- 1Department of Psychiatry & Behavioral Sciences, The Johns Hopkins University School of Medicine, Baltimore, MD, United States
- 2Department of Neurology, The Johns Hopkins University School of Medicine, Baltimore, MD, United States
- 3Department of Biostatistics, The Johns Hopkins Bloomberg School of Public Health, Baltimore, MD, United States
Background: Alzheimer’s disease (AD) is increasing in prevalence, but effective treatments for its cognitive impairment remain severely limited. This study investigates the impact of ketone body production through dietary manipulation on memory in persons with mild cognitive impairment due to early AD and explores potential mechanisms of action.
Methods: We conducted a 12-week, parallel-group, controlled feasibility trial of a ketogenic diet, the modified Atkins diet (MAD), compared to a control diet in patients with cognitive impairments attributed to AD. We administered neuropsychological assessments, including memory tests, and collected blood samples at baseline and after 12 weeks of intervention. We performed untargeted lipidomic and targeted metabolomic analyses on plasma samples to detect changes over time.
Results: A total of 839 individuals were screened to yield 38 randomized participants, with 20 assigned to receive MAD and 18 assigned to receive a control diet. Due to attrition, only 13 in the MAD arm and nine in the control arm were assessed for the primary endpoint, with two participants meeting ketosis levels used to define MAD adherence criteria. The average change from baseline in the Memory Composite Score was 1.37 (95% CI: −0.87, 4.90) points higher in the MAD group compared to the control group. The effect size of the intervention on baseline MAD change was moderate (Cohen’s D = 0.57, 95% CI: −0.67, 1.33). In the 15 participants (nine MAD, six control) assessed for lipidomic and metabolomic-lipidomics and metabolomics, 13 metabolites and 10 lipids showed significant changes from baseline to 12 weeks, including triacylglycerols (TAGs, 50:5, 52:5, and 52:6), sphingomyelins (SM, 44:3, 46:0, 46:3, and 48:1), acetoacetate, fatty acylcarnitines, glycerol-3-phosphate, and hydroxy fatty acids.
Conclusions: Attrition was greatest between baseline and week 6. All participants retained at week 6 completed the study. Despite low rates of adherence by criteria defined a priori, lipidomic and metabolomic analyses indicate significant changes from baseline in circulating lipids and metabolites between MAD and control participants at 12-week postrandomization, and MAD participants showed greater, albeit nonsignificant, improvement in memory.
1 Introduction
The prevalence of Alzheimer’s disease (AD) is increasing as the aging population grows. In addition to those with dementia, there are about five million persons in the USA with mild cognitive impairment (MCI), likely due to AD (1). Advances in drug therapies for AD have been made (2–4), but concerns have been raised regarding their safety, cost, and translation to clinical outcomes (5, 6). Alternative approaches for the treatment and prevention of AD are needed. Studies examining the influence of dietary changes on cognition and/or functioning in AD have produced promising results (7–9).
The role of lipid modulation in the pathogenesis of AD is under investigation. High serum cholesterol is associated with an increased risk of developing AD (10–17), and older persons who consume a low-cholesterol Mediterranean diet have a lower risk of developing AD (18–20). In mouse models of AD, a high-fat and high-cholesterol diet increases the production of amyloid-β, worsens synaptic damage, and further impairs learning and memory compared with mice fed standard diets (21, 22). As such, the Dietary Approaches to Stop Hypertension (DASH) and Mediterranean-DASH Intervention for Neurodegenerative Delay (MIND) diets have been among the most popular recommended diets for aging people (23, 24).
Ketone bodies produced by the metabolism of fatty acids in the liver are used by cells throughout the body, including the brain, as a source of energy (25). AD brains can metabolize ketone bodies even when glucose metabolism is impaired (26, 27). Given the clearly impaired glucose metabolism in AD (25, 28–34), increased attention has been given to the use of ketogenic diets as treatments for AD.
The ketogenic diet has proven to be an efficacious intervention for drug-resistant epilepsy but requires precise measurement of all macronutrient intake and strict adherence (35–37). Fortunately, ketosis can be induced using a more forgiving diet that is high in fat and low in carbohydrates, such as the modified Atkins diet (MAD), which is also effective in reducing epileptic seizures (35, 38, 39). The MAD is now being investigated as an intervention for several other neurologic conditions (40) and medical diseases such as obesity (41) and type 2 diabetes (42).
Studies examining the use of ketogenic diets in AD generally support the notion that ketogenic diets can enhance cognition in older adults with AD (43–47) and suggest that the degree of cognitive enhancement depends on the level and duration of ketosis, but results have been mixed. Petersson et al. (48) showed that older persons who typically consumed high carbohydrate diets had an increased risk of MCI and dementia (hazard ratio [HR] = 1.89), whereas those with high fat (HR = 0.56) and high protein (HR = 0.79) diets had decreased risks. Krikorian et al. (49) showed that among persons with MCI, only those on low-carbohydrate diets for 6 weeks showed improved memory, which was correlated with urine ketone levels. Taylor et al. (50) demonstrated improved cognition in persons with AD after 3 months on a ketogenic diet and taking a medium-chain-triglyceride (MCT) fat supplement (51). Phillips showed that AD patients on a ketogenic diet improved significantly in quality of life and daily functioning compared to those following “health-eating” guidelines over a 12-week period, but improvements in cognition were not significant (52). Ketosis may not be the only factor influencing memory performance in individuals on ketogenic diet therapy, and further investigation is warranted to examine other potential mechanisms of action.
The present study examined the effects of a 12-week MAD diet on cognition, dietary adherence, ketosis, and lipidomic-metabolomics in seniors with AD-related MCI or mild dementia.
2 Methods
We conducted a 12-week, parallel-group, 1:1 randomized controlled trial of the MAD compared to a control diet (based on the National Institute on Aging [NIA]’s dietary recommendations for seniors) for patients with MCI attributed to AD. The study was fully reviewed and approved by the Institutional Review Board of the Johns Hopkins University School of Medicine.
2.1 Participants
Complete eligibility criteria, procedures (including detailed dietary information), methods, and clinical outcomes are described in a prior publication (53). Briefly, patients with MCI or early-stage AD were recruited via the Johns Hopkins Alzheimer’s Disease Research Center registry, posted flyers, radio and newspaper advertisements, mailings to persons on the Central Maryland chapter of the Alzheimer’s Association list, and lectures at senior centers and retirement communities.
Patients were required to cohabitate with a cognitively intact study partner whose role was to help the patient shop for and prepare appropriate foods, document food intake, test and record urine ketone levels, attend study visits, and provide information regarding the patient’s functional abilities. Patients taking medications for AD were not excluded from the study, as long as they were on the same dose for 3 months or more before the study began and did not change their dose during the study. Complete eligibility criteria appear in Supplementary Table S1.
2.2 Procedures
2.2.1 Eligibility screening
Potential participants were contacted by email or telephone to assess their interest and eligibility. In-person screening visits were conducted with the participant and study partner. The in-person screening included administration of the Montreal Cognitive Assessment (MoCA) (54) to study participants, the Mini-Mental State Examination, second edition (Standard Version; MMSE-2) (55) to study partners, and the Clinical Dementia Rating Scale (CDR) (56) to participant and partner. Study partners were required to earn a T-score of ≥ 40 (adjusted for age and education) on the MMSE-2 to demonstrate intact cognition. Eligible dyads were taught how to complete food records and provided copies of informed consent forms for their review.
2.2.2 Enrollment/baseline assessments
At the enrollment/baseline visit, informed consent was obtained from the patient and the study partner (dyad). A urine sample, fasting blood sample, and vital signs were procured from the patient. The urine sample was tested for the presence of ketones, participants were offered a light snack, and a dietitian reviewed food records with the dyad.
Neuropsychological testing was performed by a research assistant who was blinded to treatment. Testing included the Mini-Mental State Examination-2 Expanded Version (MMSE-2-EV) (55), the Hopkins Verbal Learning Test—Revised (HVLT-R) (57), and the Brief Visuospatial Memory Test—Revised (BVMT-R) (58). MMSE-2-EV scores range from 0 to 90, with 90 indicating perfect performance. HVLT-R and BVMT-R delayed recall trial scores each range from 0 to 12, with 12 indicating perfect performance.
Finally, dyads who enrolled in the study were randomly assigned to one of two diets, either the MAD or the NIA, by using a random number table. Specifically, after informed consent and baseline data were obtained, the study coordinator accessed the random number generator, and if an even number was next on the list, the dyad was randomized to the MAD, and if an odd number, they were randomized to the NIA. The dyad was given their respective diet manual.
2.2.3 Educational visit
Dyads met with a dietitian and were provided with information to initiate and adhere to their diet, including instructions regarding food label reading and portion measuring, as well as sample menus and recipes. Dyads were taught how to record food intake and perform urine ketone testing. They were given a urine ketone test kit, multivitamins, and calcium with vitamin D supplements. Participants had equal time with and access to study dietitians, regardless of group assignment.
The MAD (59) includes consumption of ¾ 20 or fewer grams daily of net carbohydrates (total carbohydrates minus fiber), large amounts of fat, moderate amounts of protein, and proper hydration. The NIA diet encourages the consumption of fruits, vegetables, beans, peas, nuts, seeds, whole grains, fat-free or low-fat dairy products, seafood, lean meats, poultry, eggs, and oils. The NIA diet limits added sugars, refined grains, sodium, and solid fats.
2.2.4 Follow-up visits
Follow-up assessments, including measurement of vital signs and urine ketone levels, review of food records, and discussion with dieticians of any issues with diets or adherence, were conducted every three weeks. Neuropsychological testing was repeated at weeks 6 and 12, and blood was collected again at week 12. At study visits, dyads were offered avocados, a parking voucher, and a $25 grocery store gift card (totaling $100 across study visits).
2.3 Materials
All chemicals and solvents were ultra-pure LC-MS grade (60, 61).
2.3.1 Untargeted lipidomics by MS/MSALL triple TOF
Plasma samples were extracted using a modified Bligh and Dyer’s procedure (60, 62) to obtain a crude lipid fraction. In brief, 40 μL of each plasma sample was extracted for total lipids using 2 mL of ddH2O and 3.8 mL of methanol/dichloromethane (2:1.9, v/v), containing 12 internal standards, as reported earlier (60). Lipid analysis was conducted in MS/MSALL mode on a TripleTOF 5600 (AB Sciex, Redwood City, CA, USA) time-of-flight mass spectrometer (TOF MS) (60). Samples (50 μL of injection volume) were directly infused by HPLC at a constant flow rate of 7 µL/min. The obtained mass spectral data were processed using the LipidView database (version 1.3, Ab Sciex, Concord, Ontario, Canada) as described (60).
2.3.2 Targeted metabolomics by LC-MS/MS
Metabolites from plasma were extracted as described earlier (61). Briefly, plasma samples (150 µL) were protein precipitated using 1 mL of 70% ice-cold methanol (0.5% 1 N HCl), prespiked with 11 isotopically labeled IS (61). Chromatographic separation of metabolites was achieved on the pentafluorophenyl column (pursuit PFP, 150 mm × 2 mm, 3 µM particle size, Agilent Technologies, CA, USA). A quadrupole ion trap mass spectrometer (API4000 QTRAP LC-MS/MS, AB Sciex, ON, Canada) was used to identify metabolites in pseudo-MRM mode. The mass spectrometer was operated in electrospray ionization (ESI)-positive and ESI-negative modes individually to detect protonated [M+H]+ and deprotonated [M-H]− metabolites. Instrument control and quantification were performed using Analyst 1.4.2 and MultiQuant software (AB Sciex, Thornhill, ON, Canada). The complete list of targeted metabolites and their mass spectrometric details are provided in the Supplementary Materials.
2.4 Data analysis
Adherence for the MAD participants was determined a priori as demonstrating at least moderate urine ketone levels at three or more follow-up visits. The primary outcome for memory was defined as change from baseline to 12 weeks in the Memory Composite Score (MCS), defined as the sum of the delayed recall trials for the Hopkins Verbal Learning Test-Revised (HVLT-R) (63) (a measure of auditory-verbal learning and memory) and the Brief Visuospatial Memory Test-Revised (BVMT-R) (64) (a measure of visuospatial learning and memory).
The average treatment effect of the intervention was assessed using an unadjusted difference in means as well as a covariate-adjusted difference in means. Covariate adjustment was performed using analysis of covariance (ANCOVA), regressing the 12-week outcome on the baseline MCS and treatment assignment. Standardized effect sizes (Cohen’s D) were also computed. Confidence intervals and hypothesis tests were conducted using the 10,000 replicates of the nonparametric bootstrap using the bias-corrected and accelerated method. Missing values of covariates were imputed using mean imputation in each bootstrap sample. In analyses of cognitive outcomes, missing follow-up outcome data were addressed using inverse probability weighting (IPW) based on treatment assignment and baseline MCS. Analyses were conducted according to the allocated treatment arm, irrespective of adherence to the intervention; all randomized participants were included in analyses of cognitive outcomes.
Lipidomic and metabolomic data were analyzed using ANCOVA, where each biomarker at 12-week follow-up was regressed on treatment assignment and the biomarker assessed at baseline. Due to sample size limitations, IPW was not performed. Model-based standard errors were used to compute confidence intervals. All biomarkers were log-transformed prior to analysis. Adjustment for multiple testing was performed using the method of Benjamini and Hochberg (65).
All analyses were conducted in R version 4.3.1 (R Foundation for Statistical Computing, Vienna, Austria).
3 Results
As shown in Figure 1, 839 people were screened for the larger study from which the data for the present study were extracted (53, 66). The most common reasons for exclusion from the study were medical conditions, severe cognitive impairment, and living alone. While most eligible parties expressed interest in participating in the study, many declined due to not wanting to change their diet, as well as time and travel costs. Ultimately, 20 were enrolled and randomized to the MAD arm, while 18 were enrolled and randomized to the NIA arm. Please see Table 1 for baseline demographic and clinical factors for all enrolled and randomized participants. In total, 13 of the MAD and nine of the NIA participants completed the study, with only two MAD participants meeting adherence criteria. The most common reasons for withdrawing were no longer wanting to adhere to a diet, as well as time and travel costs. Of the completers, nine of the MAD and six of the NIA were able to provide blood samples deemed adequate for the analyses conducted in the present study; several participants were not able to produce enough blood, and several were not fasting. See Supplementary Table S2 for demographic information regarding the 15 participants included in lipidomic and metabolomic analyses. There were no adverse events.
3.1 Memory changes in MAD and control groups
The results of the 12-week baseline change in MCS analyses can be found in Table 2. Unadjusted and covariate-adjusted analyses were quantitatively similar, with MAD participants having baseline changes in MCS scores that were on average 1.37 points higher than NIA participants (95% CI: −0.87, 4.90). The estimate of the effect size on baseline change was of “medium” magnitude (Cohen’s D = 0.57, 95% CI: −0.67, 1.33) per Cohen’s (1998) criteria, with a confidence interval spanning medium magnitude negative effects to “very large” positive effects.
3.2 Lipids and metabolites with MAD and control groups
We have identified a shared presence of 378 lipid species and 68 metabolites in both the control diet and MAD groups. The comprehensive list of these commonly found lipids and metabolites, along with their respective group classifications, can be found in the Supplementary Materials. Individuals subjected to a MAD for 12 weeks displayed notable differences in plasma levels of lipids and metabolites when compared to those on the control diet. Specifically, the MAD resulted in lower plasma levels of various lipids, encompassing three triacylglycerols (TAGs, 50:5, 52:5, and 52:6), four sphingomyelins (SM, 44:3, 46:3, 46:0, and 48:1), two lysophosphatidylcholines (LysoPCs, 18:0 and 18:2), two fatty acylcarnitines (hexanoylcarnitine and stearoylcarnitine), a fatty acid (hexanoic acid), a hexose sugar (fructose), and a phosphatidylserine ether species (PS O-40:0). Conversely, individuals on MAD exhibited higher levels of a ketone body (acetoacetate), glycerol-3-phosphate, two hydroxy fatty acids (3-hydroxyoctanoate and 5-hydroxyhexanoate), two phosphatidylethanolamines (PE O-34:1 and PE O-36:3), and choline phosphate. These findings strongly indicate alterations in the circulating lipids and metabolites of individuals following a ketogenic diet therapy in comparison to those on a control diet. The modified list of changes in the lipids and metabolites, including adjusted p-values and fold-change values, can be found in Table 3.
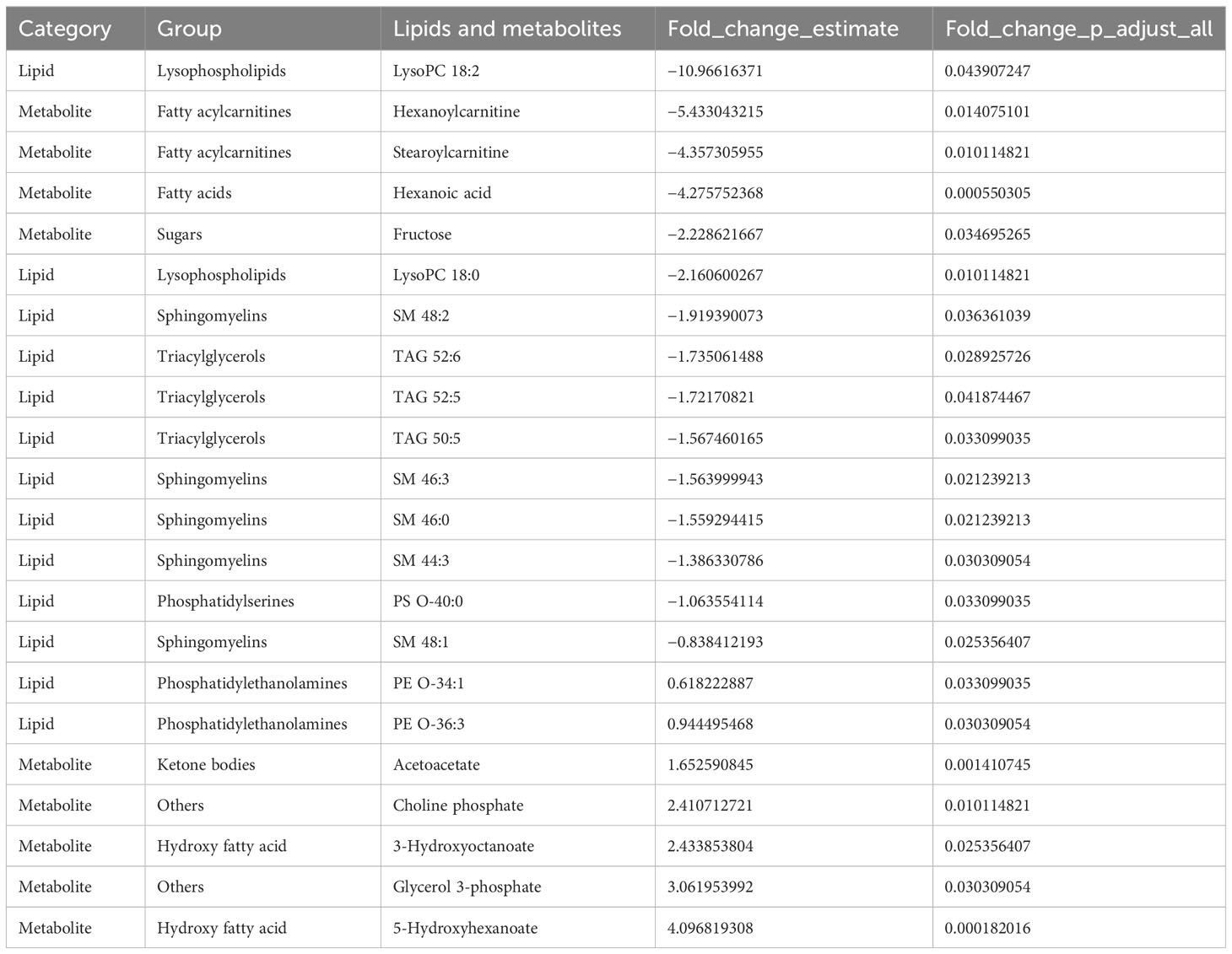
Table 3 The list of lipid and metabolite species that were differentially regulated by the MAD diet compared to the control diet.
Furthermore, our investigation revealed the presence of 77 distinct lipids that were exclusively identified in either the control or MAD groups. Notably, a significant observation was that several TAGs, along with a limited number of glycoceramides present in the control group, were conspicuously absent in the MAD group. Conversely, a multitude of ceramides and phosphatidylethanolamines detected within the MAD group remained undetected in the control group. These findings indicate that the modified Atkins diet elicited specific and discernible alterations in lipid profiles. The compilation of unique metabolites identified within each respective group can be found in the Supplementary Materials.
4 Discussion
Safe and effective therapies for mitigating memory loss in elderly people with MCI and mild dementia due to AD are severely lacking. New therapies like lecanemab, an IgG1 monoclonal antibody shown to reduce markers of amyloid in the brains of patients with early AD (67), are not without limitations. Serious concerns have arisen regarding safety and cost as well as translation to meaningful clinical outcomes (5, 6). One promising strategy for treating this progressive neurodegenerative disease is to compensate for the reduced cerebral glucose utilization in AD (28, 68) using ketone bodies as an alternative energy source. In this study, we examined the feasibility of measuring changes in cognition and the lipids and metabolites during 12 weeks of use of a ketogenic diet therapy (MAD) compared to a recommended diet for elderly individuals.
The present study demonstrated that recruitment for dietary interventions in older adults with cognitive impairment is very challenging due to participant eligibility and interest. This study had high attrition after baseline but retained all participants who were in the study at week 6, which suggests a run-in period could greatly reduce attrition after randomization. Rates of attrition were comparable across treatment groups and to those in similar studies (50, 69, 70). Possibilities for enhancing recruitment, adherence, and retention in future efficacy studies could include the following: adjusting inclusion/exclusion criteria to capture individuals with greater willingness to change their dietary habits; educating study staff and participants that remaining in the study is extremely valuable even if they do not adhere to the assigned diet; conducting more virtual assessments, whether by telephone, video, or messaging; making in-person visits less frequent, shorter, and less burdensome; using automatic/scheduled reminders for adherence to study protocol at home; including incentives for adherence; and incorporating virtual consulting/coaching with a dietitian or nutritionist to supervise shopping and meal planning/preparation.
Pilot studies are often not powered to detect meaningful differences in the primary outcome with high confidence, but they can provide useful information to investigators planning future studies in this setting. While the estimates of the effect size and treatment effect on the change in MCS were positive and the majority of their 95% confidence intervals were greater than zero, they were not statistically significant. Results of cognitive outcome analyses should also be cautiously interpreted, given the issues of attrition, adherence, and influential observation.
Following a 12-week MAD intervention, we noted a significant decrease in circulating TAGs (50:5, 52:5, and 52:6). Additionally, certain TAGs (as indicated in Table 3) that were present in the control diet group were not detected in the ketogenic diet group. This suggests an enhanced breakdown of TAGs into fatty acids, likely driven by increased lipolysis, and their subsequent utilization through β-oxidation to produce energy and ketone bodies (61, 62). This interpretation gains support from the observation of elevated levels of ketone body (acetoacetate) production as well as metabolic byproducts from medium-chain fatty acids, including 3-hydroxyoctanoate and 5-hydroxyhexanoate. Moreover, in the MAD group, lower levels of fatty acylcarnitines, specifically hexanoylcarnitine and stearoylcarnitine, which are intermediates of fatty acid oxidation, were observed when compared to the control diet group. These collective findings lend further credence to the notion that the ketogenic diet prompts an increased utilization of fatty acids for energy production through β-oxidation, ultimately leading to the production of ketone bodies.
Both acetoacetate and medium-chain hydroxy fatty acids have been linked to potential cognitive benefits (71, 72). Acetoacetate, a type of ketone body produced during fatty acid metabolism, is suggested to have neuroprotective properties and is considered a potential alternative fuel source for the brain (71, 73). Some research suggests that ketone bodies like acetoacetate may offer neuroprotection and could be beneficial for cognitive function, especially in conditions like neurodegenerative diseases and certain brain injuries (73). However, the exact mechanisms and extent of cognitive improvement are still areas of ongoing research. Medium-chain hydroxy fatty acids, such as 3-hydroxyoctanoate and 5-hydroxyhexanoate, are derived from medium-chain fatty acids and are involved in energy metabolism (72). While the impact of specific hydroxy fatty acids in relation to cognitive outcomes remains under investigation, medium-chain fatty acids, in general, have been studied for their potential cognitive benefits (72). Some studies have suggested that the consumption of MCTs, which can produce medium-chain fatty acids and their derivatives like hydroxy fatty acids, might have positive effects on cognitive function, particularly memory, and brain health (72, 74–76). It is important to note that while there is emerging evidence supporting the potential cognitive benefits of acetoacetate and certain hydroxy fatty acids, the research is still in its early stages, and more studies are needed to establish the exact impact and mechanisms of these compounds on cognitive outcomes.
This study had several important limitations. First, sample sizes were small in both study groups, with challenges in recruitment, retention, and adherence (53). Dropout and adherence may be related to participant characteristics that are directly or indirectly related to their cognitive function, which can lead to selection bias. Second, although great care was taken to enhance the probability that participants’ cognitive impairments were due to AD, it is possible that some participants did not have the pathophysiology of AD, rendering the potential benefits of ketosis less likely. Third, participants, study partners, dieticians, and study coordinators were not blinded to the assigned diet, so the potential for bias cannot be entirely ruled out. In addition, the study’s eligibility criteria intentionally resulted in a sample free of comorbidities that are common in the elderly, including cardiovascular disease and severe diabetes. As such, results from this study may not be generalizable to the larger AD population, in which these comorbidities are also common. The study site and inclusion/exclusion criteria yielded a sample limited to mostly white and highly educated participants, which greatly limits the generalizability of our findings. We targeted patients with MCI and mild dementia due to AD. Perhaps even these early-stage patients are too advanced to benefit from a ketogenic diet. Implementing MAD in less cognitively impaired persons with “subjective cognitive decline” (77–81) might result in larger effects. Finally, not all of the participants who completed the study provided blood samples deemed adequate for analysis, primarily due to nonfasting and/or limited blood supply, which may have introduced bias as well.
Data availability statement
The raw data supporting the conclusions of this article will be made available by the authors, without undue reservation.
Ethics statement
The studies involving humans were approved by The Institutional Review Board of the Johns Hopkins University School of Medicine. The studies were conducted in accordance with the local legislation and institutional requirements. The participants provided their written informed consent to participate in this study.
Author contributions
All authors listed have made a substantial, direct, and intellectual contribution to the work, and approved it for publication.
Funding
The author(s) declare financial support was received for the research, authorship, and/or publication of this article. This research was supported by grants from the William and Ella Owens Medical Research Foundation, the BrightFocus Foundation (to JB), and the National Center for Advancing Translational Sciences (NIH Grant No. UL1-TR 001079). The Hass Avocado Board generously supplied fresh avocados that were made available without cost to research participants. The Johns Hopkins ICTR Grant (UL1TR003098) provided statistical consultation.
Acknowledgments
We thank Dr. Ruihua Wang, Dr. Erie Gonzales, Dr. Barnett Shpritz, Ms. Chandler Zolliecoffer, Ms. Oluwabunmi Oyenusi, and Mr. Danny Lawson for their assistance in conducting this research. Authors’ disclosures are available online (https://www.j-alz.com/manuscript-disclosures/18-0995r2).
Conflict of interest
Dr. MC receives or has received research grants from Vitaflo, a business unit of Nestlé Health Science, Nutricia, a part of Danone, Glut1 Deficiency Foundation, and BrightFocus Foundation. Honoraria from Nutricia/Danone, Vitaflo/Nestlé Health Science, and The Neurology Center Rockville. Royalties from Demos/Springer Publishing Company. Consulting for Nutricia/Danone and Glut1 Deficiency Foundation. JFB is entitled to equity and future royalties in miDiagnostics.
The remaining authors declare that the research was conducted in the absence of any commercial or financial relationships that could be construed as a potential conflict of interest.
Publisher’s note
All claims expressed in this article are solely those of the authors and do not necessarily represent those of their affiliated organizations, or those of the publisher, the editors and the reviewers. Any product that may be evaluated in this article, or claim that may be made by its manufacturer, is not guaranteed or endorsed by the publisher.
Supplementary material
The Supplementary Material for this article can be found online at: https://www.frontiersin.org/articles/10.3389/fendo.2024.1182519/full#supplementary-material
References
1. Alzheimer's association report. Alzheimer’s disease facts and figures. Alzheimers Dement (2021) 17:327–406. doi: 10.1002/alz.12328.
2. Rabinovici GD. Controversy and progress in Alzheimer's disease - FDA approval of aducanumab. N Engl J Med (2021) 385:771–4. doi: 10.1056/NEJMp2111320.
3. Mintun MA, Lo AC, Duggan Evans C, Wessels AM, Ardayfio PA, Andersen SW, et al. Donanemab in early Alzheimer's disease. N Engl J Med (2021) 384:1691–704. doi: 10.1056/NEJMoa2100708.
4. Swanson CJ, Zhang Y, Dhadda S, Wang J, Kaplow J, Lai RYK, et al. A randomized, double-blind, phase 2b proof-of-concept clinical trial in early Alzheimer's disease with lecanemab, an anti-Abeta protofibril antibody. Alzheimers Res Ther (2021) 13:80. doi: 10.1186/s13195-021-00813-8.
5. Larkin HD. Lecanemab gains FDA approval for early Alzheimer disease. JAMA (2023). doi: 10.1001/jama.2022.24490.
6. Reardon S. FDA approves Alzheimer's drug lecanemab amid safety concerns. Nature (2023) 613:227–8. doi: 10.1038/d41586-023-00030-3.
7. Mosconi L, McHugh PF. Let food be thy medicine: diet, nutrition, and biomarkers' Risk of Alzheimer's disease. Curr Nutr Rep (2015) 4:126–35. doi: 10.1007/s13668-014-0111-5.
8. Solfrizzi V, Panza F, Frisardi V, Seripa D, Logroscino G, Imbimbo BP, et al. Diet and Alzheimer's disease risk factors or prevention: the current evidence. Expert Rev Neurother (2011) 11:677–708.
9. Mi W, van Wijk N, Cansev M, Sijben JW, Kamphuis PJ. Nutritional approaches in the risk reduction and management of Alzheimer's disease. Nutrition (2013) 29:1080–9. doi: 10.1016/j.nut.2013.01.024.
10. Reed B, Villeneuve S, Mack W, DeCarli C, Chui HC, Jagust W. Associations between serum cholesterol levels and cerebral amyloidosis. JAMA Neurol (2014) 71:195–200. doi: 10.1001/jamaneurol.2013.5390.
11. Shobab LA, Hsiung GY, Feldman HH. Cholesterol in Alzheimer's disease. Lancet Neurol (2005) 4:841–52. doi: 10.1016/S1474-4422(05)70248-9.
12. Poirier J, Apolipoprotein E. cholesterol transport and synthesis in sporadic Alzheimer's disease. Neurobiol Aging (2005) 26:355–61. doi: 10.1016/j.neurobiolaging.2004.09.003.
13. Wellington CL. Cholesterol at the crossroads: Alzheimer's disease and lipid metabolism. Clin Genet (2004) 66:1–16. doi: 10.1111/j.0009-9163.2004.00280.x.
14. Kivipelto M, Helkala EL, Laakso MP, Hanninen T, Hallikainen M, Alhainen K, et al. Apolipoprotein E epsilon4 allele, elevated midlife total cholesterol level, and high midlife systolic blood pressure are independent risk factors for late-life Alzheimer disease. Ann Internal Med (2002) 137:149–55. doi: 10.7326/0003-4819-137-3-200208060-00006.
15. Austen B, Christodoulou G, Terry JE. Relation between cholesterol levels, statins and Alzheimer's disease in the human population. J nutrition Health Aging (2002) 6:377–82.
16. Evans RM, Emsley CL, Gao S, Sahota A, Hall KS, Farlow MR, et al. Serum cholesterol, APOE genotype, and the risk of Alzheimer's disease: a population-based study of African Americans. Neurology (2000) 54:240–2. doi: 10.1212/WNL.54.1.240.
17. Notkola IL, Sulkava R, Pekkanen J, Erkinjuntti T, Ehnholm C, Kivinen P, et al. Serum total cholesterol, apolipoprotein E epsilon 4 allele, and Alzheimer's disease. Neuroepidemiology (1998) 17:14–20. doi: 10.1159/000026149.
18. Scarmeas N, Stern Y, Tang MX, Mayeux R, Luchsinger JA. Mediterranean diet and risk for Alzheimer's disease. Ann Neurol (2006) 59:912–21. doi: 10.1002/ana.20854.
19. Yassine HN, Self W, Kerman BE, Santoni G, Navalpur Shanmugam N, Abdullah L, et al. Nutritional metabolism and cerebral bioenergetics in Alzheimer's disease and related dementias. Alzheimer's Dementia n/a (2022). doi: 10.1002/alz.12845.
20. Mamalaki E, Ntanasi E, Hatzimanolis A, Basta M, Kosmidis MH, Dardiotis E, et al. The association of adherence to the mediterranean diet with depression in older adults longitudinally taking into account cognitive status: results from the HELIAD study. Nutrients (2023). doi: 10.3390/nu15020359.
21. Refolo LM, Malester B, LaFrancois J, Bryant-Thomas T, Wang R, Tint GS, et al. Hypercholesterolemia accelerates the Alzheimer's amyloid pathology in a transgenic mouse model. Neurobiol Dis (2000) 7:321–31. doi: 10.1006/nbdi.2000.0304.
22. Shie FS, Jin LW, Cook DG, Leverenz JB, LeBoeuf RC. Diet-induced hypercholesterolemia enhances brain A beta accumulation in transgenic mice. Neuroreport (2002) 13:455–9. doi: 10.1097/00001756-200203250-00019.
23. Morris MC, Tangney CC, Wang Y, Sacks FM, Bennett DA, Aggarwal NT. MIND diet associated with reduced incidence of Alzheimer's disease. Alzheimer's dementia (2015). doi: 10.1016/j.jalz.2014.11.009.
24. Levitan EB, Lewis CE, Tinker LF, Eaton CB, Ahmed A, Manson JE, et al. Mediterranean and DASH diet scores and mortality in women with heart failure: The Women's Health Initiative. Circulation. Heart failure (2013) 6:1116–23. doi: 10.1161/CIRCHEARTFAILURE.113.000495.
25. Cunnane S, Nugent S, Roy M, Courchesne-Loyer A, Croteau E, Tremblay S, et al. Brain fuel metabolism, aging, and Alzheimer's disease. Nutrition (2011) 27:3–20. doi: 10.1016/j.nut.2010.07.021.
26. Castellano CA, Nugent S, Paquet N, Tremblay S, Bocti C, Lacombe G, et al. Lower brain 18F-fluorodeoxyglucose uptake but normal 11C-acetoacetate metabolism in mild Alzheimer's disease dementia. J Alzheimer's Dis (2015) 43:1343–53. doi: 10.3233/JAD-141074.
27. Croteau E, Castellano CA, Fortier M, Bocti C, Fulop T, Paquet N, et al. A cross-sectional comparison of brain glucose and ketone metabolism in cognitively healthy older adults, mild cognitive impairment and early Alzheimer's disease. Exp Gerontol (2018) 107:18–26. doi: 10.1016/j.exger.2017.07.004.
28. An Y, Varma VR, Varma S, Casanova R, Dammer E, Pletnikova O, et al. Evidence for brain glucose dysregulation in Alzheimer's disease. Alzheimers Dement (2018) 14:318–29. doi: 10.1016/j.jalz.2017.09.011.
29. Biessels GJ, Staekenborg S, Brunner E, Brayne C, Scheltens P. Risk of dementia in diabetes mellitus: a systematic review. Lancet Neurol (2006) 5:64–74. doi: 10.1016/S1474-4422(05)70284-2.
30. Biessels GJ, Deary IJ, Ryan CM. Cognition and diabetes: a lifespan perspective. Lancet Neurol (2008) 7:184–90. doi: 10.1016/S1474-4422(08)70021-8.
31. Reger MA, Watson GS, Frey 2WH, Baker LD, Cholerton B, Keeling ML, et al. Effects of intranasal insulin on cognition in memory-impaired older adults: modulation by APOE genotype. Neurobiol Aging (2006) 27:451–8. doi: 10.1016/j.neurobiolaging.2005.03.016.
32. Watson GS, Craft S. Modulation of memory by insulin and glucose: neuropsychological observations in Alzheimer's disease. Eur J Pharmacol (2004) 490:97–113. doi: 10.1016/j.ejphar.2004.02.048.
33. Jack CR Jr., Knopman DS, Jagust WJ, Shaw LM, Aisen PS, Weiner MW, et al. Hypothetical model of dynamic biomarkers of the Alzheimer's pathological cascade. Lancet Neurol (2010) 9:119–28. doi: 10.1016/S1474-4422(09)70299-6.
34. Jack CR Jr., Knopman DS, Jagust WJ, Petersen RC, Weiner MW, Aisen PS, et al. Tracking pathophysiological processes in Alzheimer's disease: an updated hypothetical model of dynamic biomarkers. Lancet Neurol (2013) 12:207–16. doi: 10.1016/S1474-4422(12)70291-0.
35. Kossoff EH, Rowley H, Sinha SR, Vining EP. A prospective study of the modified Atkins diet for intractable epilepsy in adults. Epilepsia (2008) 49:316–9. doi: 10.1111/j.1528-1167.2007.01256.x.
36. Freeman JM, Kossoff EH, Hartman AL. The ketogenic diet: one decade later. Pediatrics (2007) 119:535–43. doi: 10.1542/peds.2006-2447.
37. Freeman JM, Vining EP, Pillas DJ, Pyzik PL, Casey JC, Kelly LM. The efficacy of the ketogenic diet-1998: a prospective evaluation of intervention in 150 children. Pediatrics (1998) 102:1358–63. doi: 10.1542/peds.102.6.1358.
38. Cervenka MC, Terao NN, Bosarge JL, Henry BJ, Klees AA, Morrison PF, et al. E-mail management of the modified Atkins Diet for adults with epilepsy is feasible and effective. Epilepsia (2012) 53:728–32. doi: 10.1111/j.1528-1167.2012.03406.x.
39. Cervenka MC, Patton K, Eloyan A, Henry B, Kossoff EH. The impact of the modified Atkins diet on lipid profiles in adults with epilepsy. Nutr Neurosci (2016) 19:131–7. doi: 10.1179/1476830514Y.0000000162.
40. Wang Y, Zhang J, Zhang Y, Yao J. Bibliometric analysis of global research profile on ketogenic diet therapies in neurological diseases: Beneficial diet therapies deserve more attention. Front Endocrinol (Lausanne) (2022) 13:1066785. doi: 10.3389/fendo.2022.1066785.
41. Moreno B, Bellido D, Sajoux I, Goday A, Saavedra D, Crujeiras AB, et al. Comparison of a very low-calorie-ketogenic diet with a standard low-calorie diet in the treatment of obesity. Endocrine (2014) 47:793–805. doi: 10.1007/s12020-014-0192-3.
42. Abbasi J. Interest in the Ketogenic diet grows for weight loss and type 2 diabetes. JAMA (2018) 319:215–7. doi: 10.1001/jama.2017.20639.
43. Rusek M, Pluta R, Ulamek-Koziol M, Czuczwar SJ. Ketogenic diet in Alzheimer's disease. Int J Mol Sci (2019) 20. doi: 10.3390/ijms20163892.
44. Lilamand M, Porte B, Cognat E, Hugon J, Mouton-Liger F, Paquet C. Are ketogenic diets promising for Alzheimer's disease? A Trans review Alzheimers Res Ther (2020) 12:42. doi: 10.1186/s13195-020-00615-4.
45. Broom GM, Shaw IC, Rucklidge JJ. The ketogenic diet as a potential treatment and prevention strategy for Alzheimer's disease. Nutrition (2019) 60:118–21. doi: 10.1016/j.nut.2018.10.003.
46. Neth BJ, Mintz A, Whitlow C, Jung Y, Solingapuram Sai K, Register TC, et al. Modified ketogenic diet is associated with improved cerebrospinal fluid biomarker profile, cerebral perfusion, and cerebral ketone body uptake in older adults at risk for Alzheimer's disease: a pilot study. Neurobiol Aging (2020) 86:54–63. doi: 10.1016/j.neurobiolaging.2019.09.015.
47. Lilamand M, Mouton-Liger F, Paquet C. Ketogenic diet therapy in Alzheimer's disease: an updated review. Curr Opin Clin Nutr Metab Care (2021) 24:372–8. doi: 10.1097/MCO.0000000000000759.
48. Petersson SD, Philippou E. Mediterranean diet, cognitive function, and dementia: A systematic review of the evidence. Adv Nutr (2016) 7:889–904. doi: 10.3945/an.116.012138.
49. Krikorian R, Shidler MD, Dangelo K, Couch SC, Benoit SC, Clegg DJ. Dietary ketosis enhances memory in mild cognitive impairment. Neurobiol Aging (2012) 33:425 e19–27. doi: 10.1016/j.neurobiolaging.2010.10.006.
50. Taylor MK, Sullivan DK, Mahnken JD, Burns JM, Swerdlow RH. Feasibility and efficacy data from a ketogenic diet intervention in Alzheimer's disease. Alzheimer's Dementia: Trans Res Clin Interventions (2017) 1-9.
51. Jadhav HB, Annapure US. Triglycerides of medium-chain fatty acids: a concise review. J Food Sci Technol (2022), 1–10. doi: 10.1007/s13197-022-05499-w.
52. Phillips MCL, Deprez LM, Mortimer GMN, Murtagh DKJ, McCoy S, Mylchreest R, et al. Randomized crossover trial of a modified ketogenic diet in Alzheimer's disease. Alzheimers Res Ther (2021) 13:51. doi: 10.1186/s13195-021-00783-x.
53. Brandt J, Buchholz A, Henry-Barron B, Vizthum D, Avramopoulos D, Cervenka MC. Preliminary report on the feasibility and efficacy of the modified Atkins diet for treatment of mild cognitive impairment and early Alzheimer's disease. J Alzheimers Dis (2019) 68:969–81. doi: 10.3233/JAD-180995.
54. Nasreddine ZS, Phillips NA, Bedirian V, Charbonneau S, Whitehead V, Collin I, et al. The Montreal Cognitive Assessment, MoCA: a brief screening tool for mild cognitive impairment. J Am Geriatr Soc (2005) 53:695–9. doi: 10.1111/j.1532-5415.2005.53221.x.
55. Folstein MF, Folstein SE, White T, Messer MA. Mini-mental state examination. In: User's Manual, 2nd edition. Psychological Assessment Resources, Inc., Lutz, FL. (2012).
57. Brandt J, Benedict RHB. The Hopkins Verbal Learning Test - Revised Lutz, FL: Psychological Assessment Resources, Inc. (2001).
58. Benedict RHB. Brief Visuospatial Memory Test, Professional Manual Lutz, FL: Psychological Assessment Resources, Inc. (1997).
59. Kossoff EH, Dorward JL. The modified Atkins diet. Epilepsia (2008) 49 Suppl 8:37–41. doi: 10.1111/j.1528-1167.2008.01831.x.
60. Chen S, Datta-Chaudhuri A, Deme P, Dickens A, Dastgheyb R, Bhargava P, et al. Lipidomic characterization of extracellular vesicles in human serum. J Circulating Biomarkers (2019) 8:1849454419879848. doi: 10.1177/1849454419879848.
61. Deme P, Rubin LH, Yu D, Xu Y, Nakigozi G, Nakasujja N, et al. Immunometabolic reprogramming in response to HIV infection is not fully normalized by suppressive antiretroviral therapy. Viruses (2022) 14:1313. doi: 10.3390/v14061313.
62. Bligh EG, Dyer WJ. A rapid method of total lipid extraction and purification. Can J Biochem Physiol (1959) 37:911–7. doi: 10.1139/o59-099.
63. Benedict RHB, Schretlen D, Groninger L, Brandt J. Hopkins verbal learning test – revised: normative data and analysis of inter-form and test-retest reliability. Clin Neuropsychologist (1998) 12:43–55.
64. Tam JW, Schmitter-Edgecombe M. The role of processing speed in the brief visuospatial memory test – revised. Clin Neuropsychologist (2013) 27:962–72. doi: 10.1080/13854046.2013.797500.
65. Benjamini Y, Hochberg Y. Controlling the false discovery rate: A practical and powerful approach to multiple testing. J R Stat Society: Ser B (Methodological) (1995) 57:289–300. doi: 10.1111/j.2517-6161.1995.tb02031.x.
66. Cervenka MC, Hocker S, Koenig M, Bar B, Henry-Barron B, Kossoff EH, et al. Phase I/II multicenter ketogenic diet study for adult superrefractory status epilepticus. Neurology (2017) 88:938–43. doi: 10.1212/WNL.0000000000003690.
67. van Dyck CH, Swanson CJ, Aisen P, Bateman RJ, Chen C, Gee M, et al. Lecanemab in early Alzheimer's disease. N Engl J Med (2023) 388:9–21. doi: 10.1056/NEJMoa2212948.
68. Meier-Ruge W, Bertoni-Freddari C, Iwangoff P. Changes in brain glucose metabolism as a key to the pathogenesis of Alzheimer's disease. Gerontology (1994) 40:246–52.
69. Edwards RR, Haythornthwaite J. Mood swings: variability in the use of the Profile of Mood States. J Pain symptom Manage (2004) 28:534. doi: 10.1016/j.jpainsymman.2004.10.004.
70. Landi F, Tua E, Onder G, Carrara B, Sgadari A, Rinaldi C, et al. Minimum data set for home care: a valid instrument to assess frail older people living in the community. Med Care (2000) 38:1184–90. doi: 10.1097/00005650-200012000-00005.
71. Jensen NJ, Wodschow HZ, Nilsson M, Rungby J. Effects of ketone bodies on brain metabolism and function in neurodegenerative diseases. Int J Mol Sci (2020) 21. doi: 10.3390/ijms21228767.
72. Page KA, Williamson A, Yu N, McNay EC, Dzuira J, McCrimmon RJ, et al. Medium-chain fatty acids improve cognitive function in intensively treated type 1 diabetic patients and support in vitro synaptic transmission during acute hypoglycemia. Diabetes (2009) 58:1237–44. doi: 10.2337/db08-1557.
73. Altayyar M, Nasser JA, Thomopoulos D, Bruneau M Jr. The implication of physiological ketosis on the cognitive brain: A narrative review. Nutrients (2022) 14. doi: 10.3390/nu14030513.
74. Traul KA, Driedger A, Ingle DL, Nakhasi D. Review of the toxicologic properties of medium-chain triglycerides. Food Chem Toxicol (2000) 38:79–98. doi: 10.1016/S0278-6915(99)00106-4.
75. Ebert D, Haller RG, Walton ME. Energy contribution of octanoate to intact rat brain metabolism measured by 13C nuclear magnetic resonance spectroscopy. J Neurosci (2003) 23:5928–35. doi: 10.1523/JNEUROSCI.23-13-05928.2003.
76. Mett J. The impact of medium chain and polyunsaturated ω-3-fatty acids on amyloid-β Deposition, oxidative stress and metabolic dysfunction associated with Alzheimer's disease. Antioxidants (Basel) (2021) 10. doi: 10.3390/antiox10121991.
77. Snitz BE, Wang T, Cloonan YK, Jacobsen E, Chang CH, Hughes TF, et al. Risk of progression from subjective cognitive decline to mild cognitive impairment: The role of study setting. Alzheimer's dementia (2018) 14:734–42. doi: 10.1016/j.jalz.2017.12.003.
78. Rabin LA, Smart CM, Amariglio RE. Subjective cognitive decline in preclinical Alzheimer's disease. Annu Rev Clin Psychol (2017) 13:369–96. doi: 10.1146/annurev-clinpsy-032816-045136.
79. Molinuevo JL, Rabin LA, Amariglio R, Buckley R, Dubois B, Ellis KA, et al. Implementation of subjective cognitive decline criteria in research studies. Alzheimer's dementia (2017) 13:296–311. doi: 10.1016/j.jalz.2016.09.012.
80. Tales A, Jessen F, Butler C, Wilcock G, Phillips J, Bayer T. Subjective cognitive decline. J Alzheimer's Dis (2015) 48 Suppl 1:S1–3. doi: 10.3233/JAD-150719.
Keywords: mild cognitive impairment, Alzheimer’s disease, lipidomic, metabolomic, ketogenic, ketone, modified Atkins diet
Citation: Buchholz A, Deme P, Betz JF, Brandt J, Haughey N and Cervenka MC (2024) A randomized feasibility trial of the modified Atkins diet in older adults with mild cognitive impairment due to Alzheimer’s disease. Front. Endocrinol. 15:1182519. doi: 10.3389/fendo.2024.1182519
Received: 08 March 2023; Accepted: 05 February 2024;
Published: 04 March 2024.
Edited by:
Kalina Duszka, University of Vienna, AustriaReviewed by:
Nicholas G. Norwitz, Harvard Medical School, United StatesStefan Kabisch, Charité University Medicine Berlin, Germany
Copyright © 2024 Buchholz, Deme, Betz, Brandt, Haughey and Cervenka. This is an open-access article distributed under the terms of the Creative Commons Attribution License (CC BY). The use, distribution or reproduction in other forums is permitted, provided the original author(s) and the copyright owner(s) are credited and that the original publication in this journal is cited, in accordance with accepted academic practice. No use, distribution or reproduction is permitted which does not comply with these terms.
*Correspondence: Alison Buchholz, YWJ1Y2hobzFAamhtaS5lZHU=
†These authors share first authorship
‡These authors share senior authorship