- Department of Endocrinology, The Affiliated Hospital of Qingdao University, Qingdao, China
Worldwide, diabetes and its complications have seriously affected people’s quality of life and become a serious public health problem. C-peptide is not only an indicator of pancreatic β-cell function, but also a biologically active peptide that can bind to cell membrane surface signaling molecules and activate downstream signaling pathways to play antioxidant, anti-apoptotic and inflammatory roles, or regulate cellular transcription through internalization. It is complex how C-peptide is related to diabetic complications. Both deficiencies and overproduction can lead to complications, but their mechanisms of action may be different. C-peptide replacement therapy has shown beneficial effects on diabetic complications in animal models when C-peptide is deficient, but results from clinical trials have been unsatisfactory. The complex pattern of the relationship between C-peptide and diabetic chronic complications has not yet been fully understood. Future basic and clinical studies of C-peptide replacement therapies will need to focus on baseline levels of C-peptide in addition to more attention also needs to be paid to post-treatment C-peptide levels to explore the optimal range of fasting C-peptide and postprandial C-peptide maintenance.
1 Introduction
Globally, the incidence of diabetes is rapidly increasing, affecting almost 537million people, with approximately 109.6 million in China (1–3). Under hyperglycemic conditions, protein and lipid glycosylation, overproduction of reactive oxygen species (ROS), tissue expression of pro-inflammatory factors, and damage to vascular endothelium all promote the occurrence of diabetic complications (4). As diabetes progresses, a variety of complications occur, such as retinopathy, kidney disease, neuropathy, cardiovascular disease, and so forth. The complications of diabetes have become a serious global health issue that significantly impacts people’s quality of life (5) The leading cause of end-stage renal disease is diabetic kidney disease (DKD), as diabetes prevalence increases, the global incidence of end-stage kidney disease is on the rise (6). Diabetes is a leading cause of death due to myocardial infarction, and The risk of coronary heart disease in diabetic patients without a history of coronary disease is similar to that in non-diabetic patients with a history of coronary disease (7). Current clinical trials are increasingly focused on the impact of novel hypoglycemic drugs on cardiovascular events and renal prognosis, and the focus of diabetes management has shifted from merely controlling blood glucose to impacting cardiovascular and renal prognosis. However, there is still no definitive treatment to reverse diabetic complications. Even if diabetic patients are given intensified glycemic control at an early stage, the occurrence of complications is still unavoidable. Therefore, finding treatments to prevent and reverse diabetic complications is a key focus of the comprehensive management of type 2 diabetes mellitus (T2DM). As beta cells secrete C-peptide and insulin at equimolar levels, C-peptide can serve as an indicator of beta-cell reserve function. In the years following C-peptide’s discovery, the traditional view is that its biological activity promotes the folding of proinsulin within beta cells. However, according to research evidence collected over the past 20 years, C-peptide is also a biologically active peptide that may influence diabetic complications (8). A normal result of a C-peptide test ranges from 0.5 ng/mL to 2.0 ng/mL (or 0.17 to 0.83 nmol/L), which may differ slightly from lab to lab. C-peptide can bind with cell membrane surface signaling molecules, activate downstream signaling pathways, play anti-oxidative, anti-apoptotic roles, regulate inflammatory responses, or regulate cellular transcription through internalization (9). In type 1 diabetes mellitus (T1DM), C-peptide and insulin are deficient, and progressive beta-cell dysfunction can also be observed in the late stage of T2DM. Evidence from animal and in vitro experiments shows that when C-peptide is deficient, C-peptide replacement therapy can improve renal lesions (10), retinopathy (11), and peripheral neuropathy (12) by exerting anti-inflammatory, anti-apoptotic, and anti-oxidative effects. In the early stages of T2DM, C-peptide secretion is higher than physiological concentration, and C-peptide can also exert a pro-inflammatory effect, damaging vascular endothelium, and promoting vascular lesion occurrence (13, 14). On one hand, further research is needed to determine the relationship between C-peptide and diabetic complications. Both deficiency and over-secretion of C-peptide can promote the onset of diabetic complications, but the underlying mechanisms might differ. On the other hand, the biological activity of C-peptide can be a new breakthrough for the treatment and prevention of diabetic complications. As a result, we have summarized the current research progress on the relationship between C-peptide and diabetic complications as follows:
2 Physiological functions of C-peptide
2.1 C-peptide and cell signal transduction
Some studies indicate that C-peptide can function as a classical peptide hormone, binding to G-protein-coupled receptors on the cell surface to initiate cascading cellular signal transduction reactions (15). However, other research suggests that C-peptide also has some non-classical intracellular functions. C-peptide demonstrates a specific binding affinity towards the cell membrane, thereby initiating intracellular signal transduction via G-protein receptors and Ca2+-dependent pathways. This process consequently enhances the activity of endothelial nitric oxide synthase (eNOS), Na+/K+/ATPase, and phosphatidylinositol-3 kinase (PI-3-K). Furthermore, it activates the mitogen-activated protein kinases (MAPK) pathway and augments the activation and expression of particular transcription factors. These outcomes hold significant implications for anti-inflammatory, anti-oxidative, and cellular protective mechanisms (16). The signaling pathways involved in C-peptide mainly include: Ca2+ dependent pathway, p38 mitogen-activated protein kinase, extracellular signal-regulated kinase-1/2 (Erk-1/2), Akt phosphorylation, and production of endothelial cell nitric oxide (NO) (17, 18).
Lindahl’s research indicates that C-peptide can be internalized in target cells. C-peptide is internalized and located in the cytoplasm of Swiss 3T3 and HEK-293 cells and can bind with cytoplasmic proteins. Labeling revealed that C-peptide is transported into the cell nucleus (19). Subsequent investigations revealed that C-peptide is capable of associating with histones present in the nucleolus, thereby enhancing the acetylation at the lysine residue 16 of histone H4 located in the promoter region of ribosomal RNA genes. This particular interaction facilitates the transcription of genes responsible for encoding ribosomal RNA (20).
The cellular biology of C-peptide is largely unknown, especially concerning the peptide’s pathway across the cell membrane for internalization. Research by Luppi and colleagues demonstrated the co-localization of C-peptide with early endosome markers that support endocytosis (8). The pathway of C-peptide internalization is still in need of further exploration, and understanding its internalization pathways can further assist us in understanding the mechanisms through which it exerts intracellular activity.
2.2 Antioxidant and anti-apoptotic effects of C-peptide
Current research evidence on endothelial cells suggests that C-peptide can directly reduce the generation of ROS (21). C-peptide exhibits the ability to influence the nicotinamide adenine dinucleotide phosphate (NADPH) oxidase that is activated on the plasma membrane by high glucose levels. It achieves this by curtailing excessive ROS production through the restoration of mitochondrial electron transport chain activity within endothelial cells (22, 23). Research by Bhatt and colleagues indicates that C-peptide can activate AMP-activated protein kinase-α (AMPK-α), an enzyme that can reduce the production of NADPH and ROS in mitochondria and endothelial cell apoptosis (23). As AMPK-α plays a core role in energy metabolism and diabetes, this target could have broad therapeutic implications.
Animal experiments have demonstrated that C-peptide can reduce cellular apoptosis in the aorta, heart, and kidneys of diabetic mice by downregulating the activation of transglutaminase 2 (TGase2), an apoptosis-promoting enzyme mediated by ROS, in endothelial cells exposed to a high-glucose environment (24). Studies conducted in vitro have demonstrated that the supplementation of C-peptide exogenously has the capacity to diminish apoptosis in pancreatic islet cells induced by high glucose concentrations (25)..
C-peptide can downregulate the activation of transcription factor p53 and mitochondrial adaptor protein P66 caused by high glucose memory, thus reducing the apoptosis of vascular endothelial cells. Exogenous supplementation with C-peptide can prevent vascular endothelial damage caused by high glucose memory (26).
Conditions characterized by prolonged high glucose and elevated ROS levels may lead to a reduction in insulin gene expression. This is potentially mediated through the downregulation of transcription factors such as pancreatic duodenal homeobox 1 (PDX-1) and musculoaponeurotic fibrosarcoma oncogene family A (MafA) (27). Furthermore, such conditions may also accelerate the rate of cell apoptosis.
2.3 C-peptide and inflammatory response
C-peptide at physiological concentrations has anti-inflammatory effects, but some reports also suggest that C-peptide has pro-inflammatory effects, which may be related to its over-secretion. C-peptide has been observed to significantly attenuate the secretion of inflammatory factors, including IL-6, IL-8, MIP-1α, and MIP-1β, in U-937 monocytes under conditions of high glucose stimulation. Additionally, it decreases their adhesion to human aortic endothelial cells. This could potentially be attributed to the inhibition of excessive ROS production, thereby reducing the activation of nuclear factor-κB (NF-κB) (28). C-peptide also has the potential to lower the expression of adhesion molecules, such as P-selectin and intercellular adhesion molecule 1, in rat mesenteric arteries. As a result, the interaction between leukocytes and endothelial cells is diminished (29).
Clinical research has uncovered that in obese patients suffering from type 2 diabetes, there exists a positive correlation between the concentration of C-peptide and pro-inflammatory chemokine ligand-2 (C-C chemokine ligand -2, CCL2), as well as E-selectin. Conversely, a negative correlation has been observed between C-peptide concentration and the anti-inflammatory factor IL-10 (30).. In the initial stages of T2DM, there is an observed increase in the secretion of C-peptide. In vitro investigations suggest that excess C-peptide can accumulate within vessel walls. C-peptide instigates the chemotaxis of CD4(+) lymphocytes and monocytes in a concentration-dependent manner, thus encouraging their migration into the vessel wall. This chemotactic process is associated with pertussis toxin-sensitive G-proteins and a mechanism that is PI-3k-dependent (13, 14). C-peptide above physiological concentrations can stimulate the production of nitrite in mouse macrophages through the activation of the calcium/JAK2/STAT1 pathway (31).
When C-peptide is deficient, exogenous supplementation of C-peptide can play an anti-inflammatory role and prevent the apoptosis of endothelial cells. However, when C-peptide is higher than the physiological dose, excess C-peptide can promote inflammation by stimulating the chemotaxis of CD4(+) lymphocytes and monocytes, as well as promoting the pro-inflammatory role of macrophages.
3 C-peptide and diabetic kidney disease
DKD is acknowledged as a severe microvascular complication prompted by chronic hyperglycemia, resulting in structural and functional alterations in the kidney. Mitochondrial dysfunction leading to an elevated production of ROS and superoxide in states of high glucose is a principal initiating factor in the onset of diabetic complications (32). Excess ROS inhibition suppresses inhibition of mammalian rapamycin complex 1 (mTORC1), AMPK, and activation of the NF-κB, protein kinase C (PKC) pathway (33). mTORC1 and AMPK are central to the regulation of autophagy, which plays an important protective role in the kidney by preventing fibrosis and inflammation (34). PKC pathway activation affects vascular function by regulating endothelial permeability, vasoconstriction, extracellular matrix (ECM) maintenance, cell growth, angiogenesis, cytokine activation and leukocyte adhesion to influence vascular function (33). Activation of NF-κB further promotes the expression of inflammatory factors and adhesion molecules, triggering inflammation and fibrosis (35). On the one hand, elevated PKC induces endothelial-type nitric oxide synthase (eNOS) and increases nitric oxide (NO) utilization in early DKD (36). Increased NO contributes to vascular endothelial growth factor (VEGF) activation, which leads to endothelial dysfunction (37, 38). In addition, PKC can lead to activation of transforming growth factor-β (TGF-β) and plasminogen activator inhibitor 1 (PAI-1), resulting in fibronectin deposition, fine increased extracellular matrix deposition, causing glomerulosclerosis and renal fibrosis (36). A cross-sectional analysis conducted in Korea, which involved 1410 type 2 diabetes patients stratified based on quartiles of fasting C-peptide, revealed an increased prevalence of DKD in the group that fell within the highest quartile as compared to the lowest (OR= 2.65, 95% CI, (1.71, 4.12)) (39). Certain studies propose that C-peptide replacement therapy could represent a novel treatment approach for DKD. Several small clinical studies have suggested that short-term C-peptide supplementation in patients with T1DM can reduce eGFR and urinary protein excretion rates, without impacting glycemic and blood pressure control (40, 41). In 2000, a study of 21 normotensive patients with microalbuminuria found a significant reduction in urinary protein excretion rate following 3 months of combined insulin and C-peptide treatment, with no significant change in eGFR (42). In a subsequent cohort study of islet-kidney transplantation in T1DM, improved islet β-cell function post-transplantation was also found to enhance patient renal outcomes (43).A Meta-analysis (44) incorporating 4 human trials, and 18 animal trials, showed that DKD patients treated with C-peptide did not have a significant difference in GFR after treatment, but two studies reported a reduction in glomerular hyperfiltration. While, in diabetic rodent models, C-peptide led to a reduction in GFR reflecting a partial reduction in glomerular hyperfiltration.
Renal tubular cell membranes host G-protein coupled receptors (GPCR) with a high affinity for C-peptide (45). These peptides instigate a cascade of reactions upon binding to GPCR. In renal cells, the elements of signaling pathways influenced by C-peptide comprise Na+/K+/ATPase activity, endothelial calcium inward flow, PKC, ERK, c-Jun amino-terminal kinase (JNK), TGF-β/Smad, Inducible nitric oxide synthase (iNOS), Peroxisome proliferator-activated receptor (PPAR-γ), and PI-3k activity (46, 47).
It is suggested that C-peptide has the capacity to alleviate albuminuria and glomerular hyperfiltration by mitigating histological damage, which includes glomerular hypertrophy, glomerular thylakoid expansion, tubular interstitial inflammation, and tubular epithelial interstitial transformation. Furthermore, C-peptide is also believed to reduce tubular sodium reabsorption and glomerular hyperfiltration. Additionally, C-peptide can inhibit cellular apoptotic processes and tissue damage in renal tissues under high glucose conditions, thereby offering renal protection (46, 47).
The protective mechanisms of C-peptide on the kidney are mainly summarized as follows:
① Modulation of endothelial cell function to ameliorate hemodynamic disturbances
C-peptide replacement therapy was found to effectively rectify diabetic nephropathy in diabetic rat models, with nitric oxide (NO) likely serving as a key medium for the renal protective effects of C-peptide (10). C-peptide was observed to directly enter the nucleus of mesangial cells and inhibit the binding of NF-κB to p300 and the iNOS promoter, reducing the acetylation of histone H3K9ac, thereby repressing the expression of iNOS induced by NF-κB/p300 (48).
C-peptide demonstrated vasodilation capabilities for small arteries, and inhibited the Na+/K+/ATPase activity on renal tubules, lessening the reabsorption of sodium, thereby reducing the hyperfiltration induced by diabetes in the glomerulus (49).
② Inflammation and Fibrosis
In studies of type 1 diabetes mellitus (T1DM) mouse models, exogenous supplementation of C-peptide was found to aid in the reduction of pro-inflammatory cytokines such as IL17 and tumor necrosis factor-alpha (TNFα), and anti-inflammatory cytokines, like IL4 and IL10, in the urine (P <0.05). Additionally, an increase in the expression of the IL10 gene and a decrease in the expression of the TNFα gene were observed in the kidneys (50).
C-peptide also can alleviate renal fibrosis, thereby exerting renal protective effects. The activation of the TGFB1/SMAD3 signaling pathway can lead to the over-synthesis of type IV collagen protein (COL4), and deficiency in matrix metalloproteinases (MMP)-9 and MMP-2, which promote ECM deposition in renal cells. Islet transplantation showed a beneficial effect on the glomerular filtration membrane structure of early-stage diabetic nephropathy rats, reducing the thickness of the glomerular basement membrane, decreasing TGF-β1 and connective tissue growth factor (CTGF), increasing the expression of anti-fibrotic factors. This anti-fibrotic mechanism might be dependent on the restoration of C-peptide levels (51). Moreover, C-peptide was able to inhibit the expression of Col4 a1- a5 mRNA in the kidney, reducing COL4 and TGF-β1 protein levels, preventing the binding of SMAD3 to its sites in the promoters of Col4a1a2, Col4a3a4, and Col4a5, thereby repressing the production of COL4 (52). Finally, cell experiments showed that early C-peptide exerts a dual effect on MMP-9. As the observation time extended, C-peptide could induce an increase in MMP-9 expression, thereby reducing ECM accumulation and reversing DKD (53).
Although C-peptide replacement therapy significantly reduced the rate of urinary protein excretion in DKD in some early preclinical and clinical studies, the impact of C-peptide on eGFR remains contentious. Due to the small size, short duration, and lack of large-scale clinical trials, the evidence for C-peptide replacement therapy as a treatment for DKD remains limited within the scope of evidence-based medicine.
4 C-peptide and diabetic retinopathy
Chronic hyperglycemia incites a range of pathophysiological alterations within retinal cells. These alterations encompass an upsurge in the expression of matrix proteins such as collagen and fibronectin, thickening of the basement membrane, heightened retinal vascular permeability, and modifications in retinal blood flow (54). Beyond the potential for vision loss, diabetic retinopathy(DR) intensifies the risk of systemic vascular complications, thereby elevating the mortality rate among patients diagnosed with T2DM (55). Despite the role that the duration of diabetes and glycemic levels play in influencing DR (56), rigorous glycemic control does not entirely inhibit the evolution and progression of retinopathy.
Existing research on T1DM has revealed that residual fasting C-peptide exhibits protective qualities against the onset of DR (57). In a 2015 cross-sectional investigation involving 2062 patients with type 2 diabetes, it was demonstrated that C-peptide levels were inversely related to the prevalence of DR, irrespective of eGFR (39). Another cross-sectional study conducted in Shanghai, encompassing 4793 community-based patients with type 2 diabetes, reported that elevated fasting C-peptide levels acted as a protective factor against the prevalence of DR. It was observed that the prevalence of DR decreased as C-peptide levels increased (OR=0.73, 95% CI (0.62, 0.86), P<0.001) (58). Within the Veterans Affairs Diabetes Trial (VADT) (59), every increment of 1 pmol/mL in baseline C-peptide was correlated with a substantial 67.2% decrease in the prevalence of DR, coupled with a 47% reduction in the risk associated with DR progression. A prospective cohort study further demonstrated that patients who underwent islet transplantation experienced improved glycemic control and their progression of DR was considerably slower compared to those receiving intensive drug therapy (60). Moon et al. (61) managed to maintain C-peptide within the physiological concentration range for 16 weeks in diabetic mice by injecting ultra-long-lasting human C-peptide delivery into the vitreous body of the mice. The results indicated that maintaining the physiological concentration of C-peptide improved the formation of retinal neovascularization induced by high blood sugar in diabetic mice.
The protective role of C-peptide is attributed to the following mechanisms. Firstly, C-peptide enhances microvascular blood flow and improves microvascular endothelial function. It increases microvascular blood flow and mitigates vascular permeability by activating eNOS (62). It also counters ROS production by activating AMPK-α, which in turn inhibits VEGF’s increase in NADPH oxidase-dependent ROS production (63). C-peptide, along with ROS scavengers, diminishes VEGF-induced stress fiber formation that escalates vascular permeability (64). Moreover, C-peptide decreases the VEGF-induced breakdown of VE-cadherin, an endothelial cell-specific adhesion molecule responsible for connecting adjacent endothelial cells, thus maintaining endothelial cell integrity and reducing vascular permeability (65). Therefore, C-peptide may shield against retinopathy by inhibiting intracellular ROS production, reducing stress fiber formation, maintaining endothelial cell integrity, and decreasing VEGF-induced increases in microvascular permeability (11). High glucose conditions can trigger vascular leakage by activating TGase2 in the retina, but C-peptide can restrain this activation in the mouse retina, thereby decreasing vascular leakage (66).
Secondly, C-peptide also participate in regulating the composition of extracellular matrix proteins. Animal experiments have indicated that exogenous C-peptide supplementation can mitigate retinopathy by inhibiting the hyperglycemia-induced increase in fibronectin and reducing ECM deposition (67). Additionally, C-peptide exhibits insulin-mimetic properties, enhancing insulin action by increasing phosphorylation of insulin receptor substrate 1 and the activity of MAPK and PI-3k when insulin concentrations are low (68). Nevertheless, the current body of research on C-peptide replacement therapy in retinopathy is limited, and further investigation is warranted to explore its therapeutic effects.
5 C-peptide and diabetic macrovascular complications
The relationship between fasting C-peptide levels and macrovascular complications remains ambiguous, necessitating further investigation. In 2012, a cross-sectional study from Korea reported no association between C-peptide and macrovascular complications in T2DM patients (39). However, a contrasting result was obtained from a 2018 community-based T2DM study from Shanghai (58), which demonstrated that the prevalence of cardiovascular disease (CVD) escalated with increasing C-peptide levels. The risk of developing CVD correspondingly increased with rising C-peptide levels in a logistic regression analysis (OR = 1.27, 95% CI (1.13, 1.42), P<0.001).
In a cohort study (69)involving 2306 patients subjected to baseline coronary angiography and categorized according to the tertiles of C-peptide levels, the highest C-peptide group displayed an increased risk of all-cause mortality (HR = 1.46, 95% CI (1.15, 1.85), P = 0.002) and cardiac-cause mortality (HR = 1.58, 95% CI (1.15, 2.18), P = 0.002) when compared to the group with the lowest C-peptide levels. Additionally, the group with the highest C-peptide levels demonstrated elevated degrees of endothelial dysfunction, increased atherosclerotic markers, and more severe coronary artery lesions (69). A meta-analysis, including sixteen observational studies, eight cohort studies, and seven cross-sectional studies, showed the association between C-peptide and increased cardiovascular events was only observed in cross-sectional studies but not in cohort studies (70).
A study including 8 male T1DM,before and during dipyridamole administration using a randomized double-blind crossover protocol with infusion of C-peptide on two different days, suggested that short-term replacement of C-peptide improves myocardial function and myocardial perfusion (71). Endothelial cell damage and subsequent apoptosis in a state of high glucose are precipitated by oxidative stress, mitochondrial dysfunction, and abnormalities in calcium regulation (30). In addition, the adhesion and migration of monocytes beneath the endothelium are also among the key events in the early stages of atherosclerosis. In such an environment, C-peptide displays a protective effect on endothelial cells, mainly through the following mechanisms:
(1) Physiological concentrations of C-peptide can alleviate high glucose-mediated endothelial dysfunction, by reducing the expression of the cell surface protein VCAM-1 and decreasing the secretion of chemokines IL-8 and monocyte chemoattractant protein-1 (MCP-1) (72). However, this effect is not evident in environments with normal glucose levels; when C-peptide is in culture media with normal glucose levels, it does not significantly reduce the expression of VCAM-1 and chemokines (72). Furthermore, C-peptide combats high glucose-induced endothelial dysfunction by reducing NF-κB activation, potentially due to C-peptide induced phosphorylation of protein substrates in the cytoplasm or its interaction with NF-κB p65/p50 subunit, preventing DNA binding, impacting the expression of VCAM-1 and the secretion of MCP-1 and IL-8 (72, 73).
(2) C-peptide also exerts a protective effect by inhibiting the generation of ROS, reducing endothelial cell death, and preserving mitochondrial structure and function (46).
(3) In bovine and rat aortic endothelial cells, C-peptide demonstrates a concentration and time-dependent increase in endothelial NO release, which is induced by C-peptide through calcium internal mediation, thereby activating eNOS or ERK-1/-2 induced by C-peptide (74). In addition, studies have shown that C-peptide has inhibitory effects on endothelial cell proliferation in great saphenous vein bypass grafts. Insulin promotes neointima thickening, smooth muscle cell proliferation, and migration, while C-peptide can inhibit these effects (75).
These mechanisms collectively explain the protective effects of C-peptide on endothelial cells in a hyperglycemic environment.
In obese patients with type 2 diabetes, higher concentrations of C-peptide are associated with inflammation and exacerbation of the atherosclerotic process (30). In non-diabetic patients, it has been found that C-peptide levels are positively correlated with the incidence of several cardiovascular diseases, such as coronary artery disease and myocardial infarction (76). Conversely, in the early stages of T2DM, C-peptide secretion increases and can be deposited into the vascular endothelium, potentially promoting atherogenesis by initiating or facilitating the migration of monocytes to the endothelium (13). C-peptide can also induce lipid deposition and promote vascular smooth muscle cell (VSMC) recruitment and proliferation, all of which contribute to the development of atherosclerosis (13, 77). C-peptide can promote the transcriptional activity of PPAR-γ, as observed in renal tubular and lung injury, although most studies indicate that PPAR-γ has anti-inflammatory and anti-atherosclerotic effects, some research suggests that increased expression of PPAR-γ in macrophages can promote the occurrence of atherosclerosis, possibly related to the upregulation of CD36 expression in macrophages, which is deeply involved in the differentiation of monocytes into macrophages and the accumulation of oxidized LDL particles (78–81). In addition, C-peptide also can induce VSMC proliferation through the activation of SRC-kinase, PI-3K, and extracellular signal-regulated kinase 1/2 (82).
C-peptide plays different roles in T1DM, T2DM, or non-diabetic individuals, indicating that the regulation of atherosclerosis by C-peptide may be jointly influenced by blood glucose, insulin, and C-peptide concentrations. However, current research is not in-depth enough to further elucidate its mechanism. Therefore, further exploration of the regulatory effects of C-peptide, insulin, and the high-glucose environment on atherosclerosis is of significant importance.
6 C-peptide and diabetic peripheral neuropathy
A cross-sectional study enrolled 14,908 patients with T2DM showed that fasting C-peptide is negatively associated with diabetic peripheral neuropathy (DPN) (83). A predictive model that included 1278 T2DM patients showed that 2-hour postprandial C-peptide/fasting C-peptide was a protective factor for DPN (84). In a type 1 diabetic rat model, C-peptide replacement therapy has been observed to prevent the development of deficits in nerve conduction velocity (NCV) and alleviate both acute and chronic DPN as T1DM naturally progresses (12). Furthermore, in BB/Wor rats characterized by reduced intraneural perfusion and increased oxidative stress, C-peptide also prevents neurovascular defects and decreases thermal nociceptive hypersensitivity (85). The mechanism by which C-peptide ameliorates neuropathy might involve the stimulation of the NO system by C-peptide, acting directly on nerve fibers or mediating vasodilation via NO, rather than by improving oxidative stress (73, 85).
The Na+/K+/ATPase, a prevalent membrane enzyme, exhibits reduced activity in peripheral nerves, leading to Na+ channel inactivation and myelin swelling (12). In BB/Wor rats, two consecutive months of C-peptide replacement treatment diminished myelin swelling and improved neural Na+/K+/ATPase defects (12). Thus, current findings indicate that in a T1DM mouse model, physiological concentrations of C-peptide can provide neuroprotective functions by enhancing neuroperfusion through NO-sensitive neurovascular mechanisms and ameliorating Na+/K+/ATPase deficits.
In a 2003 clinical trial conducted by Ekberg et al. (86), which included 46 patients, C-peptide replacement therapy for three months significantly improved sensory nerve conduction velocity (SCV) in the C-peptide treatment group, along with enhanced vibrotactile sensation, although no temperature and motor nerve conduction velocity (MCV) improvements were recorded. In 2007, the study scope was broadened to incorporate a six-month period of C-peptide replacement therapy and to include a population of T1DM patients exhibiting DPN symptoms. Findings indicated that C-peptide replacement therapy significantly improved SCV among patients displaying DPN symptoms, particularly noticeable in those with less compromised neurological function at baseline (87). In 2016, a clinical study that included 250 patients with DPN who received long-acting C-peptide (2.4 mg, 0.8 mg) weekly to DPN for 52 weeks, found significant improvements in patients’ vibration perception threshold (VPT). However, sural nerve conduction velocity (SNCV), other electrophysiological variables, or modified Toronto Clinical Neuropathy Score (mTCNS) were not significantly different from placebo control patients (88). It is worth noting that, the C-peptide of patients increased to 1.8-2.2 nmol/L (low dose) and 5.6-6.8 nmol/L (high dose), which is higher than the physiological concentration of C-peptide. As previously articulated, the infusion of ultra-long-lasting human C-peptide into the vitreous body of mice, maintaining C-peptide within the physiological concentration range, yielded favorable therapeutic outcomes. In this clinical investigation, the failure to achieve significant improvement in SNCV, other electrophysiological variables, or mTCNS in DPN patients may be attributed to the elevated C-peptide concentrations, which exceeded physiological levels. This observation leads us to conjecture that the optimal therapeutic effect may lie within a specific concentration range. Consequently, we propose that future basic and clinical research into C-peptide replacement therapies should focus on the meticulous monitoring of post-treatment C-peptide levels. Additionally, these studies should explore the optimal range for maintaining both fast C-peptide and post-radical C-peptide concentrations, as this may be pivotal in enhancing the efficacy of the treatment.
In T2DM rats, sensory NCV slowing or nociceptive hyperalgesia was only observed with reduced neurovascular perfusion and was not accompanied by increased oxidative stress, and C-peptide replacement therapy did not improve neuropathy (85). A study in a community-based population (89) discovered a negative association between C-peptide levels and the development of diabetic peripheral neuropathy in a T2DM population. Given the disparate mechanisms underpinning DPN in T1DM and T2DM, it is noteworthy that the present study specifically demonstrated no significant amelioration of DPN in T2DM patients via C-peptide replacement. This underscores the need for further investigation into the association between overproduction of C-peptide and DPN in T2DM patients, necessitating additional animal experiments.
7 C-peptide and diabetic emergencies
Diabetic emergencies include diabetic ketoacidosis (DKA), hyperglycaemic hyperosmolar state (HHS) and hypoglycemia (90). Serum C-peptide is negatively correlated with the risk of DKA (91) and hypoglycemia (92), but there is currently no research exploring the relationship between C-peptide and HHS. A retrospective study (91) involving 234 children and adolescents with T1DM, based on serum C-peptide levels at diagnosis and 15 years post-diagnosis, compared laboratory results and diabetes complication incidence between the two groups after 15 years. Results showed that patients with higher C-peptide levels (after 15 years) used lower doses of insulin, and in the group with lower C-peptide levels, the incidence rate of DKA was higher, consistent with the conclusions of the previous studies (93, 94). A study (95) based on clinical and biochemical characteristics to predict the risk of diabetic ketosis (DK) in patients with T2DM shows that 2-hour postprandial C-peptide levels are negatively correlated with the risk of diabetic ketosis. However, the number of relevant studies is relatively small, and more clinical studies are needed to support the relevant conclusions. Additionally, whether C-peptide replacement therapy has a preventive effect on ketoacidosis poisoning remains to be further researched.
A study involving 1565 patients with T2DM from the Veterans Affairs Diabetes Trial showed that measuring fasting C-peptide levels at baseline was negatively correlated with the risk of severe hypoglycemia (96). Zenz et al. (97) found that under hypoglycemic conditions, retained β-cell function (C-peptide positive) may help to regulate blood glucose levels more effectively. C-peptide positive patients have higher glucagon concentrations and endogenous glucose production (EGP) during hypoglycemia, suggesting better response mechanisms under hypoglycemic conditions Hope et al. (98) conducted continuous glucose monitoring on 17 insulin-treated T2DM patients and matched controls, and surveyed 256 insulin-treated T2DM patients and 209 T1DM patients. The results showed that patients with lower random C-peptide levels (rCP <200 pmol/l) were more prone to hypoglycemia, with more frequent and prolonged episodes. Patients with preserved C-peptide had fewer hypoglycemic events at night. This finding suggests that low rCP levels can serve as a practical, stable, and economical biomarker for hypoglycemia risk assessment, aiding the management and treatment of T2DM. C-peptide may protect against hypoglycemia by increasing α-cell response to low blood sugar and promoting glucagon secretion (99). In adult T1DM patients, β-cell responsiveness to hyperglycemia and α-cell responsiveness to hypoglycemia were only observed when residual C-peptide levels were higher. Residual C-peptide may assist in blood glucose control, and a clinical trial in Japan also showed that C-peptide is independently associated with glucagon levels (100). Moore et al. (101) explored the role of C-peptide in insulin-induced hypoglycemia by testing the effects of C-peptide infusion on glucagon secretion under isoglycemic and hypoglycemic conditions in dogs (5 males/4 females). In the experiments, glucagon secretion remained unchanged in the isoglycemic-hyperinsulinemic response in the C-peptide infusion group, whereas it increased twofold during hypoglycemia. These data suggest that the presence of C-peptide maintains glucagon secretion during isoglycemia and enhances it during hypoglycemia, which explains why T1DM patients with residual insulin secretory capacity are less susceptible to hypoglycemia. However, the regulatory mechanism of C-peptide on glucagon is yet to be reported. Lower C-peptide levels have been observed to be associated with greater glucose fluctuation and higher hypoglycemia risk, leading to attention to its p otential vital role in blood sugar stability regulation. Although C-peptide, as a byproduct of insulin synthesis, has not been fully revealed in terms of its biological functions in diabetes treatment and metabolic regulation, more and more experimental data support that the maintenance and stability of C-peptide levels are important in blood sugar control and diabetes-related complications prevention. Therefore, C-peptide could be considered an important clinical target for glucose control in diabetes treatment.
8 Conclusion
C-peptide, a bioactive peptide with a plethora of functionalities, has a profound biological significance. It exhibits antioxidant, anti-apoptotic, and anti-inflammatory effects primarily through binding with cellular surface signaling molecules to activate downstream pathways or regulating intracellular transcriptional processes.
The complex pattern of the relationship between C-peptide and diabetic chronic complications (Figure 1) has not yet been fully understood. The underlying mechanisms might be associated with: 1) dose-dependent effects of C-peptide; 2) varied affinities with different receptors under physiological and pathological conditions; 3) divergent responses in different cells and tissues; and 4) potential interactions with other molecules altering C-peptide’s effects. Future basic and clinical studies of C-peptide replacement therapies will need to focus on baseline levels of C-peptide in addition to more attention also needs to be paid to post-treatment C-peptide levels to explore the optimal range of fasting C-peptide and postprandial C-peptide maintenance.
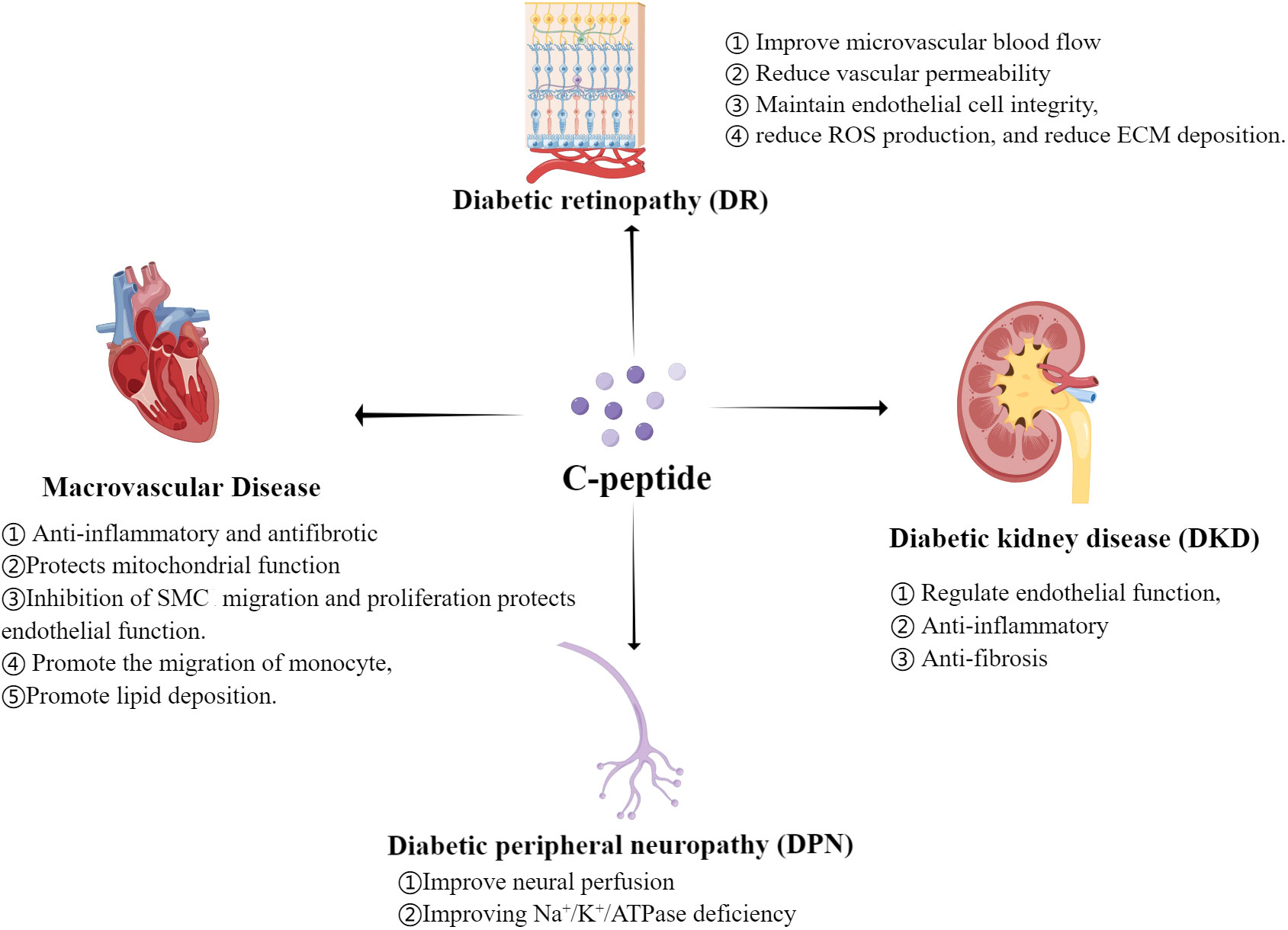
Figure 1 Regulation mechanism of C-peptide on Diabetic Chronic Complications.(By Figdraw.) ROS, reactive oxygen species; ECM, extracellular matrix; SMC, smooth muscle cell.
In conclusion, a deeper understanding of the role of C-peptide in the pathogenesis of diabetic complications could be key to their prevention and treatment. This would not only help to clarify the differences in the mechanisms underlying the onset of complications in type 1 and type 2 diabetes but also provide practical implications for clinical treatments.
Author contributions
JChen: Writing – original draft, Writing – review & editing. YH: Writing – original draft, Writing – review & editing. CL: Writing – original draft. JChi: Writing – review & editing. YW: Writing – review & editing. LX: Writing – original draft, Writing – review & editing.
Funding
This work was supported by Natural Science Foundation of Shandong Province (ZR2023MH316).
Conflict of interest
The authors declare that the research was conducted in the absence of any commercial or financial relationships that could be construed as a potential conflict of interest.
Publisher’s note
All claims expressed in this article are solely those of the authors and do not necessarily represent those of their affiliated organizations, or those of the publisher, the editors and the reviewers. Any product that may be evaluated in this article, or claim that may be made by its manufacturer, is not guaranteed or endorsed by the publisher.
References
1. Ogurtsova K, Guariguata L, Barengo NC, Ruiz PL, Sacre JW, Karuranga S, et al. IDF diabetes Atlas: Global estimates of undiagnosed diabetes in adults for 2021. Diabetes Res Clin Pract (2022) 183:109118. doi: 10.1016/j.diabres.2021.109118
2. Zheng Y, Ley SH, Hu FB. Global aetiology and epidemiology of type 2 diabetes mellitus and its complications. Nat Rev Endocrinol (2018) 14(2):88–98. doi: 10.1038/nrendo.2017.151
3. Cai Y, Yang X, Chen S, Tian K, Xu S, Deng R, et al. Regular consumption of pickled vegetables and fermented bean curd reduces the risk of diabetes: a prospective cohort study. Front Public Health (2023) 11:1155989. doi: 10.3389/fpubh.2023.1155989
4. Bakker W, Eringa E, Sipkema P, van Hinsbergh VW. Endothelial dysfunction and diabetes: roles of hyperglycemia, impaired insulin signaling and obesity. Cell Tissue Res (2009) 335(1):165–89. doi: 10.1007/s00441-008-0685-6
5. Yang J, Yu D, Wen W, Saito E, Rahman S, Shu XO, et al. Association of diabetes with all-cause and cause-specific mortality in asia: A pooled analysis of more than 1 million participants. JAMA Netw Open (2019) 2(4):e192696. doi: 10.1001/jamanetworkopen.2019.2696
6. Perkovic V, Jardine M, Neal B, Bompoint S, Heerspink HJL, Charytan DM, et al. Canagliflozin and renal outcomes in type 2 diabetes and nephropathy. N Engl J Med (2019) 380(24):2295–306. doi: 10.1056/NEJMoa1811744
7. Cui J, Liu Y, Li Y, Xu F, Liu Y. Type 2 diabetes and myocardial infarction: recent clinical evidence and perspective. Front Cardiovasc Med (2021) 8:644189. doi: 10.3389/fcvm.2021.644189
8. Luppi P, Geng X, Cifarelli V, Drain P, Trucco M. C-peptide is internalised in human endothelial and vascular smooth muscle cells via early endosomes. Diabetologia (2009) 52(10):2218–28. doi: 10.1007/s00125-009-1476-7
9. Marques R, Fontaine M, Rogers JJP. C-peptide: much more than a byproduct of insulin biosynthesis. Pancreas (2004) 29(3):231–8. doi: 10.1097/00006676-200410000-00009
10. Elbassuoni E, Aziz N, El-Tahawy N. Protective effect of C-peptide on experimentally induced diabetic nephropathy and the possible link between C-peptide and nitric oxide. Appl Physiol Nutr Metab (2018) 43(6):617–24. doi: 10.1139/apnm-2017-0617
11. Lim Y, Bhatt M, Kwon M, Park D, Lee S, Choe J, et al. Prevention of VEGF-mediated microvascular permeability by C-peptide in diabetic mice. Cardiovasc Res (2014) 101(1):155–64. doi: 10.1093/cvr/cvt238
12. Sima A, Zhang W, Sugimoto K, Henry D, Li Z, Wahren J, et al. C-peptide prevents and improves chronic Type I diabetic polyneuropathy in the BB/Wor rat. Diabetologia (2001) 44(7):889–97. doi: 10.1007/s001250100570
13. Marx N, Walcher D, Raichle C, Aleksic M, Bach H, Grüb M, et al. C-peptide colocalizes with macrophages in early arteriosclerotic lesions of diabetic subjects and induces monocyte chemotaxis in vitro. Arterioscler Thromb Vasc Biol (2004) 24(3):540–5. doi: 10.1161/01.ATV.0000116027.81513.68
14. Marx N, Walcher D, Raichle C, Aleksic M, Bach H, Grub M, et al. C-peptide induces chemotaxis of human CD4-positive cells: involvement of pertussis toxin-sensitive G-proteins and phosphoinositide 3-kinase. Arterioscler Thromb Vasc Biol (2004) 53(7):1664–70. doi: 10.1161/01.ATV.0000116027.81513.68
15. Yosten G, Kolar G, Redlinger L, Samson WK. Evidence for an interaction between proinsulin C-peptide and GPR146. J Endocrinol (2013) 218(2):B1–8. doi: 10.1530/JOE-13-0203
16. Wahren J, Larsson C. C-peptide: new findings and therapeutic possibilities. JDr Pract c (2015) 107(3):309–19. doi: 10.1016/j.diabres.2015.01.016
17. Poteryaeva O, Usynin I. Molecular mechanisms of action and physiological effects of the proinsulin C-peptide (a systematic review). JBk (2020) 66(3):196–207. doi: 10.18097/pbmc20206603196
18. Souto S, Campos J, Fangueiro J, Silva AM, Cicero N, Lucarini M, et al. Multiple cell signalling pathways of human proinsulin C-peptide in vasculopathy protection. Int J Mol Sci (2020) 21(2):645. doi: 10.3390/ijms21020645
19. Lindahl E, Nyman U, Melles E, Sigmundsson K, Stahlberg M, Wahren J, et al. Cellular internalization of proinsulin C-peptide. Cell Mol Life Sci (2007) 64(4):479–86. doi: 10.1007/s00018-007-6467-6
20. Lindahl E, Nyman U, Zaman F, Palmberg C, Cascante A, Shafqat J, et al. Proinsulin C-peptide regulates ribosomal RNA expression. J Biol Chem (2010) 285(5):3462–9. doi: 10.1074/jbc.M109.053587
21. Luppi P, Drain P. C-peptide antioxidant adaptive pathways in β cells and diabetes. JJoim (2017) 281(1):7–24. doi: 10.1111/joim.12522
22. Vejandla H, Hollander J, Kothur A, Brock RW. C-Peptide reduces mitochondrial superoxide generation by restoring complex I activity in high glucose-exposed renal microvascular endothelial cells. ISRN Endocrinol (2012) 2012:162802. doi: 10.5402/2012/162802
23. Bhatt MP, Lim YC, Kim YM, Ha KS. C-peptide activates AMPKα and prevents ROS-mediated mitochondrial fission and endothelial apoptosis in diabetes. Diabetes (2013) 62(11):3851–62. doi: 10.2337/db13-0039
24. Bhatt M, Lim Y, Hwang J, Na S, Kim YM, Ha KS. C-peptide prevents hyperglycemia-induced endothelial apoptosis through inhibition of reactive oxygen species-mediated transglutaminase 2 activation. Diabetes (2013) 62(1):243–53. doi: 10.2337/db12-0293
25. Bugliani M, Torri S, Lupi R, Del Guerra S, Grupillo M, Del Chiaro M, et al. Effects of C-peptide on isolated human pancreatic islet cells. Diabetes Metab Res Rev (2007) 23(3):215–9. doi: 10.1002/dmrr.674
26. Bhatt MP, Lee YJ, Jung SH, Kim YH, Hwang JY, Han ET, et al. C-peptide protects against hyperglycemic memory and vascular endothelial cell apoptosis. J Endocrinol (2016) 231(1):97–108. doi: 10.1530/JOE-16-0349
27. Robertson R. Chronic oxidative stress as a central mechanism for glucose toxicity in pancreatic islet beta cells in diabetes. JTJobc (2004) 279(41):42351–4. doi: 10.1074/jbc.R400019200
28. Haidet J, Cifarelli V, Trucco M, Luppi P. C-peptide reduces pro-inflammatory cytokine secretion in LPS-stimulated U937 monocytes in condition of hyperglycemia. Inflamm Res (2012) 61(1):27–35. doi: 10.1007/s00011-011-0384-8
29. Scalia R, Coyle KM, Levine BJ, Booth G, Lefer AM. C-peptide inhibits leukocyte-endothelium interaction in the microcirculation during acute endothelial dysfunction. FASEB J (2000) 14(14):2357–64. doi: 10.1096/fj.00-0183com
30. Janowska J, Chudek J, Olszanecka-Glinianowicz M, Semik-Grabarczyk E, Zahorska-Markiewicz B. Interdependencies among selected pro-inflammatory markers of endothelial dysfunction, C-peptide, anti-inflammatory interleukin-10 and glucose metabolism disturbance in obese women. Int J Med Sci (2016) 13(7):490–9. doi: 10.7150/ijms.14110
31. Lee SK, Lee JO, Kim JH, Jung JH, You GY, Park SH, et al. C-peptide stimulates nitrites generation via the calcium-JAK2/STAT1 pathway in murine macrophage Raw264.7 cells. Life Sci (2010) 86:863–8. doi: 10.1016/j.lfs.2010.03.022
32. Reidy K, Kang HM, Hostetter T, Susztak K. Molecular mechanisms of diabetic kidney disease. J Clin Invest (2014) 124(6):2333–40. doi: 10.1172/JCI72271
33. Warren AM, Knudsen ST, Cooper ME. Diabetic nephropathy: an insight into molecular mechanisms and emerging therapies. Expert Opin Ther Targets (2019) 23(7):579–91. doi: 10.1080/14728222.2019.1624721
34. Gonzalez CD, Carro Negueruela MP, Nicora Santamarina C, Resnik R, Vaccaro MI. Autophagy dysregulation in diabetic kidney disease: from pathophysiology to pharmacological interventions. Cells (2021) 10(9):2497. doi: 10.3390/cells10092497
35. Tang SCW, Yiu WH. Innate immunity in diabetic kidney disease. Nat Rev Nephrol (2020) 16(4):206–22. doi: 10.1038/s41581-019-0234-4
36. Geraldes P, King GL. Activation of protein kinase C isoforms and its impact on diabetic complications. Circ Res (2010) 106(8):1319–31. doi: 10.1161/CIRCRESAHA.110.217117
37. Noh H, King GL. The role of protein kinase C activation in diabetic nephropathy. Kidney Int Suppl (2007) 106):S49–53. doi: 10.1038/sj.ki.5002386
38. Kanwar YS, Sun L, Xie P, Liu FY, Chen S. A glimpse of various pathogenetic mechanisms of diabetic nephropathy. Annu Rev Pathol (2011) 6:395–423. doi: 10.1146/annurev.pathol.4.110807.092150
39. Kim BY, Jung CH, Mok JO, Kang SK, Kim CH. Association between serum C-peptide levels and chronic microvascular complications in Korean type 2 diabetic patients. Acta Diabetol (2012) 49(1):9–15. doi: 10.1007/s00592-010-0249-6
40. Johansson B, Sjöberg S, Wahren JJD. The influence of human C-peptide on renal function and glucose utilization in type 1 (insulin-dependent) diabetic patients. (1992) 35(2):121–8. doi: 10.1007/BF00402543
41. Johansson B, Kernell A, Sjöberg S, Wahren J. Influence of combined C-peptide and insulin administration on renal function and metabolic control in diabetes type 1. J Clin Endocrinol Metab (1993) 77(4):976–81. doi: 10.1210/jcem.77.4.8408474
42. Johansson B, Borg K, Fernqvist-Forbes E, Kernell A, Odergren T, Wahren J. Beneficial effects of C-peptide on incipient nephropathy and neuropathy in patients with Type 1 diabetes mellitus. Diabet Med (2000) 17(3):181–9. doi: 10.1046/j.1464-5491.2000.00274.x
43. Fiorina P, Folli F, Zerbini G, Maffi P, Gremizzi C, Di Carlo V, et al. Islet transplantation is associated with improvement of renal function among uremic patients with type I diabetes mellitus and kidney transplants. J Am Soc Nephrol (2003) 14(8):2150–8. doi: 10.1097/01.ASN.0000077339.20759.A3
44. Shaw JA, Shetty P, Burns KD, Fergusson D, Knoll GA. C-peptide as a therapy for kidney disease: A systematic review and meta-analysis. PloS One (2015) 10(5):e0127439. doi: 10.1371/journal.pone.0127439
45. Rigler R, Pramanik A, Jonasson P, Kratz G, Jansson OT, Nygren P, et al. Specific binding of proinsulin C-peptide to human cell membranes. Proc Natl Acad Sci USA (1999) 96(23):13318–23. doi: 10.1073/pnas.96.23.13318
46. Brunskill N. C-peptide and diabetic kidney disease. JJoim (2017) 281(1):41–51. doi: 10.1111/joim.12548
47. Yaribeygi H, Maleki M, Sathyapalan T, Sahebkar AJLs. The effect of C-peptide on diabetic nephropathy: A review of molecular mechanisms. Life Sci (2019) 237:116950. doi: 10.1016/j.lfs.2019.116950
48. Li Y, Li X, He K, Li B, Liu K, Qi J, et al. C-peptide prevents NF-κB from recruiting p300 and binding to the inos promoter in diabetic nephropathy. FASEB J (2018) 32(4):2269–79. doi: 10.1096/fj.201700891R
49. Nordquist L, Brown R, Fasching A, Persson P, Palm F. Proinsulin C-peptide reduces diabetes-induced glomerular hyperfiltration via efferent arteriole dilation and inhibition of tubular sodium reabsorption. Am J Physiol Renal Physiol (2009) 297(5):F1265–72. doi: 10.1152/ajprenal.00228.2009
50. Alves MT, Chaves ACS, Almeida APM, Simoes ESAC, Araujo SA, Mota APL, et al. Anti-inflammatory effects of C-peptide on kidney of type 1 diabetes mellitus animal model. Mol Biol Rep (2020) 47(1):721–6. doi: 10.1007/s11033-019-05152-4
51. Ni X, Xu Z, Wang J, Zheng S, Cai YJT. C-peptide and islet transplantation improve glomerular filtration barrier in diabetic nephropathy rats. Transpl Immunol (2020) 62:101322. doi: 10.1016/j.trim.2020.101322
52. Li Y, Zhong Y, Gong W, Gao X, Qi H, Liu K, et al. C-peptide prevents SMAD3 binding to alpha promoters to inhibit collagen type IV synthesis. J Mol Endocrinol (2018) 61(1):47–56. doi: 10.1530/JME-18-0009
53. Wang J, Li Y, Xu M, Li D, Wang Y, Qi J, et al. C-peptide exhibits a late induction effect on matrix metallopeptidase-9 in high glucose-stimulated rat mesangial cells. Exp Ther Med (2016) 12(6):4142–6. doi: 10.3892/etm.2016.3873
54. Fong D, Aiello L, Ferris F 3rd, Klein R. Diabetic retinopathy. Diabetes Care (2004) 27(10):2540–53. doi: 10.2337/diacare.27.10.2540
55. Cheung N, Mitchell P, Wong TJL. Diabetic retinopathy. Lancet (2010) 376(9735):124–36. doi: 10.1016/S0140-6736(09)62124-3
56. Kollias AN, Ulbig MW. Diabetic retinopathy: Early diagnosis and effective treatment. Dtsch Arztebl Int (2010) 107(5):75–83; quiz 84. doi: 10.3238/arztebl.2010.0075
57. Panero F, Novelli G, Zucco C, Fornengo P, Perotto M, Segre O, et al. Fasting plasma C-peptide and micro- and macrovascular complications in a large clinic-based cohort of type 1 diabetic patients. Diabetes Care (2009) 32(2):301–5. doi: 10.2337/dc08-1241
58. Wang Y, Wan H, Chen Y, Xia F, Zhang W, Wang C, et al. Association of C-peptide with diabetic vascular complications in type 2 diabetes. Diabetes Metab (2020) 46(1):33–40. doi: 10.1016/j.diabet.2019.04.004
59. Azad N, Agrawal L, Emanuele NV, Klein R, Bahn GD, Reaven P, et al. Association of blood glucose control and pancreatic reserve with diabetic retinopathy in the Veterans Affairs Diabetes Trial (VADT). Diabetologia (2014) 57(6):1124–31. doi: 10.1007/s00125-014-3199-7
60. Thompson D, Begg I, Harris C, Ao Z, Fung MA, Meloche RM, et al. Reduced progression of diabetic retinopathy after islet cell transplantation compared with intensive medical therapy. Transplantation (2008) 85(10):1400–5. doi: 10.1097/TP.0b013e318172ca07
61. Moon CH, Lee AJ, Jeon HY, Kim EB, Ha KS. Therapeutic effect of ultra-long-lasting human C-peptide delivery against hyperglycemia-induced neovascularization in diabetic retinopathy. Theranostics (2023) 13(8):2424–38. doi: 10.7150/thno.81714
62. Wallerath T, Kunt T, Forst T, Closs EI, Lehmann R, Flohr T, et al. Stimulation of endothelial nitric oxide synthase by proinsulin C-peptide. Nitric Oxide (2003) 9(2):95–102. doi: 10.1016/j.niox.2003.08.004
63. Ushio-Fukai M, Nakamura Y. Reactive oxygen species and angiogenesis: NADPH oxidase as target for cancer therapy. JCl (2008) 266(1):37–52. doi: 10.1016/j.canlet.2008.02.044
64. Spindler V, Schlegel N, Waschke J. Role of GTPases in control of microvascular permeability. JCr (2010) 87(2):243–53. doi: 10.1093/cvr/cvq086
65. Monaghan-Benson E, Burridge K. The regulation of vascular endothelial growth factor-induced microvascular permeability requires Rac and reactive oxygen species. JTJobc (2009) 284(38):25602–11. doi: 10.1074/jbc.M109.009894
66. Lee YJ, Jung SH, Kim SH, Kim MS, Lee S, Hwang J, et al. Essential role of transglutaminase 2 in vascular endothelial growth factor-induced vascular leakage in the retina of diabetic mice. Diabetes (2016) 65(8):2414–28. doi: 10.2337/db15-1594
67. Chakrabarti S, Khan Z, Cukiernik M, Zhang W, Sima AA. C-peptide and retinal microangiopathy in diabetes. Exp Diabesity Res (2004) 5(1):91–6. doi: 10.1080/15438600490424569
68. Grunberger G, Qiang X, Li Z, Mathews ST, Sbrissa D, Shisheva A, et al. Molecular basis for the insulinomimetic effects of C-peptide. Diabetologia (2001) 44(10):1247–57. doi: 10.1007/s001250100632
69. Marx N, Silbernagel G, Brandenburg V, Burgmaier M, Kleber ME, Grammer TB, et al. C-peptide levels are associated with mortality and cardiovascular mortality in patients undergoing angiography: the LURIC study. Diabetes Care (2013) 36(3):708–14. doi: 10.2337/dc12-1211
70. Jahromi MK, Ahmadirad H, Jamshidi S, Farhadnejad H, Mokhtari E, Shahrokhtabar T, et al. The association of serum C-peptide with the risk of cardiovascular events: a meta-analysis and systematic review. Diabetol Metab Syndr (2023) 15(1):168. doi: 10.1186/s13098-023-01142-6
71. Hansen A, Johansson BL, Wahren J, von Bibra H. C-peptide exerts beneficial effects on myocardial blood flow and function in patients with type 1 diabetes. Diabetes (2002) 51(10):3077–82. doi: 10.2337/diabetes.51.10.3077
72. Luppi P, Cifarelli V, Tse H, Piganelli J, Trucco MJD. Human C-peptide antagonises high glucose-induced endothelial dysfunction through the nuclear factor-kappaB pathway. Diabetologia (2008) 51(8):1534–43. doi: 10.1007/s00125-008-1032-x
73. Cotter M, Ekberg K, Wahren J, Cameron NE. Effects of proinsulin C-peptide in experimental diabetic neuropathy: vascular actions and modulation by nitric oxide synthase inhibition. Diabetes (2003) 52(7):1812–7. doi: 10.2337/diabetes.52.7.1812
74. Lebherz C, Marx N. C-Peptide and its career from innocent bystander to active player in diabetic atherogenesis. Curr Atheroscler Rep (2013) 15(7):339. doi: 10.1007/s11883-013-0339-3
75. Mughal R, Scragg J, Lister P, Warburton P, Riches K, O'Regan DJ, et al. Cellular mechanisms by which proinsulin C-peptide prevents insulin-induced neointima formation in human saphenous vein. Diabetologia (2010) 53(8):1761–71. doi: 10.1007/s00125-010-1736-6
76. Cabrera de Leon A, Oliva Garcia JG, Marcelino Rodriguez I, Almeida Gonzalez D, Aleman Sanchez JJ, Brito Diaz B, et al. C-peptide as a risk factor of coronary artery disease in the general population. Diabetes Vasc Dis Res (2015) 12(3):199–207. doi: 10.1177/1479164114564900
77. Vasic D, Marx N, Sukhova G, et al. C-peptide promotes lesion development in a mouse model of arteriosclerosis. J Cell Mol Med (2012) 16(4):927–35. doi: 10.1111/j.1582-4934.2011.01365.x
78. Al-Rasheed NM, Chana RS, Baines RJ, Willars GB, Brunskill NJ. Ligand-independent activation of peroxisome proliferator-activated receptor-gamma by insulin and C-peptide in kidney proximal tubular cells: dependent on phosphatidylinositol 3-kinase activity. (2004) 279(48):49747–54. doi: 10.1074/jbc.M408268200
79. Elangbam CS, Tyler RD, Lightfoot RM. Peroxisome proliferator-activated receptors in atherosclerosis and inflammation–an update. Toxicol Pathol (2001) 29(2):224–31. doi: 10.1080/019262301317052495
80. Chawla A, Barak Y, Nagy L, Liao D, Tontonoz P, Evans RM. PPAR-gamma dependent and independent effects on macrophage-gene expression in lipid metabolism and inflammation. (2001) 7(1):48–52. doi: 10.1038/83336
81. Chima RS, LaMontagne T, Piraino G, Hake PW, Denenberg A, Zingarelli B. C-peptide, a novel inhibitor of lung inflammation following hemorrhagic shock. Am J Physiol Lung Cell Mol Physiol (2011) 300(5):L730–9. doi: 10.1152/ajplung.00308.2010
82. Walcher D, Babiak C, Poletek P, Rosenkranz S, Bach H, Betz S, et al. C-Peptide induces vascular smooth muscle cell proliferation: involvement of SRC-kinase, phosphatidylinositol 3-kinase, and extracellular signal-regulated kinase 1/2. Circ Res (2006) 99(11):1181–7. doi: 10.1161/01.RES.0000251231.16993.88
83. Wang W, Ji Q, Ran X, Li C, Kuang H, Yu X, et al. Prevalence and risk factors of diabetic peripheral neuropathy: A population-based cross-sectional study in China. Diabetes Metab Res Rev (2023):e3702. doi: 10.1002/dmrr.3702
84. Lian X, Qi J, Yuan M, Li X, Wang M, Li G, et al. Study on risk factors of diabetic peripheral neuropathy and establishment of a prediction model by machine learning. BMC Med Inform Decis Mak (2023) 23(1):146. doi: 10.1186/s12911-023-02232-1
85. Stevens MJ, Zhang W, Li F, Sima AA. C-peptide corrects endoneurial blood flow but not oxidative stress in type 1 BB/Wor rats. Am J Physiol Endocrinol Metab (2004) 287(3):E497–505. doi: 10.1152/ajpendo.00048.2004
86. Ekberg K, Brismar T, Johansson BL, Jonsson B, Lindstrom P, Wahren J. Amelioration of sensory nerve dysfunction by C-Peptide in patients with type 1 diabetes. Diabetes (2003) 52(2):536–41. doi: 10.2337/diabetes.52.2.536
87. Ekberg K, Brismar T, Johansson BL, Lindstrom P, Juntti-Berggren L, Norrby A, et al. C-Peptide replacement therapy and sensory nerve function in type 1 diabetic neuropathy. Diabetes Care (2007) 30(1):71–6. doi: 10.2337/dc06-1274
88. Wahren J, Foyt H, Daniels M, Arezzo JC. Long-acting C-peptide and neuropathy in type 1 diabetes: A 12-month clinical trial. Diabetes Care (2016) 39(4):596–602. doi: 10.2337/dc15-2068
89. Qiao X, Zheng H, Zhang S, et al. C-peptide is independent associated with diabetic peripheral neuropathy: a community-based study. (2017) 9:12. doi: 10.1186/s13098-017-0208-2
90. Umpierrez G, Korytkowski M. Diabetic emergencies - ketoacidosis, hyperglycaemic hyperosmolar state and hypoglycaemia. Nat Rev Endocrinol (2016) 12(4):222–32. doi: 10.1038/nrendo.2016.15
91. Suh J, Lee HI, Lee M, Song K, Choi HS, Kwon A, et al. Insulin requirement and complications associated with serum C-peptide decline in patients with type 1 diabetes mellitus during 15 years after diagnosis. Front Endocrinol (Lausanne) (2022) 13:869204. doi: 10.3389/fendo.2022.869204
92. Christensen MB, Gaede P, Hommel E, Gotfredsen A, Norgaard K. Glycaemic variability and hypoglycaemia are associated with C-peptide levels in insulin-treated type 2 diabetes. Diabetes Metab (2020) 46(1):61–5. doi: 10.1016/j.diabet.2019.02.002
93. Lee TH, Kwon AR, Kim YJ, Chae HW, Kim HS, Kim DH. The clinical measures associated with C-peptide decline in patients with type 1 diabetes over 15 years. J Korean Med Sci (2013) 28(9):1340–4. doi: 10.3346/jkms.2013.28.9.1340
94. Lee HJ, Yu HW, Jung HW, Lee YA, Kim JH, Chung HR, et al. Factors associated with the presence and severity of diabetic ketoacidosis at diagnosis of type 1 diabetes in korean children and adolescents. J Korean Med Sci (2017) 32(2):303–9. doi: 10.3346/jkms.2017.32.2.303
95. Qi M, Shao X, Li D, Zhou Y, Yang L, Chi J, et al. Establishment and validation of a clinical model for predicting diabetic ketosis in patients with type 2 diabetes mellitus. Front Endocrinol (Lausanne) (2022) 13:967929. doi: 10.3389/fendo.2022.967929
96. Koska J, Nuyujukian DS, Bahn GD, Zhou JJ, Reaven PD. Association of low fasting C-peptide levels with cardiovascular risk, visit-to-visit glucose variation and severe hypoglycemia in the Veterans Affairs Diabetes Trial (VADT). Cardiovasc Diabetol (2021) 20(1):232. doi: 10.1186/s12933-021-01418-z
97. Zenz S, Mader JK, Regittnig W, Brunner M, Korsatko S, Boulgaropoulos B, et al. Impact of C-peptide status on the response of glucagon and endogenous glucose production to induced hypoglycemia in T1DM. J Clin Endocrinol Metab (2018) 103(4):1408–17. doi: 10.1210/jc.2017-01836
98. Hope SV, Knight BA, Shields BM, Hill AV, Choudhary P, Strain WD, et al. Random non-fasting C-peptide testing can identify patients with insulin-treated type 2 diabetes at high risk of hypoglycaemia. Diabetologia (2018) 61(1):66–74. doi: 10.1007/s00125-017-4449-2
99. Rickels MR, Evans-Molina C, Bahnson HT, Ylescupidez A, Nadeau KJ, Hao W, et al. High residual C-peptide likely contributes to glycemic control in type 1 diabetes. J Clin Invest (2020) 130(4):1850–62. doi: 10.1172/JCI134057
100. Hosokawa Y, Kozawa J, Nishizawa H, Kawamori D, Maeda N, Otsuki M, et al. Positive correlation between fasting plasma glucagon and serum C-peptide in Japanese patients with diabetes. Heliyon (2019) 5(5):e01715. doi: 10.1016/j.heliyon.2019.e01715
Keywords: C-peptide, diabetic kidney disease, diabetic retinopathy, diabetic peripheral neuropathy, non-alcoholic fatty liver disease, macrovascular complications
Citation: Chen J, Huang Y, Liu C, Chi J, Wang Y and Xu L (2023) The role of C-peptide in diabetes and its complications: an updated review. Front. Endocrinol. 14:1256093. doi: 10.3389/fendo.2023.1256093
Received: 10 July 2023; Accepted: 18 August 2023;
Published: 07 September 2023.
Edited by:
Yanshan Dai, Bristol Myers Squibb, United StatesReviewed by:
Ke Wu, Wuhan University, ChinaXiang Ou, University of South China Affiliated Changsha Central Hospital, China
Copyright © 2023 Chen, Huang, Liu, Chi, Wang and Xu. This is an open-access article distributed under the terms of the Creative Commons Attribution License (CC BY). The use, distribution or reproduction in other forums is permitted, provided the original author(s) and the copyright owner(s) are credited and that the original publication in this journal is cited, in accordance with accepted academic practice. No use, distribution or reproduction is permitted which does not comply with these terms.
*Correspondence: Yangang Wang, d2FuZ3lnQHFkdS5lZHUuY24=; Lili Xu, cWRmeXhsbEBxZHUuZWR1LmNu
†These authors have contributed equally to this article