- 1Department of Endocrinology, Qilu Hospital, Shandong University, Jinan, Shandong, China
- 2Institute of Endocrine and Metabolic Diseases of Shandong University, Jinan, Shandong, China
- 3Key Laboratory of Endocrine and Metabolic Diseases, Shandong Province Medicine and Health, Jinan, Shandong, China
- 4Jinan Clinical Research Center for Endocrine and Metabolic Diseases, Jinan, Shandong, China
Metabolic disorders remain a major global health concern in the 21st century, with increasing incidence and prevalence. Mitochondria play a critical role in cellular energy production, calcium homeostasis, signal transduction, and apoptosis. Under physiological conditions, mitochondrial transfer plays a crucial role in tissue homeostasis and development. Mitochondrial dysfunction has been implicated in the pathogenesis of metabolic disorders. Numerous studies have demonstrated that mitochondria can be transferred from stem cells to pathologically injured cells, leading to mitochondrial functional restoration. Compared to cell therapy, mitochondrial transplantation has lower immunogenicity, making exogenous transplantation of healthy mitochondria a promising therapeutic approach for treating diseases, particularly metabolic disorders. This review summarizes the association between metabolic disorders and mitochondria, the mechanisms of mitochondrial transfer, and the therapeutic potential of mitochondrial transfer for metabolic disorders. We hope this review provides novel insights into targeted mitochondrial therapy for metabolic disorders.
1 Introduction
Metabolic disorders, including diabetes, non-alcoholic fatty liver disease (NAFLD), obesity, hyperlipidemia, and gout, are major health concerns (1, 2). Diabetes has become one of the most prevalent health disparities, with an estimated 537 million adults aged 20-79 living with diabetes worldwide in 2021. This accounts for 10.5% of the global population in that age group. It is projected to rise to 643 million (11.3%) by 2030 and 783 million (12.2%) by 2045 (3). NAFLD, a common metabolic disorder, encompasses a spectrum of liver abnormalities from non-alcoholic fatty liver (NAFL) to non-alcoholic steatohepatitis (NASH), which can progress to cirrhosis and liver cancer (4–6).
Mitochondria are semi-autonomous, semi-self-replicating, highly dynamic organelles with their own circular double-stranded DNA molecules (7–9). They are responsible for coordinating cellular energy production and are involved in calcium signaling, cell growth and differentiation, cell cycle control, and cell death processes (8, 10–12). Mitochondrial dysfunction is a common underlying pathophysiological mechanism in many diseases, characterized by the generation of reactive oxygen species and accumulation of mitochondrial DNA (mtDNA) damage, leading to mitochondrial dysfunction (13–15).
In 2004, Rustom et al. discovered the existence of mitochondrial transport through tunneling nanotubes (TNTs) (16). Subsequent studies demonstrated the widespread ability of mammalian cells to acquire organelles from other cells, including the transfer of mitochondria between mammalian cells (17–21). Further research by Rustom et al. found that mesenchymal stem cells transfer mitochondria to recipient cells, rescuing injured cells, improving oxidative phosphorylation, increasing ATP production, and restoring mitochondrial function (22). Therefore, mitochondrial transfer holds potential therapeutic effects for metabolic disorders and their complications (23–25). This article provides a comprehensive review of research progress on mitochondrial transfer in the treatment of metabolic disorders, offering valuable insights into this field.
2 Association between metabolic disorders and mitochondria
Abnormal mitochondrial function contributes to pathological changes in cellular energy metabolism, disruption of fatty acid metabolism, and increased oxidative stress, leading to the development of metabolic disorders such as type 2 diabetes, obesity, dyslipidemia, and cardiovascular diseases. This section reviews the association between diabetes, obesity, NAFLD, and mitochondrial dysfunction.
2.1 Diabetes
Type 2 diabetes mellitus (T2DM) is associated with alterations in oxidative metabolism in insulin-responsive tissues. T2DM is characterized by reduced mitochondrial oxidative phosphorylation capacity and decreased mitochondrial content in skeletal muscle cells and hepatocytes. Insulin resistance occurs many years before the onset of T2DM. Acquired insulin resistance is associated with decreased mitochondrial activity in response to insulin stimulation, while inherited insulin resistance is typically linked to reduced basal mitochondrial activity, possibly due to decreased mitochondrial content (26). Peroxisome proliferator-activated receptor γ coactivator-1α (PGC-1α), a member of the transcriptional coactivator family, plays a central role in the regulation of cellular energy metabolism (27). Activation of PGC-1α has been shown to increase mitochondrial capacity for oxidative phosphorylation, restore mitochondrial superoxide production, promote insulin secretion in pancreatic β-cells, enhance insulin sensitivity in skeletal muscle and liver, and prevent diabetes microvascular complications (28). In T2DM, decreased expression of PGC-1α and its target genes leads to decreased mitochondrial ATP production (29) and increased ROS generation (30). Similar studies have also suggested that in some tissues associated with diabetic complications, exposure to excessive glucose or nutrient stress leads to decreased mitochondrial superoxide production, oxidative phosphorylation, and mitochondrial ATP generation (28). Obesity, T2DM, and aging are associated with impaired skeletal muscle oxidative capacity, reduced mitochondrial content, and decreased oxidative phosphorylation rates (31). Mitochondrial dysfunction may accelerate the progression of insulin resistance and subsequent organ dysfunction by increasing reactive oxygen species production, while lifestyle and pharmacological interventions can improve insulin resistance by enhancing oxidative phosphorylation capacity and mitochondrial content in early-stage diabetes cases (26).
2.2 NAFLD
NAFLD is considered a mitochondrial disease (32). In mouse models of simple steatosis or NASH, mitochondrial ATP synthesis is reduced, and mitochondria cannot oxidize sufficient fatty acids, leading to hepatic fat accumulation. Additionally, high-fat diet feeding leads to excessive calcium uptake by liver mitochondria, disrupting respiration and increasing ROS production (33). Mitochondrial fragmentation, mediated by Drp1 and MFF, has been shown to increase fatty acid oxidation in adipocytes and serves as a compensatory mechanism against nutrient overload (34, 35). Studies have demonstrated that hepatocyte-specific loss of mitochondrial fusion protein 2 (Mfn2) exacerbates NAFLD progression, inflammation, and hyperglycemia in high-fat diet-fed mice (36). Mitochondrial fragmentation may drive steatosis and NASH by reducing fatty acid oxidation. Moreover, mitochondrial fragmentation can activate mitophagy for the clearance of damaged mitochondria (34). It has been demonstrated that increased levels of fatty acids and ceramides raise the lysosomal pH in hepatocytes, impairing lysosomal function and halting autophagy, leading to the accumulation of damaged mitochondria (37). A recent study showed that hepatocyte-specific loss of Parkin, which selectively targets damaged mitochondria for mitophagy, exacerbates hepatic steatosis and insulin resistance in high-fat diet-fed mice (38). Therefore, reduced mitochondrial engulfment in NASH liver may be due to the failure or dormancy of mechanisms for the removal of damaged mitochondria, allowing the presence of a large number of damaged mitochondria (39).
2.3 Obesity
Mitochondria are closely associated with fatty acid synthesis metabolism and adipocyte function, thus influencing the development of obesity. Similar to diabetes, mitochondrial biogenesis is also reduced in obese individuals (40). Research by Forner et al. revealed that mitochondria in white and brown fat utilize specific isoforms for fatty acid degradation and biosynthesis. Mitochondrial isoforms Gpam, AGPAT2, AGPAT3, DGAT1, Agk, and Acp6 are enriched in the white mitochondrial fraction, and their activities are closely related to triglyceride biosynthesis in adipocytes (41). Studies have shown that decreased mitochondrial transfer from adipocytes to macrophages is associated with obesity in mice (42). Another hallmark of metabolically unhealthy adipocytes is mitochondrial dysfunction (43), which affects adipogenesis, adipokine secretion, lipid turnover, and lipolysis (44). Evidence suggests that obesity is associated with mitochondrial dysfunction in white adipose tissue, characterized by decreased mitochondrial DNA (mtDNA) content, decreased expression of electron transport chain (ETC) genes, impaired mitochondrial oxidative capacity, and elevated reactive oxygen species (ROS) levels (45). Additionally, Yu et al. reported that dysregulation of amyloid precursor protein impairs mitochondrial function in adipose tissue and promotes obesity (46).
3 Mechanisms of intercellular mitochondrial transfer
Since the discovery of mitochondrial transfer from endothelial progenitor cells to cardiomyocytes by Masamichi Koyanagi in 2005 (47), numerous scientists have identified additional evidences of intercellular mitochondrial transfer and investigated their underlying molecular mechanisms. Most studies indicate that mitochondria can be transferred through TNTs, extracellular vesicles (EVs), gap junction channels (GJCs), cell fusion, and mitochondrial extrusion. This section provides a review of the identified modes of mitochondrial transfer to date.
3.1 Mitochondrial transfer via tunneling nanotubes
In 2004, Rustom et al. observed the presence of TNTs in rat pheochromocytoma PC12 cells using 3D microscopy (16). TNTs are intercellular structures with diameters ranging from 50 to 200 nm. Subsequently, the formation of TNTs between endothelial progenitor cells and cardiomyocytes was demonstrated using MitoTracker labeling (47). Since the discovery of mitochondrial transfer through TNTs in mammalian cells, increasing evidence has emerged regarding their roles in apoptosis and tumorigenesis (Figure 1A). Wang and Gerdes found that mitochondrial transfer via TNTs could rescue apoptosis in UV-treated PC12 cells (48). Co-culturing UV-treated PC12 cells with untreated PC12 cells resulted in the formation of a novel type of TNT, through which mitochondria from healthy cells were transferred to UV-treated PC cells, protecting them from apoptosis (48). In 2022, Yang et al. demonstrated that marrow stromal cells could acquire mitochondria from rotenone-induced mitochondrial dysfunction myeloid cells through TNTs in co-culture systems, preventing mitochondrial dysfunction and apoptosis in recipient myeloid cells (49). Recent research has also revealed that tumor cells can “steal” mitochondria from immune cells through TNTs, highlighting the clinical relevance of this phenomenon (50). As mitochondria contain a separate mtDNA, their transfer through TNTs not only directly influences the metabolism of recipient cells but also affects the mitochondrial genome of the recipient cells. Valdebenito et al. found that TNTs were formed between GBM cells and primary astrocytes under co-culture and stress conditions. Mitochondria from tumor cells were transferred to primary astrocytes, and the transferred mtDNA contained genetic variants that altered the metabolism of the recipient cells (51).
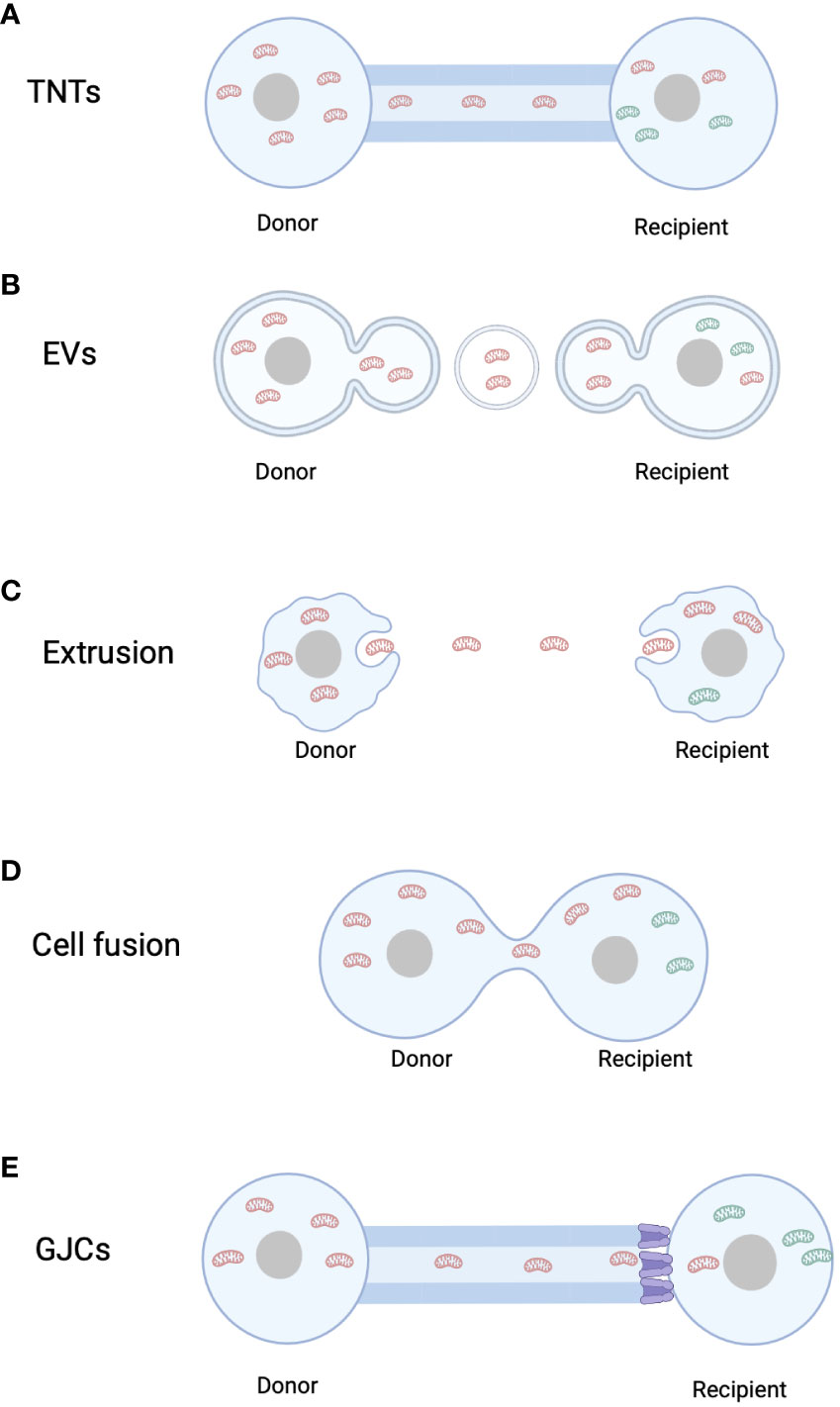
Figure 1 Mitochondrial transfer pathways. (A) Tunnel Nanotubes (TNTs). (B) Extracellular Vesicles (EVs). (C) Mitochondrial Extrusion. (D) Cell fusion. (E) Gap junction channels. Red mitochondria refer to mitochondria originating from the donor cell, whereas green mitochondria denote mitochondria originating from the recipient cell.
3.2 Mitochondrial transfer via extracellular vesicles
Cells can communicate with adjacent or distant cells through the secretion of EVs. EVs can be categorized into two types: microvesicles, which bud from the plasma membrane and have diameters ranging from 100 nm to 1000 nm, and exosomes, which are smaller vesicles (diameter < 150 nm) enriched with nuclear-derived components. While exosomes cannot carry intact mitochondria due to their small size, larger EVs have the capacity to transport complete mitochondria. Once attached to target cells, EVs can induce signal transduction through receptor-ligand interactions and can be internalized through endocytosis and/or phagocytosis or fuse with the target cell membrane, delivering their cargo into the cytoplasm (52). In 2012, Islam et al. demonstrated that bone marrow-derived mesenchymal stem cell (BMSC)-derived EVs can deliver mitochondria to damaged alveolar epithelial cells in a mouse model of lipopolysaccharide-induced acute lung injury, thereby preventing acute lung injury (53). Since then, more evidence of mitochondrial transfer via vesicles has been discovered (Figure 1B). Phinney et al. found that bone marrow-derived mesenchymal stem cells can transfer mitochondria to macrophages through microvesicles (54). Additionally, Peruzzotti-Jametti et al. demonstrated that EVs secreted by neural stem cells can rescue mitochondrial function in L929 Rho0 cells with mtDNA defects and integrate into the mitochondrial network of host inflammatory macrophages (55). Not only stem cells, but various cell types have been shown to transfer mitochondria through EVs to exert various biological functions. Hayakawa et al. found that astrocytes release extracellular mitochondrial particles after a stroke, which enter post-stroke neurons and promote ATP production and neuronal recovery (56). Hough et al. discovered that extracellular vesicles mediate mitochondrial transfer from airway myeloid progenitors to T cells (57). Collectively, these studies suggest that mitochondrial transfer through various types of extracellular vesicles is an effective mechanism for restoring cellular functionality.
3.3 Mitochondrial transfer via mitochondrial extrusion or internalization
In 2008, Nakajima et al. first described mitochondrial extrusion. Mitochondrial extrusion is a unique form of cell death mediated by tumor necrosis factor α, as it is not observed in cell death induced by the genotoxic drug cisplatin. During mitochondrial extrusion, fragmented mitochondria are detached from the cell via cell membrane vesicles, which engulf the mitochondrial fragments. When the membrane enclosing the mitochondria fuses with the cell membrane, the exposed mitochondria are released into the extracellular space, inducing cell death (Figure 1C). Mitochondrial extrusion and membrane blebbing require intact cellular scaffolds composed of actin and microtubules. Under physiological conditions, red blood cells extrude mitochondria during maturation, while under pathological conditions, cytoplasmic vesicles engulf mitochondrial fragments injected into mice with anti-Fas antibodies are extruded from hepatocytes (58). Additionally, Lyamzaev et al. found that mitochondria cluster near the cell nucleus, forming mitochondrial clusters that separate from the cytoplasm through membrane fission, termed “mitoptotic bodies,” which are then released from the cell (59).
Naked mitochondria or mitochondrial components can also be extruded and internalized without a carrier, a process known as blebbing and internalization (60). Kitani et al. demonstrated that isolated mitochondria can be internalized into cells through simple co-incubation using genetically labeled mitochondria. Mitochondrial internalization significantly improved mitochondrial function in cells depleted of mtDNA, and this effect was sustained for several days (61).
3.4 Mitochondrial transfer via cell fusion
While cell fusion can mediate mitochondrial transfer, related research in this area is limited. Cell fusion refers to the process whereby two independent cells merge their cell membranes, share organelles and cytoplasm, while maintaining intact cell nuclei. Permanent cell fusion results in the sharing of cytoplasm and the formation of a unique nuclear configuration, whereas partial cell fusion allows transient cell communication or exchange of organelles, such as mitochondria (23). Adrien et al. demonstrated that human mesenchymal stem cells can reprogram myocytes into an immature state through partial cell fusion and mitochondrial transfer (Figure 1D) (62). Co-culture of cardiac myocytes with human multipotent adipose-derived stem cells allowed partial cell fusion, facilitating the exchange of materials and mitochondria (62).
3.5 Mitochondrial transfer via gap junction channels
Gap junction channels (GJCs) are intercellular channels formed by the docking of two adjacent hemichannels (HCs) located in the plasma membranes of neighboring cells. Each HC is composed of six connexin (Cx) subunits, forming a hollow tubular structure (63). Direct transfer of mitochondria through GJCs is not possible due to the much smaller pore size of GJCs compared to mitochondria (63, 64). However, Alarcon-Martinez et al. discovered interstitial primitive TNTs (IP-TNTs), which consist of both TNTs and a distal Cx43-GJC connecting two recipient cells. Live imaging revealed the presence of mitochondria within IP-TNTs and their movement within these structures (Figure 1E) (65). However, mitochondrial transfer did not occur between recipient cells, indicating that GJCs cannot directly mediate mitochondrial transfer (65). Nonetheless, evidence suggests that GJCs based on Cx43 may play a role in TNT-mediated mitochondrial transfer. Norris used 3D electron microscopy and immunogold labeling of Cx43 to reveal that recipient cell mitochondria can internalize through Cx43-based GJC-mediated endocytosis into double-membrane autophagosome-like GJCs (66). Yao et al. observed that Cx43 in TNTs between induced pluripotent stem cell-derived mesenchymal stem cells (iPSC-MSCs) and BEAS2B cells was crucial for TNT formation, and knocking down Cx43 expression significantly affected TNT formation and reduced mitochondrial transfer between the two cell types (67). In contrast, enhanced Cx43 expression promoted mitochondrial transfer from astrocytes to neurons through TNT-like structures (68). These findings suggest that Cx43-based GJCs may contribute to TNT-mediated mitochondrial transfer.
In summary, while GJCs cannot directly mediate mitochondrial transfer, they may facilitate TNT-mediated mitochondrial transfer.
4 Methods of mitochondrial transplantation
Mitochondria can not only naturally transfer from cell to cell, but they can also be extracted, concentrated, and modified before transplantation into various cells for the treatment of metabolic diseases associated with mitochondrial dysfunction. In 1988, King et al. applied microinjection (Figure 2A) to inject isolated mitochondria containing chloramphenicol-resistant markers encoded by mitochondrial DNA (mtDNA) into human 143BTK- and HT1080-6TG cells, and found evidence of endogenous mitochondrial DNA replacement (69). However, microinjection can damage cells and is less effective than co-culture (70). In 2014, Tomoya Kitani discovered that genetically labeled mitochondria can be transferred to homogeneic and xenogeneic cells through simple co-culture (Figure 2B) (61). However, the internalization of isolated mitochondria into cells is challenging due to their relatively large size and negatively charged surface. To address this issue, Andres Caicedo developed a technique called MitoCeption, which involves slowly adding a suspension of mitochondria to recipient cells in a culture dish, followed by centrifugation and incubation in a culture incubator to enhance the efficiency of mitochondrial transplantation (Figure 2C) (71). However, this method has limitations as direct fusion and transfer between transferred and endogenous mitochondria are not possible, and the transfer efficiency is generally low. To overcome these challenges, Ting-Hsiang Wu and colleagues invented a novel method for transferring isolated mitochondria from donor mammalian cells to recipient cells using a photothermal nanoblade (Figure 2D), which consists of a 532 nm nanosecond pulsed laser, a nanoblade delivery micropipette, and a microscope. Under laser pulsation, pressure drives liquid flow in the micropipette, delivering mitochondria into the recipient cytoplasm (72). As this method requires complex laser and optical systems to operate, the team developed “MitoPunch” based on the technology of photothermal nanoblades, a pressure-driven mitochondrial transfer device that can simultaneously deliver isolated mitochondria to many target mammalian cells. This is a force-based mitochondrial transfer method previously described, which can generate stable isolated mitochondrial recipient (SIMR) cells that retain exogenous mtDNA permanently (Figure 2E). The MitoPunch device is versatile and has low assembly costs, making it a higher-throughput and more user-friendly system (73). While these methods offer high purity, the transplantation efficiency is relatively low. Mi Jin Kim developed a simple centrifugation-based method for mitochondrial transfer that can be applied to any type of cell (Figure 2F) (74). This method successfully transfers isolated mitochondria to target cells (74). Magnetic bead labeled mitochondria with the assistance of magnetic plates have also been found to be a method to improve the efficiency of mitochondrial transfer (Figure 2G) (75). Other substances have been found to facilitate the uptake of mitochondria by cells, such as cell-penetrating peptides Pep-1 (Figure 2H), biocompatible polymers (dextran‐triphenylphosphonium), which promote the uptake of mitochondria by recipient cells (76, 77). SS31, a novel cell-permeable antioxidant peptide, shows the ability to effectively mitigate and decrease the production of reactive oxygen species within isolated mitochondria (78). Furthermore, research finding indicates that doxycycline, an antibiotic drug, exhibit the potential to augment mitochondrial transfer within cancer cells, thereby facilitating the restoration of mitochondrial function (79).
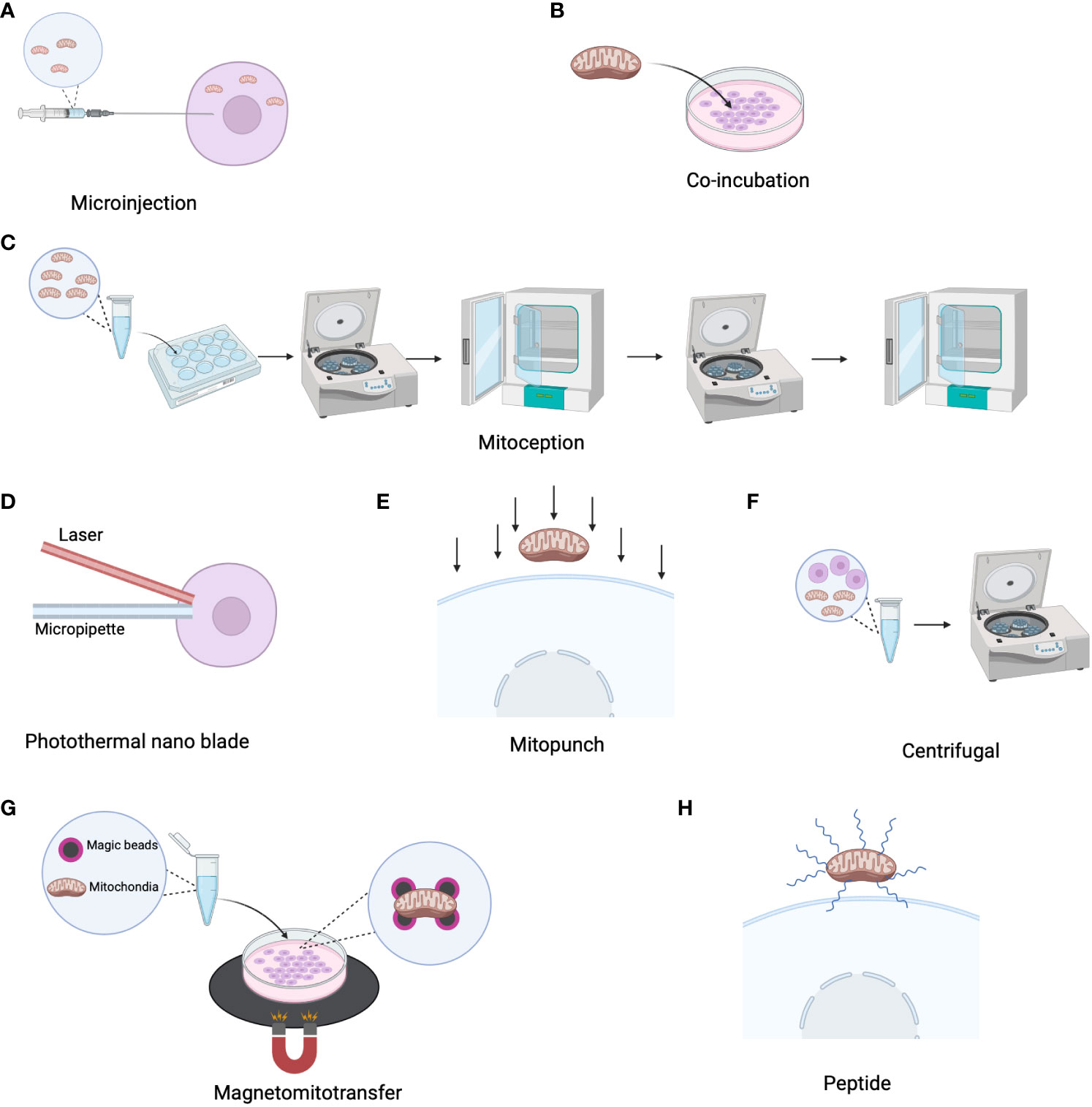
Figure 2 Mitochondrial transmission strategy. (A) Transfer isolated mitochondria to recipient cells via microinjection. (B) Co-incubate isolated mitochondria with recipient cells to facilitate mitochondria transfer. (C) Mitoception: Add mitochondrial suspension to the entire culture surface, followed by centrifugation of the culture plate at 1500 g for 15 minutes at 4°C. Subsequently, place them in a 37°C cell culture incubator and perform a second centrifugation under the same conditions after two hours. Incubate in the incubator for 24 hours. (D) Photo-nanotube: Employ laser pulses to induce pressure-driven liquid flow in the micropipette, facilitating the delivery of mitochondria into the recipient cytoplasm. (E) Mitopunch: Utilize a pressure-driven mitochondrial transfer device. (F) Centrifugal: Prepare mitochondria by centrifuging at 1500 g for 5 minutes without requiring additional incubation. (G) Magnetomitotransfer: Perform mitochondrial transfer of magnetically labeled mitochondria with the aid of magnetic plates. (H) Enhance mitochondrial transfer through peptide encapsulation on the mitochondrial surface.
5 Therapeutic potential of mitochondrial transfer in metabolic diseases
Cell therapy, as an emerging therapeutic approach, can be applied to the treatment of various diseases, including cancer, immune system disorders, and genetic diseases. However, the immunological rejection of xenogeneic cells severely limits their application. Many metabolic diseases are closely associated with mitochondrial dysfunction or mutations in mitochondrial DNA. Moreover, compared to cell therapy, mitochondria have lower immunogenicity, making them a potential tool for the treatment of various diseases through mitochondrial transfer. Clinical trials have demonstrated the application of mitochondrial transplantation in cardiovascular treatment. Pediatric patients, susceptible to myocardial ischemia-reperfusion injury following surgery, benefit from the direct injection of autologous mitochondria into the ischemic myocardium. These transplanted mitochondria enhance recovery and cell viability for up to 28 days without triggering adverse immune reactions (80). Retrospective studies involving pediatric patients with Ischemia-Reperfusion Injury (IRI) showcase the effectiveness of mitochondrial transplantation in reducing recovery duration and mitigating cardiovascular incidents (81). Furthermore, mitochondrial transplantation shows promise in addressing infertility and contributing to advancements in reproductive medicine. Despite the absence of conducted clinical trials for mitochondrial transplantation in metabolic diseases, numerous in vivo and in vitro experiments highlight its potential in treating such disorders (Figure 3).
5.1 Applications of mitochondrial transfer in the treatment of diabetes and its complications
Complications of diabetes, including cardiovascular disease, neuropathy, nephropathy, and retinopathy, have been extensively documented (82–85). The utilization of mitochondria for the treatment of diabetes has also been widely explored. Rackham et al. found that under co-culture conditions, adipose-derived mesenchymal stem cells can transfer mitochondria to human pancreatic beta cells, thereby enhancing their insulin secretion function (86).
Mitochondrial dysfunction caused by T2DM increases the susceptibility of the myocardium to ischemia-reperfusion injury. Doulamis et al. studied the therapeutic effect of mitochondria isolated from diabetic or non-diabetic rats on ischemia-reperfusion injury in the hearts of diabetic rats. The results showed that mitochondria derived from diabetic rats produced significantly less ATP than those from non-diabetic rats. Furthermore, the study found that mitochondrial transplantation significantly enhanced post-ischemic myocardial function recovery and significantly reduced cardiomyocyte damage in diabetic hearts (87). A similar conclusion was drawn from another study where offspring rats exposed to pre-gestational diabetes and a high-fat diet displayed cardiac dysfunction, mitochondrial dysfunction, and compromised cellular bioenergetics. The extracellular transplantation of healthy mitochondria into cardiac cells of these offspring rats significantly improved cellular respiration in male rats and reduced apoptosis, but increased apoptosis in female rats (88).
Diabetic nephropathy (DN) is a common complication of T2DM that reduces the quality of life of patients. Yuan et al. found that mitochondrial transfer from mesenchymal stem cells (MSCs) to macrophages (Mφ) can inhibit inflammation in the kidneys of diabetic nephropathy mice by activating PGC-1. Under co-culture conditions of MSCs and Mφ, mitochondria from MSCs (MSCs-Mito) were transferred to Mφ, improving mitochondrial function in Mφ and alleviating kidney damage in DN mice (89).
Diabetes-associated cognitive impairment (DACI) poses a risk to patient health and increases the risk of major cardiovascular events, cardiovascular death, and all-cause mortality (90). Ma et al. evaluated the cognitive behavior of db/db mice using the Morris water maze test to investigate whether transplantation of platelet-derived mitochondria (Mito-Plt) could improve DACI. The results showed that Mito-Plt injected into the lateral ventricle were internalized into hippocampal neurons. One month after Mito-Plt transplantation, DACI in db/db mice was alleviated, mitochondrial quantity increased, mitochondrial function recovered, oxidative stress and neuronal apoptosis were reduced, and the accumulation of Aβ and Tau in the hippocampus was decreased. In conclusion, Mito-Plt transplantation alleviated cognitive impairment and mitochondrial dysfunction in db/db mice. This method may have potential applications in the treatment of DACI (91).
The above evidence suggests that mitochondrial transfer has therapeutic effects in diabetes and its complications including diabetic nephropathy, diabetic cardiomyopathy, and diabetes-associated cognitive impairment.
5.2 Application of mitochondrial transfer in the treatment of NAFLD
In 2017, Fu et al. reported the utilization of isolated mitochondria from liver cancer cells as a therapeutic agent for treating high-fat diet-induced fatty liver in mice. After intravenous injection of mitochondria into mice, serum transaminase activity and cholesterol levels decreased in a dose-dependent manner. Furthermore, this mitochondrial treatment reduced lipid accumulation and oxidative damage in the livers of fatty liver mice, improving energy production in liver cells and restoring liver function. This treatment strategy provides a potential new approach for the treatment of NAFLD (92). Similarly, Paliwal et al. used mitochondria isolated from normal rat chest muscles to treat metabolic syndrome rats induced by a high-fat diet (HFD) and streptozotocin (STZ). The results showed that mitochondrial transplantation reduced the levels of systolic and diastolic blood pressure, decreased blood glucose levels, and significantly decreased blood lipid levels in metabolic syndrome rats. Histopathological analysis showed improvements in alanine aminotransferase (ALT) and aspartate aminotransferase (AST) levels, and significant restoration of liver morphology. In addition, enhanced mitochondrial biogenesis, reduced oxidative stress and inflammatory markers were observed (93). Hsu et al. demonstrated that bone marrow-derived mesenchymal stem cells (MSCs) can also treat non-alcoholic steatohepatitis (NASH). They transplanted bone marrow-derived MSCs into the livers of a non-alcoholic steatohepatitis mouse model, resulting in improvements in liver lipid content, tissue inflammation, and fibrosis. Co-culture of liver cells and MSCs revealed that mitochondria were transferred from MSCs to liver cells through TNTs. Therefore, MSCs can contribute to lipid breakdown in liver cells by providing oxidative capacity through the donation of mitochondria to liver cells, promoting the recovery of metabolic and tissue damage induced by NASH (94). Furthermore, Fu and colleagues injected isolated mitochondria from human hepatocellular carcinoma (Hep G2 cells) into the livers of fatty liver mice and found that this treatment improved liver energy production, reduced liver lipid accumulation and oxidative damage, and restored liver cell activity (92). Therefore, mitochondrial transfer may be a promising approach for the treatment of NAFLD.
5.3 Applications of mitochondrial transfer in the treatment of obesity
To date, there have been no reports on the use of mitochondrial transfer for the treatment of obesity, but studies have shown a relationship between obesity and mitochondrial transfer. Clemente-Postigo et al. found that pro-inflammatory cytokines such as interferon (IFN)-γ and lipopolysaccharide (LPS) activate macrophages toward an M1 polarization in white adipose tissue (WAT) of obese patients, while anti-inflammatory cytokines such as interleukin (IL)-4 and IL-13 drive macrophages toward an M2 polarization (95). Brestoff et al. also found that macrophages polarized to an M1 phenotype when treated with IFN-γ and LPS, resulting in reduced expression of the heparan sulfate (HS) biosynthetic genes in BV2 cells and impaired mitochondrial transfer from adipocytes to macrophages. Furthermore, in obese patients induced by a high-fat diet (HFD), both M1 and M2 macrophage populations showed reduced mitochondrial transfer (42). These studies suggest that reduced mitochondrial transfer from adipocytes to macrophages is a feature of obesity, and therefore, mitochondrial transfer may be a potential approach for the treatment of obesity.
6 Challenges
Although mitochondrial transplantation holds great potential, there are several challenges that hinder its clinical application. First, mitochondria possess their own DNA, which can be inherited through cytoplasmic transmission, raising safety concerns. During preclinical assessments of mitochondrial donation, a significant safety concern is the gradual increase in initial low levels of “mtDNA carryover” that co-transmits with the nuclear genome during mitochondrial transplantation, potentially increasing during pregnancy and posing a risk of severe mtDNA disease in offspring (96–98).
Second, xenogeneic mitochondrial transplantation raises concerns about immunological reactions, although the reaction is relatively low compared to cell transplantation. Xenogeneic mitochondria can activate innate immunity, with pattern recognition receptors (such as Toll-like receptors and Nod-like receptors) recognizing damage-associated molecular patterns (DAMPs) (99, 100). Transplantation of xenogeneic mitochondria into various tissues has been associated with increased markers of self-immunity and inflammation (101). Lin et al. further elucidated that mitochondrial stimulation directly triggers inflammatory responses in endothelial cells, leading to adhesion and activation of alloreactive T cells, ultimately increasing the risk of alloreactive transplantation rejection (102). Autologous and transplanted mitochondria may also interact with each other.
Next, it is crucial to take into account the potential impact of the isolation procedure on the structure and functionality of mitochondria obtained from cells or tissues. Research has demonstrated that this process elevates mitochondrial stress levels and promotes the generation of free radicals (103). Additionally, the isolation procedure may lead to the disruption of the outer mitochondrial membrane, facilitating the entry of cytochrome C into the buffer solution. Consequently, this disruption hampers oxygen consumption and ATP production (104). Hence, it is imperative to conduct various assays, including the utilization of fluorescent probes and electron microscopy, to thoroughly evaluate mitochondrial integrity and function prior to engaging in mitochondria transplantation (105–107).
Moreover, the efficiency of mitochondrial transplantation is often suboptimal. Despite the emergence of various methods to improve transfer efficiency, mitochondrial function is still affected. The commonly used differential centrifugation-based method suffers from low purity and is time-consuming (60, 108). However, recent advantages have introduced techniques like MitoPunch, which can simultaneously transfer mitochondria to 100,000 or more recipient cells, significantly improving the throughput and efficiency compared to existing methods (71). Continued advancements in techniques will increase the efficiency of future mitochondrial transplantation and make this approach more viable.
Additionally, the storage of mitochondria remains a challenge (109). Isolated mitochondria can maintain activity for 1-2 hours on ice. However, even with the use of preservatives, the integrity of the mitochondrial outer membrane is damaged when stored at -80°C (110). While storing mitochondria with cryoprotectants like DMSO and trehalose preserves outer membrane integrity, it impairs mitochondrial function (111, 112). Therefore, it is crucial to develop storage methods that maintain the stability and functionality of mitochondria (108).
Another consideration is whether transplanted mitochondria can effectively engage with other cellular organelles to ensure their functionality. Notably, previous studies have provided evidence that upon transplantation, exogenous mitochondria undergo fusion with endogenous counterparts (113). This transplantation process facilitates the integration of transferred mitochondria into the mitochondrial network of the recipient cell. Consequently, there exists the potential for transplanted mitochondria to establish functional associations with other organelles within the recipient cells (114).
Addressing these challenges will pave the way for the realization of mitochondrial transplantation as a viable therapeutic approach for treating various diseases including metabolic diseases.
7 Conclusion
This article provides a comprehensive review of the relationship between metabolic diseases and mitochondria, as well as the role of mitochondrial transfer in their treatment. However, despite the well-established association between mitochondrial dysfunction and metabolic diseases, there is a scarcity of studies exploring the therapeutic potential of mitochondrial transplantation or transfer. Most studies focus primarily on common diseases such as diabetes and NAFLD, leaving other metabolic diseases with limited research. In addition, most of the studies on mitochondrial transfer have been conducted in animal models, and their translation into clinical applications is yet to be realized.
Mitochondria exhibit low immunogenicity, offer convenient accessibility, and present minimal ethical concerns. As a result, employing mitochondria for the treatment of metabolic diseases holds remarkable advantages. Despite the limited number of clinical trials on mitochondrial therapy (115), advancements in technology hold immense potential for its extensive application, particularly in the treatment of metabolic disorders.
Author contributions
RC: Writing – original draft. JC: Writing – original draft, Conceptualization, Funding acquisition, Supervision, Writing – review & editing.
Funding
The author(s) declare financial support was received for the research, authorship, and/or publication of this article. This work was supported by the Shandong Excellent Young Scientists Fund Program (Overseas, 2022HWYQ-027), and the Special Foundation for Taishan Scholars (tsqn202312331).
Acknowledgments
Cartoon graphics were created in BioRender.com.
Conflict of interest
The authors declare that the research was conducted in the absence of any commercial or financial relationships that could be construed as a potential conflict of interest.
Publisher’s note
All claims expressed in this article are solely those of the authors and do not necessarily represent those of their affiliated organizations, or those of the publisher, the editors and the reviewers. Any product that may be evaluated in this article, or claim that may be made by its manufacturer, is not guaranteed or endorsed by the publisher.
References
1. Hotamisligil GS. Endoplasmic reticulum stress and the inflammatory basis of metabolic disease. Cell (2010) 140(6):900–17. doi: 10.1016/j.cell.2010.02.034
2. Costello KR, Schones DE. Chromatin modifications in metabolic disease: Potential mediators of long-term disease risk. Wiley Interdiscip Reviews: Syst Biol Med (2018) 10(4):e1416. doi: 10.1002/wsbm.1416
3. International diabetes federation. In: IDF Diabetes Atlas, 10th edn. Brussels, Belgium: International Diabetes Federation. Available at: https://diabetesatlas.org/atlas/tenth-edition/.
4. Byrne CD, Targher G. NAFLD: a multisystem disease. J Hepatol (2015) 62(1):S47–64. doi: 10.1016/j.jhep.2014.12.012
5. Friedman SL, Neuschwander-Tetri BA, Rinella M, Sanyal AJ. Mechanisms of NAFLD development and therapeutic strategies. Nat Med (2018) 24(7):908–22. doi: 10.1038/s41591-018-0104-9
6. Younossi Z, Anstee QM, Marietti M, Hardy T, Henry L, Eslam M, et al. Global burden of NAFLD and NASH: trends, predictions, risk factors and prevention. Nat Rev Gastroenterol Hepatol (2018) 15(1):11–20. doi: 10.1038/nrgastro.2017.109
7. Bharath LP, Agrawal M, McCambridge G, Nicholas DA, Hasturk H, Liu J, et al. Metformin enhances autophagy and normalizes mitochondrial function to alleviate aging-associated inflammation. Cell Metab (2020) 32(1):44–55.e6. doi: 10.1016/j.cmet.2020.04.015
8. Tiku V, Tan M-W, Dikic I. Mitochondrial functions in infection and immunity. Trends Cell Biol (2020) 30(4):263–75. doi: 10.1016/j.tcb.2020.01.006
9. Jiao H, Jiang D, Hu X, Du W, Ji L, Yang Y, et al. Mitocytosis, a migrasome-mediated mitochondrial quality-control process. Cell (2021) 184(11):2896–2910.e13. doi: 10.1016/j.cell.2021.04.027
10. Osellame LD, Blacker TS, Duchen MR. Cellular and molecular mechanisms of mitochondrial function. Best Pract Res Clin Endocrinol Metab (2012) 26(6):711–23. doi: 10.1016/j.beem.2012.05.003
11. Whitley B, Engelhart E, Hoppins S. Mitochondrial dynamics and their potential as a therapeutic target. Mitochondrion (2019) 49:269–83. doi: 10.1016/j.mito.2019.06.002
12. Bonora M, Giorgi C, Pinton P. Molecular mechanisms and consequences of mitochondrial permeability transition. Nat Rev Mol Cell Biol (2022) 23(4):266–85. doi: 10.1038/s41580-021-00433-y
13. Sorrentino V, Menzies KJ, Auwerx J. Repairing mitochondrial dysfunction in disease. Annu Rev Pharmacol Toxicol (2018) 58:353–89. doi: 10.1146/annurev-pharmtox-010716-104908
14. Peoples JN, Saraf A, Ghazal N, Pham TT, Kwong JQ. Mitochondrial dysfunction and oxidative stress in heart disease. Exp Mol Med (2019) 51(12):1–13. doi: 10.1038/s12276-019-0355-7
15. Wei PZ, Szeto CC. Mitochondrial dysfunction in diabetic kidney disease. Clin Chim Acta (2019) 496:108–16. doi: 10.1016/j.cca.2019.07.005
16. Rustom A, Saffrich R, Markovic I, Walther P, Gerdes H-H. Nanotubular highways for intercellular organelle transport. Science (2004) 303(5660):1007–10. doi: 10.1126/science.1093133
17. Spees JL, Olson SD, Whitney MJ, Prockop DJ. Mitochondrial transfer between cells can rescue aerobic respiration. Proc Natl Acad Sci (2006) 103(5):1283–8. doi: 10.1073/pnas.0510511103
18. Davis DM, Sowinski S. Membrane nanotubes: dynamic long-distance connections between animal cells. Nat Rev Mol Cell Biol (2008) 9(6):431–6. doi: 10.1038/nrm2399
19. Sherer NM, Mothes W. Cytonemes and tunneling nanotubules in cell-cell communication and viral pathogenesis. Trends Cell Biol (2008) 18(9):414–20. doi: 10.1016/j.tcb.2008.07.003
20. Abounit S, Zurzolo C. Wiring through tunneling nanotubes–from electrical signals to organelle transfer. J Cell Sci (2012) 125(5):1089–98. doi: 10.1242/jcs.083279
21. Zhang J, Zhang Y. Membrane nanotubes: novel communication between distant cells. Sci China Life Sci (2013) 56:994–9. doi: 10.1007/s11427-013-4548-3
22. Rustom A. The missing link: does tunnelling nanotube-based supercellularity provide a new understanding of chronic and lifestyle diseases? Open Biol (2016) 6(6):160057. doi: 10.1098/rsob.160057
23. Torralba D, Baixauli F, Sanchez-Madrid F. Mitochondria know no boundaries: mechanisms and functions of intercellular mitochondrial transfer. Front Cell Dev Biol (2016) 4:107. doi: 10.3389/fcell.2016.00107
24. Liu Z, Sun Y, Qi Z, Cao L, Ding S. Mitochondrial transfer/transplantation: an emerging therapeutic approach for multiple diseases. Cell Biosci (2022) 12(1):66. doi: 10.1186/s13578-022-00805-7
25. Clemente-Suárez VJ, Martín-Rodríguez A, Yáñez-Sepúlveda R, Tornero-Aguilera JF. Mitochondrial transfer as a novel therapeutic approach in disease diagnosis and treatment. Int J Mol Sci (2023) 24(10):8848. doi: 10.3390/ijms24108848
26. Szendroedi J, Phielix E, Roden M. The role of mitochondria in insulin resistance and type 2 diabetes mellitus. Nat Rev Endocrinol (2012) 8(2):92–103. doi: 10.1038/nrendo.2011.138
27. Liang H, Ward WF. PGC-1α: a key regulator of energy metabolism. Adv Physiol Educ (2006) 30(4):145–51. doi: 10.1152/advan.00052.2006
28. Sharma K. Mitochondrial hormesis and diabetic complications. Diabetes (2015) 64(3):663–72. doi: 10.2337/db14-0874
29. Mootha VK, Lindgren CM, Eriksson K-F, Subramanian A, Sihag S, Lehar J, et al. PGC-1α-responsive genes involved in oxidative phosphorylation are coordinately downregulated in human diabetes. Nat Genet (2003) 34(3):267–73. doi: 10.1038/ng1180
30. Nishikawa T, Edelstein D, Du XL, Yamagishi S-i, Matsumura T, Kaneda Y, et al. Normalizing mitochondrial superoxide production blocks three pathways of hyperglycaemic damage. Nature (2000) 404(6779):787–90. doi: 10.1038/35008121
31. Civitarese AE, Ravussin E. Minireview: mitochondrial energetics and insulin resistance. Endocrinology (2008) 149(3):950–4. doi: 10.1210/en.2007-1444
32. Pessayre D, Berson A, Fromenty B, Mansouri A. Mitochondria in steatohepatitis. In: Seminars in liver disease. 333 Seventh Avenue, New: Copyright© 2001 by Thieme Medical Publishers, Inc. (2001).
33. Arruda AP, Pers BM, Parlakgül G, Güney E, Inouye K, Hotamisligil GS, et al. Chronic enrichment of hepatic ER-mitochondria contact sites leads to calcium dependent mitochondrial dysfunction in obesity. Nat Med (2014) 20(12):1427. doi: 10.1038/nm.3735
34. Liesa M, Shirihai OS. Mitochondrial dynamics in the regulation of nutrient utilization and energy expenditure. Cell Metab (2013) 17(4):491–506. doi: 10.1016/j.cmet.2013.03.002
35. Wikstrom JD, Mahdaviani K, Liesa M, Sereda SB, Si Y, Las G, et al. Hormone-induced mitochondrial fission is utilized by brown adipocytes as an amplification pathway for energy expenditure. EMBO J (2014) 33(5):418–36. doi: 10.1002/embj.201385014
36. Sebastián D, Hernández-Alvarez MI, Segalés J, Sorianello E, Muñoz JP, Sala D, et al. Mitofusin 2 (Mfn2) links mitochondrial and endoplasmic reticulum function with insulin signaling and is essential for normal glucose homeostasis. Proc Natl Acad Sci (2012) 109(14):5523–8. doi: 10.1073/pnas.1108220109
37. Gonzalez-Rodriguez A, Mayoral R, Agra N, Valdecantos M, Pardo V, Miquilena-Colina M, et al. Impaired autophagic flux is associated with increased endoplasmic reticulum stress during the development of NAFLD. Cell Death Dis (2014) 5(4):e1179–9. doi: 10.1038/cddis.2014.162
38. Edmunds LR, Xie B, Mills AM, Huckestein BR, Undamatla R, Murali A, et al. Liver-specific Prkn knockout mice are more susceptible to diet-induced hepatic steatosis and insulin resistance. Mol Metab (2020) 41:101051. doi: 10.1016/j.molmet.2020.101051
39. Shum M, Ngo J, Shirihai OS, Liesa M. Mitochondrial oxidative function in NAFLD: Friend or foe? Mol Metab (2021) 50:101134. doi: 10.1016/j.molmet.2020.101134
40. Bhatti JS, Bhatti GK, Reddy PH. Mitochondrial dysfunction and oxidative stress in metabolic disorders—A step towards mitochondria based therapeutic strategies. Biochim Biophys Acta (BBA)-Molecular Basis Dis (2017) 1863(5):1066–77. doi: 10.1016/j.bbadis.2016.11.010
41. Forner F, Kumar C, Luber CA, Fromme T, Klingenspor M, Mann M. Proteome differences between brown and white fat mitochondria reveal specialized metabolic functions. Cell Metab (2009) 10(4):324–35. doi: 10.1016/j.cmet.2009.08.014
42. Brestoff JR, Wilen CB, Moley JR, Li Y, Zou W, Malvin NP, et al. Intercellular mitochondria transfer to macrophages regulates white adipose tissue homeostasis and is impaired in obesity. Cell Metab (2021) 33(2):270–282.e8. doi: 10.1016/j.cmet.2020.11.008
43. de Mello AH, Costa AB, Engel JDG, Rezin GT. Mitochondrial dysfunction in obesity. Life Sci (2018) 192:26–32. doi: 10.1016/j.lfs.2017.11.019
44. Boudina S, Graham TE. Mitochondrial function/dysfunction in white adipose tissue. Exp Physiol (2014) 99(9):1168–78. doi: 10.1113/expphysiol.2014.081414
45. Cedikova M, Kripnerová M, Dvorakova J, Pitule P, Grundmanova M, Babuska V, et al. Mitochondria in white, brown, and beige adipocytes. Stem Cells Int (2016) 2016. doi: 10.1155/2016/6067349
46. An YA, Crewe C, Asterholm IW, Sun K, Chen S, Zhang F, et al. Dysregulation of amyloid precursor protein impairs adipose tissue mitochondrial function and promotes obesity. Nat Metab (2019) 1(12):1243–57. doi: 10.1038/s42255-019-0149-1
47. Koyanagi M, Brandes RP, Haendeler J, Zeiher AM, Dimmeler S. Cell-to-cell connection of endothelial progenitor cells with cardiac myocytes by nanotubes: a novel mechanism for cell fate changes? Circ Res (2005) 96(10):1039–41. doi: 10.1161/01.RES.0000168650.23479.0c
48. Wang X, Gerdes H-H. Transfer of mitochondria via tunneling nanotubes rescues apoptotic PC12 cells. Cell Death Differentiation (2015) 22(7):1181–91. doi: 10.1038/cdd.2014.211
49. Yang F, Zhang Y, Liu S, Xiao J, He Y, Shao Z, et al. Tunneling nanotube-mediated mitochondrial transfer rescues nucleus pulposus cells from mitochondrial dysfunction and apoptosis. Oxid Med Cell Longevity (2022) 2022. doi: 10.1155/2022/3613319
50. Saha T, Dash C, Jayabalan R, Khiste S, Kulkarni A, Kurmi K, et al. Intercellular nanotubes mediate mitochondrial trafficking between cancer and immune cells. Nat nanotechnology (2022) 17(1):98–106. doi: 10.1038/s41565-021-01000-4
51. Valdebenito S, Malik S, Luu R, Loudig O, Mitchell M, Okafo G, et al. Tunneling nanotubes, TNT, communicate glioblastoma with surrounding non-tumor astrocytes to adapt them to hypoxic and metabolic tumor conditions. Sci Rep (2021) 11(1):14556. doi: 10.1038/s41598-021-93775-8
52. Tkach M, Théry C. Communication by extracellular vesicles: where we are and where we need to go. Cell (2016) 164(6):1226–32. doi: 10.1016/j.cell.2016.01.043
53. Islam MN, Das SR, Emin MT, Wei M, Sun L, Westphalen K, et al. Mitochondrial transfer from bone-marrow–derived stromal cells to pulmonary alveoli protects against acute lung injury. Nat Med (2012) 18(5):759–65. doi: 10.1038/nm.2736
54. Phinney DG, Di Giuseppe M, Njah J, Sala E, Shiva S, St Croix CM, et al. Mesenchymal stem cells use extracellular vesicles to outsource mitophagy and shuttle microRNAs. Nat Commun (2015) 6(1):8472. doi: 10.1038/ncomms9472
55. Peruzzotti-Jametti L, Bernstock JD, Willis CM, Manferrari G, Rogall R, Fernandez-Vizarra E, et al. Neural stem cells traffic functional mitochondria via extracellular vesicles. PloS Biol (2021) 19(4):e3001166. doi: 10.1371/journal.pbio.3001166
56. Hayakawa K, Esposito E, Wang X, Terasaki Y, Liu Y, Xing C, et al. Transfer of mitochondria from astrocytes to neurons after stroke. Nature (2016) 535(7613):551–5. doi: 10.1038/nature18928
57. Hough KP, Trevor JL, Strenkowski JG, Wang Y, Chacko BK, Tousif S, et al. Exosomal transfer of mitochondria from airway myeloid-derived regulatory cells to T cells. Redox Biol (2018) 18:54–64. doi: 10.1016/j.redox.2018.06.009
58. Nakajima A, Kurihara H, Yagita H, Okumura K, Nakano H. Mitochondrial extrusion through the cytoplasmic vacuoles during cell death. J Biol Chem (2008) 283(35):24128–35. doi: 10.1074/jbc.M802996200
59. Lyamzaev KG, Nepryakhina OK, Saprunova VB, Bakeeva LE, Pletjushkina OY, Chernyak BV, et al. Novel mechanism of elimination of malfunctioning mitochondria (mitoptosis): formation of mitoptotic bodies and extrusion of mitochondrial material from the cell. Biochim Biophys Acta (BBA)-Bioenergetics (2008) 1777(7-8):817–25. doi: 10.1016/j.bbabio.2008.03.027
60. Liu D, Gao Y, Liu J, Huang Y, Yin J, Feng Y, et al. Intercellular mitochondrial transfer as a means of tissue revitalization. Signal transduction targeted Ther (2021) 6(1):65. doi: 10.1038/s41392-020-00440-z
61. Kitani T, Kami D, Matoba S, Gojo S. Internalization of isolated functional mitochondria: involvement of macropinocytosis. J Cell Mol Med (2014) 18(8):1694–703. doi: 10.1111/jcmm.12316
62. Acquistapace A, Bru T, Lesault P-F, Figeac F, Coudert AE, Le Coz O, et al. Human mesenchymal stem cells reprogram adult cardiomyocytes toward a progenitor-like state through partial cell fusion and mitochondria transfer. Stem Cells (2011) 29(5):812–24. doi: 10.1002/stem.632
63. Delvaeye T, Vandenabeele P, Bultynck G, Leybaert L, Krysko DV. Therapeutic targeting of connexin channels: new views and challenges. Trends Mol Med (2018) 24(12):1036–53. doi: 10.1016/j.molmed.2018.10.005
64. Caicedo A, Aponte PM, Cabrera F, Hidalgo C, Khoury M. Artificial mitochondria transfer: current challenges, advances, and future applications. Stem Cells Int (2017) 2017. doi: 10.1155/2017/7610414
65. Alarcon-Martinez L, Villafranca-Baughman D, Quintero H, Kacerovsky JB, Dotigny F, Murai KK, et al. Interpericyte tunnelling nanotubes regulate neurovascular coupling. Nature (2020) 585(7823):91–5. doi: 10.1038/s41586-020-2589-x
66. Norris RP. Transfer of mitochondria and endosomes between cells by gap junction internalization. Traffic (2021) 22(6):174–9. doi: 10.1111/tra.12786
67. Yao Y, Fan X-L, Jiang D, Zhang Y, Li X, Xu Z-B, et al. Connexin 43-mediated mitochondrial transfer of iPSC-MSCs alleviates asthma inflammation. Stem Cell Rep (2018) 11(5):1120–35. doi: 10.1016/j.stemcr.2018.09.012
68. Ren D, Zheng P, Zou S, Gong Y, Wang Y, Duan J, et al. GJA1-20K enhances mitochondria transfer from astrocytes to neurons via Cx43-TnTs after traumatic brain injury. Cell Mol Neurobiol (2022) 42(6):1887–95. doi: 10.1007/s10571-021-01070-x
69. King MP, Attardi G. Injection of mitochondria into human cells leads to a rapid replacement of the endogenous mitochondrial DNA. Cell (1988) 52(6):811–9. doi: 10.1016/0092-8674(88)90423-0
70. Pinkert C, Irwin M, Johnson L, Moffatt R. Mitochondria transfer into mouse ova by microinjection. Transgenic Res (1997) 6:379–83. doi: 10.1023/A:1018431316831
71. Caicedo A, Fritz V, Brondello J-M, Ayala M, Dennemont I, Abdellaoui N, et al. MitoCeption as a new tool to assess the effects of mesenchymal stem/stromal cell mitochondria on cancer cell metabolism and function. Sci Rep (2015) 5(1):9073. doi: 10.1038/srep09073
72. Wu T-H, Wu Y-C, Sagullo E, Teitell MA, Chiou P-Y. Direct nuclear delivery of DNA by photothermal nanoblade. J Lab Automation (2015) 20(6):659–62. doi: 10.1177/2211068215583630
73. Sercel AJ, Patananan AN, Man T, Wu T-H, Yu AK, Guyot GW, et al. Stable transplantation of human mitochondrial DNA by high-throughput, pressurized isolated mitochondrial delivery. Elife (2021) 10:e63102. doi: 10.7554/eLife.63102
74. Kim MJ, Hwang JW, Yun C-K, Lee Y, Choi Y-S. Delivery of exogenous mitochondria via centrifugation enhances cellular metabolic function. Sci Rep (2018) 8(1):3330. doi: 10.1038/s41598-018-21539-y
75. Macheiner T, Fengler VHI, Agreiter M, Eisenberg T, Madeo F, Kolb D, et al. Magnetomitotransfer: An efficient way for direct mitochondria transfer into cultured human cells. Sci Rep (2016) 6(1):35571. doi: 10.1038/srep35571
76. Chang J-C, Hoel F, Liu K-H, Wei Y-H, Cheng F-C, Kuo S-J, et al. Peptide-mediated delivery of donor mitochondria improves mitochondrial function and cell viability in human cybrid cells with the MELAS A3243G mutation. Sci Rep (2017) 7(1):10710. doi: 10.1038/s41598-017-10870-5
77. Wu S, Zhang A, Li S, Chatterjee S, Qi R, Segura‐Ibarra V, et al. Polymer functionalization of isolated mitochondria for cellular transplantation and metabolic phenotype alteration. Advanced Sci (2018) 5(3):1700530. doi: 10.1002/advs.201700530
78. Cho S, Szeto HH, Kim E, Kim H, Tolhurst AT, Pinto JT. A novel cell-permeable antioxidant peptide, SS31, attenuates ischemic brain injury by down-regulating CD36. J Biol Chem (2007) 282(7):4634–42. doi: 10.1074/jbc.M609388200
79. Dijk SN, Protasoni M, Elpidorou M, Kroon AM, Taanman J-W. Mitochondria as target to inhibit proliferation and induce apoptosis of cancer cells: the effects of doxycycline and gemcitabine. Sci Rep (2020) 10(1):4363. doi: 10.1038/s41598-020-61381-9
80. Emani SM, McCully JD. Mitochondrial transplantation: applications for pediatric patients with congenital heart disease. Trans Pediatr (2018) 7(2):169. doi: 10.21037/tp.2018.02.02
81. Guariento A, Piekarski BL, Doulamis IP, Blitzer D, Ferraro AM, Harrild DM, et al. Autologous mitochondrial transplantation for cardiogenic shock in pediatric patients following ischemia-reperfusion injury. J Thorac Cardiovasc Surg (2021) 162(3):992–1001. doi: 10.1016/j.jtcvs.2020.10.151
82. Tang SC, Yiu WH. Innate immunity in diabetic kidney disease. Nat Rev Nephrol (2020) 16(4):206–22. doi: 10.1038/s41581-019-0234-4
83. Rodríguez ML, Millán I, Ortega ÁL. Cellular targets in diabetic retinopathy therapy. World J Diabetes (2021) 12(9):1442. doi: 10.4239/wjd.v12.i9.1442
84. De Geest B, Mishra M. Role of oxidative stress in diabetic cardiomyopathy. Antioxidants (2022) 11(4):784. doi: 10.3390/antiox11040784
85. Ramesh P, Yeo JL, Brady EM, McCann GP. Role of inflammation in diabetic cardiomyopathy. Ther Adv Endocrinol Metab (2022) 13:20420188221083530. doi: 10.1177/20420188221083530
86. Rackham CL, Hubber EL, Czajka A, Malik AN, King AJ, Jones PM. Optimizing beta cell function through mesenchymal stromal cell-mediated mitochondria transfer. Stem Cells (2020) 38(4):574–84. doi: 10.1002/stem.3134
87. Doulamis IP, Guariento A, Duignan T, Orfany A, Kido T, Zurakowski D, et al. Mitochondrial transplantation for myocardial protection in diabetic hearts. Eur J Cardio-Thoracic Surg (2020) 57(5):836–45. doi: 10.1093/ejcts/ezz326
88. Louwagie EJ, Larsen TD, Wachal AL, Gandy TC, Baack ML. Mitochondrial transfer improves cardiomyocyte bioenergetics and viability in male rats exposed to pregestational diabetes. Int J Mol Sci (2021) 22(5):2382. doi: 10.3390/ijms22052382
89. Yuan Y, Yuan L, Li L, Liu F, Liu J, Chen Y, et al. Mitochondrial transfer from mesenchymal stem cells to macrophages restricts inflammation and alleviates kidney injury in diabetic nephropathy mice via PGC-1α activation. Stem Cells (2021) 39(7):913–28. doi: 10.1002/stem.3375
90. De Galan B, Zoungas S, Chalmers J, Anderson C, Dufouil C, Pillai A, et al. Cognitive function and risks of cardiovascular disease and hypoglycaemia in patients with type 2 diabetes: the Action in Diabetes and Vascular Disease: Preterax and Diamicron Modified Release Controlled Evaluation (ADVANCE) trial. Diabetologia (2009) 52:2328–36. doi: 10.1007/s00125-009-1484-7
91. Ma H, Jiang T, Tang W, Ma Z, Pu K, Xu F, et al. Transplantation of platelet-derived mitochondria alleviates cognitive impairment and mitochondrial dysfunction in db/db mice. Clin Sci (2020) 134(16):2161–75. doi: 10.1042/CS20200530
92. Fu A, Shi X, Zhang H, Fu B. Mitotherapy for fatty liver by intravenous administration of exogenous mitochondria in male mice. Front Pharmacol (2017) 8:241. doi: 10.3389/fphar.2017.00241
93. Paliwal S, Jain S, Mudgal P, Verma K, Paliwal S, Sharma S. Mitochondrial transfer restores impaired liver functions by AMPK/mTOR/PI3K-AKT pathways in metabolic syndrome. Life Sci (2023) 332:122116. doi: 10.1016/j.lfs.2023.122116
94. Hsu M-J, Karkossa I, Schäfer I, Christ M, Kühne H, Schubert K, et al. Mitochondrial transfer by human mesenchymal stromal cells ameliorates hepatocyte lipid load in a mouse model of NASH. Biomedicines (2020) 8(9):350. doi: 10.3390/biomedicines8090350
95. Clemente-Postigo M, Oliva-Olivera W, Coin-Aragüez L, Ramos-Molina B, Giraldez-Perez RM, Lhamyani S, et al. Metabolic endotoxemia promotes adipose dysfunction and inflammation in human obesity. Am J Physiology-Endocrinology Metab (2019) 316(2):E319–32. doi: 10.1152/ajpendo.00277.2018
96. Kang E, Wu J, Gutierrez NM, Koski A, Tippner-Hedges R, Agaronyan K, et al. Mitochondrial replacement in human oocytes carrying pathogenic mitochondrial DNA mutations. Nature (2016) 540(7632):270–5. doi: 10.1038/nature20592
97. Yamada M, Emmanuele V, Sanchez-Quintero MJ, Sun B, Lallos G, Paull D, et al. Genetic drift can compromise mitochondrial replacement by nuclear transfer in human oocytes. Cell Stem Cell (2016) 18(6):749–54. doi: 10.1016/j.stem.2016.04.001
98. Craven L, Murphy J, Turnbull DM, Taylor RW, Gorman GS, McFarland R. Scientific and ethical issues in mitochondrial donation. New bioethics (2018) 24(1):57–73. doi: 10.1080/20502877.2018.1440725
99. Kaczmarek A, Vandenabeele P, Krysko DV. Necroptosis: the release of damage-associated molecular patterns and its physiological relevance. Immunity (2013) 38(2):209–23. doi: 10.1016/j.immuni.2013.02.003
100. Land W, Agostinis P, Gasser S, Garg A, Linkermann A. Transplantation and damage-associated molecular patterns (DAMPs). Am J Transplant (2016) 16(12):3338–61. doi: 10.1111/ajt.13963
101. Pollara J, Edwards RW, Lin L, Bendersky VA, Brennan TV. Circulating mitochondria in deceased organ donors are associated with immune activation and early allograft dysfunction. JCI Insight (2018) 3(15). doi: 10.1172/jci.insight.121622
102. Lin L, Xu H, Bishawi M, Feng F, Samy K, Truskey G, et al. Circulating mitochondria in organ donors promote allograft rejection. Am J Transplant (2019) 19(7):1917–29. doi: 10.1111/ajt.15309
103. Picard M, Taivassalo T, Ritchie D, Wright KJ, Thomas MM, Romestaing C, et al. Mitochondrial structure and function are disrupted by standard isolation methods. PloS One (2011) 6(3):e18317. doi: 10.1371/journal.pone.0018317
104. Lanza IR, Nair KS. Functional assessment of isolated mitochondria in vitro. Methods enzymology (2009) 457:349–72. doi: 10.1016/S0076-6879(09)05020-4
105. McCully JD, Cowan DB, Pacak CA, Toumpoulis IK, Dayalan H, Levitsky S. Injection of isolated mitochondria during early reperfusion for cardioprotection. Am J Physiology-Heart Circulatory Physiol (2009) 296(1):H94–H105. doi: 10.1152/ajpheart.00567.2008
106. Masuzawa A, Black KM, Pacak CA, Ericsson M, Barnett RJ, Drumm C, et al. Transplantation of autologously derived mitochondria protects the heart from ischemia-reperfusion injury. Am J Physiology-Heart Circulatory Physiol (2013) 304(7):H966–82. doi: 10.1152/ajpheart.00883.2012
107. Pacak CA, Preble JM, Kondo H, Seibel P, Levitsky S, Del Nido PJ, et al. Actin-dependent mitochondrial internalization in cardiomyocytes: evidence for rescue of mitochondrial function. Biol Open (2015) 4(5):622–6. doi: 10.1242/bio.201511478
108. D’Amato M, Morra F, Di Meo I, Tiranti V. Mitochondrial transplantation in mitochondrial medicine: current challenges and future perspectives. Int J Mol Sci (2023) 24(3):1969. doi: 10.3390/ijms24031969
109. Zhang T-g, Miao C-y. Mitochondrial transplantation as a promising therapy for mitochondrial diseases. Acta Pharm Sin B (2023) 13(3):1028–35. doi: 10.1016/j.apsb.2022.10.008
110. Yamada Y, Ito M, Arai M, Hibino M, Tsujioka T, Harashima H. Challenges in promoting mitochondrial transplantation therapy. Int J Mol Sci (2020) 21(17):6365. doi: 10.3390/ijms21176365
111. Nukala VN, Singh IN, Davis LM, Sullivan PG. Cryopreservation of brain mitochondria: a novel methodology for functional studies. J Neurosci Methods (2006) 152(1-2):48–54. doi: 10.1016/j.jneumeth.2005.08.017
112. Yamaguchi R, Andreyev A, Murphy A, Perkins G, Ellisman M, Newmeyer D. Mitochondria frozen with trehalose retain a number of biological functions and preserve outer membrane integrity. Cell Death Differentiation (2007) 14(3):616–24. doi: 10.1038/sj.cdd.4402035
113. Cowan DB, Yao R, Thedsanamoorthy JK, Zurakowski D, Del Nido PJ, McCully JD. Transit and integration of extracellular mitochondria in human heart cells. Sci Rep (2017) 7(1):17450. doi: 10.1038/s41598-017-17813-0
114. Gäbelein CG, Feng Q, Sarajlic E, Zambelli T, Guillaume-Gentil O, Kornmann B, et al. Mitochondria transplantation between living cells. PloS Biol (2022) 20(3):e3001576. doi: 10.1371/journal.pbio.3001576
Keywords: mitochondria, mitochondrial transfer, transplantation, metabolic diseases, therapy
Citation: Chen R and Chen J (2024) Mitochondrial transfer - a novel promising approach for the treatment of metabolic diseases. Front. Endocrinol. 14:1346441. doi: 10.3389/fendo.2023.1346441
Received: 29 November 2023; Accepted: 28 December 2023;
Published: 19 January 2024.
Edited by:
Jian Ma, Harbin Medical University, ChinaReviewed by:
Huichao Deng, Howard Hughes Medical Institute (HHMI), United StatesPeiqiang Yan, Harvard Medical School, United States
Huan Li, Columbia University, United States
Copyright © 2024 Chen and Chen. This is an open-access article distributed under the terms of the Creative Commons Attribution License (CC BY). The use, distribution or reproduction in other forums is permitted, provided the original author(s) and the copyright owner(s) are credited and that the original publication in this journal is cited, in accordance with accepted academic practice. No use, distribution or reproduction is permitted which does not comply with these terms.
*Correspondence: Jun Chen, anVuY2hlbjAwMUBvdXRsb29rLmNvbQ==