- 1Department of Ophthalmology, The Affiliated Eye Hospital of Nanjing Medical University, Nanjing, China
- 2The Fourth School of Clinical Medicine, Nanjing Medical University, Nanjing, China
Macrophages/microglia are immune system defense and homeostatic cells that develop from bone marrow progenitor cells. According to the different phenotypes and immune responses of macrophages (Th1 and Th2), the two primary categories of polarized macrophages/microglia are those conventionally activated (M1) and alternatively activated (M2). Macrophage/microglial polarization is a key regulating factor in the development of inflammatory disorders, cancers, metabolic disturbances, and neural degeneration. Macrophage/microglial polarization is involved in inflammation, oxidative stress, pathological angiogenesis, and tissue healing processes in ocular diseases, particularly in diabetic retinopathy (DR). The functional phenotypes of macrophages/microglia affect disease progression and prognosis, and thus regulate the polarization or functional phenotype of microglia at different DR stages, which may offer new concepts for individualized therapy of DR. This review summarizes the involvement of macrophage/microglia polarization in physiological situations and in the pathological process of DR, and discusses the promising role of polarization in personalized treatment of DR.
1 Introduction
A crucial part of innate and acquired immunity is the immune cell class known as macrophages/microglia, which are produced by bone marrow progenitor cells. In response to certain stimuli from the ecological environment, macrophages undergo a process called macrophage polarization in which they develop distinct functional features (1). In different microenvironments macrophages/microglia may be phenotypically polarized to mount specific functional programs. M1 (classically activated macrophages) and M2 (alternatively activated macrophages) are the primary subcategories of polarized macrophages/microglia (2). Furthermore, polarized macrophages/microglia can affect local immunological responses in many ways (3–5). They are essential for the regulation of infectious and metabolic diseases, tumors, and neurodegenerative disorders (6–9).
In both healthy and unhealthy conditions, macrophages/microglia are crucial for physiological homeostasis. Physically, macrophages/microglia are highly tiled and ramified, and are characterized by a tiny soma with many branches and fine cellular processes. They incessantly, randomly, and repetitively expand and contract in all directions, serving as immunological surveillance. Phagocytosis, external antigen presentation, and management via cytokine and growth factor production are the main functions of macrophages and microglia in the pathological state. They can be activated by pattern recognition receptors to promote phagocytosis of cellular debris. In addition to their phagocytic functions, activated microglia can serve as antigen-presenting cells. They release pro-inflammatory cytokines and stimulate the immune system through direct cell-cell interaction. Furthermore, they upregulate induced nitric oxide synthase (iNOS) upon activation, which increases local nitric oxide concentrations, damages peripheral neurons, and promotes local capillary leakage.
Major causes of blindness, including diabetic retinopathy (DR), oxygen-induced retinopathy (OIR), age-related macular degeneration (AMD), uveitis, and tumors (uveal melanoma), are closely related to inflammation and immunity (10–13). The polarization of macrophages/microglia has recently been found to be associated with the onset of ocular diseases (14, 15). As a result of retinal ischemia and hypoxia, inflammatory cells such as macrophages and monocytes gather around the vessels (16). Macrophages are polarized in response to environmental stimuli. The majority of pro-angiogenic substances (including vascular endothelial growth factor [VEGF]) required for ocular neovascularization are secreted by these cells. Vascular endothelial cells and smooth muscle cells proliferate, differentiate, and migrate as a result of this process, bringing about the formation of harmed vessels and eventually retinal/vitreous hemorrhage (17). This association substantially influences the progression and prognosis of ocular diseases (Figure 1).
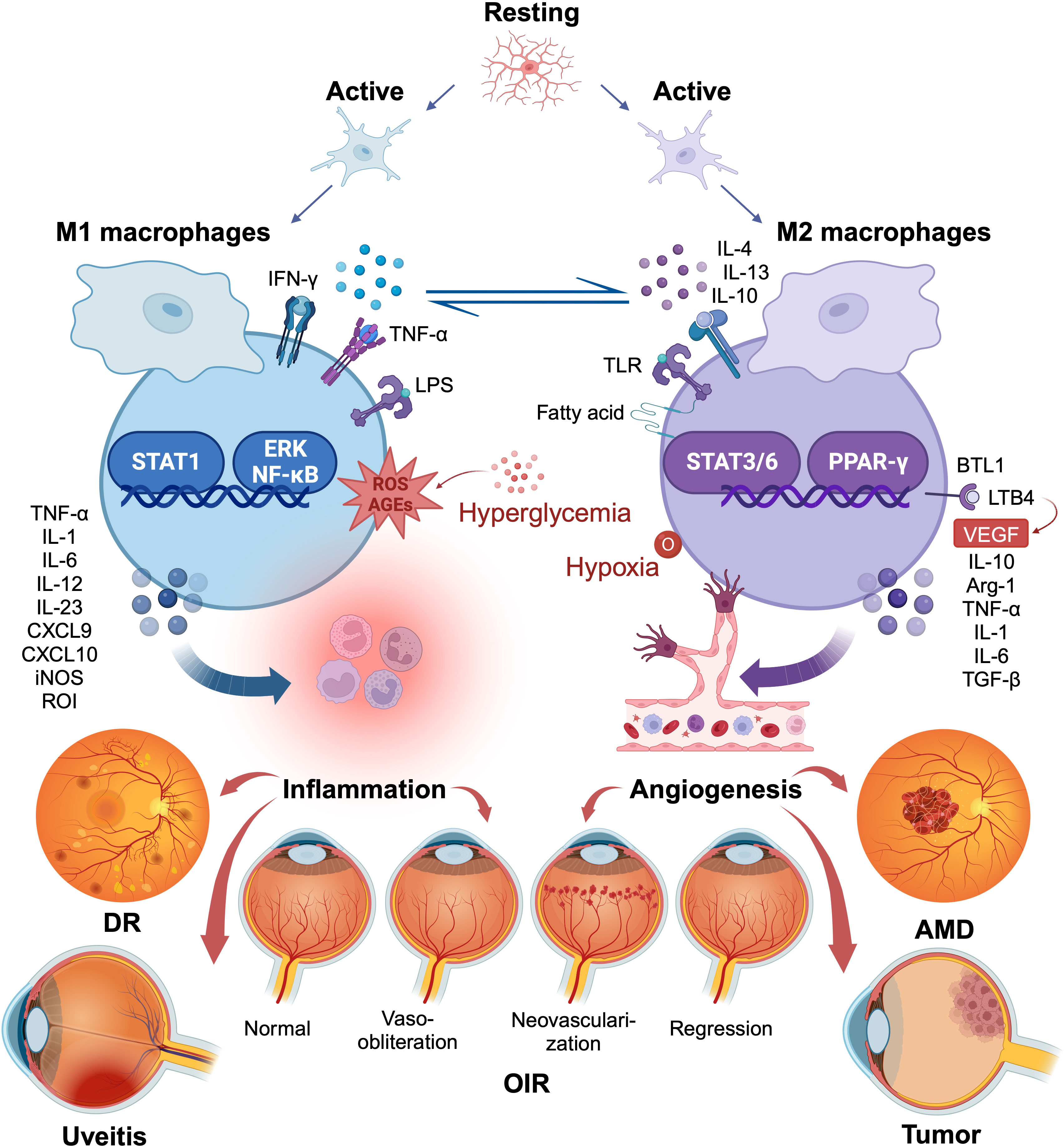
Figure 1 Macrophage/microglia polarization in several ocular disorders. The two polar opposites of the changed state of macrophage/microglia activation are M1 and M2. They are kinetically reversible; type M1 can shift to type M2 when microenvironmental conditions change, and vice versa. M1 macrophages produce pro-inflammatory chemicals that, although engulfing many pathogens, may also accelerate inflammatory processes that are detrimental. M2 macrophages, in contrast, lessen such harm, clear away necrotic cell debris, encourage tissue repair and remodeling, along with revascularization. The figure illustrates the common role of macrophage/microglia polarization in several ocular diseases. Created with BioRender.com. M1, conventionally activated macrophages; M2, alternatively activated macrophages.
Diabetes mellitus is a metabolic disease featured with hyperglycemia caused by improper insulin production and insulin resistance. Microvascular complications occur most commonly in patients with DR and their prevalence is rapidly increasing worldwide (18). DR is subdivided into non-proliferative DR (NPDR) and proliferative diabetic retinopathy (PDR) decided by whether the retina undergoes neovascularization (19). The main factor that causes permanent blindness in working-age individuals is DR, particularly PDR (20). Damage to the neurovascular unit is currently considered an important pathogenic mechanism of diabetic retinopathy (21). Neurons, glial cells, basement membranes, pericytes, and endothelial cells comprise the retinal neurovascular unit (22). Increased neural activity results in greater blood flow to meet metabolic needs. Neuronal stress and apoptosis in the early stages of DR cause microglial activation and aggravate vascular damage. Vascular abnormalities further affect neuronal cell growth, metabolism, and function, making neural damage more severe. The neurovascular unit is gradually damaged, and eventually forms a microvascular lesion (23–25). A key player in the pathophysiology of DR is VEGF. Thus, anti-VEGF medications are currently the main DR treatment (26). Anti-VEGF medications are efficient in clinically inhibiting the development of neovascularization however they have no effect on angiogenic agents other than VEGF. Other treatment modalities, such as laser photocoagulation and vitrectomy, are often accompanied by significant side effects, including damage to healthy tissues, postoperative cataracts, and neuronal apoptosis (27–29). Therefore, new treatments for DR need to be developed.
In this review, the major duties of polarized microglia and macrophages in DR are examined, their research status and prospects for DR treatment are discussed.
2 Macrophage/microglia origin, classical activation, and functions
The current view is that during embryonic development, precursor cells from the yolk sac or embryo infiltrate organs and differentiate into tissue-resident macrophages (30, 31). Tissue-resident macrophages permanently survive and constantly self renew during adulthood and peripherally derived macrophages from monocytes also exist (32). Monocyte-derived macrophages mainly differentiate from blood monocytes produced from bone marrow hematopoietic stem cells. They are stimulated by multi-colony stimulating factor (CSF) and granulocyte-macrophage CSF to develop into monocytes, pre-monocytes, and mature monocytes. Subsequently, they spread throughout the epidermal and submucosal tissues of the body where they are vital for immunological surveillance, self-stabilization, and infection resistance (33). Macrophages can be further classified into microglia, osteoblasts, and within areas, such as lung macrophages, based on their anatomical location and function type. Microglia, a type of central nervous system tissue-resident macrophages, can engulf apoptotic neurons and trigger synaptic remodeling to maintain central nervous system homeostasis (34).
Macrophages and microglia are remarkably plastic, heterogeneous cells with multiple immunological functions. They are generally classified into two groups based on their function following activation: cc or M2 and caM or M1 type (35). However, continuous in-depth research on macrophage polarization indicates this process is constantly evolving. M1 and M2 are the two extreme states of macrophages, and the phenotypes of macrophages are altered under numerous normal and pathogenic conditions (1, 36).
M1 macrophages, whose surface markers include HLA-DR, CD80, CD86, and CD197, produce pro-inflammatory cytokines (37, 38). Typically, Th1 (interferon [IFN]-gamma, tumor necrosis factor-[TNF]-alpha) or bacterial lipopolysaccharide (LPS) can induce and activate M1 polarization, causing them to release large amounts of pro-inflammatory cytokines, including TNF-alpha, interleukin (IL)-1, IL-6, IL-12, IL-18, IL-23, and the chemokine ligands CXCL-9 and CXCL-10. They also generate significant amounts of iNOS, reactive oxygen intermediates (ROI), nitric oxide, and reactive oxygen species (ROS) (39, 40). Therefore, they are effector cells in the Th1 immune response, killing intracellular pathogens, removing foreign substances, and participating in the acute pro-inflammatory response (41–43). As a result, M1 macrophages may intensify inflammatory processes that are detrimental to tissue while eliminating pathogens.
M2 macrophages secrete anti-inflammatory molecules to mitigate this damage. They generate angiogenic mediators, including transforming growth factor (TGF-beta), VEGF, and epidermal growth factor, which reduce inflammation and accelerate wound healing. However, precisely because of the secretion of these factors and their overactivation, aberrant hyperplasia may result, such as pathological angiogenesis. Four distinct populations of M2 macrophages may be identified based on the multiple activation signals they receive: M2a, M2b, M2c, and M2d, whose surface markers include CD206, CD209, CD301, and CD163 (40, 44). Type II cytokines, IL-4 or IL-13, activate M2a macrophages, which then secrete several pro-inflammatory cytokines, including IL-10 and arginase 1, and receptors, such as mannose receptor CD206 and macrophage galactose type C lectin (MGL; CD301) (40). They are insensitive to inflammatory stimuli, however, they eliminate pathogens, remove debris, stimulate angiogenesis, and aid in tissue regeneration, thus promoting Th2 immunity. Immune complexes, toll-like receptors (TLR), IL-1R ligands, and LPS can trigger M2b macrophages. These cells secrete high levels of IL-10 and low levels of IL-12. In addition, they notably release several inflammatory cytokines such as TNF-alpha, IL-1, and IL-6, which reduce acute inflammation brought on by bacterial endotoxins and promote Th2 differentiation and humoral immunity (45). IL-10, TGF-beta, glucocorticoids, or open-loop steroid hormones (such as vitamin D) can activate M2c macrophages, also referred to as inactivated macrophages. They generate large amounts of TGF-beta and IL-10, which inhibit immunological inflammation (46).
M2d macrophages, also known as tumor-associated macrophages (TAM), are dominant in tumors. IL-6 and adenosine induce them to generate a lot of IL-10 and VEGF. Evidence suggests that macrophages can inhibit immunity, promote tumor cell proliferation, and stimulate angiogenesis (47, 48). TAM release more mannose receptor and scavenger receptor A than M1 macrophages, and fewer pro-inflammatory chemicals, such as IL-1beta, IL-6, IL-12, TNF-alpha, and ROI (47). Simultaneously, the levels of several macrophage differentiation markers in TAM, including F4/80, CD11b, CD68, and CD115, are lower than those in typical M2 macrophages. Therefore, more researches are of the opinion that TAM have traits common to both M1 and M2 polarization types and are in a stage of transition between them (49, 50). Thus, the ratio of the two polarization types is influenced by the tumor microenvironment, which promotes or inhibits tumor growth (51) (Table 1).
Nevertheless, a disadvantage of this typing system is that it ignores macrophages undergoing positive division. Macrophage CSF-1 and IL-34 interact with the CSF receptor (CSF-1R) to induce macrophage proliferation and influence their differentiation, survival, and function (52). Studies have revealed that CSF-1-treated mouse microglia do not exhibit conventional M1 or M2 polarization, in contrast to earlier theories that macrophage CSF stimulation causes M2 polarization (53, 54). Therefore, the possibility of establishing M3 typing has been proposed (55). The diverse phenotypes and functions of macrophages enable them to play important roles in inflammation, pathogen resistance, tissue remodeling and repair, autoimmune regulation, and tumor suppression.
3 Retinal macrophages/microglia origin, distribution and functions
Microglia are macrophages assumed to be inherent in the central nervous system and fundamental for neuronal regeneration and immunological homeostasis (56). Primitive yolk sac macrophages are the source of microglia (57–59). In addition to tissue-resident microglia and monocyte-derived microglia in the bone marrow can penetrate the blood-retinal barrier under specific circumstances and show morphology and function similar to those of endogenous microglia in the retinal environment (60). After complete eradication of indigenous microglia in the retina, additional microglia recharge and originate from two different ways: macrophages in the ciliary body and iris, which arise peripherally (minority), and the optic nerve, which develop in the center (majority) (61, 62). This phenomenon confirms the presence of macrophage-derived microglia in the retinal periphery.
Microglia are mostly found in the inner layers of the retina in healthy individuals, including the nerve fiber layer (NFL), ganglion cell layer (GCL), inner plexiform layer (IPL), and outer plexiform layer (OPL) (25). At the age of 10-week-gestation, microglia have been found in the human retina (63). Moreover, their distribution is almost identical to that in mature retinas during development (64). Using immunocytochemistry and tomato lectin histochemistry, microglia are shown to be present in mouse retinas of 11.5-day-old embryos, distributed in the neuroblastic layer, IPL, and GCL just prior to birth, and with similar distribution as adult retinas from postnatal day 14 on (65). Microglia are also present in the OPL, ONL, IPL, GCL, and NFL of the primate retinas, resembling those of the human retinas (66). Microglia migrate to the retina in two ways. Before vascularization, most microglia migrate to the peripheral retina from nearby ocular tissues through the ciliary epithelium, primarily from the developing ciliary body and iris blood vessels. However, after vascularization, microglia originally derived from retinal blood vessels appear to migrate to the retina through the optic nerve head (67).
Conventional studies have assumed that microglia are dormant in healthy adult retinas and respond only when damaged. However, more recent studies indicate microglia have been found to be dynamic, constantly surveying the neural environment and performing tissue surveillance and intercellular communication functions (68–70). Using in vitro preparations, gradual and ongoing expansion and contraction of microglial processes may be observed, which suggest a key role in maintaining normal retinal function (71, 72). Anatomically, microglia are in direct contact with pericytes, which are strongly related to other components of the neurovascular unit nearby, to keep the normal function of the neurovascular unit and blood-retina barrier (BRB) integrity (73). Activated microglial cells are thought to play a crucial role in pathogenic situations, such as inflammation and traumatic and degenerative nerve injury. In response to damage, microglia upregulate process motility, reorient their shape and promote migratory activity (74). However, when frequently activated by alarm signals from both external and endogenous sources, microglial cells may become chronically hyperactive and secrete massive amounts of pro-inflammatory cytokines, which can result in an imbalance between the protective and injurious effects (75). Pericyte loss and endothelial cell damage result from the production of such inflammatory mediators (76). These mechanisms are present throughout DR development, and understanding the control of microglial polarization may lead to new perspectives for the development of individualized DR therapies.
4 Macrophage/microglia polarization in DR
4.1 Polarization in DR metabolism
Changes in blood glucose levels are a characteristic of diabetes mellitus. Altered glucose levels affect macrophage polarization and inflammatory cytokine secretion. Glucose transporter 1 has been reported to govern glycolysis, a process that is necessary for the generation of inflammatory cytokines and regulation of pro-inflammatory genes in microglia, indicating the participation of polarization in the pathological process (77). M1/M2 macrophage polarization is balanced in healthy human circulation. In contrast, deficiency in anti-inflammatory cells causes a significant reduction in M2 macrophage polarization, leading to an increase in M1/M2 polarization in the peripheral blood of patients with type 2 diabetes (78). Central obesity, insulin resistance, and abnormal glycemic control are typical characteristics of patients with type 2 diabetes and are all associated with a polarization imbalance. Chen et al. (79) obtained similar results. They observed that microglia stimulated by high glucose was initially polarized to the M2a phenotype, which protects against damaged tissues. However, over time, M1 pro-inflammatory factor production increases, M2 anti-inflammatory factor production decreases, and macrophages gradually tend to have the M2b phenotype (likely an intermediate phenotype between M2a and M1). In the late stage, microglia display an M1-like phenotype with pro-inflammatory effects. Furthermore, increased pro-inflammatory M1 monocyte-macrophages in the peripheral blood have been observed in individuals with preclinical diabetes, for instance impaired fasting glucose and/or impaired glucose tolerance patients (80). Similar findings were observed in the circulation of db/db mice, where pro-inflammatory cytokines (M1) and anti-inflammatory cytokines (M2) increased early (5 weeks), and by 20 weeks, db/db mice had considerably greater levels of pro-inflammatory cytokines compared to db/+ controls (81)..
Moreover, diabetes often coexists with hyperlipidemia, which is directly correlated with the onset of diabetic complications, such as DR, and accelerates progression (82). Research has shown that when TLR are activated by LPS, glucose absorption increases and glucose is oxidized to induce acetyl-CoA production, a precursor for the production of fatty acids. During inflammatory reactions, glucose metabolism is activated to promote de novo lipogenesis. Sterol regulatory element binding transcription factor 1a, which controls the production of inflammatory cytokines, is also expressed as a result of TLR activation (83). Compared to healthy people, patients with hypercholesterolemia had higher levels of pro-inflammatory CD68(+) CCR2(+) M1 monocyte macrophages, although anti-inflammatory CX3CR1(+) CD163(+)/CD206(+) M2 monocyte macrophages are decreased (84). Overall, a predominance of M1 macrophage polarization and increased secretion of pro-inflammatory cytokines have been observed in the peripheral blood of patients with diabetes.
According to recent studies, one of the most promising treatment options for diabetes may be to boost the polarization of the macrophage M2 phenotype to reduce insulin resistance and stabilize glucose/lipid metabolism (85–87). Additionally, this therapy may assist patients with diabetes in diminishing endothelial dysfunction and preventing complications of diabetes (88–90).
4.2 Microglia activation and polarization in animal models and patients with DR
An important characteristic of retinas with DR in both animals and humans is the migratory tendency of microglia. The retinal glia cells undergo alterations in both the early and late phases of DR development. A number of animal models have been created to comprehend the pathophysiology of DR. In DR model rats, M1 polarization increased, while M2 polarization decreased, and microglia were more prone to M1 polarization as the glucose concentration increased (91, 92). The iNOS (M1 marker) and arginase-1 (M2 marker) levels in the retinas of db/db mice were high at five-week-old; however, by eight-week-old, iNOS continued to rise, while arginase-1 returned to baseline levels. Retinal M1 and M2 proteins were also differentially distributed. M1-like proteins are mostly found in the outer segment, OPL, inner nuclear layer, and GCL, whereas M2-like proteins are primarily produced in the inner nuclear layer and GCL (81). Chen et al. (93) observed the activation and distribution of microglia in rats with hyperglycemia induced by Streptozotocin through OX-42 staining. The percentage of activated microglia considerably increased in the retinas of four-week-old rats with diabetes and remained high in eight- and twelve-week-old rats with diabetes. Twelve weeks following the initiation of diabetes, there appeared to be a redistribution of retinal microglia, with more in the NFL/GCL and fewer in the IPL, whereas there was no difference in the distribution of microglia in the NFL/GCL and IPL layers at 4 and 8 weeks. This demonstrates the motility of the microglia. The study by Omri et al. (94) seems to explain this phenomenon. In a Goto-Kakizaki rat model for spontaneous type 2 diabetes, the number of retinal pigment epithelium pores increased during the prophase of hyperglycemia (5 months). After long-term exposure to hyperglycemia (12 months), fewer retinal pigment epithelium pores remained, causing activated microglia/macrophages to accumulate subretinally and promote retinal injury (21, 94).
Similarly, clinical studies have demonstrated that microglia are significantly more prevalent in eyes with DR and display a hypertrophic pattern distinct from the ramified form during the resting state. The quantity of activated microglia, which are more common in the perivascular areas of the microaneurysms, slightly increases during NPDR. The number of activated microglia, which accumulate in cotton wool areas and the surrounding dilated arteries, significantly increases from NPDR to PDR. An abnormally high number of activated microglia develop in the GCL, surrounding newly formed juvenile arteries in the NFL and in the optic nerve head in PDR (95). These results imply that microglia in the retinas of DR patients are distributed similarly to 12-week-old diabetic mice. Thus, activated microglia are tightly associated with blood vessels that are closely connected geographically and promote microangiopathy in DR. Microglia/macrophages accumulate in the retina under the prolonged effects of hyperglycemia, indicating their contribution to the development of the disease. Optical coherence tomography angiography revealed that patients with PDR had significantly more macrophage-like cells surrounding their retinal vessels than healthy individuals, diabetics without DR, and patients with NPDR (96); diabetic macular edema was the most relevant factor contributing to the increase in macrophage-like cells (97). Moreover, macrophages near the vitreoretinal interface, mainly microglia, may act as biomarkers for inflammation (98). The emergence of diabetic complications, including microangiopathy, may be associated with a decrease in M2 polarization and a rise in the M1/M2 proportion. In pathological angiogenic ecology, retinal myeloid cells, especially macrophages/microglia, which are located close to endothelial cells in pathological angiogenic ecosystems, are highly glycolytic, express M1 and M2 markers more frequently, and produce more pro-inflammatory and pro-angiogenic cytokines (99). Angiogenic ability increases because of the interaction between macrophages and endothelial cells. Macrophages promote endothelial cell glycolysis and the lactate produced by glycolysis promotes macrophage activation, creating a positive feedback loop. Compared with normal retinas, patients with PDR have considerably more M1 and M2 macrophages in their fibrovascular membranes, and fibrovascular membranes development may be associated with M2 macrophages (100, 101).
The results mentioned in this section have led to the hypothesis that more than one type of polarized macrophage participate in retinal neovascularization. We can infer that in the early stages of DR, both pro-inflammatory (M1) and anti-inflammatory (M2) microglia are activated, and retinal adaptations increase to maintain their dynamic balance. Nevertheless, as the disease progresses, the number of M1 macrophages is maintained, whereas that of M2 macrophages gradually declines, favoring an inflammatory environment that causes retinal degeneration and loss of visual function.
5 Mechanisms of polarization in DR
Chronic hyperglycemia may cause gliosis by promoting the synthesis of advanced glycation end products (AGEs), which are macromolecules that are exposed to high blood glucose levels for a long period and become excessively glycated (102). Accumulated AGEs can trigger pro-inflammatory reactions via receptor (RAGE)-dependent or-independent mechanisms. In contrast to RAGE activation, which causes glial activation and the release of inflammatory cytokines, AGEs can cause endothelial cells to overexpress adhesion molecules (such as intercellular cell adhesion molecule-1) and subsequently activate leukocytes. Furthermore, oxidizing conditions and ROS generation connect retinal neuroinflammation with AGEs accumulation and create a positive feedback loop (103). Thus, inflammation and oxidative stress are the two main mechanisms involved in DR development and are closely related to macrophage polarization. Current research has mainly focused on the relationship among M1 polarization, oxidative stress, and inflammation. Both clinical and fundamental studies have shown that M2 polarization occurs in DR. Given that VEGF matters in retinal neovascularization and is a marker of M2 polarization, we also need to discuss the possible role of M2 polarization in pathological neovascularization in DR. We summarized the main signaling pathways related to polarization in Table 2.
5.1 Inflammation
Inflammation is one of the core pathological processes in DR. The expression of various inflammatory chemicals, including cytokines, chemokines, and growth factors is enhanced in the retina as a result of diabetes. The retina is already in a systemic pro-inflammatory environment before any clinical signs of DR manifest (118).
Macrophages/microglia located close to the vitreoretinal interface have been identified as possible indicators of inflammation in retinal vascular disease (98). M1 polarization and the generation of pro-inflammatory cytokines are both increased by activated NF-κB-related signaling pathways (104–106). Glucose fluctuations have been found to promote macrophage TLR4/IRF5 pathway activation, which in turn activate M1 macrophages and enhances the secretion of pro-inflammatory cytokines (108). A20, also called TNF-alpha-induced protein 3, negatively regulates M1 microglia. Glucose stimulation lowers ALKBH5 by m6A modification, resulting in reduced microglial A20 expression and promotion of M1 polarization in microglia (91). The mouse macrophage line Raw264.7 that was stimulated with high glucose levels showed that glucose primarily activates macrophages via the ROCK/JNK and ROCK/ERK pathways, promotes the conversion of the pro-inflammatory phenotype, and increases TNF-alpha expression (109). Aldose reductase, an enzyme linked to the pathophysiology of DR and strongly connected to LPS-induced M1 polarization, promotes microglial cell activation (119).
The communication between microglial cells has also been investigated. Exosomes produced from activated microglia boost microglial activation by sending polarized signals to M0 microglia via miR-155-5p (107). Inhibiting suppressor of cytokine signaling 1 and activating the NF-κB pathway, miR-155-5p eventually triggers an inflammatory cascade and intensifies the angiogenesis impact (107). In addition, studies have been conducted to determine how microglia interact with macroglia like Müller cells and astrocytes. Müller cells are susceptible to M1-polarized microglia and secrete more pro-inflammatory cytokines including IL-1beta, IL-6, and iNOS. Bidirectional signaling between the two cell types further boosts microglial activation and migration. Müller cells promote inflammatory responses throughout the retinal layer in chemotactic and sticky cell interactions, boosting microglia mobilization (120). Hyperglycemia affects retinal astrocyte proliferation, adhesion, and migration by producing inflammatory mediators (121). Translocator protein (TSPO), a microglial and astrocyte gliosis biomarker in cerebral deterioration, is acutely and selectively increased in the retinal microglia in an inflammatory environment. Likewise, astrocytes have increased levels of their endogenous ligand, diazepam-binding inhibitor (DBI). Microglial activation is adversely regulated by TSPO-mediated signaling via DBI-derived ligands (122). A network of well-coordinated astrocyte-microglial contacts is revealed by the inducibility and consequences of retinal DBI-TSPO signaling.
5.2 Oxidative stress
Free radicals are continually created in cells during metabolism under physiological conditions. Antioxidants are produced to neutralize these free radicals, ensuring a balance between them to support normal body functions. However, several metabolic issues can alter the ratio of free radicals to antioxidants, leading to an abundance of free radicals. Diabetes mellitus is a major metabolic disorder. Diabetes presents the retina with a double blow because it increases the formation of free radicals while simultaneously impairing their ability to be neutralized because of a dysfunctional antioxidant system (123). Cytosolic ROS damage the mitochondria, and mitochondrial damage enhances capillary cell death, which in turn causes diabetic retinopathy. This vicious cycle of ROS generation is further exacerbated by mitochondrial damage and continues to spread.
ERK and NF-κB signaling is activated by increased ROS levels and AGEs accumulation induced by hyperglycemia (110). Additionally, hyperglycemia stimulates HIF-1alpha-mediated VEGF production, which promotes neovascularization and serves a similar function by activating the ERK1/2-NF-κB signaling pathway in microglia (111, 112). The activation of TLR4/IRF5 signaling promotes M1 polarization and the production of matrix metalloproteinases (MMP)-9 at the same time (108). MMP-2 and MMP-9 are activated by increased ROS production, and with the aid of the heat shock protein 60, these MMPs penetrate the mitochondria. Subsequently, the mitochondrial membrane is damaged, which causes cytochrome c to leak into the cytoplasm and initiates apoptosis (124). Oxidative stress causes macrophages to polarize into the M1 form, which in turn promotes inflammation. Simultaneously, inflammatory cytokines are increased by oxidative stress and ROS may also be increased by inflammatory cytokines, forming a vicious cycle (125). Therefore, microglial polarization induces inflammation related to oxidative stress, which fosters DR emergence and progression.
Neurodegeneration and breakdown of the BRB are typical changes in the early DR stages. Before the development of clinical symptoms, the retinal neurons start decaying, and neuroretinal function is impaired prior to vascular lesions (25). Abnormal neural cell function, as well as pericyte and endothelial cell damage, are caused by the production of neurotoxic substances, including glutamate, oxidative stress, caspase-3, MMPs, and nitrous oxide (126). In the retinas of diabetic rats, activated microglia phagocytosed endothelial cells, increasing acellular capillaries and albumin leakage (127). Ding et al. (128) observed that LPS-activated M1 microglia may induce pericyte apoptosis in co-cultures. Increased levels of ROS secreted by M1 microglia may be the main reason for disruption of the inner BRB.
5.3 Angiogenesis
As mentioned in this section, fibrovascular membranes in DR formation may be associated with M2 macrophages. Recent research on DR has mostly focused on M1 polarization; studies of M2 polarization in DR are still lacking. Therefore, we summarized the role of M2 polarization in different retinal neovascular disorders to penetrate the similar potential roles and mechanisms of M2 polarization in DR.
Retinopathy of prematurity is a severe vasoproliferative disease caused by preterm birth or postnatal hyperoxia. OIR in animal models of retinopathy of prematurity are commonly used to study retinal neovascularization. Macrophages polarize toward the M2 phenotype throughout the course of retinal neovascularization, as evidenced by the fact that M1 macrophage levels change from considerably high levels at postnatal day 13 to nearly normal levels at postnatal day 21, whereas M2 macrophage levels rise from postnatal day 13 to day 21 (129). Moreover, M2 macrophages encircle emerging vessels and connect vascular sprouts. Co-culturing of human umbilical vein endothelial cells with M1- or M2-like macrophage supernatants has revealed that M2 macrophages are more effective in promoting proliferation and tube formation (130). M2 macrophage depletion by mannosylated clodronate liposomes inhibits pathological neovascularization, whereas intravitreally injecting M2 macrophages derived from the bone marrow has enhanced pathological neovascularization in OIR mice models (131). According to Wang et al. (132), increased retinal neovascularization is the outcome of M2 macrophages’ ability to attract and differentiate bone marrow-derived cells in response to stromal cell-derived factor-1 and VEGF. Furthermore, by stimulating signal transduction and activator of transcription 3, myeloid cells from circulation, particularly M2 macrophages, can travel through the lingual vascular system to the immature retina, which is ischemic and induces neovascularization (133).
Other investigations have looked into how M2 polarization influences neovascularization in AMD, which is a complicated, progressive, and degenerative disease influenced by several hereditary and environmental variables (134, 135). Two types of AMD manifest clinically: non-exudative senile macular degeneration, also called dry AMD, characterized by macular vitreous warts, pigmentary disorders, and map-like atrophy, and exudative senile macular degeneration, also called wet AMD, characterized mainly by choroidal neovascularization. The preponderance of M2 macrophages has been observed in both the mouse laser-induced choroidal neovascularization model and the atrial fluid of patients with wet AMD (136, 137). There may be a pathogenic change in the early AMD phases from pro-inflammatory M1 activity to protective M2 activity. M2 macrophages, in contrast, could serve a detrimental role by promoting fibrosis and angiogenesis. Macrophages stimulate VEGF production in laser- and AMD-associated choroidal neovascularization (138, 139). Activation of the HIF-1alpha/VEGF/VEGFR2 pathway has also been observed (113, 114). M2 macrophages are closely associated with VEGF production (132, 140). The histone acetyltransferase p300/spliced X-box binding protein 1/homocysteine-inducible endoplasmic reticulum protein with ubiquitin-like domain 1 (115), CSF1/CSF-1R (116) and prostaglandin E2/E-prostanoid 1 receptor/protein kinase C axis (117) can all, when hypoxic circumstances exist, stimulate M2 macrophage polarization, hence increase choroidal vascular endothelial cell proliferation, migration, and tube formation. M2 macrophages express leukotriene B4 (LTB4) receptor 1 (BLT1) which is drawn to the LTB to generate VEGF-A in a BLT1-dependent manner, inducing choroidal neovascularization formation (141). According to these investigations, M2 polarization is thought to be crucial for the growth of retinal neovascularization and to have a similar function in DR.
However, the specific role of M2 polarization in retinal neovascularization is still controversial. M2 macrophages are considered to play a protective function in tissue repair and remodeling (142). In a study of phase changes following OIR, in the late phase after postnatal day 17, IL-4/STAT6/PPAR-gamma signaling activity is heightened, activating M2 macrophages and peaks at postnatal day 20, causing the reduction of inflammation and the naturally occurring regression of neovascularization clusters (143). Due to a change in macrophage polarization towards an M2 phenotype, the OIR model that administered IL-17A neutralizing antibody had noticeably less retinal neovascularization than normal mice (144). Injecting umbilical cord blood-derived CD14(+) cells into OIR eyes stabilizes retinal ischemia-damaged vessels after subdivision into M2 macrophages (145). By controlling the inflammatory response, promoting tissue repair, accelerating nutritional recovery, enhancing the clearance of apoptotic cellular debris, and becoming tolerant instead of autoimmune, M2 macrophages aid in the normalization of the retinal vasculature. These effects are reinforced by the fact that M2 macrophages participate in downstream vascular anastomosis of VEGF-activated endothelial tip cells (146).
In summary, microglial polarization affects inflammation and oxidative stress caused by sustained tissue stress caused by hyperglycemia in DR, during which M1 macrophages play a critical role. The two processes interact with each other and promote the pathological alterations in DR. Pericyte loss occurs followed by endothelial cell injury due to chronic inflammation, eventually the BRB is destroyed and neoangiogenesis arises. M1 polarization has been the main subject of the majority of recent investigations on microglia polarization in DR. Other ocular neovascular disorders have been found to be related to both the pro-angiogenic and protective effects of M2 macrophages. Since DR is a typical retinal neovascularization disease caused by increased VEGF levels, the specific role of M2 macrophages in the pathogenesis of DR needs further study. Additionally, we have integrated some currently recognized polarization pathways linked to ophthalmic diseases (Figure 2). Whether the same mechanisms that cause polarization in other diseases also play a role in DR requires further investigation.
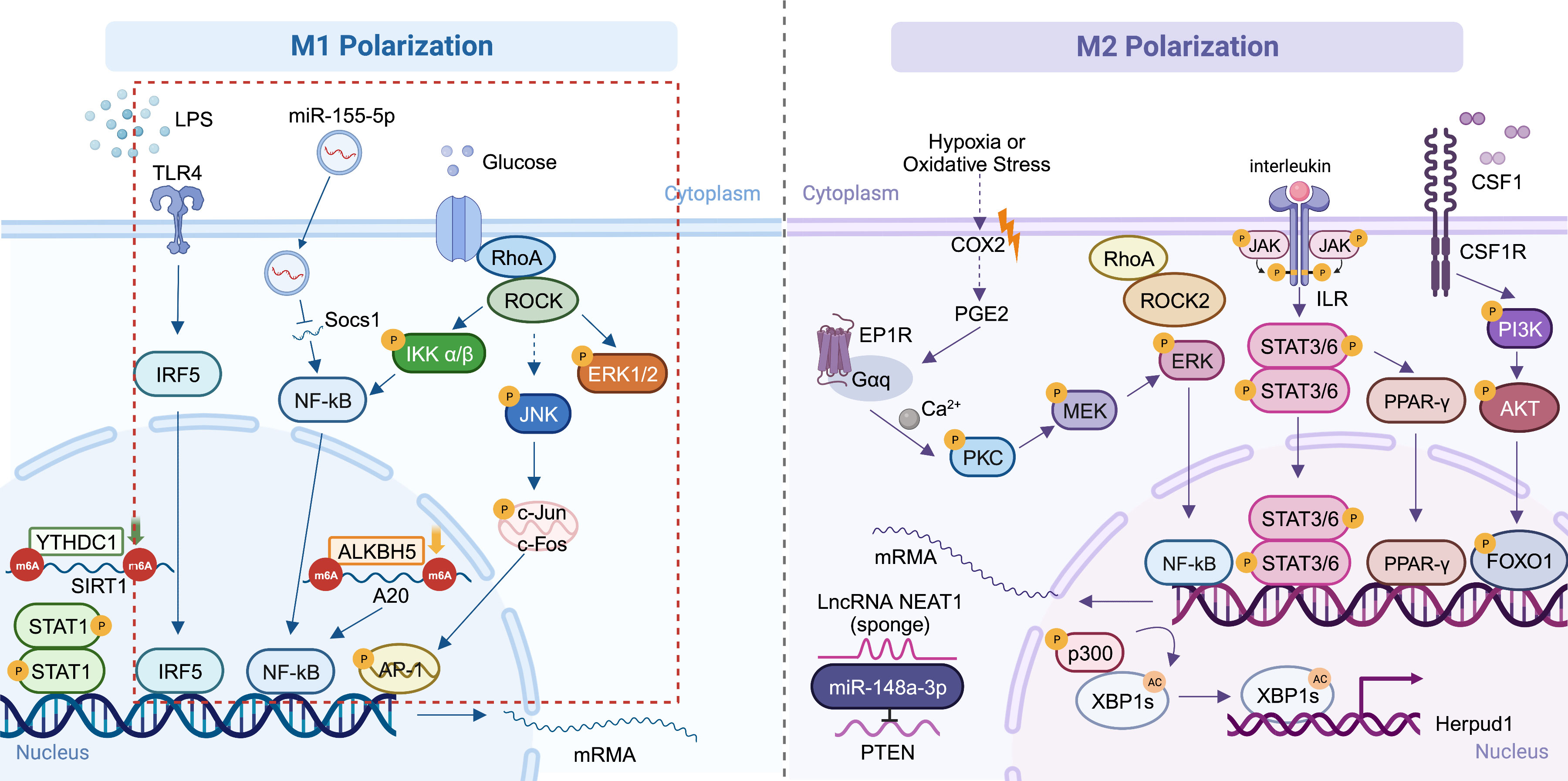
Figure 2 Mechanisms of macrophage polarization in ocular diseases. The main DR-related mechanisms are circled in red (91, 108, 109, 115–117, 134, 147, 148). Created with BioRender.com. M1, conventionally activated macrophages; M2, alternatively activated macrophages; DR, diabetic retinopathy.
6 New strategies for DR treatment targeting polarization
Considering the dominant role of M1 polarization in DR, current therapeutic approaches mainly focus on inhibiting the pro-inflammatory response to M1 polarization and promoting the switch of M1 to M2 polarization. Studies about the treatments related to polarization were summarized in Table 3.
6.1 Signaling inhibition related therapy
We can draw the conclusion that NF-κB signaling pathway really matters in M1-type polarization from the previously mentioned mechanisms. In order to effectively treat DR by targeting polarization, more attention is given to NF-κB signaling inhibitors. Melatonin (104) and pyrrolidinedithiocarbamate (149) injection into the vitreous cavity and Asiatic acid (105), cyanidin 3-O-glucoside (150) and ferulic acid (151) oral administration were reported to decrease NF-κB signaling, reduce inflammatory lesion and M1 polarization, and promote M2 polarization in the retina of rats with DR, therefore minimizing the lesions. They may simultaneously maintain BRB integrity by controlling the expression of proteins associated with barriers. The sp2-iminosugar glycolipid (sp2-IGL) family, (SS,1R)-1-docecylsulfiny-5N,6O-oxomethylidenenojirimycin ((Ss)-DS-ONJ), increases the expression of heme oxygenase-1 and IL-10 in endotoxin-stimulated microglia, thereby exerting anti-inflammatory effects by inducing arginase-1 to boost M2 responses (152). Other members of the sp2-IGL family, such as 1-dodecylsulfonyl-5N, 6O-oxomethylidenenojirimycin (DSO2-ONJ) (153) and the sp2-iminosugar dodecylsulfoxide derivative R-DS-ONJ (81) have also been shown to perform a similar function, alleviating DR. In addition, DBI-TSPO signaling in the retina is regulated by VEGF, which can alleviate the severity of inflammation, promote the production of neurotrophic factors, polarize M2 macrophages, and relieve neovascular retinopathy (154). Considering the role of aldose reductase in M1 polarization, pharmacological inhibition of aldose reductase could relieve M1 polarization induced by LPS, decelerate optic nerve degeneration, and decrease the capacity of activated microglia to induce apoptosis in retinal pigment epithelium cells, thus protecting the integrity of the BRB (119, 155–157).
6.2 Stem cell related therapy
Macrophages/microglial cells differentiate and develop from bone marrow hematopoietic cells. Based on different macrophage phenotypes and the conversion of phenotypes, a rising variety of researches have concentrated on the underlying value of bone marrow stem cell transplantation for the treatment of intravenous pathological neovascularization and retinal neurodegenerative diseases (166, 168–170). Numerous studies have demonstrated that intravenously injected stem cells or local transplantation can enhance function in ischemic regions (171–173). Stem cells can develop into endothelial (174, 175), neuronal (176, 177), and mature myeloid cells (such as, monocytes, macrophages, and dendritic cells) in vitro. According to recent studies, distinct tissue microenvironments subject myeloid cells to various stimuli and determine whether they differentiate into inflammatory macrophages (M1 and M2), endothelial cells, or dendritic cells (41, 178–180). Therefore, various myeloid cell subpopulations may have opposing effects on angiogenesis; one population may support angiogenesis in tumors (181), whereas another may suppress tip cell numbers and vascular sprouting (182).
Mesenchymal stem cells (MSCs) are found in various tissues and can differentiate in several directions. MSCs are a potential therapy for inflammatory illnesses because a significant body of research has shown that they are potent immunomodulators. They often influence microglial polarization via paracrine actions (183). MSCs inhibit NF-κB activation and excretion of TNF-alpha stimulated protein 6 in human umbilical vein endothelial cells co-stimulated by hyperglycemia and megadose of palmitic acid, thereby suppressing inflammation (162). After co-culturing with MSC-derived microvesicles, increased levels of M2 markers and fewer LPS-induced pro-inflammatory cytokines were detected in BV-2 cells, indicating their role in the phenotypic transition from M1 to M2 (163). To observe the role of MSCs in the retina, MSCs obtained from human umbilical cords were co-cultured with complete adult rat retinal explants and kept apart using a transwell membrane (164). Due to a decline in M1 markers and a raise in M2 markers, paracrine signaling of hMSC altered the microglial phenotype and the production of anti-inflammatory proteins in the retina (164). In neurological studies, MSCs have been found to induce M2 polarization in macrophages and facilitate their migration into MSCs in vitro (165). Therefore, it is conceivable that injecting MSCs into the vitreous cavity may be a potential therapeutic approach for treating DR since it may increase the number of microglia with anti-inflammatory phenotypes and help preserve the retina.
According to Marchetti et al. (145) injecting the human umbilical cord blood into the vitreous cavity has a significant curative effect, creating abundant myeloid progenitor CD14(+) cells. CD14(+) cells can stop abnormal neovascularization and polarize into M2 macrophages. For the sake of managing cell proliferation, differentiation, and the resolution of inflammation, CD14(+) cells develop into a macrophage oriented M2 type, inducing the production of genes participating in cellular signals such as IFI6, IFI1631, BCL2A1, AKT1, NF-κB1, and TGF-beta1. They not only ease oxidative stress, apoptosis, inflammation, and angiogenesis (145, 158, 159) but also stabilize the retinal vasculature (160, 161). Furthermore, transcriptome sequencing has validated the biological functions of CD14(+) cells.
Nakagawa et al. (167) also confirmed that bone marrow-derived precursor cells can migrate to the retinal vascular network, differentiate into glial cells, and promote the steady state of the blood vessels of the retina, which plays an essential role in retinal vascular reconstruction and stabilization. Therefore, they hypothesized that this protective effect may be intimately linked to macrophage/microglia polarization. To determine whether myeloid angiogenic cells exhibit pro-angiogenic characteristics, Medina et al. (166) employed a 3D Matrigel tube formation assay and discovered that myeloid angiogenic cells might cause endothelial tube development in vitro in a paracrine manner. Additional research focusing on M1/M2 markers and pro-angiogenic genes revealed that myeloid angiogenic cells significantly expressed M2 macrophage markers, such as IL-10, transforming growth factor beta 2, CD163, macrophage scavenger receptor 1, and mannose receptor C type 1. However, pro-inflammatory M1 macrophage markers, such as TNF, IL-1, IL-6, IL-12, and cytochrome c oxidase subunit II were almost undetected. The pro-angiogenic genes VEGF-beta, connective tissue growth factor, platelet-derived growth factor beta polypeptide, neuropilin 1, and MMP-9 were also strongly expressed. This study demonstrated that M1 macrophage markers were downregulated, whereas pro-angiogenic genes and M2 macrophage markers were highly upregulated.
Overall, stem cells are a promising treatment for DR because of their pluripotency. More critically, DR development may be significantly slowed by the promotion of stem cell differentiation into M2 macrophages with anti-inflammatory capabilities.
7 Discussion
As important immunoregulatory cells, the extensive distribution and function of macrophages and microglia have become a research hotspot. Recently, researches have focused more on polarization and differentiation in cancer, cardiovascular diseases, obesity, and neovascularization. In ocular diseases, macrophage/microglia polarization is associated with the onset of illness, especially DR. The inflammatory stage appears to be dominated by M1 polarization, which is also correlated with the beginning of inflammation and the onset of early tissue damage. Disease relief and tissue healing are highly correlated with M2 polarization. However, it is also crucial to remember that increased M2 polarization may play a role in several aberrant healing processes, including fibrosis and angiogenesis. Phenotype conversion not only reflects the tissue microenvironment inflammatory state but also suggests the severity of pathological neovascularization, which affects the outcome and prognosis of the disease.
The present review demonstrated that early DR, even at the preclinical stage, is accompanied by both M1 and M2 polarization, whereas only M1 polarization is effective in the latter phases. The classic change in the latter phase of DR (PDR) is the secretion of large quantities of VEGF, which results in retinal neovascularization. However, M2 polarization is often considered to result in excessive VEGF release. Therefore, further research is needed to explore whether M2 polarization continues to play a significant role in the later DR stages so that more precise treatment can be realized. Moreover, the target of current treatments is to convert M1 polarization to M2 polarization. It is still doubtful whether such medication will boost VEGF output and aggravate the lesion, despite the possibility that it will reduce inflammation. Inflammation and neovascularization signaling pathways often crosstalk, and persistent chronic inflammation can result in neovascularization. The M2 macrophages are mostly anti-inflammatory and pro-neovascular cells. If M2 macrophages encourage the formation of structurally and functionally intact neovascularization to replace pathogenic neovascularization in DR, this may represent a potential therapeutic strategy. A better approach is to focus more on the transformation of resting M0 macrophages, which should be further investigated. Stem cell therapy deserves more attention as a personalized treatment. Depending on its pluripotency, they may differentiate into various macrophage states, including M1, M2, and even quiescent M0, thereby opening up more treatment options. Depending on an individual patient’s retinal microenvironment, different measures can be implemented on a case-by-case basis, thus maximizing personalization.
Given that the current research is not in-depth, further exploration of the internal mechanisms of macrophage/microglial polarization is important for understanding ocular diseases. The “double” polarization of macrophages/microglia remains controversial, its plasticity and diversity need to be further studied and assessed. It is still being determined whether some functional categories reflect different populations because macrophage polarization characteristics are not unique. It is also possible to reverse polarization imbalances, thus intervening in disease development. These findings will likely provide new therapeutic strategies for DR and bring up new therapy options for ocular disorders.
Author contributions
YY: Writing – original draft, Visualization. JL: Writing – original draft, Writing – review & editing. YZ: Writing – original draft, Writing – review & editing. SW: Writing – original draft. ZZ: Writing – original draft. QJ: Writing – review & editing, Supervision. KL: Writing – review & editing, Supervision, Funding acquisition.
Funding
The authors declare financial support was received for the research, authorship, and/or publication of this article. The study was funded by the National Natural Science Foundation of China (82171080, 82101155), and Postgraduate Research & Practice Innovation Program of Jiangsu Province (JX10413973).
Acknowledgments
The authors would like to express their gratitude to the Affiliated Eye Hospital of Nanjing Medical University for funding the study. The study was funded by the National Natural Science Foundation of China (82171080, 82101155), and Postgraduate Research & Practice Innovation Program of Jiangsu Province (JX10413973).
Conflict of interest
The authors declare that the research was conducted in the absence of any commercial or financial relationships that could be construed as a potential conflict of interest.
Publisher’s note
All claims expressed in this article are solely those of the authors and do not necessarily represent those of their affiliated organizations, or those of the publisher, the editors and the reviewers. Any product that may be evaluated in this article, or claim that may be made by its manufacturer, is not guaranteed or endorsed by the publisher.
Glossary
References
1. Murray PJ. Macrophage polarization. Annu Rev Physiol (2017) 79:541–66. doi: 10.1146/annurev-physiol-022516-034339
2. Hu X. Microglia/macrophage polarization: Fantasy or evidence of functional diversity? J Cereb Blood Flow Metab (2020) 40(1_suppl):S134–s6. doi: 10.1177/0271678x20963405
3. Pérez S, Rius-Pérez S. Macrophage polarization and reprogramming in acute inflammation: A redox perspective. Antioxid (Basel) (2022) 11(7):1394. doi: 10.3390/antiox11071394
4. Ahamada MM, Jia Y, Wu X. Macrophage polarization and plasticity in systemic lupus erythematosus. Front Immunol (2021) 12:734008. doi: 10.3389/fimmu.2021.734008
5. Garnier M, Gibelin A, Mailleux AA, Leçon V, Hurtado-Nedelec M, Laschet J, et al. Macrophage polarization favors epithelial repair during acute respiratory distress syndrome. Crit Care Med (2018) 46(7):e692–701. doi: 10.1097/ccm.0000000000003150
6. Dong B, Wang C, Zhang J, Zhang J, Gu Y, Guo X, et al. Exosomes from human umbilical cord mesenchymal stem cells attenuate the inflammation of severe steroid-resistant asthma by reshaping macrophage polarization. Stem Cell Res Ther (2021) 12(1):204. doi: 10.1186/s13287-021-02244-6
7. Wu HM, Ni XX, Xu QY, Wang Q, Li XY, Hua J. Regulation of lipid-induced macrophage polarization through modulating peroxisome proliferator-activated receptor-gamma activity affects hepatic lipid metabolism via a Toll-like receptor 4/NF-κB signaling pathway. J Gastroenterol Hepatol (2020) 35(11):1998–2008. doi: 10.1111/jgh.15025
8. Zhang W, Zheng X, Yu Y, Zheng L, Lan J, Wu Y, et al. Renal cell carcinoma-derived exosomes deliver lncARSR to induce macrophage polarization and promote tumor progression via STAT3 pathway. Int J Biol Sci (2022) 18(8):3209–22. doi: 10.7150/ijbs.70289
9. Hsieh SW, Huang LC, Chang YP, Hung CH, Yang YH. M2b macrophage subset decrement as an indicator of cognitive function in Alzheimer’s disease. Psychiatry Clin Neurosci (2020) 74(7):383–91. doi: 10.1111/pcn.13000
10. Behnke V, Wolf A, Langmann T. The role of lymphocytes and phagocytes in age-related macular degeneration (AMD). Cell Mol Life Sci (2020) 77(5):781–8. doi: 10.1007/s00018-019-03419-4
11. Rathi S, Jalali S, Patnaik S, Shahulhameed S, Musada GR, Balakrishnan D, et al. Abnormal complement activation and inflammation in the pathogenesis of retinopathy of prematurity. Front Immunol (2017) 8:1868. doi: 10.3389/fimmu.2017.01868
12. Okunuki Y, Mukai R, Nakao T, Tabor SJ, Butovsky O, Dana R, et al. Retinal microglia initiate neuroinflammation in ocular autoimmunity. Proc Natl Acad Sci U S A (2019) 116(20):9989–98. doi: 10.1073/pnas.1820387116
13. García-Mulero S, Alonso MH, Del Carpio LP, Sanz-Pamplona R, Piulats JM. Additive role of immune system infiltration and angiogenesis in uveal melanoma progression. Int J Mol Sci (2021) 22(5):2669. doi: 10.3390/ijms22052669
14. Yu J, Chai P, Xie M, Ge S, Ruan J, Fan X, et al. Histone lactylation drives oncogenesis by facilitating m(6)A reader protein YTHDF2 expression in ocular melanoma. Genome Biol (2021) 22(1):85. doi: 10.1186/s13059-021-02308-z
15. Qu R, Zhou M, Qiu Y, Peng Y, Yin X, Liu B, et al. Glucocorticoids improve the balance of M1/M2 macrophage polarization in experimental autoimmune uveitis through the P38MAPK-MEF2C axis. Int Immunopharmacol (2023) 120:110392. doi: 10.1016/j.intimp.2023.110392
16. Zeng Y, Wen F, Mi L, Ji Y, Zhang X. Changes in macrophage-like cells characterized by en face optical coherence tomography after retinal stroke. Front Immunol (2022) 13:987836. doi: 10.3389/fimmu.2022.987836
17. Gao F, Hou H, Liang H, Weinreb RN, Wang H, Wang Y. Bone marrow-derived cells in ocular neovascularization: contribution and mechanisms. Angiogenesis (2016) 19(2):107–18. doi: 10.1007/s10456-016-9497-6
18. Teo ZL, Tham YC, Yu M, Chee ML, Rim TH, Cheung N, et al. Global prevalence of diabetic retinopathy and projection of burden through 2045: systematic review and meta-analysis. Ophthalmology (2021) 128(11):1580–91. doi: 10.1016/j.ophtha.2021.04.027
19. Cheung N, Mitchell P, Wong TY. Diabetic retinopathy. Lancet (2010) 376(9735):124–36. doi: 10.1016/s0140-6736(09)62124-3
20. Crabtree GS, Chang JS. Management of complications and vision loss from proliferative diabetic retinopathy. Curr Diabetes Rep (2021) 21(9):33. doi: 10.1007/s11892-021-01396-2
21. Mills SA, Jobling AI, Dixon MA, Bui BV, Vessey KA, Phipps JA, et al. Fractalkine-induced microglial vasoregulation occurs within the retina and is altered early in diabetic retinopathy. Proc Natl Acad Sci U.S.A. (2021) 118(51):e2112561118. doi: 10.1073/pnas.2112561118
22. Newman EA. Functional hyperemia and mechanisms of neurovascular coupling in the retinal vasculature. J Cereb Blood Flow Metab (2013) 33(11):1685–95. doi: 10.1038/jcbfm.2013.145
23. Garhöfer G, Chua J, Tan B, Wong D, Schmidl D, Schmetterer L. Retinal neurovascular coupling in diabetes. J Clin Med (2020) 9(9):2829. doi: 10.3390/jcm9092829
24. Kinuthia UM, Wolf A, Langmann T. Microglia and inflammatory responses in diabetic retinopathy. Front Immunol (2020) 11:564077. doi: 10.3389/fimmu.2020.564077
25. Altmann C, Schmidt MHH. The role of microglia in diabetic retinopathy: inflammation, microvasculature defects and neurodegeneration. Int J Mol Sci (2018) 19(1):110. doi: 10.3390/ijms19010110
26. Berrocal MH, Acaba LA, Chenworth ML. Surgical innovations in the treatment of diabetic macular edema and diabetic retinopathy. Curr Diabetes Rep (2019) 19(10):106. doi: 10.1007/s11892-019-1210-x
27. Everett LA, Paulus YM. Laser therapy in the treatment of diabetic retinopathy and diabetic macular edema. Curr Diabetes Rep (2021) 21(9):35. doi: 10.1007/s11892-021-01403-6
28. Berrocal MH, Acaba-Berrocal L. Early pars plana vitrectomy for proliferative diabetic retinopathy: update and review of current literature. Curr Opin Ophthalmol (2021) 32(3):203–8. doi: 10.1097/icu.0000000000000760
29. Duh EJ, Sun JK, Stitt AW. Diabetic retinopathy: current understanding, mechanisms, and treatment strategies. JCI Insight (2017) 2(14):e93751. doi: 10.1172/jci.insight.93751
30. Bian Z, Gong Y, Huang T, Lee CZW, Bian L, Bai Z, et al. Deciphering human macrophage development at single-cell resolution. Nature (2020) 582(7813):571–6. doi: 10.1038/s41586-020-2316-7
31. Masuda T, Amann L, Prinz M. Novel insights into the origin and development of CNS macrophage subsets. Clin Transl Med (2022) 12(11):e1096. doi: 10.1002/ctm2.1096
32. Silvin A, Uderhardt S, Piot C, Da Mesquita S, Yang K, Geirsdottir L, et al. Dual ontogeny of disease-associated microglia and disease inflammatory macrophages in aging and neurodegeneration. Immunity (2022) 55(8):1448–65.e6. doi: 10.1016/j.immuni.2022.07.004
33. Perdiguero EG, Geissmann F. The development and maintenance of resident macrophages. Nat Immunol (2016) 17(1):2–8. doi: 10.1038/ni.3341
34. Wang X, Zhao L, Zhang J, Fariss RN, Ma W, Kretschmer F, et al. Requirement for microglia for the maintenance of synaptic function and integrity in the mature retina. J Neurosci (2016) 36(9):2827–42. doi: 10.1523/jneurosci.3575-15.2016
35. Yunna C, Mengru H, Lei W, Weidong C. Macrophage M1/M2 polarization. Eur J Pharmacol (2020) . 877:173090. doi: 10.1016/j.ejphar.2020.173090
36. Guo S, Wang H, Yin Y. Microglia polarization from M1 to M2 in neurodegenerative diseases. Front Aging Neurosci (2022) 14:815347. doi: 10.3389/fnagi.2022.815347
37. Costantini A, Viola N, Berretta A, Galeazzi R, Matacchione G, Sabbatinelli J, et al. Age-related M1/M2 phenotype changes in circulating monocytes from healthy/unhealthy individuals. Aging (Albany NY) (2018) 10(6):1268–80. doi: 10.18632/aging.101465
38. Orihuela R, McPherson CA, Harry GJ. Microglial M1/M2 polarization and metabolic states. Br J Pharmacol (2016) 173(4):649–65. doi: 10.1111/bph.13139
39. Zhou T, Liu Y, Yang Z, Ni B, Zhu X, Huang Z, et al. IL-17 signaling induces iNOS+ microglia activation in retinal vascular diseases. Glia (2021) 69(11):2644–57. doi: 10.1002/glia.24063
40. Zhou L, Wang D, Qiu X, Zhang W, Gong Z, Wang Y, et al. DHZCP Modulates Microglial M1/M2 Polarization via the p38 and TLR4/NF-κB Signaling Pathways in LPS-Stimulated Microglial Cells. Front Pharmacol (2020) 11:1126. doi: 10.3389/fphar.2020.01126
41. Shapouri-Moghaddam A, Mohammadian S, Vazini H, Taghadosi M, Esmaeili SA, Mardani F, et al. Macrophage plasticity, polarization, and function in health and disease. J Cell Physiol (2018) 233(9):6425–40. doi: 10.1002/jcp.26429
42. Geng P, Zhu H, Zhou W, Su C, Chen M, Huang C, et al. Baicalin inhibits influenza A virus infection via promotion of M1 macrophage polarization. Front Pharmacol (2020) 11:1298. doi: 10.3389/fphar.2020.01298
43. Wang X, Li H, Chen S, He J, Chen W, Ding Y, et al. P300/CBP-associated factor (PCAF) attenuated M1 macrophage inflammatory responses possibly through KLF2 and KLF4. Immunol Cell Biol (2021) 99(7):724–36. doi: 10.1111/imcb.12455
44. Topf MC, Tuluc M, Harshyne LA, Luginbuhl A. Macrophage type 2 differentiation in a patient with laryngeal squamous cell carcinoma and metastatic prostate adenocarcinoma to the cervical lymph nodes. J Immunother Cancer (2017) 5(1):60. doi: 10.1186/s40425-017-0264-z
45. Fan W, Huang W, Chen J, Li N, Mao L, Hou S. Retinal microglia: Functions and diseases. Immunology (2022) 166(3):268–86. doi: 10.1111/imm.13479
46. Mantovani A, Sozzani S, Locati M, Allavena P, Sica A. Macrophage polarization: tumor-associated macrophages as a paradigm for polarized M2 mononuclear phagocytes. Trends Immunol (2002) 23(11):549–55. doi: 10.1016/S1471-4906(02)02302-5
47. Pan Y, Yu Y, Wang X, Zhang T. Tumor-associated macrophages in tumor immunity. Front Immunol (2020) 11:583084. doi: 10.3389/fimmu.2020.583084
48. Zhu C, Kros JM, Cheng C, Mustafa D. The contribution of tumor-associated macrophages in glioma neo-angiogenesis and implications for anti-angiogenic strategies. Neuro Oncol (2017) 19(11):1435–46. doi: 10.1093/neuonc/nox081
49. Jackaman C, Yeoh TL, Acuil ML, Gardner JK, Nelson DJ. Murine mesothelioma induces locally-proliferating IL-10(+)TNF-α(+)CD206(-)CX3CR1(+) M3 macrophages that can be selectively depleted by chemotherapy or immunotherapy. Oncoimmunology (2016) 5(6):e1173299. doi: 10.1080/2162402x.2016.1173299
50. Helm O, Held-Feindt J, Grage-Griebenow E, Reiling N, Ungefroren H, Vogel I, et al. Tumor-associated macrophages exhibit pro- and anti-inflammatory properties by which they impact on pancreatic tumorigenesis. Int J Cancer (2014) 135(4):843–61. doi: 10.1002/ijc.28736
51. Zhou J, Tang Z, Gao S, Li C, Feng Y, Zhou X. Tumor-associated macrophages: recent insights and therapies. Front Oncol (2020) 10:188. doi: 10.3389/fonc.2020.00188
52. Lin H, Lee E, Hestir K, Leo C, Huang M, Bosch E, et al. Discovery of a cytokine and its receptor by functional screening of the extracellular proteome. Science (2008) 320(5877):807–11. doi: 10.1126/science.1154370
53. Martinez FO, Gordon S. The M1 and M2 paradigm of macrophage activation: time for reassessment. F1000Prime Rep (2014) 6:13. doi: 10.12703/p6-13
54. De I, Nikodemova M, Steffen MD, Sokn E, Maklakova VI, Watters JJ, et al. CSF1 overexpression has pleiotropic effects on microglia in vivo. Glia (2014) 62(12):1955–67. doi: 10.1002/glia.22717
55. Walker DG, Lue LF. Immune phenotypes of microglia in human neurodegenerative disease: challenges to detecting microglial polarization in human brains. Alzheimers Res Ther (2015) 7(1):56. doi: 10.1186/s13195-015-0139-9
56. Eyo UB, Wu LJ. Microglia: Lifelong patrolling immune cells of the brain. Prog Neurobiol (2019) 179:101614. doi: 10.1016/j.pneurobio.2019.04.003
57. Gomez Perdiguero E, Klapproth K, Schulz C, Busch K, Azzoni E, Crozet L, et al. Tissue-resident macrophages originate from yolk-sac-derived erythro-myeloid progenitors. Nature (2015) 518(7540):547–51. doi: 10.1038/nature13989
58. O’Koren EG, Yu C, Klingeborn M, Wong AYW, Prigge CL, Mathew R, et al. Microglial function is distinct in different anatomical locations during retinal homeostasis and degeneration. Immunity (2019) 50(3):723–37.e7. doi: 10.1016/j.immuni.2019.02.007
59. Ginhoux F, Prinz M. Origin of microglia: current concepts and past controversies. Cold Spring Harb Perspect Biol (2015) 7(8):a020537. doi: 10.1101/cshperspect.a020537
60. McPherson SW, Heuss ND, Lehmann U, Roehrich H, Abedin M, Gregerson DS. The retinal environment induces microglia-like properties in recruited myeloid cells. J Neuroinflammation (2019) 16(1):151. doi: 10.1186/s12974-019-1546-9
61. Tay TL, Mai D, Dautzenberg J, Fernández-Klett F, Lin G, Sagar, et al. A new fate mapping system reveals context-dependent random or clonal expansion of microglia. Nat Neurosci (2017) 20(6):793–803. doi: 10.1038/nn.4547
62. Heuss ND, Pierson MJ, Roehrich H, McPherson SW, Gram AL, Li L, et al. Optic nerve as a source of activated retinal microglia post-injury. Acta Neuropathol Commun (2018) 6(1):66. doi: 10.1186/s40478-018-0571-8
63. Diaz-Araya CM, Provis JM, Penfold PL, Billson FA. Development of microglial topography in human retina. J Comp Neurol (1995) 363(1):53–68. doi: 10.1002/cne.903630106
64. Asare-Bediako B, Adu-Agyeiwaah Y, Abad A, Li Calzi S, Floyd JL, Prasad R, et al. Hematopoietic cells influence vascular development in the retina. Cells (2022) 11(20):3207. doi: 10.3390/cells11203207
65. Santos AM, Calvente R, Tassi M, Carrasco MC, Martín-Oliva D, Marín-Teva JL, et al. Embryonic and postnatal development of microglial cells in the mouse retina. J Comp Neurol (2008) 506(2):224–39. doi: 10.1002/cne.21538
66. Singaravelu J, Zhao L, Fariss RN, Nork TM, Wong WT. Microglia in the primate macula: specializations in microglial distribution and morphology with retinal position and with aging. Brain Struct Funct (2017) 222(6):2759–71. doi: 10.1007/s00429-017-1370-x
67. Li F, Jiang D, Samuel MA. Microglia in the developing retina. Neural Dev (2019) 14(1):12. doi: 10.1186/s13064-019-0137-x
68. Stevens B, Schafer DP. Roles of microglia in nervous system development, plasticity, and disease. Dev Neurobiol (2018) 78(6):559–60. doi: 10.1002/dneu.22594
69. Kierdorf K, Prinz M. Microglia in steady state. J Clin Invest (2017) 127(9):3201–9. doi: 10.1172/jci90602
70. Castanos MV, Zhou DB, Linderman RE, Allison R, Milman T, Carroll J, et al. Imaging of macrophage-like cells in living human retina using clinical OCT. Invest Ophthalmol Vis Sci (2020) 61(6):48. doi: 10.1167/iovs.61.6.48
71. Phipps JA, Vessey KA, Brandli A, Nag N, Tran MX, Jobling AI, et al. The role of angiotensin II/AT1 receptor signaling in regulating retinal microglial activation. Invest Ophthalmol Vis Sci (2018) 59(1):487–98. doi: 10.1167/iovs.17-22416
72. Vidal-Itriago A, Radford RAW, Aramideh JA, Maurel C, Scherer NM, Don EK, et al. Microglia morphophysiological diversity and its implications for the CNS. Front Immunol (2022) 13:997786. doi: 10.3389/fimmu.2022.997786
73. Nian S, Lo ACY, Mi Y, Ren K, Yang D. Neurovascular unit in diabetic retinopathy: pathophysiological roles and potential therapeutical targets. Eye Vis (Lond) (2021) 8(1):15. doi: 10.1186/s40662-021-00239-1
74. Lee JE, Liang KJ, Fariss RN, Wong WT. Ex vivo dynamic imaging of retinal microglia using time-lapse confocal microscopy. Invest Ophthalmol Vis Sci (2008) 49(9):4169–76. doi: 10.1167/iovs.08-2076
75. Huang Z, Zhou T, Sun X, Zheng Y, Cheng B, Li M, et al. Necroptosis in microglia contributes to neuroinflammation and retinal degeneration through TLR4 activation. Cell Death Differ (2018) 25(1):180–9. doi: 10.1038/cdd.2017.141
76. Spencer BG, Estevez JJ, Liu E, Craig JE, Finnie JW. Pericytes, inflammation, and diabetic retinopathy. Inflammopharmacology (2020) 28(3):697–709. doi: 10.1007/s10787-019-00647-9
77. Wang L, Pavlou S, Du X, Bhuckory M, Xu H, Chen M. Glucose transporter 1 critically controls microglial activation through facilitating glycolysis. Mol Neurodegener (2019) 14(1):2. doi: 10.1186/s13024-019-0305-9
78. Fadini GP, de Kreutzenberg SV, Boscaro E, Albiero M, Cappellari R, Kränkel N, et al. An unbalanced monocyte polarisation in peripheral blood and bone marrow of patients with type 2 diabetes has an impact on microangiopathy. Diabetologia (2013) 56(8):1856–66. doi: 10.1007/s00125-013-2918-9
79. Chen C, Wu S, Hong Z, Chen X, Shan X, Fischbach S, et al. Chronic hyperglycemia regulates microglia polarization through ERK5. Aging (Albany NY) (2019) 11(2):697–706. doi: 10.18632/aging.101770
80. Fadini GP, Cappellari R, Mazzucato M, Agostini C, Vigili de Kreutzenberg S, Avogaro A. Monocyte-macrophage polarization balance in pre-diabetic individuals. Acta Diabetol (2013) 50(6):977–82. doi: 10.1007/s00592-013-0517-3
81. Arroba AI, Alcalde-Estevez E, García-Ramírez M, Cazzoni D, de la Villa P, Sánchez-Fernández EM, et al. Modulation of microglia polarization dynamics during diabetic retinopathy in db/db mice. Biochim Biophys Acta (2016) 1862(9):1663–74. doi: 10.1016/j.bbadis.2016.05.024
82. Eid S, Sas KM, Abcouwer SF, Feldman EL, Gardner TW, Pennathur S, et al. New insights into the mechanisms of diabetic complications: role of lipids and lipid metabolism. Diabetologia (2019) 62(9):1539–49. doi: 10.1007/s00125-019-4959-1
83. Yan J, Horng T. Lipid metabolism in regulation of macrophage functions. Trends Cell Biol (2020) 30(12):979–89. doi: 10.1016/j.tcb.2020.09.006
84. Fadini GP, Simoni F, Cappellari R, Vitturi N, Galasso S, Vigili de Kreutzenberg S, et al. Pro-inflammatory monocyte-macrophage polarization imbalance in human hypercholesterolemia and atherosclerosis. Atherosclerosis (2014) 237(2):805–8. doi: 10.1016/j.atherosclerosis.2014.10.106
85. Sun J, Huang Q, Li S, Meng F, Li X, Gong X. miR-330-5p/Tim-3 axis regulates macrophage M2 polarization and insulin resistance in diabetes mice. Mol Immunol (2018) 95:107–13. doi: 10.1016/j.molimm.2018.02.006
86. Gao J, Cheng Y, Hao H, Yin Y, Xue J, Zhang Q, et al. Decitabine assists umbilical cord-derived mesenchymal stem cells in improving glucose homeostasis by modulating macrophage polarization in type 2 diabetic mice. Stem Cell Res Ther (2019) 10(1):259. doi: 10.1186/s13287-019-1338-2
87. Chen X, Zhuo S, Zhu T, Yao P, Yang M, Mei H, et al. Fpr2 deficiency alleviates diet-induced insulin resistance through reducing body weight gain and inhibiting inflammation mediated by macrophage chemotaxis and M1 polarization. Diabetes (2019) 68(6):1130–42. doi: 10.2337/db18-0469
88. Sha W, Zhao B, Wei H, Yang Y, Yin H, Gao J, et al. Astragalus polysaccharide ameliorates vascular endothelial dysfunction by stimulating macrophage M2 polarization via potentiating Nrf2/HO-1 signaling pathway. Phytomedicine (2023) 112:154667. doi: 10.1016/j.phymed.2023.154667
89. Zhu X, Xing P, Zhang P, Zhang M, Shen H, Chen L, et al. Fine-tuning of microglia polarization prevents diabetes-associated cerebral atherosclerosis. Front Immunol (2022) 13:948457. doi: 10.3389/fimmu.2022.948457
90. Sood A, Fernandes V, Preeti K, Khot M, Khatri DK, Singh SB. Fingolimod Alleviates Cognitive Deficit in Type 2 Diabetes by Promoting Microglial M2 Polarization via the pSTAT3-jmjd3 Axis. Mol Neurobiol (2023) 60(2):901–22. doi: 10.1007/s12035-022-03120-x
91. Chen T, Zhu W, Wang C, Dong X, Yu F, Su Y, et al. ALKBH5-mediated mA modification of A20 regulates microglia polarization in diabetic retinopathy. Front Immunol (2022) 13:813979. doi: 10.3389/fimmu.2022.813979
92. Lv K, Ying H, Hu G, Hu J, Jian Q, Zhang F. Integrated multi-omics reveals the activated retinal microglia with intracellular metabolic reprogramming contributes to inflammation in STZ-induced early diabetic retinopathy. Front Immunol (2022) 13:942768. doi: 10.3389/fimmu.2022.942768
93. Chen X, Zhou H, Gong Y, Wei S, Zhang M. Early spatiotemporal characterization of microglial activation in the retinas of rats with streptozotocin-induced diabetes. Graefes Arch Clin Exp Ophthalmol (2015) 253(4):519–25. doi: 10.1007/s00417-014-2727-y
94. Omri S, Behar-Cohen F, de Kozak Y, Sennlaub F, Verissimo LM, Jonet L, et al. Microglia/macrophages migrate through retinal epithelium barrier by a transcellular route in diabetic retinopathy: role of PKCζ in the Goto Kakizaki rat model. Am J Pathol (2011) 179(2):942–53. doi: 10.1016/j.ajpath.2011.04.018
95. Zeng HY, Green WR, Tso MO. Microglial activation in human diabetic retinopathy. Arch Ophthalmol (2008) 126(2):227–32. doi: 10.1001/archophthalmol.2007.65
96. Ong JX, Nesper PL, Fawzi AA, Wang JM, Lavine JA. Macrophage-like cell density is increased in proliferative diabetic retinopathy characterized by optical coherence tomography angiography. Invest Ophthalmol Vis Sci (2021) 62(10):2. doi: 10.1167/iovs.62.10.2
97. Zhang NT, Nesper PL, Ong JX, Wang JM, Fawzi AA, Lavine JA. Macrophage-like cells are increased in patients with vision-threatening diabetic retinopathy and correlate with macular edema. Diagnostics (2022) 12(11):2793. doi: 10.3390/diagnostics12112793
98. Rajesh A, Droho S, Lavine JA. Macrophages in close proximity to the vitreoretinal interface are potential biomarkers of inflammation during retinal vascular disease. J Neuroinflammation (2022) 19(1):203. doi: 10.1186/s12974-022-02562-3
99. Liu Z, Xu J, Ma Q, Zhang X, Yang Q, Wang L, et al. Glycolysis links reciprocal activation of myeloid cells and endothelial cells in the retinal angiogenic niche. Sci Transl Med (2020) 12(555):eaay1371. doi: 10.1126/scitranslmed.aay1371
100. Meng Z, Chen Y, Wu W, Yan B, Meng Y, Liang Y, et al. Exploring the immune infiltration landscape and M2 macrophage-related biomarkers of proliferative diabetic retinopathy. Front Endocrinol (Lausanne) (2022) 13:841813. doi: 10.3389/fendo.2022.841813
101. Yoshida S, Kobayashi Y, Nakama T, Zhou Y, Ishikawa K, Arita R, et al. Increased expression of M-CSF and IL-13 in vitreous of patients with proliferative diabetic retinopathy: implications for M2 macrophage-involving fibrovascular membrane formation. Br J Ophthalmol (2015) 99(5):629–34. doi: 10.1136/bjophthalmol-2014-305860
102. Xu J, Chen LJ, Yu J, Wang HJ, Zhang F, Liu Q, et al. Involvement of advanced glycation end products in the pathogenesis of diabetic retinopathy. Cell Physiol Biochem (2018) 48(2):705–17. doi: 10.1159/000491897
103. Bianco L, Arrigo A, Aragona E, Antropoli A, Berni A, Saladino A, et al. Neuroinflammation and neurodegeneration in diabetic retinopathy. Front Aging Neurosci (2022) . 14:937999. doi: 10.3389/fnagi.2022.937999
104. Tang L, Zhang C, Lu L, Tian H, Liu K, Luo D, et al. Melatonin maintains inner blood-retinal barrier by regulating microglia via inhibition of PI3K/Akt/Stat3/NF-κB signaling pathways in experimental diabetic retinopathy. Front Immunol (2022) 13:831660. doi: 10.3389/fimmu.2022.831660
105. Fang M, Wan W, Li Q, Wan W, Long Y, Liu H, et al. Asiatic acid attenuates diabetic retinopathy through TLR4/MyD88/NF-κB p65 mediated modulation of microglia polarization. Life Sci (2021) 277:119567. doi: 10.1016/j.lfs.2021.119567
106. Liu T, Zhang L, Joo D, Sun SC. NF-κB signaling in inflammation. Signal Transduct Target Ther (2017) 2:17023–. doi: 10.1038/sigtrans.2017.23
107. Chen X, Wang X, Cui Z, Luo Q, Jiang Z, Huang Y, et al. M1 microglia-derived exosomes promote activation of resting microglia and amplifies proangiogenic effects through Irf1/miR-155-5p/Socs1 axis in the retina. Int J Biol Sci (2023) 19(6):1791–812. doi: 10.7150/ijbs.79784
108. Al-Rashed F, Sindhu S, Arefanian H, Al Madhoun A, Kochumon S, Thomas R, et al. Repetitive intermittent hyperglycemia drives the M1 polarization and inflammatory responses in THP-1 macrophages through the mechanism involving the TLR4-IRF5 pathway. Cells (2020) 9(8):1892. doi: 10.3390/cells9081892
109. Cheng CI, Chen PH, Lin YC, Kao YH. High glucose activates Raw264.7 macrophages through RhoA kinase-mediated signaling pathway. Cell Signal (2015) 27(2):283–92. doi: 10.1016/j.cellsig.2014.11.012
110. Khalid M, Petroianu G, Adem A. Advanced glycation end products and diabetes mellitus: mechanisms and perspectives. Biomolecules (2022) 12(4):542. doi: 10.3390/biom12040542
111. Yu Z, Zhang T, Gong C, Sheng Y, Lu B, Zhou L, et al. Erianin inhibits high glucose-induced retinal angiogenesis via blocking ERK1/2-regulated HIF-1α-VEGF/VEGFR2 signaling pathway. Sci Rep (2016) 6:34306. doi: 10.1038/srep34306
112. Zhang T, Ouyang H, Mei X, Lu B, Yu Z, Chen K, et al. Erianin alleviates diabetic retinopathy by reducing retinal inflammation initiated by microglial cells via inhibiting hyperglycemia-mediated ERK1/2-NF-κB signaling pathway. FASEB J (2019) 33(11):11776–90. doi: 10.1096/fj.201802614RRR
113. Xu J, Tu Y, Wang Y, Xu X, Sun X, Xie L, et al. Prodrug of epigallocatechin-3-gallate alleviates choroidal neovascularization via down-regulating HIF-1α/VEGF/VEGFR2 pathway and M1 type macrophage/microglia polarization. BioMed Pharmacother (2020) 121:109606. doi: 10.1016/j.biopha.2019.109606
114. Liu X, Guo A, Tu Y, Li W, Li L, Liu W, et al. Fruquintinib inhibits VEGF/VEGFR2 axis of choroidal endothelial cells and M1-type macrophages to protect against mouse laser-induced choroidal neovascularization. Cell Death Dis (2020) 11(11):1016. doi: 10.1038/s41419-020-03222-1
115. Li W, Wang Y, Zhu L, Du S, Mao J, Wang Y, et al. The P300/XBP1s/Herpud1 axis promotes macrophage M2 polarization and the development of choroidal neovascularization. J Cell Mol Med (2021) 25(14):6709–20. doi: 10.1111/jcmm.16673
116. Zhou Y, Zeng J, Tu Y, Li L, Du S, Zhu L, et al. CSF1/CSF1R-mediated crosstalk between choroidal vascular endothelial cells and macrophages promotes choroidal neovascularization. Invest Ophthalmol Vis Sci (2021) 62(3):37. doi: 10.1167/iovs.62.3.37
117. Zhan P, Cui Y, Cao Y, Bao X, Wu M, Yang Q, et al. PGE2 promotes macrophage recruitment and neovascularization in murine wet-type AMD models. Cell Commun Signal (2022) 20(1):155. doi: 10.1186/s12964-022-00973-6
118. Joussen AM, Poulaki V, Le ML, Koizumi K, Esser C, Janicki H, et al. A central role for inflammation in the pathogenesis of diabetic retinopathy. FASEB J (2004) 18(12):1450–2. doi: 10.1096/fj.03-1476fje
119. Chang KC, Ponder J, Labarbera DV, Petrash JM. Aldose reductase inhibition prevents endotoxin-induced inflammatory responses in retinal microglia. Invest Ophthalmol Vis Sci (2014) 55(5):2853–61. doi: 10.1167/iovs.13-13487
120. Wang M, Ma W, Zhao L, Fariss RN, Wong WT. Adaptive Müller cell responses to microglial activation mediate neuroprotection and coordinate inflammation in the retina. J Neuroinflammation (2011) 8:173. doi: 10.1186/1742-2094-8-173
121. Shin ES, Huang Q, Gurel Z, Sorenson CM, Sheibani N. High glucose alters retinal astrocytes phenotype through increased production of inflammatory cytokines and oxidative stress. PloS One (2014) 9(7):e103148. doi: 10.1371/journal.pone.0103148
122. Wang M, Wang X, Zhao L, Ma W, Rodriguez IR, Fariss RN, et al. Macroglia-microglia interactions via TSPO signaling regulates microglial activation in the mouse retina. J Neurosci (2014) 34(10):3793–806. doi: 10.1523/jneurosci.3153-13.2014
123. Kowluru RA. Cross talks between oxidative stress, inflammation and epigenetics in diabetic retinopathy. Cells (2023) 12(2):300. doi: 10.3390/cells12020300
124. Kowluru RA, Mishra M. Regulation of matrix metalloproteinase in the pathogenesis of diabetic retinopathy. Prog Mol Biol Transl Sci (2017) 148:67–85. doi: 10.1016/bs.pmbts.2017.02.004
125. Kowluru RA, Kowluru A, Mishra M, Kumar B. Oxidative stress and epigenetic modifications in the pathogenesis of diabetic retinopathy. Prog Retin Eye Res (2015) 48:40–61. doi: 10.1016/j.preteyeres.2015.05.001
126. Qiu AW, Huang DR, Li B, Fang Y, Zhang WW, Liu QH. IL-17A injury to retinal ganglion cells is mediated by retinal Müller cells in diabetic retinopathy. Cell Death Dis (2021) 12(11):1057. doi: 10.1038/s41419-021-04350-y
127. Xie H, Zhang C, Liu D, Yang Q, Tang L, Wang T, et al. Erythropoietin protects the inner blood-retinal barrier by inhibiting microglia phagocytosis via Src/Akt/cofilin signalling in experimental diabetic retinopathy. Diabetologia (2021) 64(1):211–25. doi: 10.1007/s00125-020-05299-x
128. Ding X, Zhang M, Gu R, Xu G, Wu H. Activated microglia induce the production of reactive oxygen species and promote apoptosis of co-cultured retinal microvascular pericytes. Graefes Arch Clin Exp Ophthalmol (2017) 255(4):777–88. doi: 10.1007/s00417-016-3578-5
129. Zhu Y, Zhang L, Lu Q, Gao Y, Cai Y, Sui A, et al. Identification of different macrophage subpopulations with distinct activities in a mouse model of oxygen-induced retinopathy. Int J Mol Med (2017) 40(2):281–92. doi: 10.3892/ijmm.2017.3022
130. Gao S, Li C, Zhu Y, Wang Y, Sui A, Zhong Y, et al. PEDF mediates pathological neovascularization by regulating macrophage recruitment and polarization in the mouse model of oxygen-induced retinopathy. Sci Rep (2017) 7:42846. doi: 10.1038/srep42846
131. Zhou Y, Yoshida S, Nakao S, Yoshimura T, Kobayashi Y, Nakama T, et al. M2 macrophages enhance pathological neovascularization in the mouse model of oxygen-induced retinopathy. Invest Ophthalmol Vis Sci (2015) 56(8):4767–77. doi: 10.1167/iovs.14-16012
132. Wang Y, Chang T, Wu T, Xu W, Dou G, Wang Y, et al. M2 macrophages promote vasculogenesis during retinal neovascularization by regulating bone marrow-derived cells via SDF-1/VEGF. Cell Tissue Res (2020) 380(3):469–86. doi: 10.1007/s00441-019-03166-9
133. Hombrebueno JR, Lynch A, Byrne EM, Obasanmi G, Kissenpfennig A, Chen M, et al. Hyaloid vasculature as a major source of STAT3 (Signal transducer and activator of transcription 3) myeloid cells for pathogenic retinal neovascularization in oxygen-induced retinopathy. Arterioscler Thromb Vasc Biol (2020) 40(12):e367–e79. doi: 10.1161/ATVBAHA.120.314567
134. Zhang P, Lu B, Zhang Q, Xu F, Zhang R, Wang C, et al. LncRNA NEAT1 Sponges MiRNA-148a-3p to Suppress Choroidal Neovascularization and M2 macrophage polarization. Mol Immunol (2020) 127:212–22. doi: 10.1016/j.molimm.2020.08.008
135. Lai K, Li Y, Li L, Gong Y, Huang C, Zhang Y, et al. Intravitreal injection of triptolide attenuates subretinal fibrosis in laser-induced murine model. Phytomedicine (2021) 93:153747. doi: 10.1016/j.phymed.2021.153747
136. Yang Y, Liu F, Tang M, Yuan M, Hu A, Zhan Z, et al. Macrophage polarization in experimental and clinical choroidal neovascularization. Sci Rep (2016) 6:30933. doi: 10.1038/srep30933
137. Lai K, Gong Y, Zhao W, Li L, Huang C, Xu F, et al. Triptolide attenuates laser-induced choroidal neovascularization via M2 macrophage in a mouse model. BioMed Pharmacother (2020) 129:110312. doi: 10.1016/j.biopha.2020.110312
138. Song J, Lee K, Park SW, Chung H, Jung D, Na YR, et al. Lactic acid upregulates VEGF expression in macrophages and facilitates choroidal neovascularization. Invest Ophthalmol Vis Sci (2018) 59(8):3747–54. doi: 10.1167/iovs.18-23892
139. Wang Y, Xie L, Zhu M, Guo Y, Tu Y, Zhou Y, et al. Shikonin alleviates choroidal neovascularization by inhibiting proangiogenic factor production from infiltrating macrophages. Exp Eye Res (2021) 213:108823. doi: 10.1016/j.exer.2021.108823
140. Lai YS, Wahyuningtyas R, Aui SP, Chang KT. Autocrine VEGF signalling on M2 macrophages regulates PD-L1 expression for immunomodulation of T cells. J Cell Mol Med (2019) 23(2):1257–67. doi: 10.1111/jcmm.14027
141. Sasaki F, Koga T, Ohba M, Saeki K, Okuno T, Ishikawa K, et al. Leukotriene B4 promotes neovascularization and macrophage recruitment in murine wet-type AMD models. JCI Insight (2018) 3(18):e96902. doi: 10.1172/jci.insight.96902
142. Mantovani A, Biswas SK, Galdiero MR, Sica A, Locati M. Macrophage plasticity and polarization in tissue repair and remodelling. J Pathol (2013) 229(2):176–85. doi: 10.1002/path.4133
143. Li J, Yu S, Lu X, Cui K, Tang X, Xu Y, et al. The phase changes of M1/M2 phenotype of microglia/macrophage following oxygen-induced retinopathy in mice. Inflammation Res (2021) 70(2):183–92. doi: 10.1007/s00011-020-01427-w
144. Zhu Y, Tan W, Demetriades AM, Cai Y, Gao Y, Sui A, et al. Interleukin-17A neutralization alleviated ocular neovascularization by promoting M2 and mitigating M1 macrophage polarization. Immunology (2016) 147(4):414–28. doi: 10.1111/imm.12571
145. Marchetti V, Yanes O, Aguilar E, Wang M, Friedlander D, Moreno S, et al. Differential macrophage polarization promotes tissue remodeling and repair in a model of ischemic retinopathy. Sci Rep (2011) 1:76. doi: 10.1038/srep00076
146. Fantin A, Vieira JM, Gestri G, Denti L, Schwarz Q, Prykhozhij S, et al. Tissue macrophages act as cellular chaperones for vascular anastomosis downstream of VEGF-mediated endothelial tip cell induction. Blood (2010) 116(5):829–40. doi: 10.1182/blood-2009-12-257832
147. Xu Y, Cui K, Li J, Tang X, Lin J, Lu X, et al. Melatonin attenuates choroidal neovascularization by regulating macrophage/microglia polarization via inhibition of RhoA/ROCK signaling pathway. J Pineal Res (2020) 69(1):e12660. doi: 10.1111/jpi.12660
148. Zhou H, Xu Z, Liao X, Tang S, Li N, Hou S. Low expression of YTH domain-containing 1 promotes microglial M1 polarization by reducing the stability of sirtuin 1 mRNA. Front Cell Neurosci (2021) 15:774305. doi: 10.3389/fncel.2021.774305
149. Sui A, Chen X, Demetriades AM, Shen J, Cai Y, Yao Y, et al. Inhibiting NF-κB signaling activation reduces retinal neovascularization by promoting a polarization shift in macrophages. Invest Ophthalmol Vis Sci (2020) 61(6):4. doi: 10.1167/iovs.61.6.4
150. Zhao F, Gao X, Ge X, Cui J, Liu X. Cyanidin-3-o-glucoside (C3G) inhibits vascular leakage regulated by microglial activation in early diabetic retinopathy and neovascularization in advanced diabetic retinopathy. Bioengineered (2021) 12(2):9266–78. doi: 10.1080/21655979.2021.1996512
151. Sun X, Ma L, Li X, Wang J, Li Y, Huang Z. Ferulic acid alleviates retinal neovascularization by modulating microglia/macrophage polarization through the ROS/NF-κB axis. Front Immunol (2022) 13:976729. doi: 10.3389/fimmu.2022.976729
152. Cano-Cano F, Alcalde-Estévez E, Gómez-Jaramillo L, Iturregui M, Sánchez-Fernández EM, García Fernández JM, et al. Anti-inflammatory (M2) response is induced by a sp-iminosugar glycolipid sulfoxide in diabetic retinopathy. Front Immunol (2021) 12:632132. doi: 10.3389/fimmu.2021.632132
153. Alcalde-Estévez E, Arroba AI, Sánchez-Fernández EM, Mellet CO, García Fernández JM, Masgrau L, et al. The sp-iminosugar glycolipid 1-dodecylsulfonyl-5N,6O-oxomethylidenenojirimycin (DSO-ONJ) as selective anti-inflammatory agent by modulation of hemeoxygenase-1 in Bv.2 microglial cells and retinal explants. Cells Food Chem Toxicol (2018) 111:454–66. doi: 10.1016/j.fct.2017.11.050
154. Gao S, Li N, Wang Y, Lin Z, Zhu Y, Xu J, et al. Inhibition of vascular endothelial growth factor alleviates neovascular retinopathy with regulated neurotrophic/proinflammatory cytokines through the modulation of DBI-TSPO signaling. FASEB J (2022) 36(7):e22367. doi: 10.1096/fj.202101294RRR
155. Chang KC, Shieh B, Petrash JM. Aldose reductase mediates retinal microglia activation. Biochem Biophys Res Commun (2016) 473(2):565–71. doi: 10.1016/j.bbrc.2016.03.122
156. Rao M, Huang YK, Liu CC, Meadows C, Cheng HC, Zhou M, et al. Aldose reductase inhibition decelerates optic nerve degeneration by alleviating retinal microglia activation. Sci Rep (2023) 13(1):5592. doi: 10.1038/s41598-023-32702-5
157. Huang YK, Liu CC, Wang S, Cheng HC, Meadows C, Chang KC. The role of aldose reductase in beta-amyloid-induced microglia activation. Int J Mol Sci (2022) 23(23):15088. doi: 10.3390/ijms232315088
158. El Kasmi KC, Holst J, Coffre M, Mielke L, de Pauw A, Lhocine N, et al. General nature of the STAT3-activated anti-inflammatory response. J Immunol (Baltimore Md: 1950) (2006) 177(11):7880–8. doi: 10.4049/jimmunol.177.11.7880
159. Panopoulos AD, Zhang L, Snow JW, Jones DM, Smith AM, El Kasmi KC, et al. STAT3 governs distinct pathways in emergency granulopoiesis and mature neutrophils. Blood (2006) 108(12):3682–90. doi: 10.1182/blood-2006-02-003012
160. Ritter MR, Banin E, Moreno SK, Aguilar E, Dorrell MI, Friedlander M. Myeloid progenitors differentiate into microglia and promote vascular repair in a model of ischemic retinopathy. J Clin Invest (2006) 116(12):3266–76. doi: 10.1172/jci29683
161. Xu W, Cheng W, Cui X, Xu G. Therapeutic effect against retinal neovascularization in a mouse model of oxygen-induced retinopathy: bone marrow-derived mesenchymal stem cells versus Conbercept. BMC Ophthalmol (2020) 20(1):7. doi: 10.1186/s12886-019-1292-x
162. An X, Li L, Chen Y, Luo A, Ni Z, Liu J, et al. Mesenchymal stem cells ameliorated glucolipotoxicity in HUVECs through TSG-6. Int J Mol Sci (2016) 17(4):483. doi: 10.3390/ijms17040483
163. Jaimes Y, Naaldijk Y, Wenk K, Leovsky C, Emmrich F. Mesenchymal stem cell-derived microvesicles modulate lipopolysaccharides-induced inflammatory responses to microglia cells. Stem Cells (2017) 35(3):812–23. doi: 10.1002/stem.2541
164. Teixeira-Pinheiro LC, Toledo MF, Nascimento-Dos-Santos G, Mendez-Otero R, Mesentier-Louro LA, Santiago MF. Paracrine signaling of human mesenchymal stem cell modulates retinal microglia population number and phenotype in vitro. Exp Eye Res (2020) 200:108212. doi: 10.1016/j.exer.2020.108212
165. Honda N, Watanabe Y, Tokuoka Y, Hanajima R. Roles of microglia/macrophage and antibody in cell sheet transplantation in the central nervous system. Stem Cell Res Ther (2022) . 13(1):470. doi: 10.1186/s13287-022-03168-5
166. Medina RJ, O’Neill CL, O’Doherty TM, Knott H, Guduric-Fuchs J, Gardiner TA, et al. Myeloid angiogenic cells act as alternative M2 macrophages and modulate angiogenesis through interleukin-8. Mol Med (2011) 17(9-10):1045–55. doi: 10.2119/molmed.2011.00129
167. Nakagawa Y, Masuda H, Ito R, Kobori M, Wada M, Shizuno T, et al. Aberrant kinetics of bone marrow-derived endothelial progenitor cells in the murine oxygen-induced retinopathy model. Invest Ophthalmol Visual Sci (2011) 52(11):7835–41. doi: 10.1167/iovs.10-5880
168. Tzameret A, Sher I, Belkin M, Treves AJ, Meir A, Nagler A, et al. Epiretinal transplantation of human bone marrow mesenchymal stem cells rescues retinal and vision function in a rat model of retinal degeneration. Stem Cell Res (2015) 15(2):387–94. doi: 10.1016/j.scr.2015.08.007
169. Di Pierdomenico J, García-Ayuso D, Rodríguez González-Herrero ME, García-Bernal D, Blanquer M, Bernal-Garro JM, et al. Bone marrow-derived mononuclear cell transplants decrease retinal gliosis in two animal models of inherited photoreceptor degeneration. Int J Mol Sci (2020) 21(19):7252. doi: 10.3390/ijms21197252
170. Di Pierdomenico J, Gallego-Ortega A, Martínez-Vacas A, García-Bernal D, Vidal-Sanz M, Villegas-Pérez MP, et al. Intravitreal and subretinal syngeneic bone marrow mononuclear stem cell transplantation improves photoreceptor survival but does not ameliorate retinal function in two rat models of retinal degeneration. Acta Ophthalmol (2022) 100(6):e1313–e31. doi: 10.1111/aos.15165
171. Fang IM, Yang CM, Yang CH, Chiou SH, Chen MS. Transplantation of induced pluripotent stem cells without C-Myc attenuates retinal ischemia and reperfusion injury in rats. Exp Eye Res (2013) 113:49–59. doi: 10.1016/j.exer.2013.05.007
172. Goldenberg-Cohen N, Avraham-Lubin BC, Sadikov T, Goldstein RS, Askenasy N. Primitive stem cells derived from bone marrow express glial and neuronal markers and support revascularization in injured retina exposed to ischemic and mechanical damage. Stem Cells Dev (2012) 21(9):1488–500. doi: 10.1089/scd.2011.0366
173. Dreixler JC, Poston JN, Balyasnikova I, Shaikh AR, Tupper KY, Conway S, et al. Delayed administration of bone marrow mesenchymal stem cell conditioned medium significantly improves outcome after retinal ischemia in rats. Invest Ophthalmol Vis Sci (2014) 55(6):3785–96. doi: 10.1167/iovs.13-11683
174. Wang C, Li Y, Yang M, Zou Y, Liu H, Liang Z, et al. Efficient differentiation of bone marrow mesenchymal stem cells into endothelial cells in vitro. Eur J Vasc Endovasc Surg (2018) 55(2):257–65. doi: 10.1016/j.ejvs.2017.10.012
175. McDonald CA, Penny TR, Paton MCB, Sutherland AE, Nekkanti L, Yawno T, et al. Effects of umbilical cord blood cells, and subtypes, to reduce neuroinflammation following perinatal hypoxic-ischemic brain injury. J Neuroinflammation (2018) 15(1):47. doi: 10.1186/s12974-018-1089-5
176. Mishra A, Mohan KV, Nagarajan P, Iyer S, Kesarwani A, Nath M, et al. Peripheral blood-derived monocytes show neuronal properties and integration in immune-deficient rd1 mouse model upon phenotypic differentiation and induction with retinal growth factors. Stem Cell Res Ther (2020) 11(1):412. doi: 10.1186/s13287-020-01925-y
177. Bellon A, Wegener A, Lescallette AR, Valente M, Yang SK, Gardette R, et al. Transdifferentiation of human circulating monocytes into neuronal-like cells in 20 days and without reprograming. Front Mol Neurosci (2018) 11:323. doi: 10.3389/fnmol.2018.00323
178. Tacke F, Randolph GJ. Migratory fate and differentiation of blood monocyte subsets. Immunobiology (2006) 211(6-8):609–18. doi: 10.1016/j.imbio.2006.05.025
179. Sanberg PR, Park DH, Kuzmin-Nichols N, Cruz E, Hossne NA Jr., Buffolo E, et al. Monocyte transplantation for neural and cardiovascular ischemia repair. J Cell Mol Med (2010) 14(3):553–63. doi: 10.1111/j.1582-4934.2009.00903.x
180. Zhu Z, Zheng L, Li Y, Huang T, Chao YC, Pan L, et al. Potential immunotherapeutic targets on myeloid cells for neurovascular repair after ischemic stroke. Front Neurosci (2019) 13:758. doi: 10.3389/fnins.2019.00758
181. Protopsaltis NJ, Liang W, Nudleman E, Ferrara N. Interleukin-22 promotes tumor angiogenesis. Angiogenesis (2019) 22(2):311–23. doi: 10.1007/s10456-018-9658-x
182. Haupt F, Krishnasamy K, Napp LC, Augustynik M, Limbourg A, Gamrekelashvili J, et al. Retinal myeloid cells regulate tip cell selection and vascular branching morphogenesis via Notch ligand Delta-like 1. Sci Rep (2019) 9(1):9798. doi: 10.1038/s41598-019-46308-3
Keywords: diabetic retinopathy, immune system, macrophages, microglia, ocular diseases, polarization
Citation: Yao Y, Li J, Zhou Y, Wang S, Zhang Z, Jiang Q and Li K (2023) Macrophage/microglia polarization for the treatment of diabetic retinopathy. Front. Endocrinol. 14:1276225. doi: 10.3389/fendo.2023.1276225
Received: 11 August 2023; Accepted: 07 September 2023;
Published: 28 September 2023.
Edited by:
Kai Jin, Zhejiang University, ChinaReviewed by:
Yin Wang, First People’s Hospital of Kunshan, ChinaLi Xiao, University of Virginia, United States
Copyright © 2023 Yao, Li, Zhou, Wang, Zhang, Jiang and Li. This is an open-access article distributed under the terms of the Creative Commons Attribution License (CC BY). The use, distribution or reproduction in other forums is permitted, provided the original author(s) and the copyright owner(s) are credited and that the original publication in this journal is cited, in accordance with accepted academic practice. No use, distribution or reproduction is permitted which does not comply with these terms.
*Correspondence: Qin Jiang, anFpbjcxMEB2aXAuc2luYS5jb20=; Keran Li, a2F0aHlrZXJhbjg2MDMyN0AxMjYuY29t
†These authors share first authorship