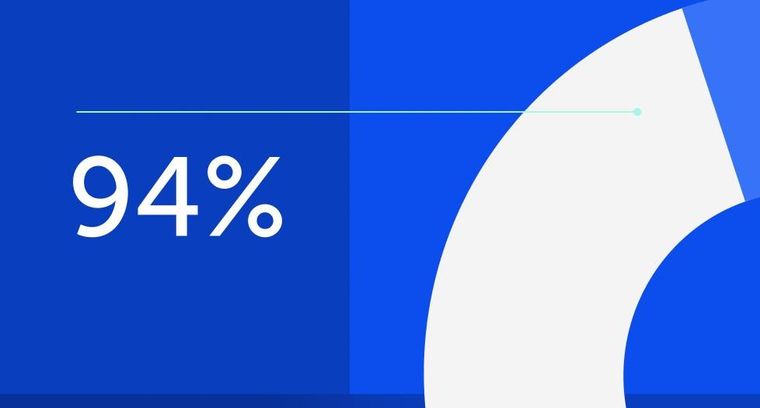
94% of researchers rate our articles as excellent or good
Learn more about the work of our research integrity team to safeguard the quality of each article we publish.
Find out more
REVIEW article
Front. Endocrinol., 18 October 2023
Sec. Obesity
Volume 14 - 2023 | https://doi.org/10.3389/fendo.2023.1280853
This article is part of the Research TopicObesity and Type 2 Diabetes Mellitus: Novel and Alternative Functional Bioactive Nutritional InterventionsView all 12 articles
Intermuscular adipose tissue (IMAT) is a unique adipose depot interspersed between muscle fibers (myofibers) or muscle groups. Numerous studies have shown that IMAT is strongly associated with insulin resistance and muscular dysfunction in people with metabolic disease, such as obesity and type 2 diabetes. Moreover, IMAT aggravates obesity-related muscle metabolism disorders via secretory factors. Interestingly, researchers have discovered that intermuscular brown adipocytes in rodent models provide new hope for obesity treatment by acting on energy dissipation, which inspired researchers to explore the underlying regulation of IMAT formation. However, the molecular and cellular properties and regulatory processes of IMAT remain debated. Previous studies have suggested that muscle-derived stem/progenitor cells and other adipose tissue progenitors contribute to the development of IMAT. Adipocytes within IMAT exhibit features that are similar to either white adipocytes or uncoupling protein 1 (UCP1)-positive brown adipocytes. Additionally, given the heterogeneity of skeletal muscle, which comprises myofibers, satellite cells, and resident mesenchymal progenitors, it is plausible that interplay between these cellular components actively participate in the regulation of intermuscular adipogenesis. In this context, we review recent studies associated with IMAT to offer insights into the cellular origins, biological properties, and regulatory mechanisms of IMAT. Our aim is to provide novel ideas for the therapeutic strategy of IMAT and the development of new drugs targeting IMAT-related metabolic diseases.
Obesity is associated with increased risks for diverse diseases, such as metabolic syndrome, type 2 diabetes, non-alcoholic fatty liver disease, and several cancers (1). Intermuscular adipose tissue (IMAT) is a unique adipose depot that expands between myofibers or adjacent muscle groups, which develops and progresses alongside the expansion of visceral and subcutaneous adipose tissue due to obesity (2). IMAT is distinct from the accumulation of lipids within myofibers, which is referred to as intramuscular lipids or intramyocellular lipids (3, 4). Imaging techniques have been increasingly used to noninvasively quantify IMAT, including computed tomography (CT) and magnetic resonance imaging (MRI) (5–7), and there is a good level agreement between IMAT assessment by MRI and histology (7). Several studies have suggested that IMAT poses a major threat to muscle metabolic disorders and physiological function, such as IR and muscle atrophy, in individuals with obesity, type 2 diabetes, and aging (5, 8, 9). Despite IMAT in the thigh being much less than subcutaneous adipose tissue (SCAT) in obese individuals, it is strongly correlated with IR (5). Additionally, IMAT in thigh muscle is independently associated with increased obesity-related heart failure risk after adjusting for cardiometabolic risk factors and other measurements of adiposity in humans (6). A separate study revealed that obesity-associated respiratory dysfunction in a mouse model was correlated with IMAT and collagen deposition within the diaphragm (10).
In recent years, several studies have suggested that IMAT adipocytes originate from muscle-resident stem/progenitor cells or other mesenchymal progenitors, resulting in the heterogeneity of intermuscular adipocytes with distinct metabolic characteristics (11, 12). For instance, human muscle-derived fibro/adipogenic progenitors (FAPs) in vitro give rise to white adipocytes that exhibit IR (13). Interestingly, one study reported the presence of brown progenitors in human skeletal muscle (14). Other researchers have demonstrated the existence of uncoupling protein 1 (UCP1)+ brown adipocytes within IMAT in mice, providing a therapeutic target for obesity by acting on energy dissipation (15). These findings indicate that there is still ongoing debate regarding the cellular origins and metabolic properties of IMAT adipocytes.
Therefore, within this context, we will review recent studies to explain the cellular origins of IMAT adipocytes and regulatory mechanisms involved in intermuscular adipogenesis. This review aims to provide new insights and potential targets for addressing IMAT-related conditions such as obesity, type 2 diabetes, and related disorders.
Multiple studies have shown that IMAT is a robust predictor of metabolic abnormalities, such as IR, in both younger and older adults (5, 16). Sachs et al. conducted the first direct sampling and analysis of IMAT in humans and they found that the conditioned media for cultivating IMAT obtained from obese individuals reduced the insulin sensitivity of myotube from donors in vitro (17). Similar to other adipose tissue depots, IMAT synthesizes and secretes various bioactive mediators, such as inflammatory cytokines, and extracellular matrix proteins, which can lead to local inflammation or systemic inflammation, ultimately leading to decreased insulin sensitivity in humans (2, 8, 17–19). Furthermore, Sachs et al. discovered that IMAT contains macrophages proportional to insulin sensitivity, and macrophage cytokine secretion within IMAT such as monocyte chemotactic protein 1 (MCP1), is negatively related to insulin sensitivity (17). In obese humans, macrophage and T cells markers were upregulated in skeletal muscle compared with lean humans (20). In addition, macrophages, T cells, and other immune cells that respond to skeletal muscle inflammation are mainly situated in IMAT in diet induced-obese mice (20). These macrophages exhibit polarization toward the proinflammatory M1 phenotype (20, 21), exacerbating skeletal muscle IR and metabolic disorders (Figure 1).
Figure 1 Schematic with potential mechanisms of myocyte inflammation and insulin resistance induced by IMAT (2). IMAT can synthesize and secrete numerous bioactive mediators such as inflammatory cytokines, adipokines, and extracellular matrix proteins to impart adverse effects, such as myocyte inflammation and insulin resistance. Moreover, these secretory inflammatory factors induces the recruitment of immune cells, particularly macrophages, which primarily infiltrate IMAT. IMAT, intermuscular adipose tissue. Created with BioRender.com.
Due to its negative impact on whole-body metabolism, IMAT adipocytes have been extensively studied in vivo and in vitro. Multiple studies have shown that adipocytes derived from muscle-resident mesenchymal progenitors in IMAT share similar characteristics with white adipocytes (9, 10, 13, 22). Liu et al. discovered that muscle-derived non-Pax3 myogenic lineage cells differentiate into white-like adipocytes in vitro (23). Girousse et al. demonstrated that the mobilization of CXCR4+ adipose stromal cells (ASCs) from SCAT toward skeletal muscle results in increased IMAT formation and subsequent impairment of glucose tolerance in mice (12).
Notably, different mouse strains exhibit differential susceptibility to diabetes and diet-induced obesity (15, 24). Almind et al. found that UCP1+ brown adipocytes within IMAT are more prevalent in obesity-resistant 129S6/SvEvTac (Sv129) mice than in C57BL/6 (B6) mice (15). Gorski et al. further found that FAPs provide a likely source for intramuscular adipocytes expressing UCP1 in obesity-resistant Sv129 mice (25). Schulz et al. reported that a subpopulation of adipogenic cells residing in murine skeletal muscle can differentiate in vitro into brown-like adipocytes when stimulated with bone morphogenetic protein 7 (BMP7) (26). Similarly, Crisan et al. demonstrated the presence of brown progenitors in human skeletal muscle that can differentiate into brown adipocytes in vitro, and they also found increased expression of UCP1 mRNA in adult human skeletal muscle, which was further enhanced by PPARγ agonist treatment (14). Additionally, Liu et al. uncovered that transplantation of brown adipose progenitors into mouse skeletal muscles leads to ectopic adipose tissue formation (27). Moreover, induced brown adipose progenitors can develop into brown adipocytes in mouse muscles, resulting in increased energy expenditure (27). Cai et al. demonstrated that transplanted brown adipose tissue (BAT) into the quadriceps femoris muscle of ob/ob mice significantly improved glucose homeostasis, alleviated obesity, and exhibited brown adipocyte characteristics (28), indicating that skeletal muscle could provide a microenvironment for brown adipogenesis.
In summary, despite the detrimental effects of IMAT on metabolism, the presence of brown adipocytes within IMAT offers a potential avenue for treating obesity (Table 1). The skeletal muscle microenvironment provides for maintaining intermuscular brown adipogenesis, offering a promising therapeutic strategy for IMAT-related morbid obesity and diabetes.
Based on previous studies, it has been indicated that muscle-resident stem/progenitor cells and other adipose tissue depot progenitors are potentially involved in the formation of adipocytes within IMAT (11, 12, 15, 35).
Multipotent SCs have the ability to differentiate into adipocytes, ultimately contributing to IMAT formation (29, 30, 40). In the absence of the myogenic transcription factor MyoD/Myf5, myoblasts derived from SCs undergo adipogenic or osteogenic differentiation (29). De Coppi et al. suggested that human SCs marked with CD44+CD56+HLA-ABC+ could differentiate into adipocytes when treated with rosiglitazone in vitro (30). Previous researches based on lineage tracing experiments have indicated that brown adipocytes can arise from Pax7/Myf5-expressing precursors in skeletal muscle (41–43). Seale et al. demonstrated that overexpression of Prdm16 in myoblasts induces their differentiation into brown adipocytes in vitro (42). Yin and colleagues illustrated that the muscle-enriched microRNA-133 represses brown adipogenesis in skeletal muscle by targeting Prdm16 mRNA in mice (43). Furthermore, Pasut et al. discovered that overexpression of the Notch1 intracellular domain (NICD1) in the Pax7-deficient SCs repressed both MyoD and microRNA-133, leading to brown adipocytes formation in regeneration muscle in mice (31). Thus, the regulation of satellite cell-derived brown adipocyte generation, targeting PRDM16 and microRNA-133, presents a crucial therapeutic target for combating obesity.
Muscle-resident mesenchymal progenitors, specifically FAPs, are characterized by positive expression of platelet—derived growth factor receptor alpha (PDGFRα) and stem cell antigen-1 (Sca-1). These cells possess the ability to proliferate and differentiate into adipocytes (10, 11, 32, 34, 39). Camps et al. uncovered the presence of an interstitial CD142− cell subpopulation within the Sca-1+PDGFRα+ population that undergoes adipogenic differentiation in skeletal muscle. They also discovered that the CD142+ cell population could inhibit adipogenesis by secreting growth differentiation factor 10 (GDF10) (35). Arrighi et al. confirmed that the PDGFRα+CD56− muscle progenitors are identical to the CD56−CD15+ progenitors (13). Furthermore, they uncovered that adipocytes derived from FAPs exhibit a deficiency in UCP1 expression in both young and adult donors, and these adipocytes are insulin-resistant (13). Laurens et al. indicated that the CD56−CD15+ cell subpopulation isolated from the muscle of obese subjects differentiated into functional white adipocytes in vitro, which impaired insulin action and myofiber signaling (22). Collectively, the abovementioned studies suggest that FAPs and other PDGFRα+ progenitors have the potential for adipogenic differentiation.
Nonetheless, a subset of myeloid‐derived cells characterized by PDGFRα−CD68+ exhibited adipogenic potential (36). Lu et al. uncovered that PDGFRβ lineage cells from muscles undergo a fate transition, contributing to the infiltration of adipose and fibrotic tissues in old mice (37). Studies found that a population of muscle cells expressing the surface protein CD34 can differentiate into adipocytes in vitro (14, 33). Liu et al. showed that muscle-derived Pax3− non-myogenic lineage cells differentiate into white-like adipocytes without UCP1 expression in vitro (23). These findings suggest that muscle-resident progenitor cells also have the potential for adipogenic differentiation under certain conditions.
In addition to muscle-resident stem/progenitors, adipose stromal cells (ASCs) from SCAT can be released into circulation under the regulation of the chemokine CXCL12 and its receptor CXCR4 in mice (44). Girousse et al. demonstrated that CXCR4+ ASCs released from SCAT, upon exposure to a high-fat diet or CXCR4 antagonist directly promoted ectopic adipocyte formation in the muscle of mice, and subsequently impaired glucose tolerance in mice (12). In addition, one study has shown that there is a reservoir of brown progenitors, that is muscle cells expressing CD34, in human skeletal muscle, which can differentiate into brown adipocyte with a high level of UCP1 in vitro (14). Moreover, induced brown adipose progenitors can develop into brown adipocytes in the limb muscles of mice (27).
Unlike classical adipose tissue depots, IMAT adipocytes exhibit heterogeneity, which may be attributed to their potential stem/progenitor cell origins (Figure 2). The characteristics of adipogenic progenitors of IMAT adipocytes play a crucial role in determining the metabolic traits of adipocytes in IMAT, thereby impacting whole-body metabolism (Table 1).
Figure 2 Schematic diagram of the cellular origins of IMAT adipocytes. IMAT adipocytes can potentially originate from muscle satellite cells, muscle-resident mesenchymal progenitors (specifically FAPs), CXCR4+ ASCs and brown progenitors. IMAT, intermuscular adipose tissue. FAPs, fibro/adipogenic progenitors. ASCs, adipose stromal cells. CXCR4, chemokine CXCL12 receptor.
Similar to classical adipose depots, intermuscular adipogenesis is regulated by a complex transcriptional cascade network that involves CCAAT enhancer-binding protein (C/EBP) family proteins, peroxisome proliferator-activated receptor gamma (PPARγ), sterol regulatory element-binding protein isoform 1c (SREBP1c), and fatty acid-binding protein (FABP4) (45, 46).
Apart from classical transcription regulators, Krüppel-like factor (KLF) family proteins play crucial roles in the differentiation of preadipocytes in livestock animals by interacting with C/EBPs and PPARγ. For instance, KLF4 inhibits the adipogenic differentiation of goat intermuscular preadipocytes in vitro by targeting C/EBPβ (47). KLF2 and KLF9 negatively regulate intermuscular adipogenesis (48, 49). KLF6 was the target gene of miR-22-3p and acted as an “on/off” switch in the differentiation of FAPs into adipocytes or myofibroblasts by regulating the matrix metallopeptidase 14 (MMP14) both in vitro and in vivo (50).
Fibroblast growth factors (FGFs) could also be potent regulators of adipogenesis in skeletal muscle. Basic FGF and FGF1 promote the differentiation of intramuscular adipocytes by regulating the expression of C/EBPα and PPARγ (51, 52). Sebastian et al. found that the conserved FGF2 increased IMAT formation in aged human skeletal muscle by inhibiting the adipogenic inhibitor SPARC (53). FGF21 negatively regulates the adipogenic differentiation of goat intermuscular preadipocytes in vitro by downregulating the expression of PPARγ and regulating the expression of numerous KLFs, including KLF3, 7, 9, 11, 14, and 16 (54).
Previous studies have shown that multiple classical signaling pathways, such as the Hedgehog (Hh), Wnt, and Notch signaling pathways, can regulate IMAT formation in mouse models (55–57). Kopinke et al. demonstrated that Hh signals inhibit adipogenesis by regulating the expression of tissue inhibitors of metalloproteinase 3 (TIMP3) and MMP14 in a mouse model of injury-induced regeneration (55). Furthermore, other researchers uncovered a specific group of FAPs that were marked with glioma-associated oncogene homolog 1 (Gli1), which exhibited elevated Hh signaling and diminished adipogenic capability in a mouse model of muscle injury (58).
Wnt signals can act as a molecular switch controlling adipogenesis (59, 60). It has been suggested that Wnt10b inhibits adipogenesis by inhibiting PPARγ (60). Deceased Wnt10b signaling in myoblasts during aging induced adipose tissue infiltration in muscle (61). Similar results were observed in muscle SCs from obese Zucker rats (62). Reggio et al. identified Wnt5a as a noncanonical Wnt ligand that affects FAP adipogenesis by repressing PPARγ expression in vitro in a β-catenin-dependent manner (57). Brack and colleagues have shown that in a mouse model, the transition from Notch to Wnt signaling in myogenic progenitors is essential for effective muscle regeneration via glycogen synthase kinase 3 beta (GSK3β) (63). These findings potentially elucidate why, despite restoring the proliferative potential of Pax7−/− SCs, NICD1 causes differentiation into brown adipose tissue (31). In addition to its effect on SC fate, Marinkovic et al. observed that myotubes inhibit FAP adipogenesis via Notch signaling in vitro (56). They further demonstrated that synergistic cooperation between Notch and inflammatory signals inhibits adipogenic differentiation in mdx FAPs (56).
In addition, Li et al. found that HMG20A exerts inhibitory effects on adipogenesis in porcine muscle SVFs and C3H10T1/2 cells through its interaction with lysine-specific demethylase 1 (LSD1) (64). Mozzetta et al. found that histone deacetylase inhibitors (HDACis) repressed the adipogenic potential of FAPs and enhanced the myogenic differentiation of SCs in young dystrophic mice but not in old mdx mice (65). Moreover, Wosczyna et al. uncovered that miR-206 repressed the adipogenic differentiation of FAPs by targeting Runx1 to limit intramuscular fatty degeneration in mice injured muscle (66).
To summarize, exploring innovative approaches to modulate the destiny of intermuscular preadipocytes or FAPs to inhibit intermuscular adipogenesis will be beneficial for controlling IMAT formation in pathological conditions.
Skeletal muscle is a complex and plastic tissue, which includes myofibers, SCs, FAPs, immune cells, endothelial cells (67). The interactions between muscle-resident cells and paracrine signals from the microenvironment regulate the expansion and differentiation of adipogenic progenitors, thereby controlling the development of IMAT.
Previous studies demonstrated that the condition of myofibers affects IMAT accumulation (68, 69). Uezumi et al. found that myofibers strongly inhibit the adipogenic differentiation of PDGFRα+ cells in injured muscle in mice (32). Other studies showed variations in the adipogenic potential of preadipocytes in different muscles (23, 70). Liu et al. found that compared to the fast extensor digitorum longus (EDL) muscle, slow soleus (SOL) muscle contains more adipogenic progenitors in mice and these progenitors from SOL exhibits a higher propensity to form adipocytes in vitro (23), with the EDL muscle primarily consisting 80% type IIx and IIb fibers (glycolytic fibers) and the SOL muscle consisting 95% type I and IIa fibers (oxidative fibers) (71). In addition, Gu et al. showed that skeletal muscle-specific overexpression of PPARγ could significantly promote intramuscular fat deposition in the longissimus dorsi muscle but not in the soleus muscle in pigs (72). They further showed that overexpression of PPARγ in porcine muscle promotes the formation of slow oxidative fibers (72). These findings imply that myofiber type plays an important role in regulating intermuscular adipogenesis.
Studies have revealed that skeletal muscle-derived exosomes encapsulate the different myomiRs involved in local skeletal muscle tissue communication (73). They also found that the levels of these myomiRs within exosomes vary between skeletal muscles with different muscle fiber-type compositions (73). Chemello and colleagues showed differential expression profiles of microRNAs such as miR-206 and miR-499 between fast and slow myofibers (74). Wosczyna et al. uncovered that the adipogenic differentiation of FAPs was abrogated by miR-206 by repressing Runx1 translation in mice (66). Jiang et al. suggested that miR-499 hindered SCs adipogenic differentiation by reducing PRDM16 in vitro (75). Based on previous studies, we speculate that myofibers play a regulatory role in intermuscular adipogenesis.
Skeletal muscle, as a secretory organ, secretes bioactive myokines, including myostatin (MSTN), IL-15, irisin and IL-6, which likely exert both local (paracrine) and long-range (endocrine) effects. Studies have highlighted the potential roles of myokines in mediating tissue crosstalk and modulating the process of intermuscular adipogenesis.
MSTN, a member of the TGF-β superfamily, is a secreted protein that is specifically expressed in skeletal muscle, and is associated with myogenesis and adipogenesis in muscle development and regeneration (76, 77). Reisz-Porszasz et al. observed that transgenic mice overexpressing Mstn in skeletal muscle exhibited reduced muscle mass and increased fat mass (78). Lin et al. showed that increased muscle development in Mstn knockout (KO) mice may be associated with reduced adipogenesis (79). Artaza et al. demonstrated that recombinant MSTN promotes the differentiation of C3H10T (1/2) multipotent mesenchymal cells into the adipogenic lineage while inhibiting myogenesis in vitro (76). Additionally, Feldman and colleagues showed that MSTN can serve as a substitute for dexamethasone in inducing adipogenesis in C3H10T (1/2) cells but not in 3T3-L1 preadipocytes, which indicates that MSTN plays a role in promoting adipogenesis in the specific early stage (77). It should be noted that the adipocytes induced by MSTN in cell cultures and transgenic mice revealed the expression of markers associated with immature adipocytes, which exhibit favorable metabolic effects (77). However, inconsistent with previous findings, Liu et al. reported that the activated myostatin/SMAD4 signal promotes the expression of miR-124-3p, and inhibits adipogenesis by downregulating the expression of glucocorticoid receptor (GR) in porcine preadipocytes (80). Sun et al. suggested that MSTN inhibits intramuscular preadipocyte adipogenesis in a dose-dependent manner in vitro (81). Furthermore, they discovered that the culture supernatant from muscle tissue inhibits adipogenic differentiation of intermuscular preadipocytes in vitro. Zhang et al. indicated that MSTN inhibits the adipogenic differentiation of muscle SCs but not adipose-derived stem cells (82). Interestingly, Babcock and colleagues observed that the expression of MSTN and its receptor, activin receptor IIB (actRIIB), varied among different myofiber types in rat (83). They suggested that MSTN and actRIIB expression tends to be higher on IIx and IIb myofibers (I < IIa < IIx < IIb). Thus, depending on the context, MSTN can exhibit a dual role in the regulation of adipogenesis in skeletal muscle, either by inhibiting or promoting it.
IL-15 is a significant factor secreted by muscle fibers. It has been shown to inhibit the differentiation of porcine preadipocytes, specifically in the longissimus dorsi muscle, by suppressing the proliferation of preadipocytes in a dose-dependent manner in vitro (84). Another study revealed that the expression of IL-15 is negatively associated with fatty infiltration in injured human muscle (85). This study also found that IL-15 can stimulate the proliferation of FAPs and prevent the adipogenic differentiation of FAPs in injured muscle in mice (85). In addition, other myokines such as irisin (86, 87), IL-6 (88) and myonectin (89) also play an important role in the regulation of adipogenesis. These myokines are released by skeletal muscle in response to exercise and nutrients, suggesting that they may serve as potential therapeutic options for inhibiting IMAT accumulation.
Dietary supplementation with trace elements, including vitamins and minerals, has the potential to regulate intermuscular adipogenesis by interacting with various regulatory factors.
Previous studies have shown that RA, an active metabolite of vitamin A, is a nutritional regulator of adipose tissue biology (90, 91). Berry et al. found that RA inhibits adipocyte differentiation in vitro by upregulating the expression of the adipogenesis inhibitors Pref-1, Sox9, and KLF2, and suppresses diet-induced obesity in mice (91). Zhao and colleagues demonstrated that RA effectively suppresses adipogenesis of FAPs in a dose-dependent manner in vitro (92). RA supplementation proves to be beneficial for obesity-impaired muscle regeneration by inhibiting both adipogenic and fibrotic differentiation of FAPs in mice (92). However, other researchers showed that neonatal supplementation with vitamin A leads to an increase in intramuscular fat levels without increasing overall fat levels (93). Their findings revealed that RA promotes angiogenesis and increases the number of intramuscular PDGFRα+ adipose progenitors in vivo, which subsequently leads to adipogenesis of intramuscular stromal vascular cells (SVCs) by activating VEGFA/VEGFR2 signaling (93). Therefore, during the early stage of IMAT development, changes in the muscle that impact extracellular matrix remodeling, along with the process of angiogenesis play a critical role (93, 94). Of note, it has also been shown that RA enhances adipocyte formation during the early stage but inhibits adipocyte hypertrophy at the terminal stage (93). While RA signaling inhibits white adipogenesis in murine cells through epigenetically inhibiting Zfp423 expression (95), it tends to downregulate ZFP423 in cattle SVCs, which aligns with the observation that RA downregulates the expression of adipogenic genes C/EBPα and PPARγ (93).
Studies have suggested a close relationship between vitamin D status and fat infiltration in muscle. Gilsanz et al. showed that serum 25-hydroxyvitamin D (25-OHD) levels were negatively correlated with the muscle fat percentage independent of body mass or subcutaneous and visceral fat measured by CT in 90 postpubertal females (96). In a clinical study on elderly individuals, IMAT in thigh muscles was significantly associated with both low vitamin D levels and poor physical performance (97), indicating that vitamin D may impact the deposition of IMAT. Ryan et al. reported that higher physiological concentrations of 1,25-OH2D3 inhibit IMAT formation (98). Supplementation with vitamin D alone or in combination with calcium can inhibit the expression of C-reactive protein (CRP), tumor necrosis factor (TNF)-α, and interleukin (IL)-6 (99), which partially explains the inhibition of IMAT formation in obese individuals. In addition, deficiency in vitamin D is associated with a decrease in the proportion and selective atrophy of type II (fast-twitch) fibers in elderly women (100), potentially altering the local microenvironment of muscles.
Apart from vitamins, the mineral content also influences the biological processes of IMAT formation in animal models (101). Afonso et al. discovered through muscle transcriptome analysis that Cu and Zn may have a negative regulatory effect on intermuscular adipogenesis in groups of Nelore steers (101). Moreover, studies have suggested that an increased iron burden plays a pivotal role in the development of sarcopenia in rats (102). Additionally, transferrin receptor 1 (Tfr1)-mediated iron homeostasis regulates skeletal muscle development, regeneration and metabolism (103–105). Ding et al. revealed that how the specific deletion of Tfr1 in SCs impairs skeletal muscle regeneration with activation of ferroptosis in mice (105), whereas SC-derived myofibers play a critical role in regulating intermuscular adipogenesis and maintaining the skeletal muscle microenvironment.
Currently, the regulatory mechanisms underlying IMAT formation are primarily investigated in domestic animal and rodent models. Accumulating evidence has suggested that the regulation of intermuscular adipogenesis involves an intricate network, involving the proliferation and differentiation of adipogenic precursors, the skeletal muscle microenvironment and nutritional regulators (Figure 3).
Figure 3 Schematic with proposed mechanisms of regulation of intermuscular adipogenesis. The regulation of intermuscular adipogenesis involves an intricate network cascade, which includes transcriptional regulators such as PPARγ, C/EBPs, and KLFs, as well as signaling molecules such as Wnt and Notch. Additionally, trace elements including vitamin A, vitamin D, calcium, and iron are involved. Furthermore, myofibers and myokines contribute to creating an essential microenvironment for intermuscular adipogenesis. IMAT, intermuscular adipose tissue. FAPs, fibro/adipogenic progenitors. C/EBPs, CCAAT enhancer-binding family proteins. PPARγ, peroxisome proliferator-activated receptor gamma. KLFs, Krüppel-like factor family proteins. MSTN, myostatin. Created with BioRender.com.
Due to the detrimental effects of IMAT infiltration in skeletal muscle, clarifying the etiology, quantity and metabolic characteristics of its development is attracting increasing attention. However, the special anatomical location of IMAT limits its accessibility and the ability to conduct in-depth mechanistic studies. Earlier studies have investigated the origin and potential molecular regulatory mechanisms of IMAT adipocytes in livestock and rodent models, offering insights for clinical interventions to mitigate IMAT infiltration. We reviewed previous studies and found that skeletal muscle-resident mesenchymal progenitors, including PDGFRα+/Sca-1+ progenitors, and ASCs from other adipose depots serve as the primary source of IMAT, exhibiting characteristics similar to those of white adipocytes (12, 13, 22). Studies have demonstrated that inhibiting the proliferation and adipogenic differentiation of intramuscular FAPs can effectively impede the formation of intramuscular adipocytes. For instance, modulation of myokines, such as MSTN in the skeletal muscle microenvironment (80, 82, 83), and muscle fiber-derived miR-206, miR-499, can contribute to this inhibition (66, 75). In addition, researchers found that ASC trafficking is regulated by the CXCR4/CXCL12 axis, and pioglitazone intermittent treatment can prevent muscle ectopic fat deposition in high fat diet induced-obese mice (12).
Moreover, human skeletal muscle contains a reservoir of brown progenitors and provides a specialized microenvironment that supports intermuscular brown adipogenesis, which holds promise as a potential therapeutic target for obesity management (14, 27). However, although the expression of UCP1 is increased in vivo through PPARγ agonist treatment, the potential of adipocytes in the IMAT depot to serve as a fuel source for adjacent skeletal muscle remains unexplored in human subjects. Therefore, it will be a major challenge that how to facilitate intermuscular brown adipogenesis rather than white adipogenesis. Lineage tracing experiments have suggested that brown adipocytes in skeletal muscle can be derived from myogenic progenitors by modifying the expression of PRDM16 and miR-133 (42, 43). So it is necessary to investigate the potential molecular mechanisms of the transition from myogenic differentiation to brown adipogenic differentiation. In mouse models, the intermuscular brown adipocytes content was also affected by the species of mice, for example, more intermuscular brown adipocytes in obesity-resistant Sv129 mice than B6 mice (15, 25).
Additionally, in the context of obesity, the inflammatory response induces the recruitment of immune cells, primarily macrophages and T cells, which are predominantly located within the intermuscular adipose tissue. Moreover, macrophages undergo polarization into the proinflammatory M1 phenotype. Further research into the characteristics and potential molecular mechanisms of inflammatory cell infiltration in IMAT will also contribute to improving the management of metabolic disorders caused by IMAT.
Up to now, our understanding of the unique biology of IMAT, including its cellular, molecular, and biochemical mechanisms, has been enhanced primarily through IMAT tissue biopsy and related methodologies. However, knowledge concerning specific components of IMAT cell composition, secretion factors, and their influence on other metabolic tissues is still in its infancy. To fully uncover the impact of this unique adipose tissue on human health and diseases, additional comprehensive investigations into the quantity and biology of IMAT are crucial. While there is much work to be done, unraveling the mechanisms of IMAT infiltration will be an exciting area of future inquiry.
TZ: Data curation, Methodology, Supervision, Writing – original draft, Writing – review & editing. JL: Supervision, Writing – review & editing, Data curation, Methodology, Writing – original draft. XL: Conceptualization, Supervision, Writing – review & editing. YL: Supervision, Writing – review & editing, Conceptualization.
The author(s) declare financial support was received for the research, authorship, and/or publication of this article.
The authors declare that the research was conducted in the absence of any commercial or financial relationships that could be construed as a potential conflict of interest.
All claims expressed in this article are solely those of the authors and do not necessarily represent those of their affiliated organizations, or those of the publisher, the editors and the reviewers. Any product that may be evaluated in this article, or claim that may be made by its manufacturer, is not guaranteed or endorsed by the publisher.
1. Blüher M. Obesity: global epidemiology and pathogenesis. Nat Rev Endocrinol (2019) 15:288–98. doi: 10.1038/s41574-019-0176-8
2. Goodpaster BH, Bergman BC, Brennan AM, Sparks LM. Intermuscular adipose tissue in metabolic disease. Nat Rev Endocrinol (2023) 19:285–98. doi: 10.1038/s41574-022-00784-2
3. Hamrick MW, McGee-Lawrence ME, Frechette DM. Fatty infiltration of skeletal muscle: mechanisms and comparisons with bone marrow adiposity. Front Endocrinol (2016) 7:69. doi: 10.3389/fendo.2016.00069
4. Goodpaster BH, Kelley DE. Skeletal muscle triglyceride: marker or mediator of obesity-induced insulin resistance in type 2 diabetes mellitus? Curr Diabetes Rep (2002) 2:216–22. doi: 10.1007/s11892-002-0086-2
5. Goodpaster BH, Thaete FL, Kelley DE. Thigh adipose tissue distribution is associated with insulin resistance in obesity and in type 2 diabetes mellitus. Am J Clin Nutr (2000) 71:885–92. doi: 10.1093/ajcn/71.4.885
6. Huynh K, Ayers C, Butler J, Neeland I, Kritchevsky S, Pandey A, et al. Association between thigh muscle fat infiltration and incident heart failure: the health ABC study. JACC. Heart Failure (2022) 10:485–93. doi: 10.1016/j.jchf.2022.04.012
7. Rossi A, Zoico E, Goodpaster BH, Sepe A, Di Francesco V, Fantin F, et al. Quantification of intermuscular adipose tissue in the erector spinae muscle by MRI: agreement with histological evaluation. Obes (Silver Spring Md.) (2010) 18:2379–84. doi: 10.1038/oby.2010.48
8. Zoico E, Rossi A, Di Francesco V, Sepe A, Olioso D, Pizzini F, et al. Adipose tissue infiltration in skeletal muscle of healthy elderly men: relationships with body composition, insulin resistance, and inflammation at the systemic and tissue level. J Gerontol Ser A Biol Sci Med Sci (2010) 65:295–9. doi: 10.1093/gerona/glp155
9. Bang E, Tanabe K, Yokoyama N, Chijiki S, Kuno S. Relationship between thigh intermuscular adipose tissue accumulation and number of metabolic syndrome risk factors in middle-aged and older Japanese adults. Exp Gerontol (2016) 79:26–30. doi: 10.1016/j.exger.2016.03.010
10. Buras ED, Converso-Baran K, Davis CS, Akama T, Hikage F, Michele DE, et al. Fibro-adipogenic remodeling of the diaphragm in obesity-associated respiratory dysfunction. Diabetes (2018) 68(1):45–56. doi: 10.2337/db18-0209
11. Joe AW, Yi L, Natarajan A, Le Grand F, So L, Wang J, et al. Muscle injury activates resident fibro/adipogenic progenitors that facilitate myogenesis. Nat Cell Biol (2010) 12:153–63. doi: 10.1038/ncb2015
12. Girousse A, Gil-Ortega M, Bourlier V, Bergeaud C, Sastourné-Arrey Q, Moro C, et al. The release of adipose stromal cells from subcutaneous adipose tissue regulates ectopic intramuscular adipocyte deposition. Cell Rep (2019) 27:323–333.e325. doi: 10.1016/j.celrep.2019.03.038
13. Arrighi N, Moratal C, Clement N, Giorgetti-Peraldi S, Peraldi P, Loubat A, et al. Characterization of adipocytes derived from fibro/adipogenic progenitors resident in human skeletal muscle. Cell Death Dis (2015) 6:e1733. doi: 10.1038/cddis.2015.79
14. Crisan M, Casteilla L, Lehr L, Carmona M, Paoloni-Giacobino A, Yap S, et al. A reservoir of brown adipocyte progenitors in human skeletal muscle. Stem Cells (Dayton Ohio) (2008) 26:2425–33. doi: 10.1634/stemcells.2008-0325
15. Almind K, Manieri M, Sivitz WI, Cinti S, Kahn CR. Ectopic brown adipose tissue in muscle provides a mechanism for differences in risk of metabolic syndrome in mice. Proc Natl Acad Sci USA (2007) 104:2366–71. doi: 10.1073/pnas.0610416104
16. Goodpaster BH, Krishnaswami S, Resnick H, Kelley DE, Haggerty C, Harris TB, et al. Association between regional adipose tissue distribution and both type 2 diabetes and impaired glucose tolerance in elderly men and women. Diabetes Care (2003) 26:372–9. doi: 10.2337/diacare.26.2.372
17. Sachs S, Zarini S, Kahn DE, Harrison KA, Perreault L, Phang T, et al. Intermuscular adipose tissue directly modulates skeletal muscle insulin sensitivity in humans. Am J Physiol Endocrinol Metab (2019) 316:E866–e879. doi: 10.1152/ajpendo.00243.2018
18. Kahn DE, Bergman BC. Keeping it local in metabolic disease: adipose tissue paracrine signaling and insulin resistance. Diabetes (2022) 71:599–609. doi: 10.2337/dbi21-0020
19. Beasley LE, Koster A, Newman AB, Javaid MK, Ferrucci L, Kritchevsky SB, et al. Inflammation and race and gender differences in computerized tomography-measured adipose depots. Obes (Silver Spring Md.) (2009) 17:1062–9. doi: 10.1038/oby.2008.627
20. Khan IM, Perrard XY, Brunner G, Lui H, Sparks LM, Smith SR, et al. Intermuscular and perimuscular fat expansion in obesity correlates with skeletal muscle T cell and macrophage infiltration and insulin resistance. Int J Obes (2005) (2015) 39:1607–18. doi: 10.1038/ijo.2015.104
21. Wu H, Ballantyne CM. Skeletal muscle inflammation and insulin resistance in obesity. J Clin Invest (2017) 127:43–54. doi: 10.1172/jci88880
22. Laurens C, Louche K, Sengenes C, Coué M, Langin D, Moro C, et al. Adipogenic progenitors from obese human skeletal muscle give rise to functional white adipocytes that contribute to insulin resistance. Int J Obes (2005) (2016) 40:497–506. doi: 10.1038/ijo.2015.193
23. Liu W, Liu Y, Lai X, Kuang S. Intramuscular adipose is derived from a non-Pax3 lineage and required for efficient regeneration of skeletal muscles. Dev Biol (2012) 361:27–38. doi: 10.1016/j.ydbio.2011.10.011
24. Bachmanov AA, Reed DR, Tordoff MG, Price RA, Beauchamp GK. Nutrient preference and diet-induced adiposity in C57BL/6ByJ and 129P3/J mice. Physiol Behav (2001) 72:603–13. doi: 10.1016/s0031-9384(01)00412-7
25. Gorski T, Mathes S, Krützfeldt J. Uncoupling protein 1 expression in adipocytes derived from skeletal muscle fibro/adipogenic progenitors is under genetic and hormonal control. J Cachexia Sarcopenia Muscle (2018) 9:384–99. doi: 10.1002/jcsm.12277
26. Schulz TJ, Huang TL, Tran TT, Zhang H, Townsend KL, Shadrach JL, et al. Identification of inducible brown adipocyte progenitors residing in skeletal muscle and white fat. Proc Natl Acad Sci USA (2011) 108:143–8. doi: 10.1073/pnas.1010929108
27. Liu Y, Fu W, Seese K, Yin A, Yin H. Ectopic brown adipose tissue formation within skeletal muscle after brown adipose progenitor cell transplant augments energy expenditure. FASEB journal: Off Publ Fed Am Societies Exp Biol (2019) 33:8822–35. doi: 10.1096/fj.201802162RR
28. Cai J, Jiang S, Quan Y, Lin J, Zhu S, Wang J, et al. Skeletal muscle provides a pro-browning microenvironment for transplanted brown adipose tissue to maintain its effect to ameliorate obesity in ob/ob mice. FASEB J (2022) 36:e22056. doi: 10.1096/fj.202101144R
29. Asakura A, Komaki M, Rudnicki M. Muscle satellite cells are multipotential stem cells that exhibit myogenic, osteogenic, and adipogenic differentiation. Differentiation; Res Biol Diversity (2001) 68:245–53. doi: 10.1046/j.1432-0436.2001.680412.x
30. De Coppi P, Milan G, Scarda A, Boldrin L, Centobene C, Piccoli M, et al. Rosiglitazone modifies the adipogenic potential of human muscle satellite cells. Diabetologia (2006) 49:1962–73. doi: 10.1007/s00125-006-0304-6
31. Pasut A, Chang NC, Gurriaran-Rodriguez U, Faulkes S, Yin H, Lacaria M, et al. Notch signaling rescues loss of satellite cells lacking pax7 and promotes brown adipogenic differentiation. Cell Rep (2016) 16:333–43. doi: 10.1016/j.celrep.2016.06.001
32. Uezumi A, Fukada S, Yamamoto N, Takeda S, Tsuchida K. Mesenchymal progenitors distinct from satellite cells contribute to ectopic fat cell formation in skeletal muscle. Nat Cell Biol (2010) 12:143–52. doi: 10.1038/ncb2014
33. Pisani DF, Dechesne CA, Sacconi S, Delplace S, Belmonte N, Cochet O, et al. Isolation of a highly myogenic CD34-negative subset of human skeletal muscle cells free of adipogenic potential. Stem Cells (Dayton Ohio) (2010) 28:753–64. doi: 10.1002/stem.317
34. Uezumi A, Fukada S, Yamamoto N, Ikemoto-Uezumi M, Nakatani M, Morita M, et al. Identification and characterization of PDGFRα+ mesenchymal progenitors in human skeletal muscle. Cell Death Dis (2014) 5:e1186. doi: 10.1038/cddis.2014.161
35. Camps J, Breuls N, Sifrim A, Giarratana N, Corvelyn M, Danti L, et al. Interstitial cell remodeling promotes aberrant adipogenesis in dystrophic muscles. Cell Rep (2020) 31:107597. doi: 10.1016/j.celrep.2020.107597
36. Xu Z, You W, Chen W, Zhou Y, Nong Q, Valencak TG, et al. Single-cell RNA sequencing and lipidomics reveal cell and lipid dynamics of fat infiltration in skeletal muscle. J Cachexia Sarcopenia Muscle (2021) 12:109–29. doi: 10.1002/jcsm.12643
37. Lu A, Tseng C, Guo P, Gao Z, Whitney KE, Kolonin MG, et al. The role of the aging microenvironment on the fate of PDGFRβ lineage cells in skeletal muscle repair. Stem Cell Res Ther (2022) 13:405. doi: 10.1186/s13287-022-03072-y
38. Uezumi A, Fukada S, Yamamoto N, Takeda S, Tsuchida K. Mesenchymal progenitors distinct from satellite cells contribute to ectopic fat cell formation in skeletal muscle. Nature cell biology (2010) 12:143–52. doi: 10.1038/ncb2014
39. Hogarth MW, Defour A, Lazarski C, Gallardo E, Diaz Manera J, Partridge TA, et al. Fibroadipogenic progenitors are responsible for muscle loss in limb girdle muscular dystrophy 2B. Nat Commun (2019) 10:2430. doi: 10.1038/s41467-019-10438-z
40. Vettor R, Milan G, Franzin C, Sanna M, De Coppi P, Rizzuto R, et al. The origin of intermuscular adipose tissue and its pathophysiological implications. Am J Physiol Endocrinol Metab (2009) 297:E987–998. doi: 10.1152/ajpendo.00229.2009
41. Fung CW, Zhou S, Zhu H, Wei X, Wu Z, Wu AR. Cell fate determining molecular switches and signaling pathways in Pax7-expressing somitic mesoderm. Cell Discov (2022) 8:61. doi: 10.1038/s41421-022-00407-0
42. Seale P, Bjork B, Yang W, Kajimura S, Chin S, Kuang S, et al. PRDM16 controls a brown fat/skeletal muscle switch. Nature (2008) 454:961–7. doi: 10.1038/nature07182
43. Yin H, Pasut A, Soleimani VD, Bentzinger CF, Antoun G, Thorn S, et al. MicroRNA-133 controls brown adipose determination in skeletal muscle satellite cells by targeting Prdm16. Cell Metab (2013) 17:210–24. doi: 10.1016/j.cmet.2013.01.004
44. Gil-Ortega M, Garidou L, Barreau C, Maumus M, Breasson L, Tavernier G, et al. Native adipose stromal cells egress from adipose tissue in vivo: evidence during lymph node activation. Stem Cells (Dayton Ohio) (2013) 31:1309–20. doi: 10.1002/stem.1375
45. Du M, Yin J, Zhu MJ. Cellular signaling pathways regulating the initial stage of adipogenesis and marbling of skeletal muscle. Meat Sci (2010) 86:103–9. doi: 10.1016/j.meatsci.2010.04.027
46. Rosen ED, MacDougald OA. Adipocyte differentiation from the inside out. Nat Rev Mol Cell Biol (2006) 7:885–96. doi: 10.1038/nrm2066
47. Xu Q, Li Y, Lin S, Wang Y, Zhu J, Lin Y. KLF4 inhibits the differentiation of goat intramuscular preadipocytes through targeting C/EBPβ Directly. Front Genet (2021) 12:663759. doi: 10.3389/fgene.2021.663759
48. Banerjee SS, Feinberg MW, Watanabe M, Gray S, Haspel RL, Denkinger DJ, et al. The Krüppel-like factor KLF2 inhibits peroxisome proliferator-activated receptor-gamma expression and adipogenesis. J Biol Chem (2003) 278:2581–4. doi: 10.1074/jbc.M210859200
49. Sun GR, Zhang M, Sun JW, Li F, Ma XF, Li WT, et al. Krüppel-like factor KLF9 inhibits chicken intramuscular preadipocyte differentiation. Br Poultry Sci (2019) 60:790–7. doi: 10.1080/00071668.2019.1657229
50. Lin Y, Wen-Jie Z, Chang-Qing L, Sheng-Xiang A, Yue Z. mir-22-3p/KLF6/MMP14 axis in fibro-adipogenic progenitors regulates fatty infiltration in muscle degeneration. FASEB J (2020) 34:12691–701. doi: 10.1096/fj.202000506R
51. Nakano S, Nakamura K, Teramoto N, Yamanouchi K, Nishihara M. Basic fibroblast growth factor is pro-adipogenic in rat skeletal muscle progenitor clone, 2G11 cells. Anim Sci J = Nihon chikusan Gakkaiho (2016) 87:99–108. doi: 10.1111/asj.12397
52. Cui S, Li X, Li R, Zhang H, Wang Y, Li Y, et al. FGF1 promotes the differentiation of goat intramuscular and subcutaneous preadipocytes. Anim Biotechnol (2021), 1-13. doi: 10.1080/10495398.2021.2016430
53. Mathes S, Fahrner A, Ghoshdastider U, Rüdiger HA, Leunig M, Wolfrum C, et al. FGF-2-dependent signaling activated in aged human skeletal muscle promotes intramuscular adipogenesis. Proc Natl Acad Sci USA (2021) 118(37). doi: 10.1073/pnas.2021013118
54. Xu Q, Lin S, Li Q, Lin Y, Xiong Y, Zhu J, et al. Fibroblast growth factor 21 regulates lipid accumulation and adipogenesis in goat intramuscular adipocyte. Anim Biotechnol (2021) 32:318–26. doi: 10.1080/10495398.2019.1691010
55. Kopinke D, Roberson EC, Reiter JF. Ciliary hedgehog signaling restricts injury-induced adipogenesis. Cell (2017) 170:340–351.e312. doi: 10.1016/j.cell.2017.06.035
56. Marinkovic M, Fuoco C, Sacco F, Cerquone Perpetuini A, Giuliani G, Micarelli E, et al. Fibro-adipogenic progenitors of dystrophic mice are insensitive to NOTCH regulation of adipogenesis. Life Sci Alliance (2019) 2(3). doi: 10.26508/lsa.201900437
57. Reggio A, Rosina M, Palma A, Cerquone Perpetuini A, Petrilli LL, Gargioli C, et al. Adipogenesis of skeletal muscle fibro/adipogenic progenitors is affected by the WNT5a/GSK3/β-catenin axis. Cell Death Differ (2020) 27(10):2921–41. doi: 10.1038/s41418-020-0551-y
58. Yao L, Tichy ED, Zhong L, Mohanty S, Wang L, Ai E, et al. Gli1 defines a subset of fibro-adipogenic progenitors that promote skeletal muscle regeneration with less fat accumulation. J Bone Mineral Res (2021) 36:1159–73. doi: 10.1002/jbmr.4265
59. Ross SE, Hemati N, Longo KA, Bennett CN, Lucas PC, Erickson RL, et al. Inhibition of adipogenesis by Wnt signaling. Sci (New York N.Y.) (2000) 289:950–3. doi: 10.1126/science.289.5481.950
60. Redshaw Z, Loughna PT. Adipogenic differentiation of muscle derived cells is repressed by inhibition of GSK-3 activity. Front vet Sci (2018) 5:110. doi: 10.3389/fvets.2018.00110
61. Vertino AM, Taylor-Jones JM, Longo KA, Bearden ED, Lane TF, McGehee RE Jr, et al. Wnt10b deficiency promotes coexpression of myogenic and adipogenic programs in myoblasts. Mol Biol Cell (2005) 16:2039–48. doi: 10.1091/mbc.e04-08-0720
62. Scarda A, Franzin C, Milan G, Sanna M, Dal Prà C, Pagano C, et al. Increased adipogenic conversion of muscle satellite cells in obese Zucker rats. Int J Obes (2005) (2010) 34:1319–27. doi: 10.1038/ijo.2010.47
63. Brack AS, Conboy IM, Conboy MJ, Shen J, Rando TA. A temporal switch from notch to Wnt signaling in muscle stem cells is necessary for normal adult myogenesis. Cell Stem Cell (2008) 2:50–9. doi: 10.1016/j.stem.2007.10.006
64. Li R, Meng S, Ji M, Rong X, You Z, Cai C, et al. HMG20A inhibit adipogenesis by transcriptional and epigenetic regulation of MEF2C expression. Int J Mol Sci (2022) 23 (18). doi: 10.3390/ijms231810559
65. Mozzetta C, Consalvi S, Saccone V, Tierney M, Diamantini A, Mitchell KJ, et al. Fibroadipogenic progenitors mediate the ability of HDAC inhibitors to promote regeneration in dystrophic muscles of young, but not old Mdx mice. EMBO Mol Med (2013) 5:626–39. doi: 10.1002/emmm.201202096
66. Wosczyna MN, Perez Carbajal EE, Wagner MW, Paredes S, Konishi CT, Liu L, et al. Targeting microRNA-mediated gene repression limits adipogenic conversion of skeletal muscle mesenchymal stromal cells. Cell Stem Cell (2021) 28:1323–1334.e1328. doi: 10.1016/j.stem.2021.04.008
67. Theret M, Rossi FMV, Contreras O. Evolving roles of muscle-resident fibro-adipogenic progenitors in health, regeneration, neuromuscular disorders, and aging. Front Physiol (2021) 12:673404. doi: 10.3389/fphys.2021.673404
68. Hosoyama T, Ishiguro N, Yamanouchi K, Nishihara M. Degenerative muscle fiber accelerates adipogenesis of intramuscular cells via RhoA signaling pathway. Differentiation; Res Biol Diversity (2009) 77:350–9. doi: 10.1016/j.diff.2008.11.001
69. Moratal C, Arrighi N, Dechesne CA, Dani C. Control of muscle fibro-adipogenic progenitors by myogenic lineage is altered in aging and duchenne muscular dystrophy. Cell Physiol Biochem (2019) 53:1029–45. doi: 10.33594/000000196
70. Chen FF, Wang YQ, Tang GR, Liu SG, Cai R, Gao Y, et al. Differences between porcine longissimus thoracis and semitendinosus intramuscular fat content and the regulation of their preadipocytes during adipogenic differentiation. Meat Sci (2019) 147:116–26. doi: 10.1016/j.meatsci.2018.09.002
71. Waddell JN, Zhang P, Wen Y, Gupta SK, Yevtodiyenko A, Schmidt JV, et al. Dlk1 is necessary for proper skeletal muscle development and regeneration. PloS One (2010) 5:e15055. doi: 10.1371/journal.pone.0015055
72. Gu H, Zhou Y, Yang J, Li J, Peng Y, Zhang X, et al. Targeted overexpression of PPARγ in skeletal muscle by random insertion and CRISPR/Cas9 transgenic pig cloning enhances oxidative fiber formation and intramuscular fat deposition. FASEB J (2021) 35:e21308. doi: 10.1096/fj.202001812RR
73. Mytidou C, Koutsoulidou A, Katsioloudi A, Prokopi M, Kapnisis K, Michailidou K, et al. Muscle-derived exosomes encapsulate myomiRs and are involved in local skeletal muscle tissue communication. FASEB J (2021) 35:e21279. doi: 10.1096/fj.201902468RR
74. Chemello F, Grespi F, Zulian A, Cancellara P, Hebert-Chatelain E, Martini P, et al. Transcriptomic Analysis of Single Isolated Myofibers Identifies miR-27a-3p and miR-142-3p as Regulators of Metabolism in Skeletal Muscle. Cell Rep (2019) 26:3784–3797 e3788. doi: 10.1016/j.celrep.2019.02.105
75. Jiang J, Li P, Ling H, Xu Z, Yi B, Zhu S. MiR-499/PRDM16 axis modulates the adipogenic differentiation of mouse skeletal muscle satellite cells. Hum Cell (2018) 31:282–91. doi: 10.1007/s13577-018-0210-5
76. Artaza JN, Bhasin S, Magee TR, Reisz-Porszasz S, Shen R, Groome NP, et al. Myostatin inhibits myogenesis and promotes adipogenesis in C3H 10T(1/2) mesenchymal multipotent cells. Endocrinology (2005) 146:3547–57. doi: 10.1210/en.2005-0362
77. Feldman BJ, Streeper RS, Farese RV Jr., Yamamoto KR. Myostatin modulates adipogenesis to generate adipocytes with favorable metabolic effects. Proc Natl Acad Sci USA (2006) 103:15675–80. doi: 10.1073/pnas.0607501103
78. Reisz-Porszasz S, Bhasin S, Artaza JN, Shen R, Sinha-Hikim I, Hogue A, et al. Lower skeletal muscle mass in male transgenic mice with muscle-specific overexpression of myostatin. Am J Physiol Endocrinol Metab (2003) 285:E876–888. doi: 10.1152/ajpendo.00107.2003
79. Lin J, Arnold HB, Della-Fera MA, Azain MJ, Hartzell DL, Baile CA. Myostatin knockout in mice increases myogenesis and decreases adipogenesis. Biochem Biophys Res Commun (2002) 291:701–6. doi: 10.1006/bbrc.2002.6500
80. Liu K, Zhang X, Wei W, Liu X, Tian Y, Han H, et al. Myostatin/SMAD4 signaling-mediated regulation of miR-124-3p represses glucocorticoid receptor expression and inhibits adipocyte differentiation. Am J Physiol Endocrinol Metab (2019) 316:E635–e645. doi: 10.1152/ajpendo.00405.2018
81. Sun WX, Dodson MV, Jiang ZH, Yu SG, Chu WW, Chen J. Myostatin inhibits porcine intramuscular preadipocyte differentiation. vitro. Domest Anim Endocrinol (2016) 55:25–31. doi: 10.1016/j.domaniend.2015.10.005
82. Zhang F, Deng B, Wen J, Chen K, Liu W, Ye S, et al. PPARγ and MyoD are differentially regulated by myostatin in adipose-derived stem cells and muscle satellite cells. Biochem Biophys Res Commun (2015) 458:375–80. doi: 10.1016/j.bbrc.2015.01.120
83. Babcock LW, Knoblauch M, Clarke MS. The role of myostatin and activin receptor IIB in the regulation of unloading-induced myofiber type-specific skeletal muscle atrophy. J Appl Physiol (Bethesda Md.: 1985) (2015) 119:633–42. doi: 10.1152/japplphysiol.00762.2014
84. He D, Jiang Z, Tian Y, Han H, Xia M, Wei W, et al. Genetic variants in IL15 promoter affect transcription activity and intramuscular fat deposition in longissimus dorsi muscle of pigs. Anim Genet (2018) 49:19–28. doi: 10.1111/age.12611
85. Kang X, Yang MY, Shi YX, Xie MM, Zhu M, Zheng XL, et al. Interleukin-15 facilitates muscle regeneration through modulation of fibro/adipogenic progenitors. Cell Commun Signal: CCS (2018) 16:42. doi: 10.1186/s12964-018-0251-0
86. Cai C, Xiao G, Qian L, Jiang S, Li B, Xie S, et al. Gene location, expression, and function of FNDC5 in meishan pigs. Sci Rep (2017) 7:7886. doi: 10.1038/s41598-017-08406-y
87. Hei W, You Z, An J, Zhao T, Li J, Zhang W, et al. FNDC5 promotes adipogenic differentiation of primary preadipocytes in mashen pigs. Genes (2022) 14(1). doi: 10.3390/genes14010090
88. Madaro L, Passafaro M, Sala D, Etxaniz U, Lugarini F, Proietti D, et al. Denervation-activated STAT3-IL-6 signalling in fibro-adipogenic progenitors promotes myofibres atrophy and fibrosis. Nat Cell Biol (2018) 20:917–27. doi: 10.1038/s41556-018-0151-y
89. Park TJ, Park A, Kim J, Kim JY, Han BS, Oh KJ, et al. Myonectin inhibits adipogenesis in 3T3-L1 preadipocytes by regulating p38 MAPK pathway. BMB Rep (2021) 54:124–9. doi: 10.5483/BMBRep.2021.54.2.262
90. Bonet ML, Canas JA, Ribot J, Palou A. Carotenoids in adipose tissue biology and obesity. Sub-cellular Biochem (2016) 79:377–414. doi: 10.1007/978-3-319-39126-7_15
91. Berry DC, DeSantis D, Soltanian H, Croniger CM, Noy N. Retinoic acid upregulates preadipocyte genes to block adipogenesis and suppress diet-induced obesity. Diabetes (2012) 61:1112–21. doi: 10.2337/db11-1620
92. Zhao L, Son JS, Wang B, Tian Q, Chen Y, Liu X, et al. Retinoic acid signalling in fibro/adipogenic progenitors robustly enhances muscle regeneration. EBioMedicine (2020) 60:103020. doi: 10.1016/j.ebiom.2020.103020
93. Yu X, Ma Y, Luo Y, Tang G, Jiang Z, Zhang J, et al. Neonatal vitamin A administration increases intramuscular fat by promoting angiogenesis and preadipocyte formation. Meat Sci (2022) 191:108847. doi: 10.1016/j.meatsci.2022.108847
94. Leeb T, Cho I-C, Park H-B, Ahn JS, Han S-H, Lee J-B, et al. A functional regulatory variant of MYH3 influences muscle fiber-type composition and intramuscular fat content in pigs. PloS Genet (2019) 15:e1008279. doi: 10.1371/journal.pgen.1008279
95. Wang B, Fu X, Zhu MJ, Du M. Retinoic acid inhibits white adipogenesis by disrupting GADD45A-mediated Zfp423 DNA demethylation. J Mol Cell Biol (2017) 9:338–49. doi: 10.1093/jmcb/mjx026
96. Gilsanz V, Kremer A, Mo AO, Wren TA, Kremer R. Vitamin D status and its relation to muscle mass and muscle fat in young women. J Clin Endocrinol Metab (2010) 95:1595–601. doi: 10.1210/jc.2009-2309
97. Tagliafico AS, Ameri P, Bovio M, Puntoni M, Capaccio E, Murialdo G, et al. Relationship between fatty degeneration of thigh muscles and vitamin D status in the elderly: a preliminary MRI study. AJR. Am J roentgenol (2010) 194:728–34. doi: 10.2214/ajr.09.3130
98. Ryan KJ, Daniel ZC, Craggs LJ, Parr T, Brameld JM. Dose-dependent effects of vitamin D on transdifferentiation of skeletal muscle cells to adipose cells. J Endocrinol (2013) 217:45–58. doi: 10.1530/joe-12-0234
99. Schleithoff SS, Zittermann A, Tenderich G, Berthold HK, Stehle P, Koerfer R. Vitamin D supplementation improves cytokine profiles in patients with congestive heart failure: a double-blind, randomized, placebo-controlled trial. Am J Clin Nutr (2006) 83:754–9. doi: 10.1093/ajcn/83.4.754
100. Sato Y, Iwamoto J, Kanoko T, Satoh K. Low-dose vitamin D prevents muscular atrophy and reduces falls and hip fractures in women after stroke: a randomized controlled trial. Cerebrovascular Dis (Basel Switzerland) (2005) 20:187–92. doi: 10.1159/000087203
101. Afonso J, Coutinho LL, Tizioto PC, da Silva Diniz WJ, de Lima AO, Rocha MIP, et al. Muscle transcriptome analysis reveals genes and metabolic pathways related to mineral concentration in Bos indicus. Sci Rep (2019) 9:12715. doi: 10.1038/s41598-019-49089-x
102. Altun M, Edström E, Spooner E, Flores-Moralez A, Bergman E, Tollet-Egnell P, et al. Iron load and redox stress in skeletal muscle of aged rats. Muscle Nerve (2007) 36:223–33. doi: 10.1002/mus.20808
103. Li Y, Cheng JX, Yang HH, Chen LP, Liu FJ, Wu Y, et al. Transferrin receptor 1 plays an important role in muscle development and denervation-induced muscular atrophy. Neural regen Res (2021) 16:1308–16. doi: 10.4103/1673-5374.301024
104. Barrientos T, Laothamatas I, Koves TR, Soderblom EJ, Bryan M, Moseley MA, et al. Metabolic catastrophe in mice lacking transferrin receptor in muscle. EBioMedicine (2015) 2:1705–17. doi: 10.1016/j.ebiom.2015.09.041
Keywords: intermuscular adipose tissue, obesity, insulin resistance, intermuscular adipogenesis, therapeutic strategy
Citation: Zhang T, Li J, Li X and Liu Y (2023) Intermuscular adipose tissue in obesity and related disorders: cellular origins, biological characteristics and regulatory mechanisms. Front. Endocrinol. 14:1280853. doi: 10.3389/fendo.2023.1280853
Received: 21 August 2023; Accepted: 01 October 2023;
Published: 18 October 2023.
Edited by:
Isabela Lovizutto Iessi, Indiana Biosciences Research Institute, United StatesReviewed by:
Debjyoti Kundu, School of Medicine, Indiana University Bloomington, United StatesCopyright © 2023 Zhang, Li, Li and Liu. This is an open-access article distributed under the terms of the Creative Commons Attribution License (CC BY). The use, distribution or reproduction in other forums is permitted, provided the original author(s) and the copyright owner(s) are credited and that the original publication in this journal is cited, in accordance with accepted academic practice. No use, distribution or reproduction is permitted which does not comply with these terms.
*Correspondence: Xi Li, bGl4aUBzaG11LmVkdS5jbg==; Yanjun Liu, bGl1eWFuanVuXzAwMUAxNjMuY29t
†These authors have contributed equally to this work
‡ORCID: Ting Zhang, orcid.org/0000-0003-3473-956X
Jun Li, orcid.org/0000-0003-3243-317X
Xi Li, orcid.org/0000-0002-2716-7308
Yanjun Liu, orcid.org/0000-0001-5667-1047
Disclaimer: All claims expressed in this article are solely those of the authors and do not necessarily represent those of their affiliated organizations, or those of the publisher, the editors and the reviewers. Any product that may be evaluated in this article or claim that may be made by its manufacturer is not guaranteed or endorsed by the publisher.
Research integrity at Frontiers
Learn more about the work of our research integrity team to safeguard the quality of each article we publish.