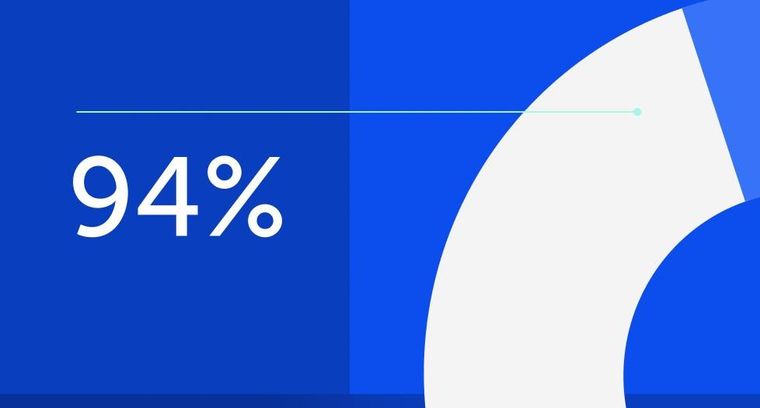
94% of researchers rate our articles as excellent or good
Learn more about the work of our research integrity team to safeguard the quality of each article we publish.
Find out more
REVIEW article
Front. Endocrinol., 22 November 2023
Sec. Cancer Endocrinology
Volume 14 - 2023 | https://doi.org/10.3389/fendo.2023.1265525
This article is part of the Research TopicMetabolic and Senescence Characteristics Associated with the Immune Microenvironment in Tumor CellsView all 18 articles
Ovarian cancer is a highly malignant gynecological cancer influenced by the immune microenvironment, metabolic reprogramming, and cellular senescence. This review provides a comprehensive overview of these characteristics. Metabolic reprogramming affects immune cell function and tumor growth signals. Cellular senescence in immune and tumor cells impacts anti-tumor responses and therapy resistance. Targeting immune cell metabolism and inducing tumor cell senescence offer potential therapeutic strategies. However, challenges remain in identifying specific targets and biomarkers. Understanding the interplay of these characteristics can lead to innovative therapeutic approaches. Further research is needed to elucidate mechanisms, validate strategies, and improve patient outcomes in ovarian cancer.
Ovarian cancer represents a substantial health concern and stands as one of the predominant contributors to cancer-related mortality among women on a global scale. The disease is characterized by its aggressive nature, late-stage diagnosis, and limited therapeutic avenues. The ovaries, responsible for the production of eggs and female hormones, serve as the primary site for the development of ovarian cancer. Existing academic inquiries have provided valuable insights into several pivotal facets of ovarian cancer. Epidemiological investigations have identified diverse risk factors encompassing age, familial history of ovarian or breast cancer, specific genetic mutations such as BRCA1 and BRCA2, as well as hormone-related factors including early menarche and late menopause. These findings have facilitated comprehension of the etiological aspects and identification of high-risk populations, thereby enabling targeted screening and prevention strategies (1, 2).
Furthermore, extensive research has been dedicated to unraveling the molecular mechanisms underpinning the development and progression of ovarian cancer. Studies have divulged multiple genetic aberrations, notably TP53 mutations, MYC and HER2 gene amplification, and dysregulation of the PI3K/AKT and RAS/RAF/MEK/ERK signaling pathways. These molecular alterations contribute to heightened cell proliferation, inhibition of apoptosis, and augmented angiogenesis, ultimately culminating in tumor growth and metastasis (3, 4). The significance of early detection and precise diagnosis of ovarian cancer cannot be overstated. Unfortunately, the disease is frequently detected at advanced stages, characterized by extraneous spread beyond the confines of the ovaries. This occurrence primarily arises due to the absence of specific symptoms and effective screening methodologies. Academic research endeavors have been concentrated on developing and refining diagnostic techniques, such as transvaginal ultrasound, serum biomarkers like CA-125, and more recently, liquid biopsies and molecular profiling. These advancements aim to enhance early detection, bolster diagnostic accuracy, and facilitate personalized treatment strategies (5, 6).
Treatment options for ovarian cancer commonly encompass a combination of surgical intervention, chemotherapy, and targeted therapies. Academic research has played a pivotal role in evaluating the efficacy of diverse treatment modalities while also identifying novel therapeutic targets. The advent of poly (ADP-ribose) polymerase (PARP) inhibitors, for instance, has brought about a revolutionary transformation in ovarian cancer treatment, particularly among patients harboring BRCA mutations. Additionally, immunotherapy, including the utilization of immune checkpoint inhibitors, is currently under exploration as a promising avenue for bolstering the immune response against ovarian cancer cells (7, 8).
In conclusion, ovarian cancer represents a complex ailment bearing substantial clinical ramifications. Academic research has significantly contributed to our understanding of the disease’s etiology, molecular mechanisms, early detection, and treatment options. These advancements hold tremendous potential for improving patient outcomes, augmenting survival rates, and ultimately alleviating the burden associated with this devastating disease. The primary objective of our review is to elucidate the interplay of these characteristics and its potential to drive innovative therapeutic approaches. However, it’s crucial to acknowledge that further research is imperative. This research should focus on unraveling the underlying mechanisms, validating these strategies, and ultimately enhancing patient outcomes in the realm of ovarian cancer.
The immune microenvironment is of paramount importance in the pathogenesis and advancement of ovarian cancer. Numerous academic studies have elucidated diverse facets of this intricate interplay between neoplastic cells and the immune system, thereby providing valuable insights into the mechanisms underlying immune evasion by tumors, infiltration patterns of immune cells, and immunotherapeutic modalities for ovarian cancer. Impairment of immunosurveillance, the process through which the immune system recognizes and eliminates cancer cells, is a common occurrence in ovarian cancer. Extensive scholarly investigations have unveiled multiple mechanisms contributing to immune evasion, encompassing the upregulation of immune checkpoint molecules, such as programmed cell death ligand 1 (PD-L1), on neoplastic cells, and the recruitment of immunosuppressive cell populations like regulatory T cells (Tregs) and myeloid-derived suppressor cells (MDSCs). These mechanisms foster an immunosuppressive microenvironment that facilitates tumor growth and metastasis (9).
Moreover, studies have underscored the significance of immune cell infiltration in ovarian cancer. Tumor-infiltrating lymphocytes (TILs), particularly cytotoxic CD8+ T cells, have demonstrated favorable associations with prognosis and enhanced survival rates in ovarian cancer patients. Conversely, the presence of immunosuppressive cells, such as Tregs and MDSCs, correlates with unfavorable clinical outcomes. The composition and functional status of immune cells within the tumor microenvironment serve as pivotal determinants of disease progression and therapeutic response (10). Furthermore, scholarly research has directed its focus towards harnessing the potential of the immune system through various immunotherapeutic strategies (11–13). Immune checkpoint inhibitors targeting the PD-1/PD-L1 axis and cytotoxic T lymphocyte-associated antigen 4 (CTLA-4) pathway have exhibited promising outcomes in clinical trials, with some patients displaying enduring responses. Combination therapies, entailing the simultaneous administration of immune checkpoint inhibitors, chemotherapy, or targeted agents, are being actively explored to augment treatment efficacy. In addition, the investigation of adoptive cell therapies, including chimeric antigen receptor (CAR) T cell therapy and tumor-infiltrating lymphocyte (TIL) therapy, is underway to evaluate their potential in the treatment of ovarian cancer (14).
A comprehensive comprehension of the dynamic interplay between the immune microenvironment and ovarian cancer has paved the way for personalized immunotherapeutic approaches. Biomarkers such as PD-L1 expression, TILs, and immune gene signatures have been extensively investigated to forecast treatment response and patient outcomes. Furthermore, scholarly research has shed light on the role of the gut microbiome in modulating systemic immune responses, highlighting its potential as a therapeutic target in ovarian cancer (15–18). To conclude, the immune microenvironment exerts a pivotal influence on ovarian cancer, encompassing tumor progression, immune evasion, and treatment response. Academic research has significantly deepened our understanding of the intricate interactions between neoplastic cells and the immune system. These findings have laid the foundation for the development of innovative immunotherapeutic strategies, holding great promise for enhancing outcomes in ovarian cancer patients.
The immune microenvironment has unveiled the pivotal role of metabolic reprogramming in immune cell function. Metabolism plays a vital role in providing energy and biosynthetic precursors necessary for immune cell activation, proliferation, and effector functions. Comprehending the intricate regulation of immune cell metabolism is imperative for unraveling the complex dynamics of immune responses in diverse disease contexts, such as cancer. Among the key metabolic pathways influencing immune cell function, glycolysis stands out prominently. Scholarly investigations have demonstrated that upon activation, immune cells, including T cells, undergo a metabolic shift towards aerobic glycolysis, even under oxygenated conditions. Termed the Warburg effect, this metabolic transition enables immune cells to promptly generate ATP and biosynthetic intermediates to meet their energetic and biosynthetic demands. Crucial players in this process encompass glycolytic enzymes and transporters like hexokinase, glucose transporters (GLUTs), and lactate dehydrogenase (LDH) (19, 20).
Additionally, studies have pinpointed key signaling pathways governing immune cell metabolism. Notably, the mammalian target of rapamycin (mTOR) pathway integrates various signals, including nutrient availability, growth factors, and cytokines, to modulate immune cell metabolism and function. mTOR promotes glycolysis and anabolic processes while suppressing oxidative phosphorylation and catabolic processes in immune cells. Other significant regulators of immune cell metabolism, namely AMP-activated protein kinase (AMPK) and hypoxia-inducible factor 1 alpha (HIF-1α), respond to nutrient and oxygen availability, respectively (21, 22).
Furthermore, metabolic reprogramming in immune cells profoundly impacts immune cell differentiation and effector functions. For instance, effector T cells rely on glycolysis to sustain their robust proliferation and cytokine production, while Tregs preferentially employ oxidative phosphorylation and fatty acid oxidation to support their suppressive function. Metabolic checkpoints, such as the intracellular ATP to AMP ratio, as well as metabolic intermediates like acetyl-CoA and α-ketoglutarate, function as signaling molecules influencing immune cell fate and function (23, 24). Importantly, dysregulation of immune cell metabolism can contribute to immune dysfunction and disease progression. Within the tumor microenvironment, metabolic competition between tumor cells and infiltrating immune cells, along with the presence of immunosuppressive factors, can restrict immune cell metabolism and function. Tumor cells may deprive immune cells of nutrients, secrete metabolites with immunosuppressive effects, or upregulate immune checkpoint molecules that attenuate immune responses. Academic research has underscored the potential of targeting immune cell metabolism to restore immune cell function and augment anti-tumor immunity in cancer immunotherapy (25, 26).
In conclusion, academic research has shed light on the regulation of immune cell metabolism in the immune microenvironment. Understanding the metabolic requirements and adaptations of immune cells assumes paramount importance in deciphering the dynamics of immune responses and devising targeted immunotherapeutic strategies. Further investigations are warranted to elucidate the intricate mechanisms governing immune cell metabolism and its modulation in various disease contexts, including cancer.
Tumor cell metabolism plays a pivotal role in shaping the immune microenvironment by influencing the availability of nutrients, signaling pathways associated with growth, and the functionality of immune cells (Figure 1). Extensive scholarly investigations have elucidated the intricate interplay between tumor cell metabolism and immune responses, shedding light on the mechanisms underlying immune evasion and proposing potential therapeutic approaches. Notably, one of the principal consequences of tumor cell metabolism is the alteration of nutrient availability within the immune microenvironment. Tumor cells exhibit heightened nutrient uptake, particularly glucose and amino acids, to support their rapid proliferation and survival. However, this heightened metabolic demand can deprive infiltrating immune cells of essential nutrients, thereby affecting their activation, proliferation, and effector functions. For instance, glucose competition can restrict the glycolytic capacity of immune cells, impairing their ability to mount an effective anti-tumor response (26, 27).
Figure 1 A comparison of metabolic pathways in ovarian cancer cells under conditions of nutrient abundance and nutrient deprivation revealed distinct metabolic alterations. (A, B) In nutrient-rich conditions, ovarian cancer cells tend to rely on glycolysis and exhibit increased glucose uptake and lactate production. Conversely, under nutrient-deficient conditions, these cells exhibit metabolic adaptations such as enhanced autophagy and utilization of alternative energy sources like fatty acids and amino acids. Understanding these metabolic differences may provide insights into novel therapeutic strategies targeting the specific metabolic vulnerabilities of ovarian cancer cells. (C) A comparison between the primary tumor microenvironment and the ovarian cancer microenvironment reveals distinct differences. In the primary tumor microenvironment, various cell types, including cancer cells, stromal cells, immune cells, and extracellular matrix components, interact to shape tumor progression. However, in the ovarian cancer microenvironment, additional factors like pericytes and specific inflammatory cytokines and chemokines contribute to its unique composition. These variations highlight the importance of considering the specific characteristics of the ovarian cancer microenvironment in understanding disease biology and developing targeted therapeutic approaches.
Furthermore, tumor cells can generate and release metabolites that shape the immune microenvironment. Enhanced glycolysis results in the accumulation of lactate, which suppresses immune cell function. Lactate hampers T cell activation, impairs cytotoxicity, and facilitates the expansion of immunosuppressive cell populations, including MDSCs and Tregs. In addition, tumor-derived metabolites such as kynurenine and adenosine exert immunosuppressive effects by inhibiting T cell proliferation and cytokine production (28, 29). Moreover, tumor cell metabolism influences the signaling pathways associated with growth within the immune microenvironment. Metabolic alterations in tumor cells can lead to the production of growth factors, cytokines, and chemokines that promote both tumor progression and modulation of immune cell behavior. For example, the activation of the mTOR pathway in tumor cells can induce the secretion of VEGF, which facilitates angiogenesis and tumor growth (30). The study investigated the role of mTOR, AP-1, and VEGF in vascular endothelial cell proliferation, showing mTOR downregulation and VEGF upregulation as potential strategies to inhibit restenosis and maintain normal vascular endothelial cell growth after PCI or CABG. This suggests a crucial role for the mTOR/AP-1/VEGF pathway in regulating vascular endothelial cell growth (30). Additionally, tumor cell metabolism can shape the composition and functionality of immune cell populations by releasing factors such as transforming growth factor-beta (TGF-β) and interleukin-10 (IL-10), which suppress immune responses (31, 32).
Moreover, metabolic crosstalk between tumor cells and immune cells can influence the polarization of immune cells and impact functional outcomes. Tumor-associated macrophages (TAMs), for example, undergo metabolic reprogramming in response to tumor-derived signals, leading to their polarization toward an immunosuppressive phenotype (33). This study provides a novel mechanism by which macrophages within a pre-metastatic niche acquire their immunosuppressive phenotype and identifies an important link among exosomes, metabolism, and metastasis (33). Tumor cells also have the capacity to modulate the metabolism of dendritic cells, impairing their ability to present antigens and promoting immune tolerance. These metabolic alterations collectively contribute to the establishment of an immunosuppressive microenvironment that facilitates tumor growth and evades immune surveillance (24, 34). Therefore, a comprehensive understanding of the effects of tumor cell metabolism in the immune microenvironment is imperative for the development of effective therapeutic strategies. Targeting tumor cell metabolism, including glycolysis or specific metabolic pathways, holds promise for overcoming immune suppression and augmenting anti-tumor immunity. Researchers are exploring the combination of metabolic interventions with immunotherapies, such as immune checkpoint blockade or adoptive cell therapies, as a potential approach to enhance treatment outcomes (25, 35).
Tumor cell metabolism exerts significant and multifaceted effects on the immune microenvironment, impacting nutrient availability, growth signaling, and immune cell function. Academic research has considerably deepened our understanding of these complex interactions and revealed potential avenues for therapeutic intervention. However, further studies are warranted to unravel the intricate mechanisms underlying the metabolic crosstalk between tumor cells and immune cells and to translate these findings into effective clinical strategies.
Tumor cells demonstrate metabolic alterations that exert influences on nutrient availability, signaling pathways, and immune cell function, thereby contributing to the progression of tumors and the evasion of immune responses. Notably, a significant consequence of tumor cell metabolism is the reconfiguration of metabolic pathways in immune cells. Through various mechanisms, tumor cells possess the capability to modify the metabolic profiles of infiltrating immune cells, including T cells and macrophages. This reprogramming of immune cell metabolism results in a shift in their metabolic preferences, ultimately impacting their effector functions and fostering an immunosuppressive microenvironment. The Warburg effect, characterized by enhanced glycolytic metabolism, is frequently observed in tumor cells. Consequently, this metabolic phenotype leads to heightened glucose consumption and lactate production. The accumulation of lactate within the tumor microenvironment can induce extracellular acidification, consequently impairing immune cell function.
Additionally, tumor cells can induce metabolic reprogramming in immune cells through the release of immunosuppressive factors and metabolites. For instance, the production of adenosine by tumor cells inhibits T cell proliferation and function by impeding mitochondrial metabolism and attenuating immune responses. Tumor-derived kynurenine, a metabolite of tryptophan, can promote the differentiation of Tregs, thereby further contributing to immune suppression within the tumor microenvironment (36, 37). Moreover, tumor cells can modulate signaling pathways that are involved in immune cell metabolism. Activation of the mTOR pathway in tumor cells influences immune cell metabolism by altering nutrient availability and growth factor signaling. Factors derived from tumor cells, such as VEGF and TGF-β, can foster angiogenesis and immune cell polarization, thereby impacting metabolic reprogramming within the immune microenvironment (27, 32). Comprehensive comprehension of the effects of tumor cell metabolism on the reprogramming of metabolic pathways in the immune microenvironment is of paramount importance in the development of effective therapeutic strategies. Targeting the metabolic vulnerabilities of both tumor cells and immune cells holds immense potential for augmenting anti-tumor immune responses. Ongoing exploration of approaches like metabolic inhibitors, immunomodulatory agents, and combination therapies seeks to overcome immune suppression and enhance treatment outcomes.
The intricate interplay of metabolism between tumor cells and immune cells assumes a pivotal role in shaping immune responses and facilitating tumor progression. Further investigation into the multifaceted mechanisms underlying metabolic reprogramming will undoubtedly advance the development of innovative therapeutic interventions.
Immune cell senescence is characterized by irreversible cell cycle arrest and changes in functional properties, leading to immune dysfunction and compromised anti-tumor immune responses. Replicative senescence, a key characteristic of immune cell senescence, occurs due to cumulative cell divisions and telomere attrition. Senescent immune cells exhibit shortened telomeres, resulting in genomic instability and reduced proliferative capacity. Additionally, senescent immune cells display altered cell cycle regulators, including increased expression of cyclin-dependent kinase inhibitors (CDKIs) such as p16INK4a and p21Cip1/Waf1, contributing to cell cycle arrest (38, 39).
Functional alterations are observed in senescent immune cells, which significantly affect their ability to mount effective immune responses. For instance, senescent T cells exhibit decreased proliferation, impaired cytotoxicity, and compromised production of effector cytokines, such as interferon-gamma (IFN-γ) and tumor necrosis factor-alpha (TNF-α) (Figure 2). Senescent natural killer (NK) cells demonstrate reduced cytotoxicity and impaired cytokine secretion. Similarly, senescent dendritic cells (DCs) exhibit diminished antigen presentation capacity and altered cytokine production, thereby influencing the initiation and regulation of immune responses (40–42). The Senescence-Associated Secretory Phenotype (SASP) is a complex milieu of factors secreted by senescent cells, which play a pivotal role in the tumor microenvironment of ovarian cancer. SASP components include pro-inflammatory cytokines, chemokines, growth factors, and extracellular matrix remodeling enzymes. These factors influence both tumor and immune cells. Pro-inflammatory cytokines, such as IL-6 and IL-8, promote tumorigenesis, angiogenesis, and immunosuppression, fostering a favorable environment for ovarian cancer progression. Chemokines, like CXCL1, attract immune cells to the tumor site, impacting the antitumor immune response. Growth factors, such as EGF and TGF-β, contribute to cell proliferation and immune evasion. Understanding how SASP factors modulate the interactions between tumor and immune cells is critical for developing effective therapeutic strategies against ovarian cancer. The SASP contributes to chronic inflammation within the immune microenvironment, fostering tumor progression and immune dysfunction. Additionally, the SASP can induce senescence in neighboring cells, thereby perpetuating the senescence-associated phenotype (43, 44).
Figure 2 The figure depicts the intricate composition of the microenvironment in ovarian cancer. The ovarian cancer microenvironment encompasses various components, including ovarian cancer cells, stromal cells, immune cells, pericytes, and the extracellular matrix (ECM). The ECM comprises a complex network of inflammatory cytokines, chemokines, and other secreted molecules, contributing to the dynamic interactions within the microenvironment.
Furthermore, the tumor microenvironment and immunosuppressive factors influence immune cell senescence. Factors derived from tumors, such as TGF-β and IL-10, can expedite immune cell senescence and impair their function. Chronic exposure to inflammatory signals present in the tumor microenvironment also contributes to immune cell senescence through the activation of various pathways, including NF-κB and mTOR (45, 46). Gaining insights into the characteristics of immune cell senescence within the immune microenvironment is essential for the development of strategies to overcome immune dysfunction and enhance anti-tumor immune responses. Potential approaches to improving immunotherapeutic outcomes involve targeting senescent immune cells, modulating the SASP, and rejuvenating immune cell function. Furthermore, interventions addressing the root causes of immune cell senescence, such as senescence-inducing factors or DNA damage, hold promise for restoring immune cell functionality (47, 48).
Senescent immune cells exhibit altered proliferative capacity, functional impairments, and the acquisition of a pro-inflammatory secretory phenotype. Understanding the underlying mechanisms of immune cell senescence will pave the way for innovative therapeutic strategies aimed at restoring immune function and enhancing anti-tumor immune responses.
Senescent immune cells manifest functional alterations that actively contribute to immune dysfunction and compromised anti-tumor immune responses, thereby necessitating a comprehensive comprehension of these effects for the advancement of strategies aimed at invigorating immune cell function and enhancing immunotherapeutic approaches. A pivotal repercussion of senescence on immune cell function is the diminished proliferative capacity experienced by these cells. Senescent immune cells undergo an irreversible cell cycle arrest, thereby limiting their potential for expansion and impeding the efficacy of immune responses. This hampered proliferative capacity is primarily attributed to the upregulation of cell cycle inhibitors, notably p16INK4a and p21Cip1/Waf1, which hinder cell cycle progression (38). In addition to reduced proliferative capacity, senescent immune cells exhibit altered functional properties. For instance, senescent T cells display diminished cytotoxicity, impaired cytokine production, and a decreased ability to activate other immune cells. These functional deficiencies compromise the capacity of T cells to eradicate cancer cells and control tumor growth. Similarly, senescent NK cells demonstrate diminished cytotoxicity and compromised production of effector cytokines, thereby impairing their capacity to identify and eliminate target cells (40, 42).
Furthermore, senescence exerts an impact on the immunosurveillance capabilities of immune cells. Senescent immune cells demonstrate impaired antigen presentation capacity, consequently affecting T cell activation and the initiation of immune responses. DCs, which are crucial antigen-presenting cells, undergo functional alterations during senescence, leading to reduced antigen uptake, diminished expression of major histocompatibility complex (MHC) molecules, and altered cytokine production. Consequently, their ability to initiate and regulate immune responses is compromised (41, 49). Senescent immune cells also contribute to chronic inflammation within the immune microenvironment. Senescent cells secrete factors associated with the SASP, including pro-inflammatory cytokines, chemokines, and growth factors, which promote chronic inflammation, further hindering immune cell function and facilitating tumor progression. The SASP can induce senescence in neighboring cells, thereby propagating the senescent phenotype and perpetuating immune dysfunction (43).
Moreover, senescent immune cells can foster an immunosuppressive microenvironment. These cells express inhibitory receptors, such as PD-1, which interact with their ligands, such as PD-L1, resulting in immune cell exhaustion and impaired anti-tumor immune responses. Furthermore, the interaction between senescent immune cells and immunosuppressive cells, including Tregs and MDSCs, contributes to immune suppression within the tumor microenvironment (50). By understanding the effects of senescence on immune cell function, valuable insights into the mechanisms underlying immune dysfunction in aging and cancer can be obtained. Targeting senescent immune cells, modulating the SASP, and rejuvenating immune cell function emerge as potential therapeutic strategies for reinstating immune competence and enhancing anti-tumor immune responses.
Senescence initially functions as a tumor-suppressive mechanism, known as oncogene-induced senescence (OIS), by halting the proliferation of premalignant cells triggered by oncogenic signals or DNA damage. Moreover, OIS promotes immune clearance of these cells, preventing the formation of fully malignant tumors. However, the SASP factors released by senescent cells can paradoxically promote tumor progression. SASP creates a pro-inflammatory microenvironment that supports tumor cell proliferation, survival, and angiogenesis through activation of signaling pathways such as NF-κB and STAT3 (51, 52).
Senescent immune cells, including senescent T cells and NK cells, exhibit compromised cytotoxicity and impaired cytokine production, impairing their ability to eliminate cancer cells. Furthermore, senescent immune cells secrete immunosuppressive factors that facilitate tumor immune evasion and promote tumor progression. Additionally, the accumulation of senescent cells within the tumor microenvironment contributes to tumor growth and therapy resistance. Senescent cells display metabolic reprogramming and enhanced survival mechanisms that promote their persistence in the tumor, while also acquiring stem cell-like properties through senescence-associated stemness. These properties contribute to tumor heterogeneity and therapy resistance (53, 54).
The phenomenon of senescence bypass further complicates the role of senescence in tumor progression. Tumor cells can evade senescence-induced growth arrest through various mechanisms, such as inactivation of tumor suppressor pathways or activation of telomerase. This enables continuous proliferation of tumor cells, thereby contributing to tumor growth and metastasis. Additionally, senescence influences the response to cancer therapy (54). Senescent cells within the tumor microenvironment promote therapy resistance through their pro-survival and immunosuppressive properties (55). Furthermore, the SASP factors secreted by senescent cells impact the tumor microenvironment, thereby influencing the efficacy of chemotherapy, radiation, and immunotherapy (56).
In conclusion, the relationship between senescence and tumor progression is intricate. Although senescence initially acts as a barrier against tumor development through OIS, the presence of SASP and altered immune surveillance associated with senescent cells can paradoxically promote tumor growth, invasion, and therapy resistance. A comprehensive understanding of the interplay between senescence and tumor progression is imperative for the development of targeted therapies that harness the tumor-suppressive aspects of senescence while mitigating its pro-tumorigenic effects.
Metabolic reprogramming plays a crucial role in the development and treatment of ovarian cancer. This reprogramming not only affects tumor cells but also exerts a significant influence on immune cell metabolism and function within the tumor microenvironment. An in-depth comprehension of the regulation of immune cell metabolism is imperative for the advancement of innovative therapeutic strategies that exploit the metabolic vulnerabilities specific to ovarian cancer.
Immune cells undergo metabolic adaptations to fulfill their distinct functions in the immune microenvironment. Notably, activated T cells exhibit a metabolic shift towards aerobic glycolysis, resembling that of tumor cells, in order to support their proliferation and effector functions. Glucose metabolism provides the necessary energy and biosynthetic intermediates for T cell activation, cytokine production, and cytotoxicity (23, 57). Metabolic regulation also affects the differentiation and function of other immune cell populations, such as DCs. DCs, which are pivotal in initiating immune responses, undergo metabolic changes to facilitate their antigen presentation capacity. Specifically, glycolysis and fatty acid oxidation are relied upon by DCs to generate energy and biosynthetic precursors for antigen processing and presentation (58, 59).
Moreover, metabolic alterations within the tumor microenvironment can impact immune cell function and promote immune evasion. Competition for limited nutrient resources between tumor cells and immune cells leads to nutrient deprivation and metabolic stress within the immune microenvironment. Consequently, this nutrient competition impairs immune cell metabolism, compromises immune cell activation, and suppresses anti-tumor immune responses (60, 61).
In addition to nutrient competition, metabolic waste products produced by tumor cells, such as lactate and adenosine, possess immunosuppressive effects.
The metabolic characteristics of immune cells in the tumor microenvironment can be targeted for therapeutic interventions as well. Strategies that enhance oxidative metabolism in T cells or inhibit glycolysis in immunosuppressive cells have demonstrated promise in preclinical models (27, 62).
Understanding the intricate interplay between metabolic reprogramming and immune cell function in ovarian cancer holds paramount importance in the development of effective therapeutic approaches. Targeting metabolic pathways in immune cells, mitigating nutrient competition, and disrupting immunosuppressive metabolic signaling represent potential avenues for augmenting the efficacy of immunotherapy and overcoming immune evasion in ovarian cancer.
Current academic research has emphasized the potential of directing efforts towards tumor cell metabolism as a viable and promising strategy in the advancement and management of ovarian cancer. Distinct metabolic modifications serve as distinguishing features of cancer, including ovarian cancer, and precise targeting of specific metabolic pathways holds significant therapeutic promise. A notable metabolic alteration observed in ovarian cancer is the heightened occurrence of glycolysis, commonly known as the Warburg effect. Ovarian cancer cells exhibit escalated glucose uptake and glycolytic flux to sustain their rapid proliferation and survival. Promising results have been demonstrated in preclinical studies through the targeting of key enzymes involved in glycolysis, such as hexokinase and lactate dehydrogenase, which impedes tumor growth and increases the susceptibility of cancer cells to other treatment modalities (63, 64).
Another crucial metabolic pathway in ovarian cancer is fatty acid metabolism, wherein cancer cells utilize fatty acids as an energy source and as building blocks for membrane synthesis. Inhibition of pivotal enzymes engaged in fatty acid synthesis, such as acetyl-CoA carboxylase and fatty acid synthase, has exhibited anti-tumor effects in models of ovarian cancer (65, 66).
Moreover, the targeting of amino acid metabolism has emerged as a prospective therapeutic strategy. Ovarian cancer cells exhibit heightened uptake and utilization of amino acids to sustain their growth and survival. Inhibiting enzymes involved in amino acid metabolism, such as glutaminase and asparagine synthetase, has demonstrated promise in preclinical models by restricting tumor growth and sensitizing cancer cells to chemotherapy (67).
Additionally, the modulation of the tumor microenvironment to influence nutrient availability and metabolic signaling has shown potential. Disruption of angiogenesis, the process of new blood vessel formation, can restrict nutrient supply to the tumor and impair cancer cell metabolism. Consequently, the targeting of angiogenic pathways, including VEGF signaling, has been explored as a therapeutic approach in the context of ovarian cancer (68).
It is worth noting that the heterogeneous nature of ovarian cancer and the adaptability of metabolic pathways within the tumor necessitate a combination approach when targeting tumor cell metabolism. Current investigations involve combinations of metabolic inhibitors with chemotherapy, targeted therapies, or immunotherapies in order to enhance treatment effectiveness and overcome drug resistance (69).
In conclusion, the targeting of tumor cell metabolism presents a promising avenue for the advancement and management of ovarian cancer. Modulating glycolysis, fatty acid metabolism, amino acid metabolism, and angiogenesis hold potential for impeding tumor growth and increasing the sensitivity of cancer cells to therapy. Integration of metabolic inhibitors with other treatment modalities through combination strategies may yield synergistic effects and ultimately improve patient outcomes.
Immune cell senescence, characterized by irreversible cell cycle arrest and functional decline, exerts a multifaceted influence on ovarian cancer progression and therapeutic approaches. While senescent immune cells have been implicated in tumor promotion, they can also contribute to anti-tumor immune responses. Comprehending the impact of immune cell senescence on ovarian cancer and the potential for targeting senescent cells in immunotherapy is crucial for the development of effective treatment strategies. Accumulation of senescent immune cells, including senescent T cells and NK cells, occurs within the ovarian cancer tumor microenvironment, accompanied by phenotypic and functional alterations. Senescent T cells display reduced proliferation, impaired cytotoxicity, and altered cytokine secretion, leading to compromised anti-tumor activity. Similarly, senescent NK cells exhibit diminished cytotoxicity and cytokine production, impairing their ability to eliminate cancer cells (70). The presence of senescent immune cells in ovarian cancer is associated with immunosuppression and tumor progression. These cells secrete various factors, including components of the SASP, which promote tumor growth, angiogenesis, and immune evasion. SASP factors like interleukin-6 (IL-6) and tTGF-β contribute to the immunosuppressive microenvironment by recruiting Tregs and MDSCs (71, 72).
Despite their tumor-promoting effects, senescent immune cells can also confer benefits in ovarian cancer. Senescent immune cells positive for senescence-associated β-galactosidase (SA-β-gal) can enhance the priming of tumor-specific T cell responses and facilitate the recruitment of effector T cells to the tumor site. Additionally, senescent immune cells secrete chemokines that attract immune effector cells, potentially promoting anti-tumor immune responses (73, 74). Immunotherapy has emerged as a promising avenue for treating ovarian cancer, and researchers are exploring the impact of immune cell senescence on immunotherapeutic strategies. Immune checkpoint inhibitors, such as antibodies targeting PD-1, have demonstrated efficacy in various cancers, including ovarian cancer. However, the presence of senescent immune cells and the immunosuppressive microenvironment can restrict the response to immunotherapy (75, 76). Combining immunotherapy with strategies targeting senescent immune cells holds promise for improving treatment outcomes (Table 1). Selective elimination or rejuvenation of senescent immune cells, using senolytic agents or senescence-reversing interventions, respectively, may enhance the effectiveness of immunotherapy by reducing immunosuppression and restoring immune cell function (77).
In conclusion, immune cell senescence in the context of ovarian cancer elicits both tumor-promoting and anti-tumor effects. Understanding the intricate interplay among senescent immune cells, the tumor microenvironment, and immunotherapy is imperative for the development of effective treatment strategies. Targeting senescent immune cells and modulating the immunosuppressive microenvironment may enhance the outcomes of immunotherapeutic interventions in ovarian cancer.
Tumor cell senescence, a state of permanent growth arrest, has been implicated in the emergence of treatment resistance in ovarian cancer. A growing body of evidence suggests that senescent tumor cells play a role in therapy resistance through diverse mechanisms. Gaining a comprehensive understanding of the link between tumor cell senescence and treatment resistance is crucial for advancing therapeutic strategies in ovarian cancer. Senescent tumor cells exhibit altered characteristics and release a variety of factors collectively referred to as the SASP. The SASP includes pro-inflammatory cytokines, growth factors, and enzymes involved in remodeling the extracellular matrix. These factors can promote cancer cell survival, evasion of the immune system, and resistance to therapy. Consequently, they contribute to the development of a tumor-supportive microenvironment that facilitates tumor cell survival and treatment resistance (71).
One of the mechanisms through which senescent tumor cells foster treatment resistance is by inducing a senescence-associated quiescent state. Senescent cells can enter a dormant state characterized by reduced metabolic activity and heightened resistance to chemotherapy. This quiescent state enables tumor cells to evade the cytotoxic effects of chemotherapy drugs, leading to treatment resistance and the recurrence of the disease (78, 79). Senescent tumor cells can also activate survival signaling pathways, such as the PI3K/Akt and MAPK/ERK pathways, which are associated with resistance to chemotherapy and targeted therapies. These signaling pathways promote cell survival, DNA repair, and the efflux of drugs, thus contributing to treatment resistance and limiting the effectiveness of cancer therapies.
Furthermore, senescent tumor cells can undergo epithelial-mesenchymal transition (EMT), a process linked to increased invasiveness, metastasis, and resistance to chemotherapy. EMT empowers tumor cells to acquire stem-like properties, enhance drug efflux, and evade immune surveillance, thereby causing therapy resistance and disease progression (80, 81). Additionally, senescence-induced alterations in the tumor microenvironment can contribute to treatment resistance. Senescent tumor cells have the ability to modulate the immune microenvironment, promoting the recruitment of immunosuppressive cells. These immunosuppressive cells establish an immune milieu that suppresses anti-tumor immune responses and impedes the effectiveness of immunotherapies (82, 83). By understanding the association between tumor cell senescence and treatment resistance, we can gain insights into potential therapeutic strategies. Targeting senescent tumor cells or modulating the associated pathways could overcome treatment resistance and enhance patient outcomes. Strategies such as senolytic therapy, which selectively eliminates senescent cells, or combination therapies that target both senescent tumor cells and their microenvironment, hold promise for overcoming treatment resistance and improving the efficacy of ovarian cancer therapies (84, 85).
Tumor cell senescence is closely linked to treatment resistance in ovarian cancer. Senescent tumor cells contribute to therapy resistance through various mechanisms, including the induction of a quiescent state, activation of survival signaling pathways, promotion of EMT, and modulation of the tumor microenvironment. Understanding and targeting these senescence-associated mechanisms may present novel opportunities to overcome treatment resistance and enhance therapeutic outcomes in ovarian cancer.
The metabolic milieu of the tumor microenvironment in ovarian cancer exerts a significant influence on the induction of senescence in immune cells. The scarcity of nutrients and oxygen, coupled with the acidic and hypoxic conditions, can incite immune cells (86). Tumor cells often secrete metabolically demanding factors, further exacerbating nutrient competition. Dysfunctional mitochondria in immune cells due to these unfavorable conditions can also trigger senescence.
Senescent immune cells within the tumor microenvironment often exhibit a pro-inflammatory phenotype, secreting cytokines and chemokines. These senescence-associated factors can promote chronic inflammation, favoring tumor growth, angiogenesis, and immune evasion (87, 88). Moreover, senescent immune cells might lose their cytotoxic functions, impairing their ability to target tumor cells effectively. Senescent immune cells may lose their ability to proliferate and become less responsive to antigens. This, in turn, compromises the antitumor immune response. Additionally, the secretion of pro-inflammatory factors by senescent immune cells can contribute to the immunosuppressive character of the tumor microenvironment (89, 90).
Understanding the intricate interplay between the metabolic state, senescence induction in immune cells, and their subsequent effects on both tumor and immune cells is pivotal for developing targeted therapeutic approaches in the context of ovarian cancer.
Immunotherapy has emerged as a promising therapeutic modality in the realm of ovarian cancer treatment, presenting novel opportunities to enhance patient outcomes. This section aims to elucidate recent advancements in immunotherapeutic strategies for ovarian cancer, encompassing immune checkpoint inhibitors, adoptive cell therapies, cancer vaccines, and combination approaches.
Targeting PD-1 and PD-L1, immune checkpoint inhibitors have exhibited clinical efficacy among specific subsets of ovarian cancer patients. Clinical trials have revealed enduring responses and augmented survival rates in patients receiving PD-1/PD-L1 blockade, both as a monotherapy and in conjunction with other agents (8, 91). Significant studies have explored the utilization of pembrolizumab, nivolumab, and atezolizumab within advanced ovarian cancer contexts. Adoptive cell therapies, including chimeric antigen receptor (CAR) T cell therapy and tumor-infiltrating lymphocyte (TIL) therapy, have demonstrated promise for ovarian cancer treatment. CAR T cell therapies that target tumor-associated antigens, such as mesothelin and folate receptor alpha, have shown encouraging outcomes in early-phase clinical trials (92). TIL therapy, involving the isolation and expansion of tumor-infiltrating lymphocytes derived from patient tumors, exhibits potential in generating tumor-specific immune responses (93). Cancer vaccines endeavor to activate the patient’s immune system against tumor antigens. In ovarian cancer, therapeutic vaccines targeting tumor-associated antigens, such as NY-ESO-1 and WT1, have been the subject of investigation in clinical trials. These vaccines are capable of eliciting antigen-specific immune responses and have displayed potential when employed in conjunction with other immunotherapies or chemotherapy (94, 95). The integration of diverse immunotherapy strategies or the amalgamation of immunotherapy with standard treatments has garnered attention in the context of ovarian cancer. Combinations of immune checkpoint inhibitors with chemotherapy, targeted therapies, or other immunotherapies strive to augment antitumor immune responses and surmount resistance mechanisms (96, 97). Ongoing preclinical and clinical investigations aim to identify optimal combination regimens. The identification of predictive biomarkers to discern patients likely to respond to immunotherapy assumes critical importance. PD-L1 expression on tumor cells and tumor-infiltrating immune cells has been explored as a prospective biomarker in ovarian cancer, although its predictive value remains a subject of controversy (98, 99). Other immune-related biomarkers, such as tumor mutational burden and immune cell infiltrates, are under exploration to refine patient selection criteria and enhance treatment outcomes.
In conclusion, immunotherapy exhibits promise as a modality for ovarian cancer treatment. Immune checkpoint inhibitors, adoptive cell therapies, cancer vaccines, and combination approaches present novel avenues to enhance patient outcomes. Further research endeavors should focus on optimizing immunotherapy strategies, identifying predictive biomarkers, and surmounting resistance mechanisms, with the ultimate goal of providing more efficacious and personalized treatments for patients with ovarian cancer.
Despite the encouraging outcomes observed in ovarian cancer immunotherapy, there exist several challenges and areas warranting further investigation. In accordance with extant academic research, this section aims to delineate prospective research avenues and challenges concerning immunotherapy in the context of ovarian cancer.
The persistence of immunotherapy resistance represents a significant hurdle in ovarian cancer treatment. Tumor cells employ diverse mechanisms to elude immune surveillance, encompassing the upregulation of immune checkpoint molecules, immunosuppressive cell populations, and impairment of immune cell functionality. To augment the efficacy of immunotherapy, comprehending these resistance mechanisms and formulating strategies to surmount them is imperative (100). A primary priority lies in the identification of dependable biomarkers that can predict treatment response and facilitate the selection of patients who are most likely to benefit from immunotherapy. Although current biomarkers such as PD-L1 expression have displayed inconsistent predictive value in ovarian cancer, future research should concentrate on identifying novel biomarkers, including immune-related genetic signatures, tumor mutational burden, and immune cell profiles. These biomarkers can guide patient selection and aid in treatment decision-making (101, 102). The integration of immunotherapies with other treatment modalities, such as chemotherapy, targeted therapies, and radiation, holds promise for fortifying antitumor immune responses and circumventing resistance. Optimal combination regimens, treatment sequencing, and timing necessitate investigation through preclinical and clinical studies to maximize therapeutic outcomes (103). An emerging research realm involves the development of personalized immunotherapy approaches tailored to individual patient characteristics. This encompasses leveraging patient-specific tumor antigens, adoptive cell therapies, and neoantigen-based vaccines. Capitalizing on patient-specific immunotherapies has the potential to elicit improved treatment responses and long-term remissions (104, 105). The intricate tumor microenvironment plays a pivotal role in modulating immune responses in ovarian cancer. Deciphering the cellular and molecular constituents of the tumor microenvironment, including immune cell subsets, stromal cells, and extracellular matrix components, is essential for unraveling intricate interactions and devising strategies to manipulate the microenvironment in favor of antitumor immunity (106, 107). Targeted therapies that selectively inhibit key oncogenic pathways have demonstrated efficacy in subsets of ovarian cancer patients. By combining these targeted therapies with immunotherapy, treatment responses can potentially be enhanced by synergistically targeting both tumor cell-intrinsic and immune cell-extrinsic factors (108, 109). As immunotherapy gains recognition as a standard ovarian cancer treatment option, ensuring the long-term safety and monitoring of patients becomes paramount. Research efforts should be directed towards comprehending and managing immune-related adverse events, evaluating the long-term effects on immune function, and developing strategies for achieving durable responses without excessive toxicity (110).
In conclusion, although immunotherapy has exhibited promising results in the treatment of ovarian cancer, further research is necessary to surmount resistance mechanisms, identify reliable biomarkers, optimize combination strategies, and devise personalized approaches. Additionally, comprehending the tumor microenvironment and exploring the integration of immunotherapy with targeted therapies constitute crucial research directions. Moreover, guaranteeing the long-term safety and monitoring of patients undergoing immunotherapy remains a challenge that warrants ongoing investigation.
Ovarian cancer is a complex disease with limited treatment options, and there is a growing interest in harnessing the immune system to improve patient outcomes. In this comprehensive review, we have discussed the metabolic and senescence characteristics associated with the immune microenvironment in ovarian cancer, as well as their implications for tumor development and treatment. We have explored the role of the immune microenvironment, the regulation of immune cell metabolism, the effects of tumor cell metabolism, the reprogramming of metabolic pathways, immune cell senescence, and the relationship between senescence and tumor progression. Additionally, we have examined the potential of these characteristics in ovarian cancer development and treatment, including their impact on immunotherapy and targeted therapies. Lastly, we have outlined the advances in immunotherapy, future research directions, and the challenges that lie ahead.
One of the key findings highlighted in this review is the critical role of the immune microenvironment in ovarian cancer. The tumor microenvironment is composed of various immune cell populations, stromal cells, and extracellular matrix components, which interact with tumor cells to shape the immune response. Understanding the composition and dynamics of the immune microenvironment is essential for designing effective immunotherapeutic strategies. It has been demonstrated that the immune microenvironment in ovarian cancer is characterized by an immunosuppressive phenotype, marked by the presence of regulatory T cells, myeloid-derived suppressor cells, and M2 macrophages. These immune cells create an inhibitory environment that hampers antitumor immune responses.
Furthermore, the metabolic reprogramming of immune cells and tumor cells within the immune microenvironment plays a crucial role in ovarian cancer progression. Immune cells, such as T cells and dendritic cells, rely on specific metabolic pathways to support their activation, proliferation, and effector functions. Tumor cells, on the other hand, exhibit altered metabolic profiles that promote their survival, growth, and immune evasion. By targeting these metabolic pathways, it is possible to modulate immune cell function and enhance antitumor immune responses.
Senescence, a state of irreversible cell cycle arrest, is another important characteristic of the immune microenvironment in ovarian cancer. Senescent immune cells exhibit altered functional properties, including decreased proliferation, impaired cytokine production, and altered immune surveillance. These senescent cells accumulate in the tumor microenvironment and contribute to immune dysfunction and tumor progression. Understanding the effects of senescence on immune cell function is crucial for developing strategies to overcome immune senescence and restore immune competence in ovarian cancer.
The implications of these characteristics for ovarian cancer treatment are significant. Immunotherapy has emerged as a promising therapeutic approach, and several clinical trials have demonstrated encouraging results. However, resistance mechanisms and limited patient selection criteria remain major challenges. Overcoming resistance mechanisms, identifying reliable biomarkers, and optimizing combination strategies are key areas of future research. Personalized immunotherapy approaches tailored to individual patients’ characteristics, such as patient-specific tumor antigens and neoantigen-based vaccines, hold promise for improving treatment outcomes.
Combining immunotherapy with targeted therapies is another avenue to explore. Targeted therapies that inhibit specific oncogenic pathways have shown efficacy in subsets of ovarian cancer patients. Combining these targeted therapies with immunotherapy has the potential to enhance treatment responses by synergistically targeting both tumor cell-intrinsic and immune cell-extrinsic factors.
Despite the advances in immunotherapy, there are still challenges to address. Long-term safety and monitoring of patients receiving immunotherapy are essential, as immune-related adverse events can occur. Additionally, understanding the complex interplay between the tumor microenvironment and the immune system is crucial for designing effective therapeutic strategies.
In conclusion, the metabolic and senescence characteristics associated with the immune microenvironment in ovarian cancer have significant implications for tumor development and treatment. Understanding these characteristics and their interactions is essential for improving patient outcomes. Advances in immunotherapy, personalized approaches, and combination strategies hold promise for enhancing antitumor immune responses in ovarian cancer. However, further research is needed to overcome resistance mechanisms, identify reliable biomarkers, optimize treatment regimens, and ensure long-term safety and monitoring. By addressing these challenges, we can pave the way for more effective and personalized immunotherapeutic approaches for ovarian cancer patients.
JX: Funding acquisition, Writing – original draft. YF: Writing – original draft. JH: Writing – review & editing. YW: Writing – review & editing. XJ: Software, Visualization, Writing – review & editing. XW: Funding acquisition, Visualization, Writing – review & editing. LH: Conceptualization, Writing – review & editing. ZH: Conceptualization, Project administration, Writing – review & editing.
The author(s) declare financial support was received for the research, authorship, and/or publication of this article. This study was funded by the Research foundation of Guangzhou Women and Children’s Medical Center for Clinical Doctor (2020BS027, 2021BS044), Plan on enhancing scientific research in GMU (02-410-2302169XM), and the Science and Technology Program of Guangzhou, China (2023A04J1244).
The authors declare that the research was conducted in the absence of any commercial or financial relationships that could be construed as a potential conflict of interest.
All claims expressed in this article are solely those of the authors and do not necessarily represent those of their affiliated organizations, or those of the publisher, the editors and the reviewers. Any product that may be evaluated in this article, or claim that may be made by its manufacturer, is not guaranteed or endorsed by the publisher.
1. Collaborative Group on Epidemiological Studies of Ovarian Cancer, Beral V, Doll R, Hermon C, Peto R, Reeves G. Ovarian cancer and oral contraceptives: collaborative reanalysis of data from 45 epidemiological studies including 23,257 women with ovarian cancer and 87,303 controls. Lancet (2008) 371(9609):303–14. doi: 10.1016/S0140-6736(08)60167-1
2. Kurman RJ, Shih Ie M. The dualistic model of ovarian carcinogenesis: revisited, revised, and expanded. Am J Pathol (2016) 186(4):733–47. doi: 10.1016/j.ajpath.2015.11.011
3. Cancer Genome Atlas Research Network. Integrated genomic analyses of ovarian carcinoma. Nature (2011) 474(7353):609–15. doi: 10.1038/nature10166
4. Vaughan S, Coward JI, Bast RC Jr, Berchuck A, Berek JS, Brenton JD, et al. Rethinking ovarian cancer: recommendations for improving outcomes. Nat Rev Cancer (2011) 11(10):719–25. doi: 10.1038/nrc3144
5. Buys SS, Partridge E, Black A, Johnson CC, Lamerato L, Isaacs C, et al. Effect of screening on ovarian cancer mortality: the Prostate, Lung, Colorectal and Ovarian (PLCO) Cancer Screening Randomized Controlled Trial. Jama (2011) 305(22):2295–303. doi: 10.1001/jama.2011.766
6. Kurman RJ, Visvanathan K, Roden R, Wu TC, Shih I-M. Early detection and treatment of ovarian cancer: shifting from early stage to minimal volume of disease based on a new model of carcinogenesis. Am J Obstet Gynecol (2008) 198(4):351–6. doi: 10.1016/j.ajog.2008.01.005
7. Pujade-Lauraine E, Korach J, Huzarski T, Byrski T, Pautier P, Friedlander M, et al. Olaparib tablets as maintenance therapy in patients with platinum-sensitive, relapsed ovarian cancer and a BRCA1/2 mutation (SOLO2/ENGOT-Ov21): a double-blind, randomised, placebo-controlled, phase 3 trial. Lancet Oncol (2017) 18(9):1274–84. doi: 10.1016/S1470-2045(17)30469-2
8. Hamanishi J, Mandai M, Ikeda T, Minami M, Kawaguchi A, Murayama T, et al. Safety and antitumor activity of anti-PD-1 antibody, nivolumab, in patients with platinum-resistant ovarian cancer. J Clin Oncol (2015) 33(34):4015–22. doi: 10.1200/JCO.2015.62.3397
9. Hamanishi J, Mandai M, Abiko K, Matsumura N, Baba T, Yoshioka Y, et al. The comprehensive assessment of local immune status of ovarian cancer by the clustering of multiple immune factors. Clin Immunol (2011) 141(3):338–47. doi: 10.1016/j.clim.2011.08.013
10. Zhang L, Conejo-Garcia JR, Katsaros D, Gimotty PA, Massobrio M, Regnani G, et al. Intratumoral T cells, recurrence, and survival in epithelial ovarian cancer. N Engl J Med (2003) 348(3):203–13. doi: 10.1056/NEJMoa020177
11. Martinez A, Delord J-P, Ayyoub M, Devaud C. Preclinical and clinical immunotherapeutic strategies in epithelial ovarian cancer. Cancers (Basel) (2020) 12(7):1761. doi: 10.3390/cancers12071761
12. Yoon H, Kim A, Jang H. Immunotherapeutic approaches in ovarian cancer. Curr Issues Mol Biol (2023) 45(2):1233–49. doi: 10.3390/cimb45020081
13. Launonen IM, Lyytikäinen N, Casado J, Anttila EA, Szabó A, Haltia U-M, et al. Single-cell tumor-immune microenvironment of BRCA1/2 mutated high-grade serous ovarian cancer. Nat Commun (2022) 13(1):835. doi: 10.1038/s41467-022-28389-3
14. Monk BJ, Colombo N, Oza AM, Fujiwara K, Birrer MJ, Randall L, et al. Chemotherapy with or without avelumab followed by avelumab maintenance versus chemotherapy alone in patients with previously untreated epithelial ovarian cancer (JAVELIN Ovarian 100): an open-label, randomised, phase 3 trial. Lancet Oncol (2021) 22(9):1275–89. doi: 10.1016/S1470-2045(21)00342-9
15. Martin DH, Zozaya M, Lillis R, Miller J, Ferris MJ. The microbiota of the human genitourinary tract: trying to see the forest through the trees. Trans Am Clin Climatol Assoc (2012) 123:242–56.
16. Zitvogel L, Ma Y, Raoult D, Kroemer G, Gajewski TF. The microbiome in cancer immunotherapy: Diagnostic tools and therapeutic strategies. Science (2018) 359(6382):1366–70. doi: 10.1126/science.aar6918
17. Hawkins SM, Nephew KP. Unintended consequences of antibiotic therapy on the microbiome delivers a gut punch in ovarian cancer. Cancer Res (2022) 82(24):4511–2. doi: 10.1158/0008-5472.CAN-22-3013
18. Chambers LM, Rhoades ELE, Bharti R, Braley C, Tewari S, Trestan L, et al. Disruption of the gut microbiota confers cisplatin resistance in epithelial ovarian cancer. Cancer Res (2022) 82(24):4654–69. doi: 10.1158/0008-5472.CAN-22-0455
19. Pearce EL, Pearce EJ. Metabolic pathways in immune cell activation and quiescence. Immunity (2013) 38(4):633–43. doi: 10.1016/j.immuni.2013.04.005
20. Wang R, Green DR. Metabolic reprogramming and metabolic dependency in T cells. Immunol Rev (2012) 249(1):14–26. doi: 10.1111/j.1600-065X.2012.01155.x
21. Laplante M, Sabatini DM. mTOR signaling in growth control and disease. Cell (2012) 149(2):274–93. doi: 10.1016/j.cell.2012.03.017
22. Gunton JE. Hypoxia-inducible factors and diabetes. J Clin Invest (2020) 130(10):5063–73. doi: 10.1172/JCI137556
23. Chang CH, Pearce EL. Emerging concepts of T cell metabolism as a target of immunotherapy. Nat Immunol (2016) 17(4):364–8. doi: 10.1038/ni.3415
24. O'Neill LA, Kishton RJ, Rathmell J. A guide to immunometabolism for immunologists. Nat Rev Immunol (2016) 16(9):553–65. doi: 10.1038/nri.2016.70
25. Scharping NE, Delgoffe GM. Tumor microenvironment metabolism: A new checkpoint for anti-tumor immunity. Vaccines (Basel) (2016) 4(4):46. doi: 10.3390/vaccines4040046
26. Ho PC, Bihuniak JD, Macintyre AN, Staron M, Liu X, Amezquita R, et al. Phosphoenolpyruvate is a metabolic checkpoint of anti-tumor T cell responses. Cell (2015) 162(6):1217–28. doi: 10.1016/j.cell.2015.08.012
27. Chang CH, Qiu J, O'Sullivan D, Buck MD, Noguchi T, Curtis JD, et al. Metabolic competition in the tumor microenvironment is a driver of cancer progression. Cell (2015) 162(6):1229–41. doi: 10.1016/j.cell.2015.08.016
28. Fischer K, Hoffmann P, Voelkl S, Meidenbauer N, Ammer J, Edinger M, et al. Inhibitory effect of tumor cell-derived lactic acid on human T cells. Blood (2007) 109(9):3812–9. doi: 10.1182/blood-2006-07-035972
29. Kachikwu EL, Iwamoto KS, Liao Y-P, DeMarco JJ, Agazaryan N, Economou JS, et al. Radiation enhances regulatory T cell representation. Int J Radiat Oncol Biol Phys (2011) 81(4):1128–35. doi: 10.1016/j.ijrobp.2010.09.034
30. Wang S, Lu J, You Q, Huang H, Chen Y, Liu K, et al. The mTOR/AP-1/VEGF signaling pathway regulates vascular endothelial cell growth. Oncotarget (2016) 7(33):53269–76. doi: 10.18632/oncotarget.10756
31. Curiel TJ, Wei S, Dong H, Alvarez X, Cheng P, Mottram P, et al. Blockade of B7-H1 improves myeloid dendritic cell-mediated antitumor immunity. Nat Med (2003) 9(5):562–7. doi: 10.1038/nm863
32. Sceneay J, Smyth MJ, Möller A. The pre-metastatic niche: finding common ground. Cancer Metastasis Rev (2013) 32(3-4):449–64. doi: 10.1007/s10555-013-9420-1
33. Morrissey SM, Zhang F, Ding C, Montoya-Durango DE, Hu X, Yang C, et al. Tumor-derived exosomes drive immunosuppressive macrophages in a pre-metastatic niche through glycolytic dominant metabolic reprogramming. Cell Metab (2021) 33(10):2040–2058.e10. doi: 10.1016/j.cmet.2021.09.002
34. Colegio OR, Chu N-Q, Szabo AL, Chu T, Rhebergen AM, Jairam V, et al. Functional polarization of tumour-associated macrophages by tumour-derived lactic acid. Nature (2014) 513(7519):559–63. doi: 10.1038/nature13490
35. Lemos H, Mohamed E, Huang L, Ou R, Pacholczyk G, Arbab AS, et al. STING promotes the growth of tumors characterized by low antigenicity via IDO activation. Cancer Res (2016) 76(8):2076–81. doi: 10.1158/0008-5472.CAN-15-1456
36. Vaupel P, Multhoff G. Adenosine can thwart antitumor immune responses elicited by radiotherapy : Therapeutic strategies alleviating protumor ADO activities. Strahlenther Onkol (2016) 192(5):279–87. doi: 10.1007/s00066-016-0948-1
37. Liu XQ, Wang X. Indoleamine 2,3-dioxygenase in tumor induced tolerance. Chin Med J (Engl) (2009) 122(24):3072–7.
38. Effros RB. Replicative senescence of CD8 T cells: effect on human ageing. Exp Gerontol (2004) 39(4):517–24. doi: 10.1016/j.exger.2003.09.024
39. Liu Y, Sanoff HK, Cho H, Burd CE, Torrice C, Ibrahim JG, et al. Expression of p16(INK4a) in peripheral blood T-cells is a biomarker of human aging. Aging Cell (2009) 8(4):439–48. doi: 10.1111/j.1474-9726.2009.00489.x
40. Wikby A, Nilsson B-O, Forsey R, Thompson J, Strindhall J, Löfgren S, et al. The immune risk phenotype is associated with IL-6 in the terminal decline stage: findings from the Swedish NONA immune longitudinal study of very late life functioning. Mech Ageing Dev (2006) 127(8):695–704. doi: 10.1016/j.mad.2006.04.003
41. Sadighi Akha AA. Aging and the immune system: An overview. J Immunol Methods (2018) 463:21–6. doi: 10.1016/j.jim.2018.08.005
42. Focosi D, Bestagno M, Burrone O, Petrini M. CD57+ T lymphocytes and functional immune deficiency. J Leukoc Biol (2010) 87(1):107–16. doi: 10.1189/jlb.0809566
43. Coppé JP, Desprez P-Y, Krtolica A, Campisi J. The senescence-associated secretory phenotype: the dark side of tumor suppression. Annu Rev Pathol (2010) 5:99–118. doi: 10.1146/annurev-pathol-121808-102144
44. Coppé JP, Patil CK, Rodier F, Krtolica A, Beauséjour CM, Parrinello S, et al. A human-like senescence-associated secretory phenotype is conserved in mouse cells dependent on physiological oxygen. PloS One (2010) 5(2):e9188. doi: 10.1371/journal.pone.0009188
45. Braumüller H, Wieder T, Brenner E, Aßmann S, Hahn M, Alkhaled M, et al. T-helper-1-cell cytokines drive cancer into senescence. Nature (2013) 494(7437):361–5. doi: 10.1038/nature11824
46. Laberge RM, Sun Y, Orjalo AV, Patil CK, Freund A, Zhou L, et al. MTOR regulates the pro-tumorigenic senescence-associated secretory phenotype by promoting IL1A translation. Nat Cell Biol (2015) 17(8):1049–61. doi: 10.1038/ncb3195
47. Herbig U, Ferreira M, Condel L, Carey D, Sedivy JM. Cellular senescence in aging primates. Science (2006) 311(5765):1257. doi: 10.1126/science.1122446
48. Palmer AK, Xu M, Zhu Y, Pirtskhalava T, Weivoda MM, Hachfeld CM, et al. Targeting senescent cells alleviates obesity-induced metabolic dysfunction. Aging Cell (2019) 18(3):e12950. doi: 10.1111/acel.12950
49. Sathaliyawala T, Kubota M, Yudanin N, Turner D, Camp P, Thome JJC, et al. Distribution and compartmentalization of human circulating and tissue-resident memory T cell subsets. Immunity (2013) 38(1):187–97. doi: 10.1016/j.immuni.2012.09.020
50. Palmer AK, Gustafson B, Kirkland JL, Smith U. Cellular senescence: at the nexus between ageing and diabetes. Diabetologia (2019) 62(10):1835–41. doi: 10.1007/s00125-019-4934-x
51. Collado M, Serrano M. Senescence in tumours: evidence from mice and humans. Nat Rev Cancer (2010) 10(1):51–7. doi: 10.1038/nrc2772
52. Kuilman T, Michaloglou C, Mooi WJ, Peeper DS. The essence of senescence. Genes Dev (2010) 24(22):2463–79. doi: 10.1101/gad.1971610
53. Radspieler MM, Schindeldecker M, Stenzel P, Försch S, Tagscherer KE, Herpel E, et al. Lamin-B1 is a senescence-associated biomarker in clear-cell renal cell carcinoma. Oncol Lett (2019) 18(3):2654–60. doi: 10.3892/ol.2019.10593
54. Xue W, Zender L, Miething C, Dickins RA, Hernando E, Krizhanovsky V, et al. Senescence and tumour clearance is triggered by p53 restoration in murine liver carcinomas. Nature (2007) 445(7128):656–60. doi: 10.1038/nature05529
55. Mosteiro L, Pantoja C, Alcazar N, Marión RM, Chondronasiou D, Rovira M, et al. Tissue damage and senescence provide critical signals for cellular reprogramming in vivo. Science (2016) 354(6315):aaf4445. doi: 10.1126/science.aaf4445
56. Acosta JC, O'Loghlen A, Banito A, Guijarro MV, Augert A, Raguz S, et al. Chemokine signaling via the CXCR2 receptor reinforces senescence. Cell (2008) 133(6):1006–18. doi: 10.1016/j.cell.2008.03.038
57. Siska PJ, Rathmell JC. T cell metabolic fitness in antitumor immunity. Trends Immunol (2015) 36(4):257–64. doi: 10.1016/j.it.2015.02.007
58. Everts B, Amiel E, Huang SC-C, Smith AM, Chang C-H, Lam WY, et al. TLR-driven early glycolytic reprogramming via the kinases TBK1-IKKε supports the anabolic demands of dendritic cell activation. Nat Immunol (2014) 15(4):323–32. doi: 10.1038/ni.2833
59. Pearce EL, Poffenberger MC, Chang C-H, Jones RG. Fueling immunity: insights into metabolism and lymphocyte function. Science (2013) 342(6155):1242454. doi: 10.1126/science.1242454
60. Ho PC, Liu PS. Metabolic communication in tumors: a new layer of immunoregulation for immune evasion. J Immunother Cancer (2016) 4:4. doi: 10.1186/s40425-016-0109-1
61. Swartz MA, Iida N, Roberts EW, Sangaletti S, Wong MH, Yull FE, et al. Tumor microenvironment complexity: emerging roles in cancer therapy. Cancer Res (2012) 72(10):2473–80. doi: 10.1158/0008-5472.CAN-12-0122
62. Buck MD, O'Sullivan D, Pearce EL. T cell metabolism drives immunity. J Exp Med (2015) 212(9):1345–60. doi: 10.1084/jem.20151159
63. Matassa DS, Amoroso MR, Lu H, Avolio R, Arzeni D, Procaccini C, et al. Oxidative metabolism drives inflammation-induced platinum resistance in human ovarian cancer. Cell Death Differ (2016) 23(9):1542–54. doi: 10.1038/cdd.2016.39
64. Lu W, Sun J, Yoon JS, Zhang Y, Zheng L, Murphy E, et al. Mitochondrial protein PGAM5 regulates mitophagic protection against cell necroptosis. PloS One (2016) 11(1):e0147792. doi: 10.1371/journal.pone.0147792
65. Menendez JA, Lupu R. Fatty acid synthase and the lipogenic phenotype in cancer pathogenesis. Nat Rev Cancer (2007) 7(10):763–77. doi: 10.1038/nrc2222
66. Zaidi N, Lupien L, Kuemmerle NB, Kinlaw WB, Swinnen JV, Smans K, et al. Lipogenesis and lipolysis: the pathways exploited by the cancer cells to acquire fatty acids. Prog Lipid Res (2013) 52(4):585–9. doi: 10.1016/j.plipres.2013.08.005
67. Chen H, Liu H, Qing G. Targeting oncogenic Myc as a strategy for cancer treatment. Signal Transduct Target Ther (2018) 3:5. doi: 10.1038/s41392-018-0008-7
68. Annese T, Tamma R, Ruggieri S, Ribatti D. Erythropoietin in tumor angiogenesis. Exp Cell Res (2019) 374(2):266–73. doi: 10.1016/j.yexcr.2018.12.013
69. Monk BJ, Poveda A, Vergote I, Raspagliesi F, Fujiwara K, Bae D-S, et al. Anti-angiopoietin therapy with trebananib for recurrent ovarian cancer (TRINOVA-1): a randomised, multicentre, double-blind, placebo-controlled phase 3 trial. Lancet Oncol (2014) 15(8):799–808. doi: 10.1016/S1470-2045(14)70244-X
70. Chou JP, Effros RB. T cell replicative senescence in human aging. Curr Pharm Des (2013) 19(9):1680–98. doi: 10.2174/138161213805219711
71. Acosta JC, Banito A, Wuestefeld T, Georgilis A, Janich P, Morton JP, et al. A complex secretory program orchestrated by the inflammasome controls paracrine senescence. Nat Cell Biol (2013) 15(8):978–90. doi: 10.1038/ncb2784
72. Krtolica A, Parrinello S, Lockett S, Desprez PY, Campisi J. Senescent fibroblasts promote epithelial cell growth and tumorigenesis: a link between cancer and aging. Proc Natl Acad Sci USA (2001) 98(21):12072–7. doi: 10.1073/pnas.211053698
73. Campisi J, d'Adda di Fagagna F. Cellular senescence: when bad things happen to good cells. Nat Rev Mol Cell Biol (2007) 8(9):729–40. doi: 10.1038/nrm2233
74. Sagiv A, Krizhanovsky V. Immunosurveillance of senescent cells: the bright side of the senescence program. Biogerontology (2013) 14(6):617–28. doi: 10.1007/s10522-013-9473-0
75. Doroshow DB, Bhalla S, Beasley MB, Sholl LM, Kerr KM, Gnjatic S, et al. PD-L1 as a biomarker of response to immune-checkpoint inhibitors. Nat Rev Clin Oncol (2021) 18(6):345–62. doi: 10.1038/s41571-021-00473-5
76. Pardoll DM. The blockade of immune checkpoints in cancer immunotherapy. Nat Rev Cancer (2012) 12(4):252–64. doi: 10.1038/nrc3239
77. Zhu Y, Doornebal EJ, Pirtskhalava T, Giorgadze N, Wentworth M, Fuhrmann-Stroissnigg H, et al. New agents that target senescent cells: the flavone, fisetin, and the BCL-X(L) inhibitors, A1331852 and A1155463. Aging (Albany NY) (2017) 9(3):955–63. doi: 10.18632/aging.101202
78. Sosa MS, Bragado P, Aguirre-Ghiso JA. Mechanisms of disseminated cancer cell dormancy: an awakening field. Nat Rev Cancer (2014) 14(9):611–22. doi: 10.1038/nrc3793
79. Milanovic M, Fan DNY, Belenki D, Däbritz JHM, Zhao Z, Yu Y, et al. Senescence-associated reprogramming promotes cancer stemness. Nature (2018) 553(7686):96–100. doi: 10.1038/nature25167
80. Ye X, Weinberg RA. Epithelial-mesenchymal plasticity: A central regulator of cancer progression. Trends Cell Biol (2015) 25(11):675–86. doi: 10.1016/j.tcb.2015.07.012
81. Li J, Zhou BP. Activation of β-catenin and Akt pathways by Twist are critical for the maintenance of EMT associated cancer stem cell-like characters. BMC Cancer (2011) 11:49. doi: 10.1186/1471-2407-11-49
82. Malaquin N, Vercamer C, Bouali F, Martien S, Deruy E, Wernert N, et al. Senescent fibroblasts enhance early skin carcinogenic events via a paracrine MMP-PAR-1 axis. PloS One (2013) 8(5):e63607. doi: 10.1371/journal.pone.0063607
83. Ruhland MK, Loza AJ, Capietto A-H, Luo X, Knolhoff BL, Flanagan KC, et al. Stromal senescence establishes an immunosuppressive microenvironment that drives tumorigenesis. Nat Commun (2016) 7:11762. doi: 10.1038/ncomms11762
84. Zhu Y, Tchkonia T, Fuhrmann-Stroissnigg H, Dai HM, Ling YY, Stout MB, et al. Identification of a novel senolytic agent, navitoclax, targeting the Bcl-2 family of anti-apoptotic factors. Aging Cell (2016) 15(3):428–35. doi: 10.1111/acel.12445
85. Demaria M, O'Leary MN, Chang J, Shao L, Liu S, Alimirah F, et al. Cellular senescence promotes adverse effects of chemotherapy and cancer relapse. Cancer Discovery (2017) 7(2):165–76. doi: 10.1158/2159-8290.CD-16-0241
86. Koizume S, Ito S, Nakamura Y, Yoshihara M, Furuya M, Yamada R, et al. Lipid starvation and hypoxia synergistically activate ICAM1 and multiple genes in an Sp1-dependent manner to promote the growth of ovarian cancer. Mol Cancer (2015) 14:77. doi: 10.1186/s12943-015-0351-z
87. Ferraresi A, Girone C, Esposito A, Vidoni C, Vallino L, Secomandi E, et al. How autophagy shapes the tumor microenvironment in ovarian cancer. Front Oncol (2020) 10:599915. doi: 10.3389/fonc.2020.599915
88. Luo Z, Wang Q, Lau WB, Lau B, Xu L, Zhao L, et al. Tumor microenvironment: The culprit for ovarian cancer metastasis? Cancer Lett (2016) 377(2):174–82. doi: 10.1016/j.canlet.2016.04.038
89. Sánchez-Prieto M, Sánchez-Borrego R, Lubián-López DM, Pérez-López FR. Etiopathogenesis of ovarian cancer. An inflamm-aging entity? Gynecol Oncol Rep (2022) 42:101018.
90. Hao X, Zhao B, Zhou W, Liu H, Fukumoto T, Gabrilovich D, et al. Sensitization of ovarian tumor to immune checkpoint blockade by boosting senescence-associated secretory phenotype. iScience (2021) 24(1):102016. doi: 10.1016/j.isci.2020.102016
91. Pujade-Lauraine E, Fujiwara K, Dychter SS, Devgan G, Monk BJ. Avelumab (anti-PD-L1) in platinum-resistant/refractory ovarian cancer: JAVELIN Ovarian 200 Phase III study design. Future Oncol (2018) 14(21):2103–13. doi: 10.2217/fon-2018-0070
92. Hassan R, Thomas A, Alewine C, Le DT, Jaffee EM, Pastan I, et al. Mesothelin immunotherapy for cancer: ready for prime time? J Clin Oncol (2016) 34(34):4171–9. doi: 10.1200/JCO.2016.68.3672
93. Stevanović S, Draper LM, Langhan MM, Campbell TE, Kwong ML, Wunderlich JR, et al. Complete regression of metastatic cervical cancer after treatment with human papillomavirus-targeted tumor-infiltrating T cells. J Clin Oncol (2015) 33(14):1543–50. doi: 10.1200/JCO.2014.58.9093
94. Odunsi K, Qian F, Matsuzaki J, Mhawech-Fauceglia P, Andrews C, Hoffman EW, et al. Vaccination with an NY-ESO-1 peptide of HLA class I/II specificities induces integrated humoral and T cell responses in ovarian cancer. Proc Natl Acad Sci USA (2007) 104(31):12837–42. doi: 10.1073/pnas.0703342104
95. Dohi S, Ohno S, Ohno Y, Takakura M, Kyo S, Soma G-I, et al. WT1 peptide vaccine stabilized intractable ovarian cancer patient for one year: a case report. Anticancer Res (2011) 31(7):2441–5.
96. Konstantinopoulos PA, Waggoner S, Vidal GA, Mita M, Moroney JW, Holloway R, et al. Single-arm phases 1 and 2 trial of niraparib in combination with pembrolizumab in patients with recurrent platinum-resistant ovarian carcinoma. JAMA Oncol (2019) 5(8):1141–9. doi: 10.1001/jamaoncol.2019.1048
97. Zamarin D, Burger RA, Sill MW, Powell DJ Jr, Lankes HA, Feldman MD, et al. Randomized phase II trial of nivolumab versus nivolumab and ipilimumab for recurrent or persistent ovarian cancer: an NRG oncology study. J Clin Oncol (2020) 38(16):1814–23. doi: 10.1200/JCO.19.02059
98. Darb-Esfahani S, Kunze CA, Kulbe H, Sehouli J, Wienert S, Lindner J, et al. Prognostic impact of programmed cell death-1 (PD-1) and PD-ligand 1 (PD-L1) expression in cancer cells and tumor-infiltrating lymphocytes in ovarian high grade serous carcinoma. Oncotarget (2016) 7(2):1486–99. doi: 10.18632/oncotarget.6429
99. Webb JR, Milne K, Nelson BH. PD-1 and CD103 are widely coexpressed on prognostically favorable intraepithelial CD8 T cells in human ovarian cancer. Cancer Immunol Res (2015) 3(8):926–35. doi: 10.1158/2326-6066.CIR-14-0239
100. Vonderheide RH. The immune revolution: A case for priming, not checkpoint. Cancer Cell (2018) 33(4):563–9. doi: 10.1016/j.ccell.2018.03.008
101. Colvin EK. Tumor-associated macrophages contribute to tumor progression in ovarian cancer. Front Oncol (2014) 4:137. doi: 10.3389/fonc.2014.00137
102. Zhang AW, McPherson A, Milne K, Kroeger DR, Hamilton PT, Miranda A, et al. Interfaces of Malignant and immunologic clonal dynamics in ovarian cancer. Cell (2018) 173(7):1755–1769.e22. doi: 10.1016/j.cell.2018.03.073
103. Tang J, Shalabi A, Hubbard-Lucey VM. Comprehensive analysis of the clinical immuno-oncology landscape. Ann Oncol (2018) 29(1):84–91. doi: 10.1093/annonc/mdx755
104. Tran E, Robbins PF, Lu Y-C, Prickett TD, Gartner JJ, Jia L, et al. T-cell transfer therapy targeting mutant KRAS in cancer. N Engl J Med (2016) 375(23):2255–62. doi: 10.1056/NEJMoa1609279
105. Ott PA, Hu Z, Keskin DB, Shukla SA, Sun J, Bozym DJ, et al. An immunogenic personal neoantigen vaccine for patients with melanoma. Nature (2017) 547(7662):217–21. doi: 10.1038/nature22991
106. Binnewies M, Roberts EW, Kersten K, Chan V, Fearon DF, Merad M, et al. Understanding the tumor immune microenvironment (TIME) for effective therapy. Nat Med (2018) 24(5):541–50. doi: 10.1038/s41591-018-0014-x
107. Balkwill FR, Capasso M, Hagemann T. The tumor microenvironment at a glance. J Cell Sci (2012) 125(Pt 23):5591–6. doi: 10.1242/jcs.116392
108. Chen G, Emens LA. Chemoimmunotherapy: reengineering tumor immunity. Cancer Immunol Immunother (2013) 62(2):203–16. doi: 10.1007/s00262-012-1388-0
109. Burger RA, Brady MF, Bookman MA, Fleming GF, Monk BJ, Huang H, et al. Incorporation of bevacizumab in the primary treatment of ovarian cancer. N Engl J Med (2011) 365(26):2473–83. doi: 10.1056/NEJMoa1104390
Keywords: ovarian cancer, metabolism, senescence, immune microenvironment, therapeutic strategies
Citation: Xiong J, Fu Y, Huang J, Wang Y, Jin X, Wan X, Huang L and Huang Z (2023) Metabolic and senescence characteristics associated with the immune microenvironment in ovarian cancer. Front. Endocrinol. 14:1265525. doi: 10.3389/fendo.2023.1265525
Received: 23 July 2023; Accepted: 31 October 2023;
Published: 22 November 2023.
Edited by:
Houjuan Zhu, Institute of Materials Research and Engineering (A*STAR), SingaporeReviewed by:
Lin Zhang, Clinical Center (NIH), United StatesCopyright © 2023 Xiong, Fu, Huang, Wang, Jin, Wan, Huang and Huang. This is an open-access article distributed under the terms of the Creative Commons Attribution License (CC BY). The use, distribution or reproduction in other forums is permitted, provided the original author(s) and the copyright owner(s) are credited and that the original publication in this journal is cited, in accordance with accepted academic practice. No use, distribution or reproduction is permitted which does not comply with these terms.
*Correspondence: Zheng Huang, aHozMTMzQDE2My5jb20=; Liu Huang, aGx6cXp5QDEyNi5jb20=
†These authors have contributed equally to this work
Disclaimer: All claims expressed in this article are solely those of the authors and do not necessarily represent those of their affiliated organizations, or those of the publisher, the editors and the reviewers. Any product that may be evaluated in this article or claim that may be made by its manufacturer is not guaranteed or endorsed by the publisher.
Research integrity at Frontiers
Learn more about the work of our research integrity team to safeguard the quality of each article we publish.