- 1Department of Paediatrics, Medical School, University of Pécs, Pécs, Hungary
- 2National Laboratory on Human Reproduction, University of Pécs, Pécs, Hungary
- 3Doctoral School of Health Sciences, Faculty of Health Sciences, University of Pécs, Pécs, Hungary
- 4Department of Obstetrics and Gynaecology, Medical School, University of Pécs, Pécs, Hungary
- 5Obstetrics and Gynecology, Magyar Imre Hospital Ajka, Ajka, Hungary
Growing evidence shows that maternal nutrition from preconception until lactation has an important effect on the development of non-communicable diseases in the offspring. Biological responses to environmental stress during pregnancy, including undernutrition or overnutrition of various nutrients, are transmitted in part by DNA methylation. The aim of the present narrative review is to summarize literature data on altered DNA methylation patterns caused by maternal macronutrient or vitamin intake and its association with offspring’s phenotype (obesity and related metabolic changes). With our literature search, we found evidence for the association between alterations in DNA methylation pattern of different genes caused by maternal under- or overnutrition of several nutrients (protein, fructose, fat, vitamin D, methyl-group donor nutrients) during 3 critical periods of programming (preconception, pregnancy, lactation) and the development of obesity or related metabolic changes (glucose, insulin, lipid, leptin, adiponectin levels, blood pressure, non-alcoholic fatty liver disease) in offspring. The review highlights that maternal consumption of several nutrients could individually affect the development of offspring’s obesity and related metabolic changes via alterations in DNA methylation.
1 Introduction
The prevalence of obesity has become a global epidemic worldwide, that affects people of all ages. In the European Region, almost 60% of adults and nearly one in three children are obese or overweight according to the report of the World Health Organization (1). Obesity-related non-communicable diseases (metabolic syndrome (MS), type 2 diabetes mellitus, and cardiovascular disease), are linked to increased mortality. Parallel with the increase of obesity, the prevalence of MS is also rising already in childhood and adolescence (2), therefore the investigation of underlying mechanisms behind their development is an emerging field.
Obesity occurs through different mechanisms, and it is an archetype of multifactorial diseases. According to the developmental origins of health and disease (DOHAD) hypothesis, inadequate nutritional exposures during the three critical periods (preconception, pregnancy, and lactation/early postnatal period) may play a critical role in determining the risk of obesity in the progeny (3, 4).
While the original DOHAD studies mainly concentrated on maternal undernutrition (where the consumption of all nutrients was insufficient at the same time), there is also growing evidence demonstrating an association between inadequate maternal intake of individual macro and micro-nutrients during the perinatal period and the development of obesity and related metabolic changes in the progeny (5). Both intrauterine malnutrition and overnutrition of different nutrients may represent a stress state for the embryo and may cause early programming that increases the risk of adult chronic disease development (6). Epigenetic mechanisms in the background of intrauterine programming have been proposed as one of the underlying effects (7). Gene expression is modulated by epigenetic mechanisms without altering the DNA sequences (7). DNA methylation, small non-coding microRNAs, and histone modifications are the main components of epigenetic mechanisms. One of the most studied epigenetic modifications is DNA methylation. DNA is methylated at the cytosine bases at the carbon-5′ position in cytosine-guanine dinucleotide residues. The methyl group addition affects the gene expression by (usually) inhibiting transcription. Alterations in the methylation of promoter regions of several genes are responsible for the plasticity of early programming. Changes in DNA methylation can occur throughout life, but much of the epigenome is established during embryogenesis and early development of the fetus (8). Shortly after fertilization both the paternal and maternal genomes are demethylated followed by de novo methylation just before implantation (9). These are critical periods in the growing embryo where nutritional factors may influence the methylome (9). Evidence mostly from animal models shows that changes in early postnatal nutrition quality and/or intake influence offspring’s epigenetic and expression profile in various organs including body weight regulation, suggesting the early postnatal period as another critical window of epigenetic regulations (10). During cell division, many epigenetic changes that occurred throughout the development (pre- and postnatally) survive, resulting in constant consequences of these marks on gene expression throughout life (11) that may predispose individuals to an altered metabolism resulting in the development of non-communicable diseases in later life (12).
In recent times, the number of animal and human studies investigating the epigenetic effect of maternal over- or undernutrition of several macro- and micronutrients on the development of obesity and related metabolic changes in the offspring has increased. In most of the previous investigations, the combined effect of several types of nutrients has been studied, which makes the interpretation difficult. Consequently, it is inevitable to investigate each macro- and micronutrient individually to better understand the exact molecular basis behind it. For some of the investigated nutrients, like nutrients involved in the one-carbon metabolism, the exact role in DNA methylation is clearly described, while for other nutrients (long-chain polyunsaturated fatty acids (LCPUFA), vitamin D, fructose) the clear mechanism of how the nutrients can alter DNA methylation pattern remains an important topic of research.
The purpose of this narrative literature review is to summarize the most recent data concerning alterations of offspring’s DNA methylation patterns caused by maternal macronutrient, vitamin or methyl-group donor intake during pregnancy leading to the development of obesity and related metabolic changes in the offspring.
2 Materials and methods
This narrative review specifically focuses on published studies investigating maternal individual nutrient intake before conception, during pregnancy, and lactation + alterations in DNA methylation pattern of offspring + measurements included obesity development and/or related metabolic changes (dyslipidemia, hyperglycemia, insulin resistance, hypertension, fatty liver disease) in offspring in the same study.
The literature search was conducted in PubMed, Medline, and the Cochrane Library in May 2023 using keywords: 1) maternal nutrition AND epigenetic AND protein, 2) maternal nutrition AND epigenetic AND fat, 3) maternal nutrition AND epigenetic AND carbohydrate, 4) maternal nutrition AND epigenetic AND vitamin, 5) maternal nutrition AND epigenetic AND folate.) with restriction of the languages (included articles published in English).Animal and human studies were included where the associations of the three following parameters were investigated together: 1. the DNA methylation pattern of at least one gene of the offspring; 2. data on at least one of the individual nutrient intakes of the mother during pregnancy; 3. at least one of the outcome measures included obesity development or related metabolic changes in the offspring. Those manuscripts not fulfilling the above-described criteria, or investigating in vitro models were excluded.
To summarize those investigations where the epigenetic effect of maternal starvation, maternal overnutrition or obesity and diabetes on the development of offspring obesity and related metabolic changes was described without a detailed description of the nutrients involved in the starvation/overnutrition is beyond the scope of this narrative review. The studies where the epigenetic effect of maternal intake of a combination of different nutritional groups was investigated were excluded from this narrative review (except for the methyl-group donor nutrients). A comprehensive review of the exact mechanisms of how individual nutrients affect epigenetic alterations is beyond the scope of this narrative review.
3 Results
3.1 Association between maternal carbohydrate overnutrition during pregnancy, DNA methylation pattern, obesity and related metabolic changes in the offspring
A current review summarized the role of maternal carbohydrate overnutrition on the early origin of MS in the offspring (13). The authors found an overall effect of the excess maternal carbohydrate intake on increased body weight and hepatic lipid content of the offspring (particularly in males). However, high sucrose supplementation led to favorable metabolic outcomes of female progeny in several rat models (13). The consumption of nutrients containing fructose has increased during pregnancy in recent decades, despite rising evidence about its numerous negative effects on offspring’s health (development of obesity, hepatic steatosis, dyslipidemia, insulin resistance, hypertension, and hypoadiponectinaemia) (14–19).
Results from studies suggest a role of maternal fructose intake (independent of other nutrients) on offspring’s DNA methylation pattern of different genes (20, 21) leading to the development of obesity and related metabolic changes in the offspring (results summarized in Table 1). Our literature research was not able to identify similar studies on the independent effect of other monosaccharides or di- and polysaccharides.
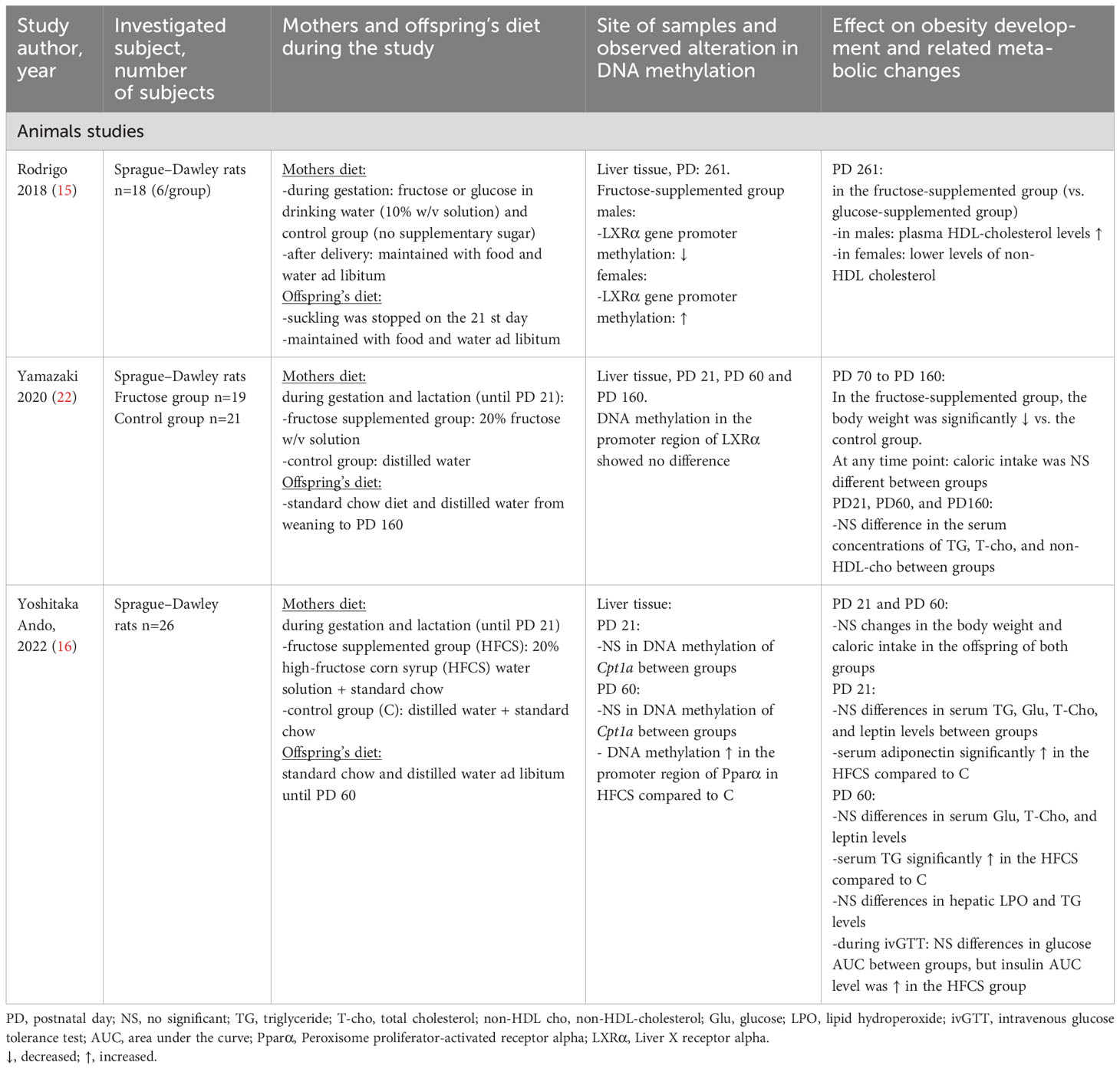
Table 1 Summary of studies investigating the maternal intake of carbohydrates during pregnancy on the development of offspring’s obesity and related metabolic changes via alteration in the DNA methylation pattern of different genes.
In a study, where pregnant rats were fed with a 20% fructose-containing water solution during gestation and lactation, the progeny developed hyperlipidemia and insulin resistance at 60 days of age (only male offspring were investigated in this study) (16). Furthermore, hepatic peroxisome proliferator-activated receptor α (Pparα) expression was suppressed by the increased DNA methylation of the promoter region compared to controls suggesting a role of Pparα hypermethylation caused by excessive fructose intake in the development of hyperlipidemia and insulin resistance in the progeny (16).
In another study, 10% w/v solution fructose or glucose was supplied to pregnant rats throughout gestation. Elevated plasma HDL-cholesterol levels were observed in the male progeny and lower levels of non-HDL cholesterol were presented in the female progeny from fructose-supplemented mothers compared to glucose-supplemented or control groups. Genes (Liver X-receptor, scavenger receptor B1, ATP-binding cassette (ABC)G5 and cholesterol 7-alpha hydroxylase gene) playing an important role in cholesterol metabolism showed decreased gene expression in males and increased in the female offspring from fructose-supplemented mothers. Furthermore, Liver X-receptorα gene (LXRα) promoter methylation elevated in males and decreased in the corresponding group of females from mothers fed fructose (15).
Yamazaki et al. investigated the epigenetic effect of maternal fructose supplementation (20% fructose w/v solution) on the lipid status of male rat offspring and found decreased serum HDL-cholesterol levels and decreased LXRα gene expression in the offspring’s liver tissue on the 160th postnatal day, however, in this study no differences in DNA methylation level of LXRα promoter region could be detected (22).
These studies in animals suggest that a high fructose diet during pregnancy is associated with decreased HDL-cholesterol levels (that may be gender-specific for females), hyperlipidemia, and insulin resistance through alterations in the methylation pattern of certain genes (LXRα and Pparα) playing an important role in the regulation of lipid homeostasis, the oxidation and transport of fatty acids. The above studies were conducted in animals, but in fact, the 10% w/v of fructose supplementation is similar to sugary beverages and the amount of calories originating from simple sugars (glucose, fructose) in the above investigations is very close to the energy intake of heavy consumers of sugary beverages in humans (23). Consequently, these studies may suggest an epigenetic role of maternal consumption of fructose containing beverages in the development of obesity and related metabolic changes in the offspring of humans as well.
3.2 Association between maternal protein intake during pregnancy, DNA methylation pattern, obesity, and related metabolic changes in the offspring
Animal studies show growing evidence that the progeny of mothers with low protein diet during pregnancy become more obese, hypertensive, and glucose intolerant as compared to the offspring of dams consuming standard diet (24–26). The offspring of mothers exposed to high protein consumption during pregnancy have higher body fat content and levels of signaling molecules associated with reduced insulin sensitivity as they age compared to progeny of control dams (the mother consuming a standard diet) (27). The results in humans are more conflicting, as inverse, direct, and null associations were also published between maternal intake of protein and obesity development in the offspring (28). Epigenetic mechanisms may stand behind these associations, as some amino acids (e.g., methionine, serine, glycine, and histidine) play an important role in the supply of methyl donors for the methylation of the DNA (29). Branched-chain amino acids stimulate insulin secretion, which may lead to insulin resistance and obesity (30, 31). Epigenetic studies provide evidence that inappropriate maternal protein intake can lead to alteration in DNA methylation in genes having a role in the regulation of growth, body composition, and metabolism (lipid, carbohydrate homeostasis, and blood pressure regulation) in the progeny (32–36), however, the number of investigations studying the effect of the alterations in DNA methylation induced by maternal protein malnutrition on the development of obesity or related metabolic changes in the offspring is very limited (Table 2). In a study, pregnant sows were divided into three diet groups during pregnancy: control group (CO), containing 12% crude protein (CP), low protein group (LP): containing 6% CP, and, high protein group (HP): containing 30% CP. The body weight of fetuses did not differ between CO, HP, and LP groups at gestational day 95. Significantly lower birth weight was observed in LP in comparison to CO pigs. At postnatal days 28 and 185 no differences in body weight were observed among CO, HP, and LP groups. Protein restriction had a significant effect on hepatic global methylation at gestational day 95 (38).
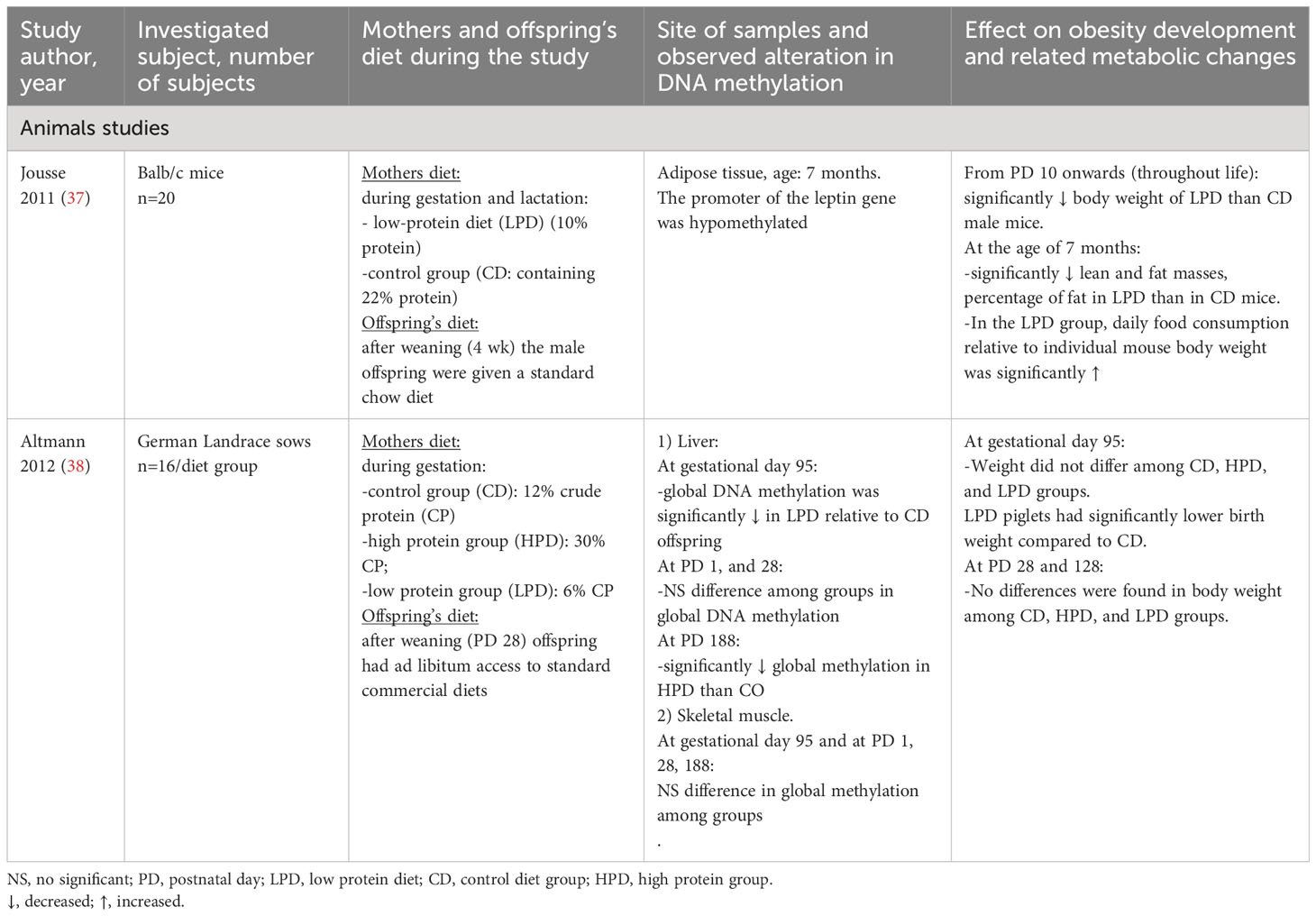
Table 2 Summary of studies investigating the maternal intake of protein during pregnancy on the development of offspring obesity and related metabolic changes via alteration in the DNA methylation pattern of different genes.
Higher food intake and lower body weight were observed in the mice offspring of mothers consuming a low-protein diet (LPD) (10% protein) compared to mice born to mothers consuming a control diet. Moreover, the removal of methyl-groups from the promoter region of the leptin gene was observed by the offspring (37).
The most investigated amino acid in relation to the development of epigenetically induced obesity is methionine, which plays a significant role in the one-carbon metabolism as a methyl-group donor for DNA methylation. The literature search could not identify publications where the association between methionine intake of the mother during pregnancy influenced the DNA methylation pattern of the offspring and as a consequence led to the development of obesity and related metabolic changes.
These animal studies suggest that a low-protein diet during pregnancy is associated with lower birth weight and the development of obesity and lower blood glucose concentration through alterations in the methylation pattern of certain genes (LEP, G6PC, and alteration in global hepatic DNA methylation) having an important role in the regulation of food intake, and glucose homeostasis (37, 38).
3.3 Association between maternal high-fat diet and LCPUFA intake during pregnancy, DNA methylation pattern, obesity, and related metabolic changes in the offspring
The development and growth of the offspring are affected by the dietary fat intake of the mother during pregnancy and lactation. The International Society for the Study of Fatty Acids and Lipids (ISSFAL) and the consensus statement for dietary fats have stated that the dietary fat consumption throughout gestation, as a proportion of energy intake, should not be different as that is recommended for the general population (39). Recent animal and human studies show that a maternal high-fat diet (HFD) has an effect on the general health of the offspring including the development of obesity, diabetes mellitus, and cardiovascular diseases (40–43). Some of these phenotypic differences caused by a maternal HFD have been associated with epigenetic changes through several pathways (44).
3.3.1 High-fat diet
In a study by Marco et al. the progeny of mother rats fed HFD (60% fat) during gestation had higher birth weights and ate more postnatally, leading to higher body weight at the age of 110 days compared to controls (45). Hypermethylation across the promoter region of the Pro-opiomelanocortin (POMC) was observed in the offspring from the HFD group (only female offspring were investigated).
In another study, researchers found elevated blood pressure (at 6 months and at 1 year), and increased levels of leptin (at 3 and 8 weeks, 6 months, and 1 year) in the offspring of pregnant rats exposed to HFD (HFD: 18.7% lard, 2.5% maltodextrin, 11.4% sucrose, 12% casein, 0.3% cholate, 1.3% cholesterol, and 52% standard chow) during pregnancy compared to chow-exposed offspring (the ratio of males to females offspring was 1:1 in every group). The HFD-exposed pups had a higher birth weight and higher body weight at 3 weeks of age compared to controls, but this observation no longer existed at 8 weeks after birth. In addition, an increased expression of the leptin gene and hypomethylation of the leptin promoter was found in the adipose tissues of the HFD-exposed progeny (46). In the same study, the authors found elevated blood pressure, serum leptin levels and significantly reduced overall DNA methylation of the leptin promoter in preschool-aged children born to women with hypertriglyceridemia (serum triglyceride > 3.28 mM) during gestation (46).
In a maternal HFD mouse model (containing 60 kcal% fat), decreased DNA methylation at the promoter of the leptin gene and hypermethylation at the promoter region of the leptin receptor and adiponectin genes with higher plasma levels of leptin and lower levels of adiponectin were reported in the adult offspring (results are presented with males and females merged). In contrast to the study of Lin et al. in this study, the authors could not observe differences in birthweight in the HFD pups compared to the low-fat diet controls, but in the post-natal period (week 6 of age), HFD offspring had higher body weight and consumed more calories compared to controls. Furthermore, HFD pups exhibited insulin resistance and hyperlipidemia (47).
Male offspring of maternal HF (60% fat) diet had rapid early weight gain, increased adiposity, and hyperleptinemia compared to offspring of low fat (10% fat) diet at 2 days and 3 weeks of life. The Pomc gene from the arcuate nucleus punches showed hypermethylation in the enhancer (nPE1 and nPE2) regions and in the promoter sequence mediating leptin effects (48).
In mice, maternal HFD (45% fat) had no significant effect on progeny’s body weight but was associated with hyperglycemia and glucose intolerance in male pups. The livers of offsprings exposed to a maternal HFD had hypomethylation in the mitogen-activated protein kinase 4 (Map2k4) gene and hypermethylation in the insulin receptor substrate 2 (Irs2) gene, which are involved in the insulin signaling pathway and the MAPK pathway (49). No difference in birth weight was observed in the progeny of mothers consuming HFD (45% fat) compared to controls, but HFD was associated with the development of non-alcoholic steatohepatitis and hypermethylation in genes implicated in lipid accumulation and liver fibrosis (Both male and female offspring were studied separately. However, since phenotypes of liver pathology were most prominent in males, only data from male offsprings were presented.) (50).
These studies in animals and humans suggest that HFD in pregnancy is associated with the development of offspring’s obesity and related metabolic changes through alterations in the methylation pattern of several genes (POMC, LEP, Map2k4, Irs2, Ephb2, Fgf21) having an important role in the regulation of energy balance, food intake, insulin resistance, and hypertension. However, drawing conclusions from these studies is difficult, as the time period of HFD of mothers varies (before pregnancy, during pregnancy, lactation period). A summary of the studies is presented in Table 3.
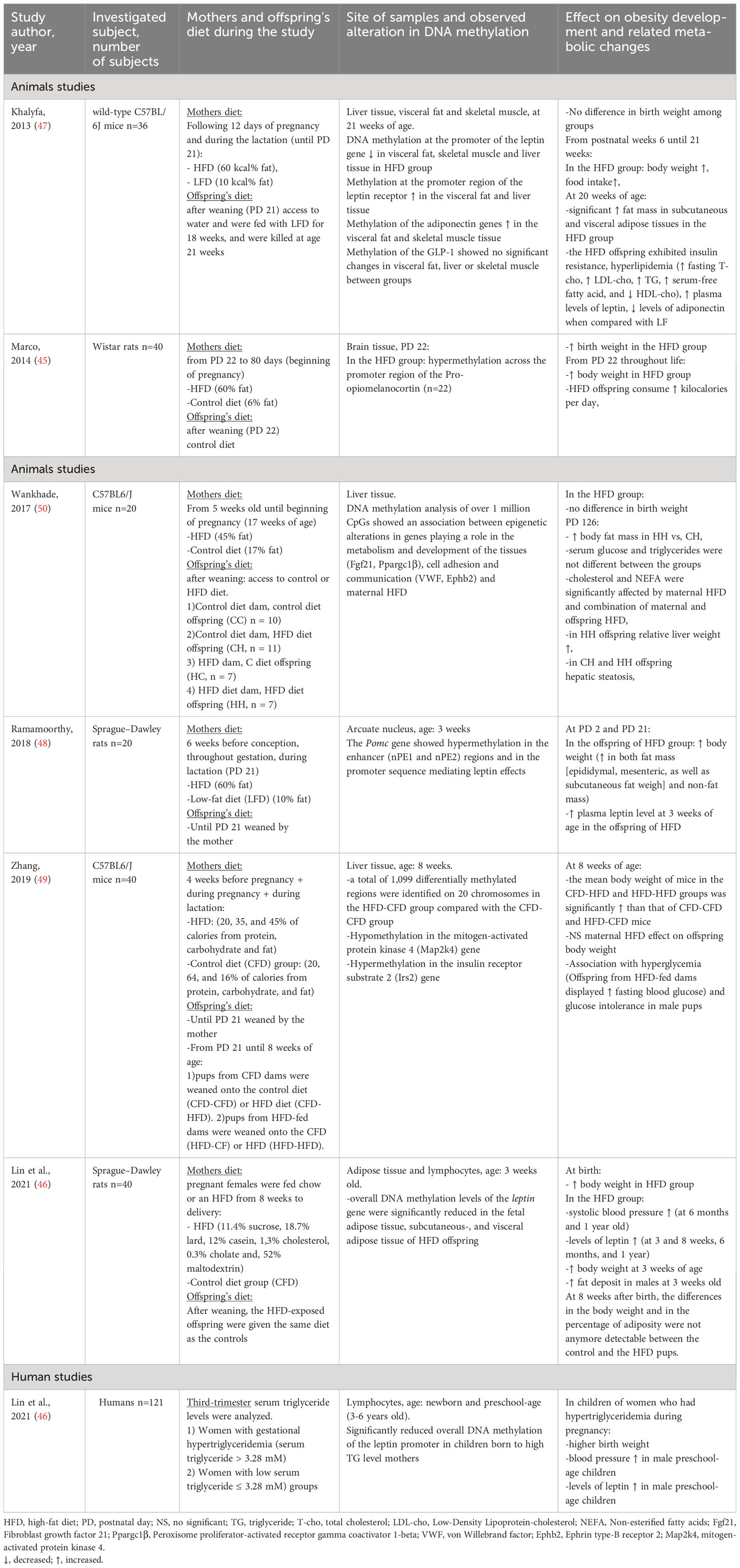
Table 3 Summary of studies investigating the maternal intake of fats during pregnancy on the development of offspring’s obesity and related metabolic changes via alteration in the DNA methylation pattern of different genes.
3.3.2 LCPUFA
Long-chain polyunsaturated fatty acids (LCPUFAs) including docosahexaenoic acid (DHA) and eicosapentaenoic acid (EPA) are essential in the development of the fetus. LCPUFAs on the n-3 and n-6 series are obtained by nutrition or produced by polyunsaturated fatty acid (PUFA) precursors. Since the DHA synthesis is reduced in the developing fetus, it must be obtained through the placenta from the mother (51). The ISSFAL and the consensus statement for dietary fats developed a recommendation for pregnant women only for the DHA intake: during pregnancy, it is recommended to consume at least 200 mg/day of DHA, which can be obtained by consuming one to two portions of sea fish (including oily fish) per week (39).
Previous studies of maternal supplementation with some LCPUFAs (Docosahexaenoic Acid, Arachidonic Acid, and Eicosapentaenoic Acid) have shown an association between a higher duration of pregnancy, reduced risk of preterm delivery, higher birth weight, and between brain development and visual function of the offspring (39, 52, 53). Until now, only a few studies have investigated the potential benefits of maternal n-3 LCPUFA intake in preventing offspring’s risk of obesity and related metabolic changes and the results are still conflicting (53). Many factors, including epigenetic mechanisms, may contribute to the observed effects. In recent times, some studies conducted in large, placebo-controlled, randomized trials reported an association between the maternal consumption of DHA and alteration in the offspring’s DNA methylation pattern in different genes, playing a significant role in appetite regulation or in the development of low birth weight, hypertension, diabetes, and cardiovascular diseases (54–56). However, the presence of obesity and related metabolic changes in the offspring was not investigated in the same study.
3.4 Association between maternal vitamin intake during pregnancy, DNA methylation pattern, obesity, and related metabolic changes in the offspring
Animal studies suggest that a higher multivitamin consumption during gestation increases body weight, food intake, and characteristics of the MS of the progeny (57–59). The number of studies investigating the role of altered DNA methylation patterns caused by maternal vitamin intake (especially the vitamins playing a role in the one-carbon metabolism and vitamin D) during pregnancy in the development of obesity and related metabolic changes has increased recently (58, 60) (Table 4).
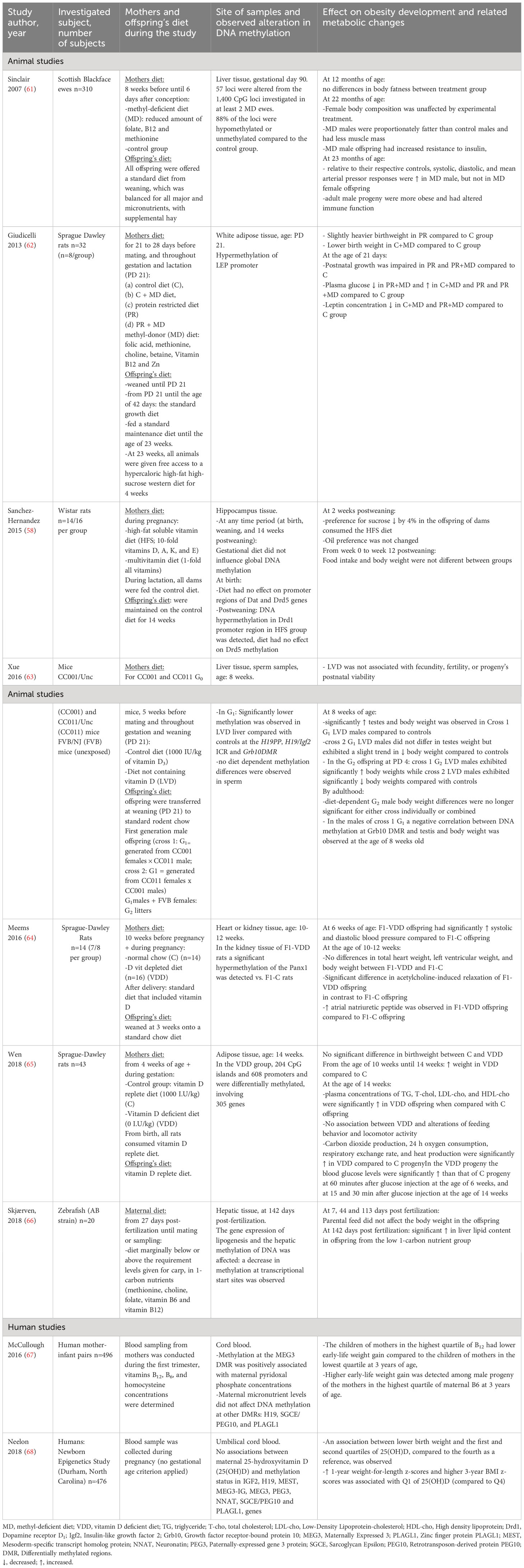
Table 4 Summary of studies investigating the maternal intake of vitamins and methyl-group donors during pregnancy on the development of offspring’s obesity and related metabolic changes via alteration in the DNA methylation pattern of different genes.
3.4.1 Multivitamin diet
Throughout gestation, Wistar rats consumed a high-fat soluble vitamin diet (HFS; 10-fold of D, A, K, and E) or a recommended multivitamin diet. In the offspring of rats fed HFS diet during pregnancy the sucrose preference decreased by 4%, but food intake and body weight were not affected. Gene expressions of the hypothalamic orexigenic neuropeptide Y (Npy) and anorexigenic POMC were increased in weaning and adult subjects. However, the gestational diet did not affect the global DNA methylation, a hypermethylation was detected in the promoter region of dopamine receptor 1 (Drd1) (only male offspring were investigated in this study) (58).
3.4.2 Vitamin D
Vitamin D plays an important role in different metabolic processes in the body. Few natural foods contain vitamin D (fatty fish species, fish liver oils, beef, and egg) (69). The skin is the major source of vitamin D in humans, where it is synthesized from its precursor (70). Studies showed relationships between maternal vitamin D status and glucose tolerance, preeclampsia, cesarean section, altered placental vascular pathology, reproductive failure, infection rates, adverse birth outcomes, abnormal fetal growth patterns, and the risk of preterm birth (71, 72). Furthermore, the impact of intrauterine vitamin D deficiency on later health outcomes like bone development, whole-body mineral content, long-term metabolic health - early postnatal overweight, differences in body fat mass, obesity, increased risk for insulin resistance and type 1 diabetes, hypertension -, and immunologically mediated diseases were also shown in several studies (71, 73, 74). The results in these fields are not consistent and are still under debate. Despite the increasing research data showing these associations between the health and development of the progeny in utero and in later life and the vitamin D status of the mother, the D vitamin consumption requirement of the mother during pregnancy, is still contradictory (71). Animal and human research show that vitamin D status of the mother throughout gestation may determine the progeny’s health via epigenetic modifications, since vitamin D may alter the expression of various genes, including the genes that indirectly or directly influence DNA methylation. Although, the mechanism behind this remains uncertain and is still under investigation (75).
Vitamin D deficiency of the mother in mice resulted in altered DNA methylation and body weight in two generations of the male progeny (63). Assayed loci in sperm and liver were mainly hypomethylated (63). A multigenerational diet-dependent effect was shown on body composition and body weight, although an effect on testis weight was detected only in the first generation.
In the offspring (10 male and 10 female) of vitamin D-depleted rats, increased systolic and diastolic blood pressure and the hypermethylation of the promoter region of the Pannexin-1 gene were observed (64).
An epigenome-wide DNA methylation study showed that the male progeny of vitamin D-deficient rats had altered methylation at 812 loci linked to 305 genes in the adipose tissue (65). 141 genes were differentially expressed of the methylated genes including 7 genes related to obesity or lipid metabolisms (Spon1, H19, Hif1a, Adrb2, Smad3, Zfp36, Vldlr). These transcriptional and epigenetic alterations were associated with obesity- and related metabolic changes in the progeny: from 10 weeks of age onwards vitamin D-deficient progeny had higher weight compared to controls. When compared with control offspring, total cholesterol, triglycerides, high-density lipoprotein, low-density lipoprotein, adipose tissue volume, and peak blood glucose were significantly increased but did not result in changes in locomotor activity (vertical, fine, and horizontal motor activity) and feeding behavior (food or water intake) in vitamin D-deficient offspring at the age of 14 weeks (in this study only male offspring were investigated) (65).
The Newborn Epigenetics Study (human) found an association between reduced plasma 25-hydroxyvitamin D of the mother and lower birth weight, higher weight-for-length at 1 year, and body mass index (BMI) z-scores at 3 years in offspring (female/male infants=51%/47%). But no association between midterm 25-hydroxyvitamin D levels and DNA methylation (in cord blood samples) of genes playing a role in fetal growth (MEST, IGF2, H19, NNAT, PEG3, SGCE/PEG10, PLAGL1, MEG3 and, MEG3-IG) was detected (68).
This current animal and human studies raise the possibility that vitamin D deficiency throughout gestation may play a significant effect in the development of obesity and related metabolic changes (dyslipidemia, hyperglycemia, and elevated blood pressure) in the offspring, which could be associated with changes in the methylation of different genes (H19/Igf2, Grb10, and Panx1) playing role in body weight and growth regulation and cell proliferation (63–65, 68).
3.4.3 Methyl-group donors playing role in one-carbon cycle metabolism
Growing evidence suggests that maternal intake of vitamins playing a role in the one-carbon metabolism (folate, folic acid, Vitamin B12, and Vitamin B6) can program obesity and the development of non-communicable chronic diseases in offspring (76). This may be mediated by epigenetic processes. For DNA methylation, one-carbon cycle metabolism nutrients are essential (76). As a methyl-group donor, folate is involved in the one-carbon cycle metabolism (76), it is converted to S-adenosylmethionine (SAM). For the methylation of the DNA, SAM will donate a methyl-group (77). Vitamin B12 and B6 are acting as co-enzymes for a source of methyl groups (76).
The deficiency of folate has been associated with numerous birth outcomes such as spontaneous abortion, preterm birth, low birth weight, and neural tube defects (78, 79). Humans cannot synthesize folate, they have to obtain it from dietary sources (beans, cereals, liver, egg yolks, and green leafy vegetables) (78). The WHO approved the recommendation in 2006, that for reproductive-aged women the daily intake of folic acid should be 400 ug from contemplating pregnancy until 12 weeks of gestation (80). No consistent agreement currently exists on the need to maintain this high folic acid intake during the entire pregnancy period.
Low maternal B12 levels have been associated with the development of offspring obesity, hypertension, alteration in lipid metabolism, insulin resistance, and metabolic syndrome (81–83). The natural sources of vitamin B12 are for example eggs, meat, milk, and seafood. No international consensus exists for the definition of B12 deficiency in pregnancy until now, except for a few agreements that it should be between 120 and 220 pmol/L (84).
Evidence for the association between the availability of methyl donors throughout pregnancy and the development of obesity and related metabolic changes in the progeny caused by alterations in DNA methylation pattern originates from investigations of the yellow Avy agouti mice. In yellow Avy agouti mice, maternal diets low in methyl donors (vitamin B12, folic acid, choline, betaine, and methionine) resulted in hypomethylation of the agouti gene (33) which predisposed the progeny to increased somatic growth, obesity, hyperinsulinemia, and diabetes (85). During critical stages of early embryonic development, an inadequate maternal one-carbon cycle metabolism nutrient intake has been related to DNA hypomethylation at the agouti locus (86), predisposing to adult obesity and diabetes (87).
In rats, a maternal methyl-group donor supplemented diet decreased the leptin concentration of the offspring and caused decreased methylation of the leptin gene promoter. Female and male offspring exhibited altered post-natal growth, but the long-term body weight gain increased only in males (62).
A study in zebrafish presented that a maternal one-carbon cycle metabolism nutrient-deficient diet (choline, methionine, B9, B12, and B6) affected the hepatic methylation of DNA and gene expression of lipogenesis resulting in the accumulation of lipids in the liver of the progeny (only male offspring were investigated) (66).
In sheep models, the restriction of folate, B12, and methionine during the conception stage of the mother resulted in elevated blood pressure and, increased insulin resistance in its (male and female) offspring (61). Furthermore, increased adiposity and male-specific demethylation of affected loci were detected in the adult male progeny (61).
In the human study of McCullough et al. (Newborn Epigenetics Study) the associations between maternal homocysteine concentrations, vitamins B12, and B6, on progeny’s birth weight and weight gain, and DNA methylation at 4 DMR-s (PLAGL1, SGCE/PEG10, MEG3, and H19) was investigated. The progeny (50% males) of the mothers in the lowest quartile of B12 had higher early-life weight gain in comparison to the progeny of the mothers in the highest quartile of B12. Furthermore, higher early-life weight gain was observed among the male progeny of the mothers in the highest quartile of maternal B6. MEG3 DMR methylation was positively associated with maternal B6 concentrations (67).
Recent studies showed an association between altered DNA methylation patterns of genes (most investigated genes: IGF2, H19, LINE-1, ZAC1, MEG3) and maternal intake of methyl-group donors on offspring birth weight (60). In these studies, the effect on progeny’s long-term health was not investigated, despite the well-described associations between adult chronic diseases and lower birth weight (88). The results suggest that folate deficiency of the mother during pregnancy may predispose the offspring to develop non-communicable diseases in later life. This could be partly caused by low fetal growth mediated by epigenetic DNA modification in certain genes.
In summary, animal and human studies suggest that methyl-group donor intake in the prenatal period and pregnancy and lactation is associated with the development of obesity and related metabolic changes (dyslipidemia, glucose homeostasis, insulin resistance, decreased leptin concentration, and elevated blood pressure) by changing the methylation pattern of several genes (POMC, LEP, Map2k4, Irs2, Ephb2, Fgf21) playing an important role in the regulation of energy balance, food intake, insulin resistance, and hypertension.
4 Discussion
Recently, several studies showed an association between different nutrient intake and epigenetic modifications that alter gene expression and may result in an increased susceptibility to develop obesity and related metabolic changes (reviewed by Milagro 2012 and by Cai et al., 2021) (89, 90). The joint effect of several types of nutrients is however difficult to interpret, therefore it is necessary to investigate each ingredient separately.
Maternal consumption of different nutrients throughout pregnancy could be associated with the development of obesity and related metabolic changes in the offspring via alteration in the DNA methylation pattern of different genes (Figure 1). The number of investigations where the individual nutrient intake of the mother during gestation (or gestation + lactation or gestation + just before gestation or gestation + just before gestation + lactation), the DNA methylation pattern of the offspring, and measurements included obesity development or related metabolic changes in the offspring’s later life were investigated in the same study are scarce. With our literature search, we found an association between alterations in the DNA methylation pattern of different genes caused by maternal under- or overnutrition of several nutrients (protein, fructose, fat, vitamin D, methyl-group donor nutrients) during the prenatal period, pregnancy, and lactation period and the development of obesity or related metabolic changes (glucose, insulin, lipid levels, blood pressure, non-alcoholic fatty liver disease) in the offspring. The summary of genes where altered DNA methylation was described in the offspring as a result of altered individual nutrient intake of the mother during pregnancy in the different tissues is presented in Figure 2.
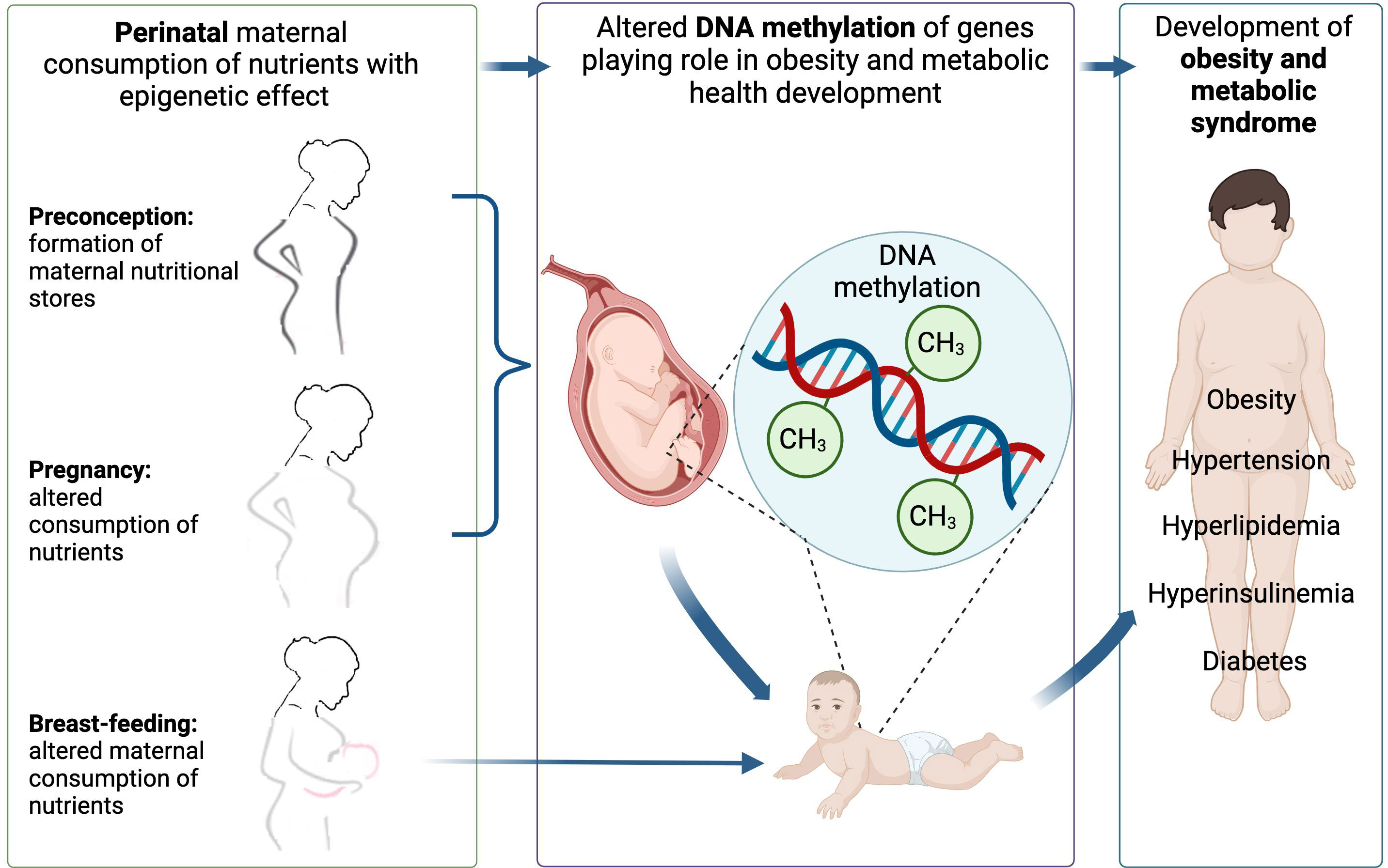
Figure 1 The association between perinatal maternal dietary factors and DNA methylation on the development of offspring obesity. Image was created with BioRender.com.
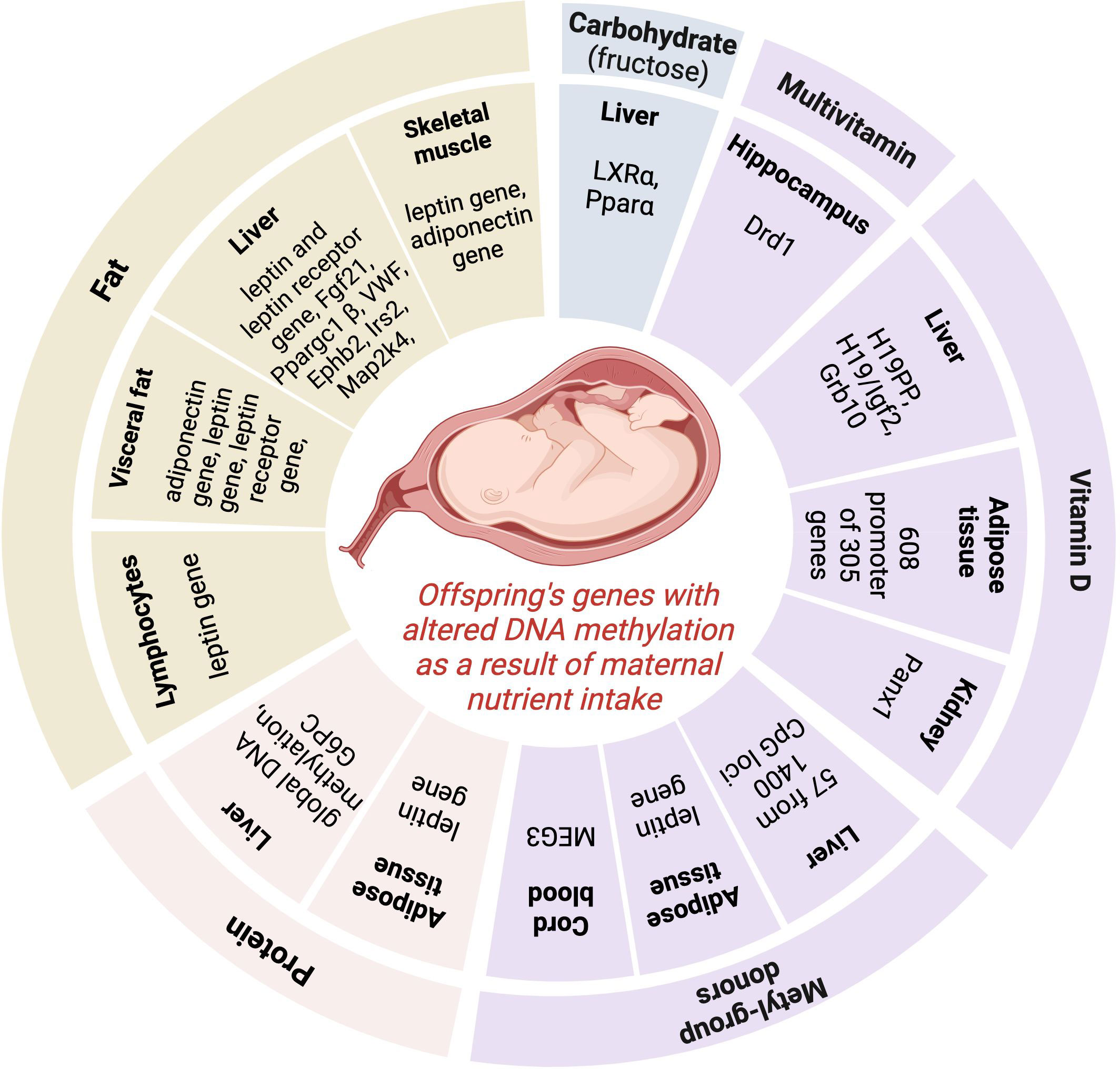
Figure 2 The summary of genes where altered DNA methylation was described in the offspring as a result of modified individual nutrient intake of the mother during pregnancy in the different tissues. Pparα, Peroxisome proliferator-activated receptor alpha; LXRα, Liver X receptor alpha; G6PC, glucose-6-phosphatase gene; Fgf21, Fibroblast growth factor 21; Ppargc1β, Peroxisome proliferator-activated receptor gamma coactivator 1-beta; VWF, von Willebrand factor; Ephb2, Ephrin type-B receptor 2; Irs2, Insulin Receptor Substrate 2; Map2k4, mitogen-activated protein kinase 4; Drd1, Dopamine receptor D1; Igf2, Insulin-like growth factor 2; Grb10, Growth factor receptor-bound protein 10; MEG3, Maternally Expressed 3. Image was created with BioRender.com.
We can see a big heterogeneity of identified genes (playing a role in different pathways of metabolism) having altered DNA methylation patterns in the offspring caused by changed individual nutrient intake of the mater during pregnancy. Only in the case of maternal fat intake can we detect a focus: the DNA alteration of the leptin gene was shown in several tissues, highlighting the role of this pathway in the epigenetic role of fat intake during pregnancy on the development of obesity in offspring. However, it is difficult to draw conclusions from these results and find emerging patterns of the epigenetic effect of each nutrient in obesity development because of several reasons: 1/the low number of complex investigations (nutrient intake during pregnancy + DNA methylation pattern of off-spring + measurements of obesity and/or related metabolic changes in the same study); 2/differences in DNA methylation assessment (investigation of global DNA methylation pattern or DNA methylation pattern of target genes); 3/the heterogeneity of cells studied (blood, hepatic cells, kidney cells, aorta cells, sperm, arcuate nucleus); 4/differences in the timing of the nutritional intervention (during gestation or just before gestation + gestation or just before gestation + gestation + lactation, or gestation + lactation or just before gestation); 5/difference in the gender of the offsprings included in the study (investigating only male- or only female offsprings or male + female offsprings together). Furthermore, evidence has shown that epigenetic gene regulation is rarely caused by 1 epigenetic modification alone; most regulation involves synergy among a variety of epigenetic modifications, in which histone and DNA methylation, are highly linked (90, 91). Synergies among different epigenetic modifications in the epigenetic regulation of early embryo development are summarized in the review of Cai et al. (90). As, in the present study, our aim was to summarize the results of those studies where the effect of alterations in DNA methylation caused by maternal nutrient intake was investigated, we can’t exclude that the results shown in our study are influenced by synergies among different epigenetic modifications.
Although an increasing body of evidence (using mainly in vitro models) highlights that individual nutrient availability, during the pre-implantation embryo development (the critical time period of the demethylation and remethylation), can trigger lasting epigenetic alterations in the offspring (92) The literature search was not able to detect (in utero) studies that investigated the complex association between alteration of DNA methylation pattern of the offspring caused by individual nutrient intake of the mother focusing exclusively on the pre-implantation period and the development of obesity or related metabolic changes in offspring’s later life. In studies investigating the associations between the individual carbohydrate and protein intake of the mother and alteration of the DNA methylation pattern of the offspring, the mothers were supplemented during whole gestation or whole gestation + lactation period. In the studies investigating the associations between the individual fat intake of the mother and DNA methylation pattern of the offspring, the timing of interventions was the following: 1/mothers were supplemented 58 days or 12 weeks before pregnancy, 2/two weeks before pregnancy + during pregnancy, 3/4 weeks before pregnancy+ pregnancy + lactation and 4/during whole gestation. Studies investigating the associations between the individual vitamin or methyl-group donor intake of the mother and alteration of DNA methylation pattern of the offspring the mothers were supplemented before pregnancy (5 weeks or 21-28 days) + during pregnancy + lactation or before pregnancy (8 weeks) until 6 days after conception or before pregnancy (4 weeks or 10 days) + during pregnancy. The above-described heterogeneity of the studies does not allow us to draw conclusions about the associations between the timing of nutrient intake of the mother and the resulting alterations in the DNA methylation pattern of the offspring.
Several other factors, for example, the epigenetic effect of the maternal conditions (e.g. maternal gut microbiome, obesity, diabetes) (93–97) or other maternal lifestyle factors (e.g. exposure to tobacco and alcohol, endocrine disruptors, stress) (93) could influence epigenetic changes and consequently, our results, that makes the interpretation of the findings even more difficult and emphasizes the importance of the multi-omics studies in this field.
The results were mainly drawn from animal experiments. Studies conducted in humans are very limited in this field and the follow-up of the examined offspring does not last into adulthood, which does not allow us to draw conclusions for later life.
The summary of the results of our narrative literature review demonstrates mounting evidence that the offspring’s DNA methylation pattern may be affected by the altered maternal intake of individual nutrients (fructose, fat, protein, vitamins, methyl-group donors) during pregnancy leading to the development of obesity and related metabolic changes in the offspring. For some of the investigated nutrients, like nutrients involved in the one-carbon metabolism, the exact role in DNA methylation is clearly described, while for other nutrients (LCPUFA, Vitamin D, fructose) the clear background of the mechanism of how the nutrients can alter DNA methylation pattern remains an important topic of investigation.
The main conclusion of the present review is that more studies are needed to better understand the associations between individual nutrient availability during pregnancy, alteration in DNA methylation, and the development of offspring’s obesity and related metabolic changes in later life.
Author contributions
SB: Conceptualization, Data curation, Investigation, Writing – original draft. IC: Investigation, Methodology, Visualization, Writing – original draft. RF: Data curation, Investigation, Visualization, Writing – original draft. RV: Investigation, Methodology, Writing – original draft. SF: Conceptualization, Investigation, Methodology, Writing – original draft. TE: Conceptualization, Supervision, Writing – original draft. DM: Conceptualization, Investigation, Methodology, Supervision, Writing – review & editing.
Funding
The author(s) declare financial support was received for the research, authorship, and/or publication of this article. Project no. RRF-2.3.1-21-2022-00012, titled National Laboratory on Human Reproduction has been implemented with the support provided by the Recovery and Resilience Facility of the European Union within the framework of Program Széchenyi Plan Plus. Project no. TKP2021-EGA-10” has been implemented with the support provided from the National Research, Development and Innovation Fund of Hungary, financed under the TKP2021-EGA” funding scheme.
Acknowledgments
We thank Edina Mendl for her administrative and technical support in the study.
Conflict of interest
The authors declare that the research was conducted in the absence of any commercial or financial relationships that could be construed as a potential conflict of interest.
Publisher’s note
All claims expressed in this article are solely those of the authors and do not necessarily represent those of their affiliated organizations, or those of the publisher, the editors and the reviewers. Any product that may be evaluated in this article, or claim that may be made by its manufacturer, is not guaranteed or endorsed by the publisher.
References
1. World Health Organization. Regional Office for Europe. WHO European Regional Obesity: Report 2022 (2022). Available at: https://www.who.int/europe/publications/i/item/9789289057738.
2. Bitew ZW, Alemu A, Tenaw Z, Alebel A, Worku T, Ayele EG. Prevalence of metabolic syndrome among children and adolescents in high-income countries: A systematic review and meta-analysis of observational studies. BioMed Res Int. (2021) 2021. doi: 10.1155/2021/6661457
3. Barker DJP. The origins of the developmental origins theory. J Intern Med. (2007) 261:412–7. doi: 10.1111/j.1365-2796.2007.01809.x
4. Lin Y-J. Metabolic syndrome in children and adolescents born premature and small-for-gestational age: A scenario of Developmental Origins of Health and Disease (DOHaD). Pediatr Neonatol. (2018) 59:109–10. doi: 10.1016/j.pedneo.2018.02.009
5. Koletzko B, Godfrey KM, Poston L, Szajewska H, van Goudoever JB, de Waard M, et al. Nutrition during pregnancy, lactation and early childhood and its implications for maternal and long-term child health: the early nutrition project recommendations. Ann Nutr Metab. (2019) 74:93–106. doi: 10.1159/000496471
6. Krishnaveni GV, Srinivasan K. Maternal nutrition and offspring stress response—Implications for future development of non-communicable disease: A perspective from India. Front Psychiatry. (2019) 10:795. doi: 10.3389/fpsyt.2019.00795
7. Lillycrop KA, Burdge GC. Maternal diet as a modifier of offspring epigenetics. J Dev Orig Health Dis. (2015) 6:88–95. doi: 10.1017/S2040174415000124
8. Reik W. Stability and flexibility of epigenetic gene regulation in mammalian development. Nature. (2007) 447:425–32. doi: 10.1038/nature05918
9. Faulk C, Dolinoy DC. Timing is everything. Epigenetics. (2011) 6:791–7. doi: 10.4161/epi.6.7.16209
10. Marousez L, Lesage J, Eberlé D. Epigenetics: linking early postnatal nutrition to obesity programming? Nutrients. (2019) 11:2966. doi: 10.3390/nu11122966
11. Guibert S, Forné T, Weber M. Global profiling of DNA methylation erasure in mouse primordial germ cells. Genome Res. (2012) 22:633–41. doi: 10.1101/gr.130997.111
12. Glier MB, Green TJ, Devlin AM. Methyl nutrients, DNA methylation, and cardiovascular disease. Mol Nutr Food Res. (2014) 58:172–82. doi: 10.1002/mnfr.201200636
13. Šeda O. Parental overnutrition by carbohydrates in developmental origins of metabolic syndrome. Physiol Res. (2021) 70:S585–96. doi: 10.33549/physiolres.934806
14. Jen V, Erler NS, Tielemans MJ, Braun KVE, Jaddoe VWV, Franco OH, et al. Mothers’ intake of sugar-containing beverages during pregnancy and body composition of their children during childhood: The Generation R Study. Am J Clin Nutr. (2017) 105:834–41. doi: 10.3945/ajcn.116.147934
15. Rodrigo S, Fauste E, de la Cuesta M, Rodríguez L, Álvarez-Millán JJ, Panadero MI, et al. Maternal fructose induces gender-dependent changes in both LXRα promoter methylation and cholesterol metabolism in progeny. J Nutr Biochem. (2018) 61:163–72. doi: 10.1016/j.jnutbio.2018.08.011
16. Ando Y, Yamada H, Munetsuna E, Yamazaki M, Kageyama I, Teshigawara A, et al. Maternal high-fructose corn syrup consumption causes insulin resistance and hyperlipidemia in offspring via DNA methylation of the Pparα promoter region. J Nutr Biochem. (2022) 103:108951. doi: 10.1016/j.jnutbio.2022.108951
17. Rodríguez L, Panadero MI, Rodrigo S, Roglans N, Otero P, Álvarez-Millán JJ, et al. Liquid fructose in pregnancy exacerbates fructose-induced dyslipidemia in adult female offspring. J Nutr Biochem. (2016) 32:115–22. doi: 10.1016/j.jnutbio.2016.02.013
18. Rodríguez L, Panadero MI, Roglans N, Otero P, Rodrigo S, Álvarez-Millán JJ, et al. Fructose only in pregnancy provokes hyperinsulinemia, hypoadiponectinemia, and impaired insulin signaling in adult male, but not female, progeny. Eur J Nutr. (2016) 55:665–74. doi: 10.1007/s00394-015-0886-1
19. Gugliucci A. Maternal fructose consumption can affect offspring metabolic outcomes. J Physiol. (2017) 595:4133–4. doi: 10.1113/JP274438
20. Munetsuna E, Yamazaki M, Ohashi K, Yamada H. Epigenetics and fructose metabolism: A new mechanism of fructose effects. In: Molecular Nutrition: Carbohydrates. Elsevier (2019). p. 353–68. doi: 10.1016/B978-0-12-849886-6.00010-0
21. DiStefano JK. Fructose-mediated effects on gene expression and epigenetic mechanisms associated with NAFLD pathogenesis. Cell Mol Life Sci. (2020) 77:2079–90. doi: 10.1007/s00018-019-03390-0
22. Yamazaki M, Munetsuna E, Yamada H, Ando Y, Mizuno G, Fujii R, et al. Maternal fructose consumption down-regulates Lxra expression via miR-206-mediated regulation. J Nutr Biochem. (2020) 82. doi: 10.1016/j.jnutbio.2020.108386
23. Rodrigo S, Rodríguez L, Otero P, Panadero MI, García A, Barbas C, et al. Fructose during pregnancy provokes fetal oxidative stress: The key role of the placental heme oxygenase-1. Mol Nutr Food Res. (2016) 60:2700–11. doi: 10.1002/mnfr.201600193
24. Chamson-Reig A, Thyssen SM, Hill DJ, Arany E. Exposure of the pregnant rat to low protein diet causes impaired glucose homeostasis in the young adult offspring by different mechanisms in males and females. Exp Biol Med. (2009) 234:1425–36. doi: 10.3181/0902-RM-69
25. Zambrano E, Bautista CJ, Deás M, Martínez-Samayoa PM, González-Zamorano M, Ledesma H, et al. A low maternal protein diet during pregnancy and lactation has sex- and window of exposure-specific effects on offspring growth and food intake, glucose metabolism and serum leptin in the rat. J Physiol. (2006) 571:221–30. doi: 10.1113/jphysiol.2005.100313
26. Mcmullen S, Langley-Evans SC. Maternal low-protein diet in rat pregnancy programs blood pressure through sex-specific mechanisms. Am J Physiol Regul Integr Comp Physiol. (2005) 288:85–90. doi: 10.1152/ajpregu.00435.2004.-Animal
27. Maurer AD, Reimer RA. Maternal consumption of high-prebiotic fibre or -protein diets during pregnancy and lactation differentially influences satiety hormones and expression of genes involved in glucose and lipid metabolism in offspring in rats. Br J Nutr. (2011) 105:329–38. doi: 10.1017/S0007114510003533
28. Maslova E, Hansen S, Grunnet LG, Strøm M, Bjerregaard AA, Hjort L, et al. Maternal protein intake in pregnancy and offspring metabolic health at age 9-16 y: results from a Danish cohort of gestational diabetes mellitus pregnancies and controls. Am J Clin Nutr. (2017) 106:623–59. doi: 10.3945/ajcn.115
29. Wang J, Wu Z, Li D, Li N, Dindot SV, Satterfield MC, et al. Nutrition, epigenetics, and metabolic syndrome. Antioxid Redox Signal. (2012) 17:282–301. doi: 10.1089/ars.2011.4381
30. Zhao J, Stewart ID, Baird D, Mason D, Wright J, Zheng J, et al. Causal effects of maternal circulating amino acids on offspring birthweight: a Mendelian randomisation study. EBioMedicine. (2023) 88:104441. doi: 10.1016/j.ebiom.2023.104441
31. Lu J, Gu Y, Liu H, Wang L, Li W, Li W, et al. Daily branched-chain amino acid intake and risks of obesity and insulin resistance in children: A cross-sectional study. Obesity. (2020) 28:1310–6. doi: 10.1002/oby.22834
32. Lillycrop KA, Phillips ES, Jackson AA, Hanson MA, Burdge GC. Dietary protein restriction of pregnant rats induces and folic acid supplementation prevents epigenetic modification of hepatic gene expression in the offspring. J Nutr. (2005) 135:1382–6. doi: 10.1093/jn/135.6.1382
33. Wolff GL, Kodell RL, Moore SR, Cooney CA. Maternal epigenetics and methyl supplements affect agouti gene expression in A vy /a mice. FASEB J. (1998) 12:949–57. doi: 10.1096/fasebj.12.11.949
34. Lan X, Cretney EC, Kropp J, Khateeb K, Berg MA, Peñagaricano F, et al. Maternal diet during pregnancy induces gene expression and DNA methylation changes in fetal tissues in sheep. Front Genet. (2013) 4:49. doi: 10.3389/fgene.2013.00049
35. Gong L, Pan YX, Chen H. Gestational low protein diet in the rat mediates Igf2 gene expression in male offspring via altered hepatic DNA methylation. Epigenetics. (2010) 5:619–26. doi: 10.4161/epi.5.7.12882
36. Reamon-Buettner SM, Buschmann J, Lewin G. Identifying placental epigenetic alterations in an intrauterine growth restriction (IUGR) rat model induced by gestational protein deficiency. Reprod Toxicol. (2014) 45:117–24. doi: 10.1016/j.reprotox.2014.02.009
37. Jousse C, Parry L, Lambert-Langlais S, Maurin A, Averous J, Bruhat A, et al. Perinatal undernutrition affects the methylation and expression of the leptin gene in adults: implication for the understanding of metabolic syndrome. FASEB J. (2011) 25:3271–8. doi: 10.1096/fj.11-181792
38. Altmann S, Murani E, Schwerin M, Metges CC, Wimmers K, Ponsuksili S. Maternal dietary protein restriction and excess affects offspring gene expression and methylation of non-SMC subunits of condensin i in liver and skeletal muscle. Epigenetics. (2012) 7:239–52. doi: 10.4161/epi.7.3.19183
39. Koletzko B, Cetin I, Thomas Brenna J. Dietary fat intakes for pregnant and lactating women. Br J Nutr. (2007) 98:873–7. doi: 10.1017/S0007114507764747
40. Gawlińska K, Gawliński D, Filip M, Przegaliński E. Relationship of maternal high-fat diet during pregnancy and lactation to offspring health. Nutr Rev. (2021) 79:709–25. doi: 10.1093/nutrit/nuaa020
41. Ainge H, Thompson C, Ozanne SE, Rooney KB. A systematic review on animal models of maternal high fat feeding and offspring glycaemic control. Int J Obes. (2011) 35:325–35. doi: 10.1038/ijo.2010.149
42. Prasad M, Rajagopal P, Devarajan N, Veeraraghavan VP, Palanisamy CP, Cui B, et al. A comprehensive review on high -fat diet-induced diabetes mellitus: an epigenetic view. J Nutr Biochem. (2022) 107:109037. doi: 10.1016/j.jnutbio.2022.109037
43. Gademan MGJ, Vermeulen M, Oostvogels AJJM, Roseboom TJ, Visscher TLS, van Eijsden M, et al. Maternal Prepregancy BMI and Lipid Profile during Early Pregnancy Are Independently Associated with Offspring’s Body Composition at Age 5–6 Years: The ABCD Study. PloS One. (2014) 9:e94594. doi: 10.1371/journal.pone.0094594
44. Harmancıoğlu B, Kabaran S. Maternal high fat diets: impacts on offspring obesity and epigenetic hypothalamic programming. Front Genet. (2023) 14:1158089. doi: 10.3389/fgene.2023.1158089
45. Marco A, Kisliouk T, Tabachnik T, Meiri N, Weller A. Overweight and CpG methylation of the Pomc promoter in offspring of high-fat-diet-fed dams are not “reprogrammed” by regular chow diet in rats. FASEB J. (2014) 28:4148–57. doi: 10.1096/fj.14-255620
46. Lin XH, Gao L, Tian S, Klausen C, Guo MX, Gao Q, et al. Maternal high-fat-diet exposure is associated with elevated blood pressure and sustained increased leptin levels through epigenetic memory in offspring. Sci Rep. (2021) 11. doi: 10.1038/s41598-020-79604-4
47. Khalyfa A, Carreras A, Hakim F, Cunningham JM, Wang Y, Gozal D. Effects of late gestational high-fat diet on body weight, metabolic regulation and adipokine expression in offspring. Int J Obes. (2013) 37:1481–9. doi: 10.1038/ijo.2013.12
48. Gali Ramamoorthy T, Allen T-J, Davies A, Harno E, Sefton C, Murgatroyd C, et al. Maternal overnutrition programs epigenetic changes in the regulatory regions of hypothalamic Pomc in the offspring of rats. Int J Obes. (2018) 42:1431–44. doi: 10.1038/s41366-018-0094-1
49. Zhang Q, Xiao X, Zheng J, Li M, Yu M, Ping F, et al. A maternal high-fat diet induces DNA methylation changes that contribute to glucose intolerance in offspring. Front Endocrinol (Lausanne). (2019) 10:871. doi: 10.3389/fendo.2019.00871
50. Wankhade UD, Zhong Y, Kang P, Alfaro M, Chintapalli SV, Thakali KM, et al. Enhanced offspring predisposition to steatohepatitis with maternal high-fat diet is associated with epigenetic and microbiome alterations. PloS One. (2017) 12. doi: 10.1371/journal.pone.0175675
51. Innis SM. Dietary omega 3 fatty acids and the developing brain. Brain Res. (2008) 1237:35–43. doi: 10.1016/j.brainres.2008.08.078
52. Carlson SE, Colombo J. Docosahexaenoic acid and arachidonic acid nutrition in early development. Adv Pediatr. (2016) 63:453–71. doi: 10.1016/j.yapd.2016.04.011
53. Amatruda M, Ippolito G, Vizzuso S, Vizzari G, Banderali G, Verduci E. Epigenetic effects of n-3 LCPUFAs: A role in pediatric metabolic syndrome. Int J Mol Sci. (2019) 20:2118. doi: 10.3390/ijms20092118
54. Lee HS, Barraza-Villarreal A, Biessy C, Duarte-Salles T, Sly PD, Ramakrishnan U, et al. Dietary supplementation with polyunsaturated fatty acid during pregnancy modulates DNA methylation at IGF2/H19 imprinted genes and growth of infants. Physiol Genomics. (2014) 46:851–7. doi: 10.1152/physiolgenomics.00061.2014
55. van Dijk SJ, Zhou J, Peters TJ, Buckley M, Sutcliffe B, Oytam Y, et al. Effect of prenatal DHA supplementation on the infant epigenome: results from a randomized controlled trial. Clin Epigenet. (2016) 8. doi: 10.1186/s13148-016-0281-7
56. Bianchi M, Alisi A, Fabrizi M, Vallone C, Ravà L, Giannico R, et al. Maternal intake of n-3 polyunsaturated fatty acids during pregnancy is associated with differential methylation profiles in cord blood white cells. Front Genet. (2019) 10:1050. doi: 10.3389/fgene.2019.01050
57. Cho CE, Sánchez-Hernández D, Reza-López SA, Huot PSP, Kim YI, Anderson GH. Obesogenic phenotype of offspring of dams fed a high multivitamin diet is prevented by a post-weaning high multivitamin or high folate diet. Int J Obes. (2013) 37:1177–82. doi: 10.1038/ijo.2012.210
58. Sanchez-Hernandez D, Poon AN, Kubant R, Kim H, Huot PSP, Cho CE, et al. A gestational diet high in fat-soluble vitamins alters expression of genes in brain pathways and reduces sucrose preference, but not food intake, in wistar male rat offspring. Appl Physiology Nutr Metab. (2015) 40:424–31. doi: 10.1139/apnm-2014-0480
59. Szeto IMY, Aziz A, Das PJ, Taha AY, Okubo N, Reza-Lopez S, et al. High multivitamin intake by Wistar rats during pregnancy results in increased food intake and components of the metabolic syndrome in male offspring. Am J Physiology-Regulatory Integr Comp Physiol. (2008) 295:R575–82. doi: 10.1152/ajpregu.90354.2008
60. Bokor S, Vass RA, Funke S, Ertl T, Molnár D. Epigenetic effect of maternal methyl-group donor intake on offspring’s health and disease. Life. (2022) 12. doi: 10.3390/life12050609
61. Sinclair KD, Allegrucci C, Singh R, Gardner DS, Sebastian S, Bispham J, et al. DNA methylation, insulin resistance, and blood pressure in offspring determined by maternal periconceptional B vitamin and methionine status. Proc Natl Acad Sci. (2007) 104:19351–6. doi: 10.1073/pnas.0707258104
62. Giudicelli F, Brabant AL, Grit I, Parnet P, Amarger V. Excess of methyl donor in the perinatal period reduces postnatal leptin secretion in rat and interacts with the effect of protein content in diet. PloS One. (2013) 8. doi: 10.1371/journal.pone.0068268
63. Xue J, Schoenrock SA, Valdar W, Tarantino LM, Ideraabdullah FY. Maternal vitamin D depletion alters DNA methylation at imprinted loci in multiple generations. Clin Epigenet. (2016) 8. doi: 10.1186/s13148-016-0276-4
64. Meems LMG, Mahmud H, Buikema H, Tost J, Michel S, Takens J, et al. Parental vitamin D deficiency during pregnancy is associated with increased blood pressure in offspring via Panx1 hypermethylation. Am J Physiol Heart Circ Physiol. (2016) 311:1459–69. doi: 10.1152/ajpheart.00141.2016.-Vitamin
65. Wen J, Hong Q, Wang X, Zhu L, Wu T, Xu P, et al. The effect of maternal vitamin D deficiency during pregnancy on body fat and adipogenesis in rat offspring. Sci Rep. (2018) 8:365. doi: 10.1038/s41598-017-18770-4
66. Skjærven KH, Jakt LM, Fernandes JMO, Dahl JA, Adam AC, Klughammer J, et al. Parental micronutrient deficiency distorts liver DNA methylation and expression of lipid genes associated with a fatty-liver-like phenotype in offspring. Sci Rep. (2018) 8. doi: 10.1038/s41598-018-21211-5
67. McCullough LE, Miller EE, Mendez MA, Murtha AP, Murphy SK, Hoyo C. Maternal B vitamins: effects on offspring weight and DNA methylation at genomically imprinted domains. Clin Epigenet. (2016) 8:1–11. doi: 10.1186/s13148-016-0174-9
68. Benjamin Neelon SE, White AJ, Vidal AC, Schildkraut JM, Murtha AP, Murphy SK, et al. Maternal Vitamin D, DNA methylation at imprint regulatory regions and offspring weight at birth, 1 year and 3 years. Int J Obes. (2018) 42:587–93. doi: 10.1038/ijo.2017.160
69. Christakos S, Dhawan P, Verstuyf A, Verlinden L, Carmeliet G. Vitamin D: metabolism, molecular mechanism of action, and pleiotropic effects. Physiol Rev. (2016) 96:365–408. doi: 10.1152/physrev.00014.2015.-1,25-Dihydrox
70. Holick MF, Chen TC, Lu Z, Sauter E. Vitamin D and skin physiology: A D-lightful story. J Bone Mineral Res. (2007) 22:V28–33. doi: 10.1359/jbmr.07s211
71. Hollis BW, Wagner CL. Vitamin D supplementation during pregnancy: Improvements in birth outcomes and complications through direct genomic alteration. Mol Cell Endocrinol. (2017) 453:113–30. doi: 10.1016/j.mce.2017.01.039
72. von Websky K, Hasan AA, Reichetzeder C, Tsuprykov O, Hocher B. Impact of vitamin D on pregnancy-related disorders and on offspring outcome. J Steroid Biochem Mol Biol. (2018) 180:51–64. doi: 10.1016/j.jsbmb.2017.11.008
73. Holick MF. High prevalence of vitamin D inadequacy and implications for health. Mayo Clin Proc. (2006) 81:353–73. doi: 10.4065/81.3.353
74. Ideraabdullah FY, Belenchia AM, Rosenfeld CS, Kullman SW, Knuth M, Mahapatra D, et al. Maternal vitamin D deficiency and developmental origins of health and disease (DOHaD). J Endocrinol. (2019) 241:R65–80. doi: 10.1530/JOE-18-0541
75. Ong LTC, Booth DR, Parnell GP. Vitamin D and its effects on DNA methylation in development, aging, and disease. Mol Nutr Food Res. (2020) 64:2000437. doi: 10.1002/mnfr.202000437
76. Rush EC, Katre P, Yajnik CS. Vitamin B12: one carbon metabolism, fetal growth and programming for chronic disease. Eur J Clin Nutr. (2014) 68:2–7. doi: 10.1038/ejcn.2013.232
77. Anderson OS, Sant KE, Dolinoy DC. Nutrition and epigenetics: an interplay of dietary methyl donors, one-carbon metabolism and DNA methylation. J Nutr Biochem. (2012) 23:853–9. doi: 10.1016/j.jnutbio.2012.03.003
78. Fekete K, Berti C, Cetin I, Hermoso M, Koletzko BV, Decsi T. Perinatal folate supply: Relevance in health outcome parameters. Matern Child Nutr. (2010) 6:23–38. doi: 10.1111/j.1740-8709.2010.00261.x
79. Tamura T, Picciano MF. Folate and human reproduction1–3. Am J Clin Nutr. (2006) 83:993–1016. doi: 10.1093/ajcn/83.5.993
80. World Health Organization. Guideline: optimal serum and red blood cell folate concentrations in women of reproductive age for prevention of neural tube defects (2015). World Health Organization. Available online at: https://apps.who.int/iris/handle/10665/161988 (Accessed April 25, 2023).
81. Kumar KA, Lalitha A, Pavithra D, Padmavathi IJN, Ganeshan M, Rao KR, et al. Maternal dietary folate and/or vitamin B12 restrictions alter body composition (adiposity) and lipid metabolism in Wistar rat offspring. J Nutr Biochem. (2013) 24:25–31. doi: 10.1016/j.jnutbio.2012.01.004
82. Ghosh S, Sinha JK, Putcha UK, Raghunath M. Severe but not moderate vitamin B12 deficiency impairs lipid profile, induces adiposity, and leads to adverse gestational outcome in female C57BL/6 mice. Front Nutr. (2016) 3:1. doi: 10.3389/fnut.2016.00001
83. Yajnik CS, Deshpande SS, Jackson AA, Refsum H, Rao S, Fisher DJ, et al. Vitamin B12 and folate concentrations during pregnancy and insulin resistance in the offspring: The Pune Maternal Nutrition Study. Diabetologia. (2008) 51:29–38. doi: 10.1007/s00125-007-0793-y
84. Sukumar N, Adaikalakoteswari A, Venkataraman H, Maheswaran H, Saravanan P. Vitamin B12 status in women of childbearing age in the UK and its relationship with national nutrient intake guidelines: results from two National Diet and Nutrition Surveys. BMJ Open. (2016) 6. doi: 10.1136/BMJOPEN-2016-011247
85. Miltenberger RJ, Mynatt RL, Wilkinson JE, Woychik RP. The role of the agouti gene in the yellow obese syndrome. J Nutr. (1997) 127:1902S–7S. doi: 10.1093/jn/127.9.1902S
86. Waterland RA. Do maternal methyl supplements in mice affect DNA methylation of offspring? J Nutr. (2003) 133:238. doi: 10.1093/jn/133.1.238
87. Fall C. Maternal nutrition: effects on health in the next generation. Indian J Med Res. (2009) 130:593–9.
88. de Mendonça ELSS, de Lima Macêna M, Bueno NB, de Oliveira ACM, Mello CS. Premature birth, low birth weight, small for gestational age and chronic non-communicable diseases in adult life: A systematic review with meta-analysis. Early Hum Dev. (2020) 149:105154. doi: 10.1016/j.earlhumdev.2020.105154
89. Milagro FI, Mansego ML, De Miguel C, Martínez JA. Dietary factors, epigenetic modifications and obesity outcomes: Progresses and perspectives. Mol Aspects Med. (2013) 34:782–812. doi: 10.1016/j.mam.2012.06.010
90. Cai S, Quan S, Yang G, Chen M, Ye Q, Wang G, et al. Nutritional status impacts epigenetic regulation in early embryo development: A scoping review. Adv Nutr. (2021) 12:1877–92. doi: 10.1093/advances/nmab038
91. Delcuve GP, Rastegar M, Davie JR. Epigenetic control. J Cell Physiol. (2009) 219:243–50. doi: 10.1002/jcp.21678
92. Breton-Larrivée M, Elder E, McGraw S. DNA methylation, environmental exposures and early embryo development. Anim Reprod. (2019) 16:465–74. doi: 10.21451/1984-3143-AR2019-0062
93. Barua S, Junaid MA. Lifestyle, pregnancy and epigenetic effects. Epigenomics. (2015) 7:85–102. doi: 10.2217/epi.14.71
94. Sikder MAA, Rashid RB, Ahmed T, Sebina I, Howard DR, Ullah MA, et al. Maternal diet modulates the infant microbiome and intestinal Flt3L necessary for dendritic cell development and immunity to respiratory infection. Immunity. (2023) 56:1098–1114.e10. doi: 10.1016/j.immuni.2023.03.002
95. Radford-Smith DE, Anthony DC. Mechanisms of maternal diet-induced obesity affecting the offspring brain and development of affective disorders. Metabolites. (2023) 13:455. doi: 10.3390/metabo13030455
96. Gomez-Arango LF, Barrett HL, McIntyre HD, Callaway LK, Morrison M, Nitert MD. Contributions of the maternal oral and gut microbiome to placental microbial colonization in overweight and obese pregnant women. Sci Rep. (2017) 7:2860. doi: 10.1038/s41598-017-03066-4
Keywords: obesity, metabolic syndrome, maternal, nutrition, epigenetic, DNA methylation
Citation: Bokor S, Csölle I, Felső R, Vass RA, Funke S, Ertl T and Molnár D (2024) Dietary nutrients during gestation cause obesity and related metabolic changes by altering DNA methylation in the offspring. Front. Endocrinol. 15:1287255. doi: 10.3389/fendo.2024.1287255
Received: 01 September 2023; Accepted: 01 February 2024;
Published: 20 February 2024.
Edited by:
Amanda Brandon, The University of Sydney, AustraliaReviewed by:
Deanne Helena Hryciw, Griffith University, AustraliaKeshari Thakali, University of Arkansas for Medical Sciences, United States
Copyright © 2024 Bokor, Csölle, Felső, Vass, Funke, Ertl and Molnár. This is an open-access article distributed under the terms of the Creative Commons Attribution License (CC BY). The use, distribution or reproduction in other forums is permitted, provided the original author(s) and the copyright owner(s) are credited and that the original publication in this journal is cited, in accordance with accepted academic practice. No use, distribution or reproduction is permitted which does not comply with these terms.
*Correspondence: Szilvia Bokor, Ym9rb3Iuc3ppbHZpYUBwdGUuaHU=