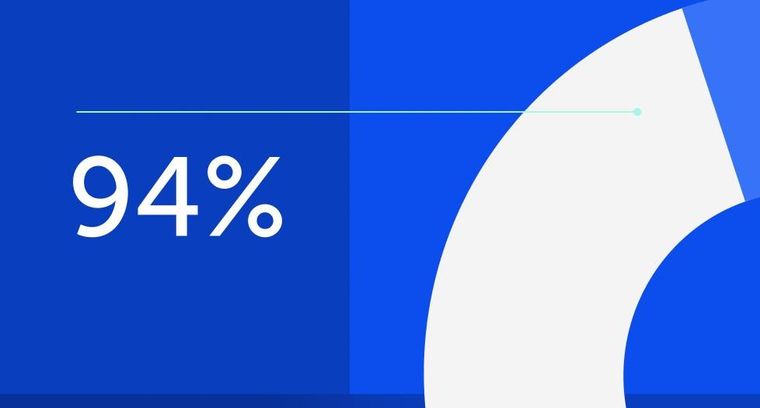
94% of researchers rate our articles as excellent or good
Learn more about the work of our research integrity team to safeguard the quality of each article we publish.
Find out more
REVIEW article
Front. Endocrinol., 23 August 2023
Sec. Obesity
Volume 14 - 2023 | https://doi.org/10.3389/fendo.2023.1250487
This article is part of the Research TopicNovel Regulatory Mechanisms behind Thermogenesis of Brown and Beige Adipocytes - Volume IIView all 8 articles
An energy imbalance cause obesity: more energy intake or less energy expenditure, or both. Obesity could be the origin of many metabolic disorders, such as type 2 diabetes and cardiovascular disease. UCP1 (uncoupling protein1), which is highly and exclusively expressed in the thermogenic adipocytes, including beige and brown adipocytes, can dissipate proton motive force into heat without producing ATP to increase energy expenditure. It is an attractive strategy to combat obesity and its related metabolic disorders by increasing non-shivering adipocyte thermogenesis. Adipocyte thermogenesis has recently been reported to be regulated by several new genes. This work provided novel and potential targets to activate adipocyte thermogenesis and resist obesity, such as secreted proteins ADISSP and EMC10, enzyme SSU72, etc. In this review, we have summarized the latest research on adipocyte thermogenesis regulation to shed more light on this topic.
Obesity is becoming more and more prevalent all over the world and is a huge risk to human health (1). An energy imbalance causes obesity: more energy intake or less energy expenditure, or both. So it is effective to prevent and treat obesity by reducing energy intake or increasing energy expenditure. GLP-1 has been clinically used to treat obesity effectively by decreasing energy intake (2, 3). In these years, lots of studies have reported that non-shivering adipocyte thermogenesis, which always maintains the body temperature, can be promoted to increase energy expenditure and resist obesity. It is a promising strategy to combat obesity by activating the thermogenic adipocytes (4, 5).
Adipose tissue is classically divided into white adipose tissue (WAT) and brown adipose tissue (BAT). Morphologically, white adipocyte has a unique large lipid droplet, while brown adipocyte has many small lipid droplets and more functional mitochondria (6). Functionally, white adipocyte stores energy and secrets many cytokines, including adiponectin and leptin et ac., to regulate metabolism (7); brown adipocyte highly express a mitochondrial inner membrane protein UCP1 (Uncoupling protein 1), which can dissipate proton motive force into heat without producing ATP. Under cold or pharmacological conditions, a brown-like adipocyte called a beige adipocyte or brite adipocyte, is induced in white adipose tissue (8). Like brown adipocyte, beige adipocyte have many small lipid droplets and highly expressed UCP1 to produce heat; brown and beige adipocytes are also called thermogenic adipocytes (Figure 1). Brown and beige adipocytes are functionally the same, but their origins differ. Beige and white adipocytes are mostly Myf5 negative (9–11), while brown adipocytes are Myf5 positive and share a precursor cell with myocyte (12). Prdm16 is the master gene for brown and beige adipocyte identity. Overexpression of Prdm16 in myoblast can trans-differentiate it into brown adipocyte (13, 14), and the knockout of Prdm16 in white adipocyte tissue abolished the induction of beige adipocyte by cold (15, 16).
Figure 1 Three types of adipocytes: white adipocyte, beige adipocyte, and brown adipocyte. Beige adipocyte and brown adipocytes are thermogenic adipocytes for non-shivering thermogenesis.
Interestingly, when the beta-adrenergic signaling pathway was ablated in white adipose tissue, a kind of glycolytic beige adipocyte was induced from Myf5 positive precursor cells (17). Brown adipose tissue is located in the interscapular depot in mammals, and white adipose tissue is mainly located in the subcutaneous and visceral depot (6). It is noted that brown adipose tissue gradually disappeared when humans grew up (18, 19). There are brown and beige adipocytes in the deep human neck (20, 21). In addition, beige and brown adipocytes positively correlate with body weight (22, 23).
Cold exposure is the most effective way to stimulate adipocyte thermogenesis. Upon cold exposure, adipose tissue was sympathetically innervated. The secreted norepinephrine binds with a beta-adrenergic receptor in adipocytes and activates the Gs signaling pathway and adenylyl cyclase to elevate the cycle adenosine (cAMP) contents. And then, cAMP binds with the regulatory subunit of protein kinase A (PKA) to activate its catalytic subunit C to phosphorylate the following transcriptional factor CREB. The phosphorylated protein CREB enters the nucleus and upregulates thermogenic genes, including Ucp1 et al. (24–27). Many studies have reported that many kinds of cytokines, transcriptional factors, kinases, and membrane receptors, like FGF21, IRF4, and ZFP516 et al., are involved in adipocyte thermogenesis (28–31). Here, we have summarized the latest studies involved in adipocyte thermogenesis listed in Table 1 and Figure 2.
Figure 2 A schematic picture illustrates the latest studies on adipocyte thermogenesis regulation. The green color stands for the positive regulators, and the brown color stands for the negative ones.
Due to differential splicing, endoplasmic reticulum membrane complex subunit 10 has two isoforms: membrane EMC10 and secreted EMC10 (scEMC10) (45, 46). ScEMC10 serum contents in white and Chinese Han human populations were positively correlated with obesity and insulin resistance. After bariatric surgery or exercise and caloric restriction, serum scEMC10 levels decreased. The whole-body Emc10 knockout mice, displaying more adipocyte thermogenesis and higher whole-body energy expenditure, resisted obesity and insulin resistance induced by high-fat diets. Conversely, Emc10 overexpression mice were more easily induced into obesity and insulin resistance on high-fat diets, had less adipocyte thermogenesis, and lower whole-body energy expenditure. In addition, the circulating neutralizing antibody to EMC10 also helped mice to reduce body weight gain. Mechanically, secreted EMC10 can be taken up by adipocytes and interacted with the Protein Kinase A catalytic subunit α to inhibit it from phosphorylating CREB. So the decreased phosphorylated CREB proteins led to a reduction in adipocyte thermogenesis (47).
ADISSP (Adipose secreted signaling protein) is a new uncharacterized adipokine, highly and selectively expressed in brown adipose tissue in humans and mice. The expression of this protein is higher in adipose tissue in normal people than in obese people and is negatively correlated with body weight index. The overexpression of Adissp in adipose tissue by Ap2 promoter-driven promoted adipocyte thermogenesis, augmented oxygen consumption, increased glycolysis, elevated body temperature, and resisted body weight gain induced by high-fat diets. In contrast, adipose-specific Adissp knockout mice had lower body temperature, less adipocyte thermogenesis, and were more susceptible to high-fat diet-induced obesity and hyperglycemia. ADISSP promoted white adipocyte browning and brown adipocyte activity by paracrine signaling but not by endocrine signaling. A more sensitive assay is lacking to measure ADISSP endogenous circulating contents. The PKA signaling pathway can be activated independently of the beta-adrenergic signaling pathway by ADISSP (32). Though a surface receptor in adipocytes binds to ADISSP, the receptor gene is still not confirmed and unknown.
HIFα (Hypoxia-inducible factor α, HIFα) has two family members: HIF1α and HIF2α (48). These two genes were upregulated in white and brown adipose tissue after cold exposure. The adipose Hif1α, or Hif2α, or double-specific knockout mice had more beige adipocytes in the inguinal adipose tissue and higher energy expenditure compared to the wild-type mice upon cold exposure or CL316243 (beta-adrenergic agonist) stimulation. By RNA-Seq analysis, Prkaca, which encodes PKA catalytic subunit α (PKA Cα), was a highly ranked gene in adipose tissue of Hif2α knockout mice. Using in silico analysis, miR-3085-3p, directly regulated by HIF2α, was identified to target the evolutionarily conserved region of 3’ UTR of Prkaca. So, adipocyte HIF2α could suppress PKA Cα-mediated thermogenic properties by promoting miR-3085-3p expression (33).
Ovo-like zinc finger 2 (OVOL2) belongs to the Ovo family of zinc-finger transcription factors family and is highly conserved in invertebrates and vertebrates (49). The C57BL/6J mice with Oval2 mutations induced by Nethyl-N-nitrosourea (ENU), named Oval2 boh/boh, were characterized by increased body weight without affecting food intake under a standard chow diet. The boh mutation is a single nucleotide transition from G to A, causing the substitution of tyrosine for a conserved cysteine, and does not affect the OVOL2 protein’s stability. Ovol2 knockout is embryonically lethal. The heterozygotes with the boh allele and the null allele of Ovol2 (Ovol2 boh/-) exhibited overall stronger phenotypes: obesity, reduced energy expenditure, and adipocyte thermogenesis. Overexpression of Ovol2 in adipocytes reduced total body and liver fat and improved insulin sensitivity in mice fed on a high-fat diet. Ovol2 is highly expressed in white adipose stromal cells but not in mature white adipocytes. OVOL2 can block adipogenesis by interacting with the C-terminal portion of C/EBPα to inhibit its adipogenic function in both mouse and human adipocytes (34). The mechanism of OVOL2 in brown adipocytes remains elusive and needs more investigation.
Transmembrane protein 86A (TMEM86A) is a putative lysoplasmalogenase, a close homolog of TMEM86B, and a YhhN family protein member (50, 51). Tmem86a expression was significantly upregulated in adipose tissue on high-fat diets compared to the standard chow diet and heavily enriched in mature adipocytes. Transcriptome-profiling (GEO: GSE94753) also shows that TMEM86A expression is upregulated in abdominal subcutaneous WAT from female patients with obesity compared to individuals without obesity. The adipose tissue-specific Tmem86a knockout mice had increased mitochondrial metabolism, adipocyte thermogenesis, and energy expenditure and exhibited significantly lower body weight gain. Mechanistically, the untargeted lipidomics analysis suggested that Tmem86a overexpression downregulated the content of lysoplasmalogens, including plasmatic lysophosphatidylethanolamine 18:0 (LPE P-18:0) and adipocyte-specific Tmem86a knockout (AKO) increases LPE P-18:0 content in adipose tissue. LPE P-18:0 could reduce the activity of PDE3B (phosphodiesterase 3b), which is abundantly expressed in adipocytes and can degrade cAMP. Furthermore, LPE P-18:0 treatment reduced body weight and fat mass, increased energy expenditure, and strongly activated the PKA signaling pathway in adipose tissue (35).
SSU72 is a dual-specific protein phosphatase and is expressed in a tissue-specific manner. Recent studies have demonstrated that SSU72 plays an important role in controlling the carboxyl-terminal domain (CTD) function of RNA polymerase II (RNAPII) and monitoring the liver (52–54). In adipose tissue, SSU72 phosphatase was highly enriched in brown adipose tissue relative to white adipose tissue. Ssu72 mRNA and protein levels were increased significantly in BAT and WAT after cold exposure. BAT from adipose-specific Ssu72 knockout mice appeared pale and showed enlarged mitochondria and disorganized cristae structures compared to WT mice. The knockout mice were much more cold-sensitive and intolerant after cold exposure. The expression of thermogenic genes and fatty acid β-oxidation genes was significantly attenuated in the brown adipose tissue of AKO mice.
When endoplasmic reticulum (ER) homeostasis is disrupted, PKR-like ER-regulated kinase (PERK) is activated to phosphorylate the α subunit of eukaryotic initiation factor 2 (eIF2α) at serine 51 (Ser51). EIF2α phosphorylation represses most of the proteins’ translation to reduce ER stress. Dephosphorylation of eIF2α by its phosphatase GADD34 in the liver can improve insulin sensitivity and reduce hepatosteatosis in mice fed high-fat diets. By RNA-Seq analysis, most PERK-eIF2α target genes were significantly upregulated in AKO BAT. Mechanistically, SSU72 can directly interact with eIF2α to inhibit its phosphorylation and increase the protein translation of mitochondrial oxidative phosphorylation and adipocyte thermogenesis in BAT. Furthermore, metabolic dysfunction in Ssu72-abated BAT could return to almost normal after restoring Ssu72 expression (36).
PR domain-containing 16 (PRDM16) is a master gene that controls the biogenesis of brown and beige adipocytes by forming a complex with transcriptional and epigenetic factors (12, 13, 15). PRDM16 is dynamically regulated at the post-translational level. Overexpression of euchromatic histone-lysine N-methyltransferase 1 (EHMT1) or chronic treatment with synthetic ligands of peroxisome proliferator-activated receptor-γ (PPARγ) prolongs PRDM16 protein’s half-life (55, 56). CUL2–APPBP2 complex as the ubiquitin E3 ligase was identified to determine PRDM16 protein stability by catalyzing its polyubiquitination. CUL2 functions as a scaffold protein by interacting with an E2 enzyme, elongation B (ELOB), elongation C (ELOC), and APPBP2 substrate receptor, also found in RING E3 ligase complexes (57, 58). Cul2 depletion in white adipocytes extended the half-life of PRDM16 protein and significantly increased uncoupled cellular respiration. Overexpression of Cul2 in adipocytes reduced brown/beige-fat-selective genes’ expression. Consistent with the results of Cul2 deletion, deletion of Appbp2 also led to higher PRDM16 protein levels and increased expression of brown/beige-fat-selective genes compared with the control cells. APPBP2 (S561N) variant, associated with lower levels of 2 h postprandial serum glucose and insulin, weakly interacted with PRDM16 protein relative to WT APPBP2. Differentiated primary adipocytes from Appbp2 mutant mice expressed higher thermogenic gene levels than WT adipocytes. Besides, adipose-specific Cul2 or Appbp2 knockout mice expressed higher levels of PRDM16 protein and adipose thermogenesis, displayed significantly higher whole-body energy expenditure, and gained less body weight than controls (37).
The transforming growth factor β (TGFβ) signaling pathway is complex and associated with various human pathologies (59). By comparing the transcriptome of human supraclavicular BAT (scBAT) and subcutaneous WAT and analyzing the transcriptome of the human multipotent adipose-derived stem (hMADS) cells differentiated into beige and white adipocytes, Gpr180 was found to be upregulated in brown fat on both tissue and cellular level. The knockdown of Gpr180 shifted brown and beige adipocytes towards a white-like phenotype. The whole body or inducible adipose tissue knockout of Gpr180 diminished brown and beige adipocyte function and impaired insulin resistance.
The knockdown of Gpr180 did not affect cAMP levels and phosphorylation of PKA substrates. However, it reduced the phosphorylation of SMAD3 protein at serine 423 in the matured adipocyte. Besides, TGFβ1-induced phosphorylation of SMAD3 and upregulation of Ucp1 were attenuated in beige adipocytes without GPR180, which indicates that GPR180 is required for full activation of the TGFβ signaling machinery. Collagen triple helix repeat containing 1 (CTHRC1) was identified as GRP180’s potential ligand. Overexpression of Cthrc1 in male mice prevented body weight gain and increased energy expenditure during the HFD-induced weight gain. In sum, GPR180 and CTHRC1 are the components of the TGFβ signaling pathway for adipocyte thermogenesis. These components regulate low-grade SMAD3 phosphorylation and control thermogenic adipocyte function, whole-body energy, and glucose homeostasis (38).
Circulating IL18 level is associated with body weight, insulin resistance, and metabolic syndrome in humans and mice (60). Il18 ablation in mice led to hyperphagia, obesity, insulin resistance, and decreased energy expenditure. Whole-body deletion of Il18r increased body weight and decreased energy expenditure, but white adipocyte browning was enhanced in mice on a chow diet (61, 62). This can be explained by the NaCl co-transporter (NCC) acting as an alternative receptor for IL18’s differential function in adipocyte thermogenesis. A single knockout of Ncc or a combined knockout of Il18r and Ncc, but not a single knockout of Il18r, blocked adipocyte thermogenesis. Consistent with this, brown adipocytes from Ncc−/− and Il18r −/− Ncc−/− mice, but not those from Il18r −/− mice, showed decreased levels of IL18 and induced uncoupled respiration.
Furthermore, Ncc fi/fi Ucp1Cre mice gained more body weight, had lower adipocyte thermogenesis, and showed worse glucose intolerance and insulin resistance on high-fat diets. There is no difference in UCP1 positive areas and thermogenic genes’ expression in adipose tissue between Il18rfi/fi Ucp1Cre mice and control mice. However, the concise mechanism of IL18 for Ucp1 promotion is still unclear. It is only known that it is not dependent on the cAMP signaling pathway in brown adipocytes. Overall, IL18 uses NCC to promote thermogenesis in BAT but uses IL18R to enhance glucose sensitivity in WAT (39).
The mitochondrial cristae biogenesis protein optic atrophy 1 (OPA1) was significantly downregulated in the human subcutaneous adipose tissue (SAT) of heavy co-twins by gene expression analysis and correlated with mitochondrial gene expression. Opa1tg mice with ubiquitous mild Opa1 overexpression were slightly resistant to high-fat diet-induced obesity, glucose intolerance, and hepatic steatosis compared to their littermate controls. Similarly, resistance to obesity was observed in a mouse model of Opa1 haploinsufficiency (63). Opa1tg mice produce more heat at room temperature by promoting BAT function and WAT browning. Opa1 facilitates cell-autonomous adipocyte browning by upregulating Kdm3a, a member of the Jumanji demethylase. This increases brown and beige thermogenic activity by controlling the H3K9 methylation status of Adrb1 and Ucp1 (64, 65). Metabolomic profiling further revealed that fumarate levels produced from the urea cycle derived Kdm3a-dependent Ucp1 induction in adipocytes. Moreover, overexpression of Opa1 can increase cAMP contents to activate CREB to upregulate the rate-limiting urea cycle enzyme Cps1 (carbamoyl phosphate synthetase-1) in adipose tissue (40). Although OPA1 may not depend on its pro-mitochondrial fusion role, more research is needed to determine the mechanism by which it activates CREB.
By dissipating energy as heat, UCP1 mediates adaptive thermogenesis. Long-chain fatty acids bind on UCP1 to drive proton leak, while purine nucleotides bind on UCP1 to block this uncoupling process (66, 67). Sulfenylation of UCP1 on Cys253 is essential for acute cold-induced uncoupled respiration, while the lysine succinylation of UCP1 reduces its activity and stability (68). Cytosolic calcium can directly stimulate adenylyl cyclase activity to increase cAMP production and PKA activation to induce thermogenesis (69). Two endoplasmic reticulum-located calcium channels, sarco/endoplasmic reticulum Ca2+-ATPase 2b (SERCA2b) and ryanodine receptor 2 (RyR2), are involved in ATP-dependent and UCP1-independent calcium cycling machinery to dissipate energy as heat in a beige adipocyte (70).
The mitochondrial calcium uniporter (MCU) complex, which consists of a pore-forming subunit (MCU) and several regulatory subunits, including essential MCU regulator (EMRE) and mitochondrial calcium uptake 1 (MICU1) et al., is a key regulator of mitochondrial calcium (71). Mcu BKO (Mcuf/f with Ucp1Cre mice) and Emre BKO (AAV-Emre gRNA into BAT of Rosa26-LSL-Cas9 with AdipoqCre) mice are hypothermic. They could not maintain their core body temperatures when challenged with cold exposure. Upon adrenergic stimulation, MCU recruits UCP1 through EMRE to form an MCU-EMRE-UCP1 complex (thermo porter), which increases mitochondrial calcium uptake to accelerate the tricarboxylic acid cycle and supply more protons that promote uncoupled respiration. A mutant EMCU (unable to conduct Ca2+) could interact with UCP1 at a similar level as the WT EMCU, decreasing the level of UCP1-dependent respiration. MICU1 is the gatekeeper to prevent Ca2+ overload in mitochondria by interacting with MCU. Their interaction markedly decreased upon cold exposure or NE/CL-316,243 treatment. AAV-Micu1 BKO (AAV-Micu1 gRNA into Rosa26-LSL-Cas9 with Adipoq-Cre) enhanced brown adipocyte uncoupled respiration and energy expenditure when activated by NE. The mice carrying the enforcedly assembled thermo porter gained less body weight, more glucose and insulin tolerance, and increased animal energy expenditure; the opposite metabolic phenotypes were observed in Mcu BKO and Emre BKO mice (41).
Because UCP1 loss causes the depletion of most components of the mitochondrial electron transport chain (ETC) in BAT, the interpretation of phenotypes of Ucp1 KO mice is bewildering and confusing (72). Thermogenic reactive oxygen species (ROS) could reversibly modify a regulatory site (C253) on UCP1 to elevate UCP1-dependent respiration (67). Based on these findings, a mouse model in which the regulatory C253 site on UCP1 is mutated to an alanine (UCP1 C253A mouse) was generated to examine its role in regulating energy homeostasis and metabolic disease. Quantitative proteomics of BAT from WT, UCP1 KO, and UCP1 C253A mice demonstrated that BAT from UCP1 C253A mice maintained expression of the full mitochondrial metabolic proteins. Both male and female UCP1 C253A mice exhibited significantly lower VO2 consumption, energy expenditure, and VCO2 production in response to cold exposure. Fed on a high-fat, high-sucrose (HFHS) diet, male, and female WT and UCP1 C253A mice gained indistinguishable body weight, and their food intake was identical. Remarkably, male but not female C253A mice exhibited more glucose intolerance than WT mice. The proteomic analysis of adipose tissues from both male and female mice on HFHS diets suggested that UCP1 C253A strongly agonizes WAT inflammation in male but not female mice. The mutation of UCP1 C253A increased mitochondrial protein oxidation and systemic inflammation in male mice since the inflammatory cytokine expression was attenuated upon the supplementation of MitoQ (73), a mitochondria-targeted antioxidant. UCP1 C253A male mice treated with β-estradiol showed the decreased expression of inflammatory cytokines, which were significantly increased in BAT of untreated C253A mice (42).
COVID-19 caused patients adipose atrophy, weight loss, and cachexia by activating adipocyte thermogenesis. A transgenic mouse (ACE2Tg) that knocked in human angiotensin-converting enzyme 2 (ACE2) demonstrated progressive weight loss alongside ‘wild-type’ SARS-CoV-2 virus infection. SARS-CoV-2 infection augments adipose thermogenesis and increases thermogenic genes’ expression and UCP1 positive cells by histological and immunohistochemical analysis in BAT, sWAT (subcutaneous WAT) and vWAT (visceral WAT). SARS-CoV-2-infected adipose tissues suffered from hypoxia with high HIF1α expression and contained high levels of VEGF, a main target of HIF1α (74, 75). VEGF is a crucial angiogenic factor that augments adipose tissue browning (76, 77). Anti-VEGF mouse neutralizing antibody largely restored the sWAT and vWAT mass relative to the non-immune IgG (NIIgG)-treated sWAT and vWAT. As seen in mouse models, browning phenotypes of adipose tissues were also observed in SARS-CoV-2-infected Syrian hamsters and human patients who died of severe COVID-19 (43).
Zn is one of life’s most important essential trace elements and has been associated with insulin resistance and adiposity (78). The low level of plasma Zn is associated with obesity. Its supplementation significantly reduces body weight and plasma cholesterol and triglycerides (79, 80). Cold induces sympathetic innervation, which promotes UCP1 expression and Zn secretion from thermogenic adipocytes. However, Zn did not directly influence thermogenic genes’ expression in primary beige adipocytes. Zn stimulated the length of primary sympathetic neurons’ neurite outgrowth to contribute to thermogenesis. Zn injection induced sympathetic innervation in scWAT and BAT.
On the contrary, local injection of Zn chelator TPEN in scWAT and BAT caused a decrease in sympathetic innervation. Furthermore, 6-hydroxidopamine (6-OHDA), which locally ablates sympathetic fibers in BAT and scWAT of Zn-treated HFD mice, blocked the anti-obesity effect of Zn treatment. MT2, cysteine-rich proteins that bind to Zn with high affinity, in scWAT and BAT are upregulated by high-fat diets. MT2 expression is increased in human scWAT samples from obese individuals and positively correlated with body mass index (BMI). Adipose tissue-specific Mt2 knockout increased VO2, thermogenic genes’ expression, and UCP1 protein levels in scWAT and BAT. At the same time Mt2 overexpression in BAT and scWAT resulted in decreased sympathetic innervation, VO2, and thermogenic gene expression without changes in body weight (44).
Adipocyte thermogenesis is regulated by complex transcriptional factors like PRDM16, PPARγ, EBF2, ect, and has been studied extensively (8, 81). Recently, Emc10, Tmem86a, Hif2α, and Adissp have been reported to be involved in adipocyte thermogenesis by regulating the PKA-CREB signaling pathway. This pathway is mainly activated by cold exposure or beta-adrenergic agonists and has been proven to be the most effective way to activate adipocyte thermogenesis. Secreted EMC10 can interact with the Protein Kinase A catalytic subunit α to inhibit it from phosphorylating CREB, HIF2α negatively regulated the expression of Prkaca. In contrast, TMEM86A blocked the activation of PKA by reducing the level of cAMP. Conversely, OPA1 can increase cAMP contents to activate the PKA-CREB pathway. In addition, the PKA signaling pathway can be directly activated by ADISSP, but its concise molecular mechanism was not clarified. Thus, regulating the PKA-CREB pathway in adipocyte thermogenesis could still be an important research area. Upon ER stress, PERK is activated to increase eIF2α phosphorylation to repress most of the proteins’ translation. SSU72 can directly interact with eIF2α to inhibit its phosphorylation and increase the protein translation of adipocyte thermogenesis in BAT. Otherwise, the knockdown or knockout of PERK was not tested to examine whether it can promote or inhibit adipocyte thermogenesis in this study. ISR (Integrated stress response) pathway, including PERK, PKR, HRI, and GCN2 is also known to regulate phosphorylation of eIF2α (82), so there are still more studies needed to investigate the regulation of eIF2α phosphorylation in adipocyte thermogenesis.
UCP1 protein is always regarded as an independent uncoupling protein to dissipate proton motive force without producing ATP, a new role of UCP1 in adipocyte thermogenesis has been expanded so that it can form a MCU-EMRE-UCP1 complex to increase mitochondrial calcium uptake to accelerate the tricarboxylic acid cycle and supply more protons that promote uncoupled respiration. It was previously reported that SERCA2b and RYR2 in the endoplasmic reticulum promote UCP1-independent adipocyte thermogenesis by regulating ATP-dependent calcium cycling machinery in beige adipocytes (70). This recent work revealed how Ca2+ regulates adipocyte thermogenesis in mitochondria and found that UCP1 can interact with other proteins to exert its effect. Investigating whether more proteins can interact with UCP1 to play roles in adipocyte thermogenesis is intriguing. Oval2 is reported to promote white adipogenesis and regulate beige and brown adipocyte thermogenesis. It’s unknown whether this gene would affect beige or brown adipogenesis since they all share a similar adipogenic mechanism depending on the activation of PPARγ (83). In addition, there is also an alternative way to promote adipocyte thermogenesis by increasing sympathetic innervation in adipose tissue. COVID-19 infection promoted the angiogenesis of adipose tissue and Zn stimulated the length of primary sympathetic neurons’ neurite outgrowth so that more adipocytes could be innervated for adipose thermogenesis. Although the research works mentioned here are novel and interesting, most of them are firstly reported here and based on a single study from a single research group. We consider that it will be more compelling and reliable if these results could be repeated by other research groups. Moreover, studies including more experimental repetitions are needed, in order to confirm that these genes are also effective in human adipocyte thermogenesis. In Table 1, we listed research works that include human studies. These research works have just tested the expression levels or functions of these relevant genes, using experiments performed either in vitro or in adipose tissue from healthy and obese human patients. However, they did not include sufficient data to support their associations with human energy expenditure. As such, it is still necessary to demonstrate that these genes are able to in vivo regulate human energy expenditure.
It is intriguing to combat human metabolic disorders and obesity by targeting adipocyte thermogenesis. In humans, mild cold exposure, which can activate adrenergic receptor signaling pathways, could increase the rates of glucose, fatty acid uptake, and oxidative metabolism in the brown adipose tissue (84, 85). Moreover, the oral administration of mirabegron (a human β3 adrenergic receptor agonist approved for overactive bladder treatment), could activate human brown adipose tissue and increase whole-body energy expenditure (86, 87). Furthermore, a retrospective cohort study concluded that humans with positive BAT had a healthier body fat distribution, with a decreased visceral adipose tissue content and an increased subcutaneous adipose tissue content, as well as improved metabolic symptoms, such as lower blood glucose and lipids, and decreased liver fat accumulation (88). Moreover, another independent retrospective cohort study has also reported an association of human positive BAT with improved cardiometabolic health in terms of dyslipidemia, coronary artery disease, congestive heart failure, and hypertension, especially in overweight or obese individuals (89). However, progress in combating obesity by targeting human thermogenic adipocyte tissue is relatively slow due to issues including ethical reasons. Nevertheless, extensive rodent research has demonstrated the feasibility of combating obesity by targeting thermogenic adipose tissues (90, 91). In sum, though most of these recent findings have been analyzed or verified in human adipocytes or adipose tissue, it is still a long and arduous way to transform these basic studies into a clinic.
TN, HZ, and LM wrote and revised the manuscript. JL revised the manuscript. All authors contributed to the article and approved the submitted version.
This study was supported in part by the National Natural Science Foundation of China (81970729), the Natural Science Foundation of Guangdong province (2022A1515010283), initial funding from Hubei University of Arts and Science (2059200), and Pearl River S&T Nova Program of Guangzhou (201806010166).
The authors would like to thank all the reviewers who participated in the review and EditSpring for its linguistic assistance during the preparation of this manuscript.
The authors declare that the research was conducted in the absence of any commercial or financial relationships that could be construed as a potential conflict of interest.
All claims expressed in this article are solely those of the authors and do not necessarily represent those of their affiliated organizations, or those of the publisher, the editors and the reviewers. Any product that may be evaluated in this article, or claim that may be made by its manufacturer, is not guaranteed or endorsed by the publisher.
1. Jaacks LM, Vandevijvere S, Pan A, McGowan CJ, Wallace C, Imamura F, et al. The obesity transition: stages of the global epidemic. Lancet Diabetes Endocrinol (2019) 7(3):231–40. doi: 10.1016/S2213-8587(19)30026-9
2. Gallwitz B. Clinical perspectives on the use of the gip/glp-1 receptor agonist tirzepatide for the treatment of type-2 diabetes and obesity. Front Endocrinol (Lausanne) (2022) 13:1004044. doi: 10.3389/fendo.2022.1004044
3. Drucker DJ. Mechanisms of action and therapeutic application of glucagon-like peptide-1. Cell Metab (2018) 27(4):740–56. doi: 10.1016/j.cmet.2018.03.001
4. Cohen P, Kajimura S. The cellular and functional complexity of thermogenic fat. Nat Rev Mol Cell Biol (2021) 22(6):393–409. doi: 10.1038/s41580-021-00350-0
5. Wang Z, Yu X, Chen Y. Recruitment of thermogenic fat: trigger of fat burning. Front Endocrinol (Lausanne) (2021) 12:696505. doi: 10.3389/fendo.2021.696505
6. Zwick RK, Guerrero-Juarez CF, Horsley V, Plikus MV. Anatomical, physiological, and functional diversity of adipose tissue. Cell Metab (2018) 27(1):68–83. doi: 10.1016/j.cmet.2017.12.002
7. Rosen ED, Spiegelman BM. What we talk about when we talk about fat. Cell (2014) 156(1-2):20–44. doi: 10.1016/j.cell.2013.12.012
8. Wang W, Seale P. Control of brown and beige fat development. Nat Rev Mol Cell Biol (2016) 17(11):691–702. doi: 10.1038/nrm.2016.96
9. Wu J, Bostrom P, Sparks LM, Ye L, Choi JH, Giang AH, et al. Beige adipocytes are a distinct type of thermogenic fat cell in mouse and human. Cell (2012) 150(2):366–76. doi: 10.1016/j.cell.2012.05.016
10. Sanchez-Gurmaches J, Hung CM, Sparks CA, Tang Y, Li H, Guertin DA. Pten loss in the myf5 lineage redistributes body fat and reveals subsets of white adipocytes that arise from myf5 precursors. Cell Metab (2012) 16(3):348–62. doi: 10.1016/j.cmet.2012.08.003
11. Shan T, Liang X, Bi P, Zhang P, Liu W, Kuang S. Distinct populations of adipogenic and myogenic myf5-lineage progenitors in white adipose tissues. J Lipid Res (2013) 54(8):2214–24. doi: 10.1194/jlr.M038711
12. Seale P, Bjork B, Yang W, Kajimura S, Chin S, Kuang S, et al. Prdm16 controls a brown fat/skeletal muscle switch. Nature (2008) 454(7207):961–7. doi: 10.1038/nature07182
13. Seale P, Kajimura S, Yang W, Chin S, Rohas LM, Uldry M, et al. Transcriptional control of brown fat determination by prdm16. Cell Metab (2007) 6(1):38–54. doi: 10.1016/j.cmet.2007.06.001
14. Kajimura S, Seale P, Kubota K, Lunsford E, Frangioni JV, Gygi SP, et al. Initiation of myoblast to brown fat switch by a prdm16-C/ebp-beta transcriptional complex. Nature (2009) 460(7259):1154–8. doi: 10.1038/nature08262
15. Seale P, Conroe HM, Estall J, Kajimura S, Frontini A, Ishibashi J, et al. Prdm16 determines the thermogenic program of subcutaneous white adipose tissue in mice. J Clin Invest (2011) 121(1):96–105. doi: 10.1172/JCI44271
16. Cohen P, Levy Julia D, Zhang Y, Frontini A, Kolodin Dmitriy P, Svensson Katrin J, et al. Ablation of prdm16 and beige adipose causes metabolic dysfunction and a subcutaneous to visceral fat switch. Cell (2014) 156(1-2):304–16. doi: 10.1016/j.cell.2013.12.021
17. Chen Y, Ikeda K, Yoneshiro T, Scaramozza A, Tajima K, Wang Q, et al. Thermal stress induces glycolytic beige fat formation via a myogenic state. Nature (2019) 565(7738):180–5. doi: 10.1038/s41586-018-0801-z
18. Aherne W, Hull D. Brown adipose tissue and heat production in the newborn infant. J Pathol Bacteriol (1966) 91(1):223–34. doi: 10.1002/path.1700910126
20. Cypess AM, White AP, Vernochet C, Schulz TJ, Xue R, Sass CA, et al. Anatomical localization, gene expression profiling and functional characterization of adult human neck brown fat. Nat Med (2013) 19(5):635–9. doi: 10.1038/nm.3112
21. Lidell ME, Betz MJ, Dahlqvist Leinhard O, Heglind M, Elander L, Slawik M, et al. Evidence for two types of brown adipose tissue in humans. Nat Med (2013) 19(5):631–4. doi: 10.1038/nm.3017
22. Lee P, Greenfield JR, Ho KK, Fulham MJ. A critical appraisal of the prevalence and metabolic significance of brown adipose tissue in adult humans. Am J Physiol Endocrinol Metab (2010) 299(4):E601–6. doi: 10.1152/ajpendo.00298.2010
23. Pfannenberg C, Werner MK, Ripkens S, Stef I, Deckert A, Schmadl M, et al. Impact of age on the relationships of brown adipose tissue with sex and adiposity in humans. Diabetes (2010) 59(7):1789–93. doi: 10.2337/db10-0004
24. Xue B, Coulter A, Rim JS, Koza RA, Kozak LP. Transcriptional synergy and the regulation of ucp1 during brown adipocyte induction in white fat depots. Mol Cell Biol (2005) 25(18):8311–22. doi: 10.1128/MCB.25.18.8311-8322.2005
25. Torres-Quesada O, Mayrhofer JE, Stefan E. The many faces of compartmentalized pka signalosomes. Cell Signal (2017) 37:1–11. doi: 10.1016/j.cellsig.2017.05.012
26. Rim JS, Kozak LP. Regulatory motifs for creb-binding protein and nfe2l2 transcription factors in the upstream enhancer of the mitochondrial uncoupling protein 1 gene. J Biol Chem (2002) 277(37):34589–600. doi: 10.1074/jbc.M108866200
27. Collins S. Beta-adrenergic receptors and adipose tissue metabolism: evolution of an old story. Annu Rev Physiol (2022) 84:1–16. doi: 10.1146/annurev-physiol-060721-092939
28. Kong X, Banks A, Liu T, Kazak L, Rao RR, Cohen P, et al. Irf4 is a key thermogenic transcriptional partner of pgc-1alpha. Cell (2014) 158(1):69–83. doi: 10.1016/j.cell.2014.04.049
29. Lee P, Linderman JD, Smith S, Brychta RJ, Wang J, Idelson C, et al. Irisin and fgf21 are cold-induced endocrine activators of brown fat function in humans. Cell Metab (2014) 19(2):302–9. doi: 10.1016/j.cmet.2013.12.017
30. Fisher FM, Kleiner S, Douris N, Fox EC, Mepani RJ, Verdeguer F, et al. Fgf21 regulates pgc-1alpha and browning of white adipose tissues in adaptive thermogenesis. Genes Dev (2012) 26(3):271–81. doi: 10.1101/gad.177857.111
31. Dempersmier J, Sambeat A, Gulyaeva O, Paul SM, Hudak CS, Raposo HF, et al. Cold-inducible zfp516 activates ucp1 transcription to promote browning of white fat and development of brown fat. Mol Cell (2015) 57(2):235–46. doi: 10.1016/j.molcel.2014.12.005
32. Chen Q, Huang L, Pan D, Hu K, Li R, Friedline RH, et al. A brown fat-enriched adipokine adissp controls adipose thermogenesis and glucose homeostasis. Nat Commun (2022) 13(1):7633. doi: 10.1038/s41467-022-35335-w
33. Han JS, Jeon YG, Oh M, Lee G, Nahmgoong H, Han SM, et al. Adipocyte hif2alpha functions as a thermostat via pka calpha regulation in beige adipocytes. Nat Commun (2022) 13(1):3268. doi: 10.1038/s41467-022-30925-0
34. Zhang Z, Jiang Y, Su L, Ludwig S, Zhang X, Tang M, et al. Obesity caused by an ovol2 mutation reveals dual roles of ovol2 in promoting thermogenesis and limiting white adipogenesis. Cell Metab (2022) 34(11):1860–74 e4. doi: 10.1016/j.cmet.2022.09.018
35. Cho YK, Yoon YC, Im H, Son Y, Kim M, Saha A, et al. Adipocyte lysoplasmalogenase tmem86a regulates plasmalogen homeostasis and protein kinase a-dependent energy metabolism. Nat Commun (2022) 13(1):4084. doi: 10.1038/s41467-022-31805-3
36. Park EJ, Kim HS, Lee DH, Kim SM, Yoon JS, Lee JM, et al. Ssu72 phosphatase is essential for thermogenic adaptation by regulating cytosolic translation. Nat Commun (2023) 14(1):1097. doi: 10.1038/s41467-023-36836-y
37. Wang Q, Li H, Tajima K, Verkerke ARP, Taxin ZH, Hou Z, et al. Post-translational control of beige fat biogenesis by prdm16 stabilization. Nature (2022) 609(7925):151–8. doi: 10.1038/s41586-022-05067-4
38. Balazova L, Balaz M, Horvath C, Horvath A, Moser C, Kovanicova Z, et al. Gpr180 is a component of tgfbeta signalling that promotes thermogenic adipocyte function and mediates the metabolic effects of the adipocyte-secreted factor cthrc1. Nat Commun (2021) 12(1):7144. doi: 10.1038/s41467-021-27442-x
39. Zhang X, Luo S, Wang M, Cao Q, Zhang Z, Huang Q, et al. Differential il18 signaling via il18 receptor and na-cl co-transporter discriminating thermogenesis and glucose metabolism regulation. Nat Commun (2022) 13(1):7582. doi: 10.1038/s41467-022-35256-8
40. Bean C, Audano M, Varanita T, Favaretto F, Medaglia M, Gerdol M, et al. The mitochondrial protein opa1 promotes adipocyte browning that is dependent on urea cycle metabolites. Nat Metab (2021) 3(12):1633–47. doi: 10.1038/s42255-021-00497-2
41. Xue K, Wu D, Wang Y, Zhao Y, Shen H, Yao J, et al. The mitochondrial calcium uniporter engages ucp1 to form a thermoporter that promotes thermogenesis. Cell Metab (2022) 34(9):1325–41.e6. doi: 10.1016/j.cmet.2022.07.011
42. Mills EL, Harmon C, Jedrychowski MP, Xiao H, Gruszczyk AV, Bradshaw GA, et al. Cysteine 253 of ucp1 regulates energy expenditure and sex-dependent adipose tissue inflammation. Cell Metab (2022) 34(1):140–57 e8. doi: 10.1016/j.cmet.2021.11.003
43. Jing X, Wu J, Dong C, Gao J, Seki T, Kim C, et al. Covid-19 instigates adipose browning and atrophy through vegf in small mammals. Nat Metab (2022) 4(12):1674–83. doi: 10.1038/s42255-022-00697-4
44. Jiang J, Zhou D, Zhang A, Yu W, Du L, Yuan H, et al. Thermogenic adipocyte-derived zinc promotes sympathetic innervation in male mice. Nat Metab (2023) 5(3):481–94. doi: 10.1038/s42255-023-00751-9
45. Junes-Gill KS, Gallaher TK, Gluzman-Poltorak Z, Miller JD, Wheeler CJ, Fan X, et al. Hhss1: A novel secreted factor and suppressor of glioma growth located at chromosome 19q13.33. J Neurooncol (2011) 102(2):197–211. doi: 10.1007/s11060-010-0314-6
46. O’Donnell JP, Phillips BP, Yagita Y, Juszkiewicz S, Wagner A, Malinverni D, et al. The architecture of emc reveals a path for membrane protein insertion. Elife (2020) 9. doi: 10.7554/eLife.57887
47. Wang X, Li Y, Qiang G, Wang K, Dai J, McCann M, et al. Secreted emc10 is upregulated in human obesity and its neutralizing antibody prevents diet-induced obesity in mice. Nat Commun (2022) 13(1):7323. doi: 10.1038/s41467-022-34259-9
48. Keith B, Johnson RS, Simon MC. Hif1alpha and hif2alpha: sibling rivalry in hypoxic tumour growth and progression. Nat Rev Cancer (2011) 12(1):9–22. doi: 10.1038/nrc3183
49. Kumar A, Bhandari A, Sinha R, Sardar P, Sushma M, Goyal P, et al. Molecular phylogeny of ovol genes illustrates a conserved C2h2 zinc finger domain coupled by hypervariable unstructured regions. PloS One (2012) 7(6):e39399. doi: 10.1371/journal.pone.0039399
50. Wu LC, Pfeiffer DR, Calhoon EA, Madiai F, Marcucci G, Liu S, et al. Purification, identification, and cloning of lysoplasmalogenase, the enzyme that catalyzes hydrolysis of the vinyl ether bond of lysoplasmalogen. J Biol Chem (2011) 286(28):24916–30. doi: 10.1074/jbc.M111.247163
51. Jurkowitz MS, Patel A, Wu LC, Krautwater A, Pfeiffer DR, Bell CE. The yhhn protein of legionella pneumophila is a lysoplasmalogenase. Biochim Biophys Acta (2015) 1848(2):742–51. doi: 10.1016/j.bbamem.2014.11.011
52. Krishnamurthy S, He X, Reyes-Reyes M, Moore C, Hampsey M. Ssu72 is an rna polymerase ii ctd phosphatase. Mol Cell (2004) 14(3):387–94. doi: 10.1016/s1097-2765(04)00235-7
53. Kim SH, Jeon Y, Kim HS, Lee JK, Lim HJ, Kang D, et al. Hepatocyte homeostasis for chromosome ploidization and liver function is regulated by ssu72 protein phosphatase. Hepatology (2016) 63(1):247–59. doi: 10.1002/hep.28281
54. Kim HS, Yoon JS, Jeon Y, Park EJ, Lee JK, Chen S, et al. Ssu72-hnf4alpha signaling axis classify the transition from steatohepatitis to hepatocellular carcinoma. Cell Death Differ (2022) 29(3):600–13. doi: 10.1038/s41418-021-00877-x
55. Ohno H, Shinoda K, Ohyama K, Sharp LZ, Kajimura S. Ehmt1 controls brown adipose cell fate and thermogenesis through the prdm16 complex. Nature (2013) 504(7478):163–7. doi: 10.1038/nature12652
56. Ohno H, Shinoda K, Spiegelman BM, Kajimura S. Ppargamma agonists induce a white-to-brown fat conversion through stabilization of prdm16 protein. Cell Metab (2012) 15(3):395–404. doi: 10.1016/j.cmet.2012.01.019
57. Pause A, Lee S, Worrell RA, Chen DY, Burgess WH, Linehan WM, et al. The von hippel-lindau tumor-suppressor gene product forms a stable complex with human cul-2, a member of the cdc53 family of proteins. Proc Natl Acad Sci U.S.A. (1997) 94(6):2156–61. doi: 10.1073/pnas.94.6.2156
58. Koren I, Timms RT, Kula T, Xu Q, Li MZ, Elledge SJ. The eukaryotic proteome is shaped by E3 ubiquitin ligases targeting C-terminal degrons. Cell (2018) 173(7):1622–35 e14. doi: 10.1016/j.cell.2018.04.028
59. Heldin CH, Moustakas A. Signaling receptors for tgf-beta family members. Cold Spring Harb Perspect Biol (2016) 8(8). doi: 10.1101/cshperspect.a022053
60. Esposito K, Nappo F, Marfella R, Giugliano G, Giugliano F, Ciotola M, et al. Inflammatory cytokine concentrations are acutely increased by hyperglycemia in humans: role of oxidative stress. Circulation (2002) 106(16):2067–72. doi: 10.1161/01.cir.0000034509.14906.ae
61. Netea MG, Joosten LA, Lewis E, Jensen DR, Voshol PJ, Kullberg BJ, et al. Deficiency of interleukin-18 in mice leads to hyperphagia, obesity and insulin resistance. Nat Med (2006) 12(6):650–6. doi: 10.1038/nm1415
62. Pazos P, Lima L, Tovar S, Gonzalez-Touceda D, Dieguez C, Garcia MC. Divergent responses to thermogenic stimuli in bat and subcutaneous adipose tissue from interleukin 18 and interleukin 18 receptor 1-deficient mice. Sci Rep (2015) 5:17977. doi: 10.1038/srep17977
63. Alavi MV, Fuhrmann N, Nguyen HP, Yu-Wai-Man P, Heiduschka P, Chinnery PF, et al. Subtle neurological and metabolic abnorMalities in an opa1 mouse model of autosomal dominant optic atrophy. Exp Neurol (2009) 220(2):404–9. doi: 10.1016/j.expneurol.2009.09.026
64. Abe Y, Rozqie R, Matsumura Y, Kawamura T, Nakaki R, Tsurutani Y, et al. Jmjd1a is a signal-sensing scaffold that regulates acute chromatin dynamics via swi/snf association for thermogenesis. Nat Commun (2015) 6:7052. doi: 10.1038/ncomms8052
65. Abe Y, Fujiwara Y, Takahashi H, Matsumura Y, Sawada T, Jiang S, et al. Histone demethylase jmjd1a coordinates acute and chronic adaptation to cold stress via thermogenic phospho-switch. Nat Commun (2018) 9(1):1566. doi: 10.1038/s41467-018-03868-8
66. Chouchani ET, Kazak L, Spiegelman BM. New advances in adaptive thermogenesis: ucp1 and beyond. Cell Metab (2019) 29(1):27–37. doi: 10.1016/j.cmet.2018.11.002
67. Chouchani ET, Kazak L, Jedrychowski MP, Lu GZ, Erickson BK, Szpyt J, et al. Mitochondrial ros regulate thermogenic energy expenditure and sulfenylation of ucp1. Nature (2016) 532(7597):112–6. doi: 10.1038/nature17399
68. Wang G, Meyer JG, Cai W, Softic S, Li ME, Verdin E, et al. Regulation of ucp1 and mitochondrial metabolism in brown adipose tissue by reversible succinylation. Mol Cell (2019) 74(4):844–57.e7. doi: 10.1016/j.molcel.2019.03.021
69. Maus M, Cuk M, Patel B, Lian J, Ouimet M, Kaufmann U, et al. Store-operated ca(2+) entry controls induction of lipolysis and the transcriptional reprogramming to lipid metabolism. Cell Metab (2017) 25(3):698–712. doi: 10.1016/j.cmet.2016.12.021
70. Ikeda K, Kang Q, Yoneshiro T, Camporez JP, Maki H, Homma M, et al. Ucp1-independent signaling involving serca2b-mediated calcium cycling regulates beige fat thermogenesis and systemic glucose homeostasis. Nat Med (2017) 23(12):1454–65. doi: 10.1038/nm.4429
71. De Stefani D, Rizzuto R, Pozzan T. Enjoy the trip: calcium in mitochondria back and forth. Annu Rev Biochem (2016) 85:161–92. doi: 10.1146/annurev-biochem-060614-034216
72. Kazak L, Chouchani ET, Stavrovskaya IG, Lu GZ, Jedrychowski MP, Egan DF, et al. Ucp1 deficiency causes brown fat respiratory chain depletion and sensitizes mitochondria to calcium overload-induced dysfunction. Proc Natl Acad Sci U.S.A. (2017) 114(30):7981–6. doi: 10.1073/pnas.1705406114
73. Rodriguez-Cuenca S, Cocheme HM, Logan A, Abakumova I, Prime TA, Rose C, et al. Consequences of long-term oral administration of the mitochondria-targeted antioxidant mitoq to wild-type mice. Free Radic Biol Med (2010) 48(1):161–72. doi: 10.1016/j.freeradbiomed.2009.10.039
74. Makino Y, Cao R, Svensson K, Bertilsson G, Asman M, Tanaka H, et al. Inhibitory pas domain protein is a negative regulator of hypoxia-inducible gene expression. Nature (2001) 414(6863):550–4. doi: 10.1038/35107085
75. Xue Y, Petrovic N, Cao R, Larsson O, Lim S, Chen S, et al. Hypoxia-independent angiogenesis in adipose tissues during cold acclimation. Cell Metab (2009) 9(1):99–109. doi: 10.1016/j.cmet.2008.11.009
76. Cao Y. Adipose tissue angiogenesis as a therapeutic target for obesity and metabolic diseases. Nat Rev Drug Discovery (2010) 9(2):107–15. doi: 10.1038/nrd3055
77. Seki T, Hosaka K, Fischer C, Lim S, Andersson P, Abe M, et al. Ablation of endothelial vegfr1 improves metabolic dysfunction by inducing adipose tissue browning. J Exp Med (2018) 215(2):611–26. doi: 10.1084/jem.20171012
78. Rios-Lugo MJ, Madrigal-Arellano C, Gaytan-Hernandez D, Hernandez-Mendoza H, Romero-Guzman ET. Association of serum zinc levels in overweight and obesity. Biol Trace Elem Res (2020) 198(1):51–7. doi: 10.1007/s12011-020-02060-8
79. Torkanlou K, Bibak B, Abbaspour A, Abdi H, Saleh Moghaddam M, Tayefi M, et al. Reduced serum levels of zinc and superoxide dismutase in obese individuals. Ann Nutr Metab (2016) 69(3-4):232–6. doi: 10.1159/000454894
80. Thoen RU, Barther NN, Schemitt E, Bona S, Fernandes S, Coral G, et al. Zinc supplementation reduces diet-induced obesity and improves insulin sensitivity in rats. Appl Physiol Nutr Metab (2019) 44(6):580–6. doi: 10.1139/apnm-2018-0519
81. Chi J, Cohen P. The multifaceted roles of prdm16: adipose biology and beyond. Trends Endocrinol Metab (2016) 27(1):11–23. doi: 10.1016/j.tem.2015.11.005
82. Costa-Mattioli M, Walter P. The integrated stress response: from mechanism to disease. Science (2020) 368(6489). doi: 10.1126/science.aat5314
83. Mota de Sa P, Richard AJ, Hang H, Stephens JM. Transcriptional regulation of adipogenesis. Compr Physiol (2017) 7(2):635–74. doi: 10.1002/cphy.c160022
84. Ouellet V, Labbe SM, Blondin DP, Phoenix S, Guerin B, Haman F, et al. Brown adipose tissue oxidative metabolism contributes to energy expenditure during acute cold exposure in humans. J Clin Invest (2012) 122(2):545–52. doi: 10.1172/JCI60433
85. UD M, Raiko J, Saari T, Kudomi N, Tolvanen T, Oikonen V, et al. Human brown adipose tissue [(15)O]O2 pet imaging in the presence and absence of cold stimulus. Eur J Nucl Med Mol Imaging (2016) 43(10):1878–86. doi: 10.1007/s00259-016-3364-y
86. Baskin AS, Linderman JD, Brychta RJ, McGehee S, Anflick-Chames E, Cero C, et al. Regulation of human adipose tissue activation, gallbladder size, and bile acid metabolism by a beta3-adrenergic receptor agonist. Diabetes (2018) 67(10):2113–25. doi: 10.2337/db18-0462
87. O’Mara AE, Johnson JW, Linderman JD, Brychta RJ, McGehee S, Fletcher LA, et al. Chronic mirabegron treatment increases human brown fat, hdl cholesterol, and insulin sensitivity. J Clin Invest (2020) 130(5):2209–19. doi: 10.1172/JCI131126
88. Wibmer AG, Becher T, Eljalby M, Crane A, Andrieu PC, Jiang CS, et al. Brown adipose tissue is associated with healthier body fat distribution and metabolic benefits independent of regional adiposity. Cell Rep Med (2021) 2(7):100332. doi: 10.1016/j.xcrm.2021.100332
89. Becher T, Palanisamy S, Kramer DJ, Eljalby M, Marx SJ, Wibmer AG, et al. Brown adipose tissue is associated with cardiometabolic health. Nat Med (2021) 27(1):58–65. doi: 10.1038/s41591-020-1126-7
90. Pan R, Chen Y. Latest advancements on combating obesity by targeting human brown/beige adipose tissues. Front Endocrinol (Lausanne) (2022) 13:884944. doi: 10.3389/fendo.2022.884944
Keywords: obesity, adipocyte thermogenesis, adrenergic signaling pathway, PKA, UCP1
Citation: Nie T, Lu J, Zhang H and Mao L (2023) Latest advances in the regulatory genes of adipocyte thermogenesis. Front. Endocrinol. 14:1250487. doi: 10.3389/fendo.2023.1250487
Received: 30 June 2023; Accepted: 07 August 2023;
Published: 23 August 2023.
Edited by:
Claire Joanne Stocker, Aston University, United KingdomReviewed by:
Antonia Lanni, University of Campania Luigi Vanvitelli, ItalyCopyright © 2023 Nie, Lu, Zhang and Mao. This is an open-access article distributed under the terms of the Creative Commons Attribution License (CC BY). The use, distribution or reproduction in other forums is permitted, provided the original author(s) and the copyright owner(s) are credited and that the original publication in this journal is cited, in accordance with accepted academic practice. No use, distribution or reproduction is permitted which does not comply with these terms.
*Correspondence: Tao Nie, bmllX3Rhb0BoYnVhcy5lZHUuY24=; Hua Zhang, NjY0Nzk3MDQ3QHFxLmNvbQ==; Liufeng Mao, bWxmXzkyOTVAMTI2LmNvbQ==
Disclaimer: All claims expressed in this article are solely those of the authors and do not necessarily represent those of their affiliated organizations, or those of the publisher, the editors and the reviewers. Any product that may be evaluated in this article or claim that may be made by its manufacturer is not guaranteed or endorsed by the publisher.
Research integrity at Frontiers
Learn more about the work of our research integrity team to safeguard the quality of each article we publish.