive summary: heart disease and stroke statistics–2016 update: A report from the American heart association. Circulation. (2016) 133:447–54. doi: 10.1161/CIR.0000000000000366
12. Li M, Iismaa SE, Naqvi N, Nicks A, Husain A, Graham RM. Thyroid hormone action in postnatal heart development. Stem Cell Res. (2014) 13:582–91. doi: 10.1016/j.scr.2014.07.001
13. Dillmann WH. Biochemical basis of thyroid hormone action in the heart. Am J Med. (1990) 88:626–30. doi: 10.1016/0002-9343(90)90530-q
14. Kahaly GJ, Dillmann WH. Thyroid hormone action in the heart. Endocr Rev. (2005) 26:704–28. doi: 10.1210/er.2003-0033
15. Holt E, Sjaastad I, Lunde PK, Christensen G, Sejersted OM. Thyroid hormone control of contraction and the Ca(2+)-ATPase/phospholamban complex in adult rat ventricular myocytes. J Mol Cell Cardiol. (1999) 31:645–56. doi: 10.1006/jmcc.1998.0900
16. Kiss E, Jakab G, Kranias EG, Edes I. Thyroid hormone-induced alterations in phospholamban protein expression. Regulatory effects on sarcoplasmic reticulum Ca2+ transport and myocardial relaxation. Circ Res. (1994) 75:245–51. doi: 10.1161/01.RES.75.2.245
17. Dore R, Watson L, Hollidge S, Krause C, Sentis SC, Oelkrug R, et al. Resistance to thyroid hormone induced tachycardia in RTHalpha syndrome. Nat Commun. (2023) 14:3312. doi: 10.1038/s41467-023-38960-1
18. Shimizu I, Minamino T. Physiological and pathological cardiac hypertrophy. J Mol Cell Cardiol. (2016) 97:245–62. doi: 10.1016/j.yjmcc.2016.06.001
19. Klein I, Danzi S. Thyroid disease and the heart. Circulation. (2007) 116:1725–35. doi: 10.1161/CIRCULATIONAHA.106.678326
20. Thomas TA, Kuzman JA, Anderson BE, Andersen SM, Schlenker EH, Holder MS, et al. Thyroid hormones induce unique and potentially beneficial changes in cardiac myocyte shape in hypertensive rats near heart failure. Am J Physiol Heart Circ Physiol. (2005) 288:H2118–22. doi: 10.1152/ajpheart.01000.2004
21. Gerdes AM, Iervasi G. Thyroid replacement therapy and heart failure. Circulation. (2010) 122:385–93. doi: 10.1161/circulationaha.109.917922
22. Gerdes AM, Moore JA, Hines JM. Regional changes in myocyte size and number in propranolol-treated hyperthyroid rats. Lab Invest. (1987) 57:708–13.
23. Ito K, Kagaya Y, Shimokawa H. Thyroid hormone and chronically unloaded hearts. Vascul Pharmacol. (2010) 52:138–41. doi: 10.1016/j.vph.2009.10.004
24. Yao J, Eghbali M. Decreased collagen gene expression and absence of fibrosis in thyroid hormone-induced myocardial hypertrophy. Response of cardiac fibroblasts to thyroid hormone in vitro. Circ Res. (1992) 71:831–9. doi: 10.1161/01.res.71.4.831
25. Kerp H, Hönes GS, Tolstik E, Hones-Wendland J, Gassen J, Moeller LC, et al. Protective effects of thyroid hormone deprivation on progression of maladaptive cardiac hypertrophy and heart failure. Front Cardiovasc Med. (2021) 8:683522. doi: 10.3389/fcvm.2021.683522
26. Bazgir F, Nau J, Nakhaei-Rad S, Amin E, Wolf MJ, Saucerman JJ, et al. The microenvironment of the pathogenesis of cardiac hypertrophy. Cells. (2023) 12:1780–814. doi: 10.3390/cells12131780
27. van Berlo JH, Maillet M, Molkentin JD. Signaling effectors underlying pathologic growth and remodeling of the heart. J Clin Invest. (2013) 123:37–45. doi: 10.1172/jci62839
28. Lorenz K, Schmitt JP, Schmitteckert EM, Lohse MJ. A new type of ERK1/2 autophosphorylation causes cardiac hypertrophy. Nat Med. (2009) 15:75–83. doi: 10.1038/nm.1893
29. Schmid E, Neef S, Berlin C, Tomasovic A, Kahlert K, Nordbeck P, et al. Cardiac RKIP induces a beneficial beta-adrenoceptor-dependent positive inotropy. Nat Med. (2015) 21:1298–306. doi: 10.1038/nm.3972
30. Engels K, Rakov H, Zwanziger D, Hönes GS, Rehders M, Brix K, et al. Efficacy of protocols for induction of chronic hyperthyroidism in male and female mice. Endocrine. (2016) 54:47–54. doi: 10.1007/s12020-016-1020-8
31. Engels K, Rakov H, Hones GS, Brix K, Kohrle J, Zwanziger D, et al. Aging alters phenotypic traits of thyroid dysfunction in male mice with divergent effects on complex systems but preserved thyroid hormone action in target organs. Journals Gerontol Ser A Biol Sci Med Sci. (2019) 74(8):1162–9. doi: 10.1093/gerona/glz040
32. Weiss RE, Korcarz C, Chassande O, Cua K, Sadow PM, Koo E, et al. Thyroid hormone and cardiac function in mice deficient in thyroid hormone receptor-alpha or -beta: an echocardiograph study. Research Support, Non-U.S. Gov't Research Support, U.S. Gov't, P.H.S. Am J Physiol Endocrinol Metab. (2002) 283:E428–35. doi: 10.1152/ajpendo.00019.2002
33. Kerp H, Engels K, Kramer F, Doycheva D, Hönes GS, Zwanziger D, et al. Age effect on thyroid hormone brain response in male mice. Endocrine. (2019) 66:596–606. doi: 10.1007/s12020-019-02078-6
34. Tomasovic A, Brand T, Schanbacher C, Kramer S, Hummert MW, Godoy P, et al. Interference with ERK-dimerization at the nucleocytosolic interface targets pathological ERK1/2 signaling without cardiotoxic side-effects. Nat Commun. (2020) 11:1733. doi: 10.1038/s41467-020-15505-4
35. Rakov H, De Angelis M, Renko K, Hoenes S, Zwanziger D, Moeller LC, et al. Aging is associated with low thyroid state and organ specific sensitivity to thyroxine. Thyroid. (2019) 29(12):1723–33. doi: 10.1089/thy.2018.0377
36. Pantos C, Dritsas A, Mourouzis I, Dimopoulos A, Karatasakis G, Athanassopoulos G, et al. Thyroid hormone is a critical determinant of myocardial performance in patients with heart failure: potential therapeutic implications. Eur J Endocrinol. (2007) 157:515–20. doi: 10.1530/EJE-07-0318
37. Leask A. Getting to the heart of the matter: new insights into cardiac fibrosis. Circ Res. (2015) 116:1269–76. doi: 10.1161/CIRCRESAHA.116.305381
38. Maisel A. B-type natriuretic peptide levels: a potential novel "white count" for congestive heart failure. J Card Fail. (2001) 7:183–93. doi: 10.1054/jcaf.2001.24609
39. Brandt RR, Wright RS, Redfield MM, Burnett JC Jr. Atrial natriuretic peptide in heart failure. J Am Coll Cardiol. (1993) 22:86A–92A. doi: 10.1016/0735-1097(93)90468-g
40. Li X, Tan W, Zheng S, Pyle WG, Zhu C, Chen H, et al. Differential mRNA expression and circular RNA-based competitive endogenous RNA networks in the three stages of heart failure in transverse aortic constriction mice. Front Physiol. (2022) 13:777284. doi: 10.3389/fphys.2022.777284
41. Lips DJ, deWindt LJ, van Kraaij DJ, Doevendans PA. Molecular determinants of myocardial hypertrophy and failure: alternative pathways for beneficial and maladaptive hypertrophy. Eur Heart J May. (2003) 24:883–96. doi: 10.1016/s0195-668x(02)00829-1
42. Visser WE, Bombardieri CR, Zevenbergen C, Barnhoorn S, Ottaviani A, van der Pluijm I, et al. Tissue-specific suppression of thyroid hormone signaling in various mouse models of aging. PloS One. (2016) 11:e0149941. doi: 10.1371/journal.pone.0149941
43. Isoyama S, Sato F, Takishima T. Effect of age on coronary circulation after imposition of pressure-overload in rats. Hypertension. (1991) 17:369–77. doi: 10.1161/01.hyp.17.3.369
44. Isoyama S, Wei JY, Izumo S, Fort P, Schoen FJ, Grossman W. Effect of age on the development of cardiac hypertrophy produced by aortic constriction in the rat. Circ Res. (1987) 61:337–45. doi: 10.1161/01.res.61.3.337
45. Isoyama S, Nitta-Komatsubara Y. Acute and chronic adaptation to hemodynamic overload and ischemia in the aged heart. Heart Fail Rev. (2002) 7:63–9. doi: 10.1023/A:1013701923065
46. Sopko NA, Turturice BA, Becker ME, Brown CR, Dong F, Popovic ZB, et al. Bone marrow support of the heart in pressure overload is lost with aging. PloS One. (2010) 5:e15187. doi: 10.1371/journal.pone.0015187
47. Geng X, Hwang J, Ye J, Shih H, Coulter B, Naudin C, et al. Aging is protective against pressure overload cardiomyopathy via adaptive extracellular matrix remodeling. Am J Cardiovasc Dis. (2017) 7:72–82.
48. Florini JR, Saito Y, Manowitz EJ. Effect of age on thyroxine-induced cardiac hypertrophy in mice. J Gerontol. (1973) 28:293–7. doi: 10.1093/geronj/28.3.293
49. Hönes GS, Härting N, Mittag J, Kaiser FJ. TRalpha2 –an untuned second fiddle or fine-tuning thyroid hormone action? Int J Mol Sci. (2022) 23:6998. doi: 10.3390/ijms23136998
50. Sylvén C, Jansson E, Sotonyi P, Waagstein F, Barkhem T, Brönnegård M. Cardiac nuclear hormone receptor mRNA in heart failure in man. Life Sci. (1996) 59:1917–22. doi: 10.1016/s0024-3205(96)00539-5
51. Kinugawa K, Minobe WA, Wood WM, Ridgway EC, Baxter JD, Ribeiro RC, et al. Signaling pathways responsible for fetal gene induction in the failing human heart: evidence for altered thyroid hormone receptor gene expression. Circulation. (2001) 103:1089–94. doi: 10.1161/01.cir.103.8.1089
52. Kinugawa K, Jeong MY, Bristow MR, Long CS. Thyroid hormone induces cardiac myocyte hypertrophy in a thyroid hormone receptor alpha1-specific manner that requires TAK1 and p38 mitogen-activated protein kinase. Mol Endocrinol. (2005) 19:1618–28. doi: 10.1210/me.2004-0503
53. Bachman ES, Hampton TG, Dhillon H, Amende I, Wang J, Morgan JP, et al. The metabolic and cardiovascular effects of hyperthyroidism are largely independent of beta-adrenergic stimulation. Endocrinology. (2004) 145:2767–74. doi: 10.1210/en.2003-1670
54. Herrmann B, Harder L, Oelkrug R, Chen J, Gachkar S, Nock S, et al. Central hypothyroidism impairs heart rate stability and prevents thyroid hormone-induced cardiac hypertrophy and pyrexia. Thyroid. (2020) 30:1205–16. doi: 10.1089/thy.2019.0705
55. Wang X, Wang H, Li Q, Wang P, Xing Y, Zhang F, et al. Effect of levothyroxine supplementation on the cardiac morphology and function in patients with subclinical hypothyroidism: A systematic review and meta-analysis. J Clin Endocrinol Metab. (2022) 107:2674–83. doi: 10.1210/clinem/dgac417
56. Cappola AR, Fried LP, Arnold AM, Danese MD, Kuller LH, Burke GL, et al. Thyroid status, cardiovascular risk, and mortality in older adults. Jama. (2006) 295:1033–41. doi: 10.1001/jama.295.9.1033
57. Mourouzis I, Mantzouratou P, Galanopoulos G, Kostakou E, Roukounakis N, Kokkinos AD, et al. Dose-dependent effects of thyroid hormone on post-ischemic cardiac performance: potential involvement of Akt and ERK signalings. Mol Cell Biochem. (2012) 363:235–43. doi: 10.1007/s11010-011-1175-9
58. Pantos CI, Trikas AG, Pissimisis EG, Grigoriou KP, Stougiannos PN, Dimopoulos AK, et al. Effects of acute triiodothyronine treatment in patients with anterior myocardial infarction undergoing primary angioplasty: evidence from a pilot randomized clinical trial (ThyRepair study). Thyroid. (2022) 32:714–24. doi: 10.1089/thy.2021.0596
59. Jabbar A, Ingoe L, Junejo S, Carey P, Addison C, Thomas H, et al. Effect of levothyroxine on left ventricular ejection fraction in patients with subclinical hypothyroidism and acute myocardial infarction: A randomized clinical trial. Jama. (2020) 324:249–58. doi: 10.1001/jama.2020.9389
Keywords: maladaptive cardiac hypertrophy, thyroid hormone, transverse aortic constriction, aging, thyroid hormone receptor, deiodinase, pressure overload, heart failure
Citation: Kerp H, Gassen J, Grund SC, Hönes GS, Dörr S, Mittag J, Härting N, Kaiser F, Moeller LC, Lorenz K and Führer D (2024) Cardiac recovery from pressure overload is not altered by thyroid hormone status in old mice. Front. Endocrinol. 15:1339741. doi: 10.3389/fendo.2024.1339741
Received: 16 November 2023; Accepted: 01 February 2024;
Published: 22 February 2024.
Edited by:
Patrick Osei-Owusu, Case Western Reserve University, United StatesReviewed by:
Soledad Bárez-López, Spanish National Research Council (CSIC), SpainConstantinos Pantos, National and Kapodistrian University of Athens, Greece
Copyright © 2024 Kerp, Gassen, Grund, Hönes, Dörr, Mittag, Härting, Kaiser, Moeller, Lorenz and Führer. This is an open-access article distributed under the terms of the Creative Commons Attribution License (CC BY). The use, distribution or reproduction in other forums is permitted, provided the original author(s) and the copyright owner(s) are credited and that the original publication in this journal is cited, in accordance with accepted academic practice. No use, distribution or reproduction is permitted which does not comply with these terms.
*Correspondence: Dagmar Führer, RGFnbWFyLmZ1ZWhyZXJAdWstZXNzZW4uZGU=; Kristina Lorenz, bG9yZW56QHRveGkudW5pLXd1ZXJ6YnVyZy5kZQ==
†These authors have contributed equally to this work and share last authorship