- 1Centro de Investigación Mariña, Laboratorio de Fisioloxía Animal, Departamento de Bioloxía Funcional e Ciencias da Saúde, Facultade de Bioloxía, Universidade de Vigo, Vigo, Spain
- 2Departamento de Fisiología de Peces y Biotecnología, Instituto de Acuicultura Torre de la Sal, Instituto de Acuicultura Torre de la Sal - Consejo Superior de Investigaciones Científicas (IATS-CSIC), Castellón, Spain
- 3Departamento de Fisiología, Facultad de Biología, Universidad de Santiago de Compostela, Santiago de Compostela, Spain
This study explored changes in brain serotonin content and activity together with hypothalamic neuropeptide mRNA abundance around feeding time in rainbow trout, as well as the effect of one-day fasting. Groups of trout fed at two (ZT2) and six (ZT6) hours after lights on were sampled from 90 minutes before to 240 minutes after feeding, while additional groups of non-fed trout were also included in the study. Changes in brain amine and metabolite contents were measured in hindbrain, diencephalon and telencephalon, while in the diencephalon the mRNA abundance of tryptophan hydroxylase (tph1, tph2), serotonin receptors (5htr1a, 5htr1b and 5htr2c) and several neuropeptides (npy, agrp1, cartpt, pomca1, crfb) involved in the control of food intake were also assessed. The results showed changes in the hypothalamic neuropeptides that were consistent with the expected role for each in the regulation of food intake in rainbow trout. Serotonergic activity increased rapidly at the time of food intake in the diencephalon and hindbrain and remained high for much of the postprandial period. This increase in serotonin abundance was concomitant with elevated levels of pomca1 mRNA in the diencephalon, suggesting that serotonin might act on brain neuropeptides to promote a satiety profile. Furthermore, serotonin synthesis and neuronal activity appear to increase already before the time of feeding, suggesting additional functions for this amine before and during food intake. Exploration of serotonin receptors in the diencephalon revealed only small changes for gene expression of 5htr1b and 5htr2c receptors during the postprandial phase. Therefore, the results suggest that serotonin may play a relevant role in the regulation of feeding behavior in rainbow trout during periprandial time, but a better understanding of its interaction with brain centers involved in receiving and processing food-related signals is still needed.
1 Introduction
Feeding behavior involves the coordination of multiple complex neural circuits that allow the animal to obtain food at the appropriate times and regulate the amount it eats. In vertebrates, sensory information related to feeding status, whether from olfactory and/or gustatory receptors, gastrointestinal digestive processes, circulating nutrient levels or the individual’s own behavior, are integrated at the central nervous system and activates stimulatory/inhibitory outputs that control food intake (1–3). Two populations of neuropeptidergic neurons of the hypothalamus are involved in the control of food intake in mammals, the first expressing the orexigenic neuropeptide Y (NPY) and agouti-related peptide (AgRP), and the second expressing the anorexigenic proopiomelacortin (POMC) and cocaine- and amphetamine-regulated transcript (CART) (4, 5). In fish, the existence of homologous neuronal populations is still under debate, but orexigenic and anorexigenic effects of neuropeptides have been reported for several fish species (see rev. 6, 7). In addition, circadian signals also modulate feeding behavior by acting through different peripheral and central regulatory pathways in order to accommodate food intake to certain times of the day that are more conducive from the point of view of digestive activity, energy homeostasis and adaptation to the environment (6, 8). Accordingly, fish subjected to a programmed feeding regime periodically increase or decrease the abundance of factors that regulate appetite and satiety, respectively, with these changes coinciding mostly with the preprandial and postprandial phases (3).
In addition to neuropeptides, several brain neurotransmitters are involved in feeding regulation, including the monoamines (9, 10). Serotonin (5-hydroxytryptamine, 5HT) is widely distributed in central nervous system and is one of the most important neurotransmitters affecting the regulation of food intake (11). 5HT synthesis takes place from the amino acid L-tryptophan, which is first hydroxylated by tryptophan hydroxylase (TPH, EC 1.14.16.4) to form 5-hydroxytryptophan (5HTP), and then decarboxylated by L-aromatic acid decarboxylase (AADC, 4.1.1.28) to give serotonin. Due to the rapid activity of AADC, the levels of 5HTP are usually low, and TPH is thought to act as the rate-limiting enzyme of 5HT biosynthesis (12). Once the serotonergic neuron is activated, the amine stored in vesicles in neuronal terminals is released exocytotically to the synaptic cleft, where it binds to specific 5HT receptors (5HTR), either auto or heteroreceptors (13). Subsequently, sodium-dependent serotonin transporters (SERT or slc6a4) mediate the reuptake of 5HT to neuronal endings, where it is metabolized to 5-hydroxyindoleacetic acid (5HIAA) by the sequential action of monoamine oxidase and aldehyde dehydrogenase (14). 5HT synthesis, reuptake and degradation are well-conserved processes through phylogeny, so that 5HT is one of the most widespread signaling molecules in vertebrates, including fish (15).
Evidence in mammals suggests that the central 5HTergic system acts as a suppressor of food anticipatory activity (FAA) and as a potent satiety signal (16). Multiple studies have focused on 5HTR subtypes involved in feeding regulation, pointing to a priority role of the postsynaptic 5HT2C and 5HT1B receptors and probably the presynaptic 5HT1A receptor (17–19). 5HT2C receptor is widely expressed in the CNS and specifically in brain areas related to energy metabolism, including the ventral tegmental area (VTA), the arcuate nucleus (ARC) and the paraventricular nucleus of the hypothalamus (PVN), among others (20). Of utmost interest is that 5HT2C receptor is co-expressed in hypothalamic (ARC) POMC neurons, which are first-order neurons in the regulation of food intake and energy balance (21). ARC POMC neurons produce melanocyte-stimulating hormone (αMSH), an endogenous agonist of the melanocortin-4 receptor (MC4R) whose activation promotes downstream effects that inhibit food intake (22–24). Additionally, activation of 5HT1B receptors downregulates the firing rate in hypothalamic NPY/AgRP neurons and collaterally increases neuronal release of POMC, which also leads to loss of appetite (10, 21). The presynaptic autoreceptor 5HT1A inhibits serotonergic activity and may led to a down-regulation of the 5HT-mediated appetite inhibition (25).
In fish, monoamine systems are widely spread in brain with major clusters of 5HTergic neurons lying in the diencephalon (26), including the hypothalamic area where the control center for food intake is located (27). Pharmacological studies, i.e., by using 5HT or drugs that modulate serotonergic transmission such as fluoxetine and d-fenfluramine administered peripherally or intracerebroventricularly, showed an inhibitory influence of 5HT on food intake (28–30) and weight gain (31). In addition, treatments with serotonergic agonists or antagonists showed a close functional relationship between brain 5HT and changes in the expression of neuropeptides involved in the hypothalamic control of feeding. However, the specific mechanisms are not entirely clear in fish. For instance, the anorectic effect induced by increased 5HT in goldfish brain may be mediated by CRF (28), whereas fluoxetine up-regulated both crf1 and npy gene expression in female goldfish (31). In trout, the decrease in food intake induced by agonistic activation of 5HT2C receptors was followed by an increase in hypothalamic mRNA abundance of pomca1, cart and crf (32–34). Meanwhile, the involvement of 5HT1B receptors in feeding control remains unclear (30).
In teleosts, hypothalamic neuropeptide levels show changes that respond to a number of metabolic, humoral and neural signals that provide information about food intake, nutritional status and circadian timing (35–37). Some of these signals can converge on brain serotonergic neurons at different levels to modulate their activity during feeding time (27, 29, 32). Therefore, 5HT may be a critical element in the interaction with brain neuropeptide circuits, being able to modulate feeding behavior. However, whether there is a temporal relationship between brain 5HTergic activity and the regulatory mechanisms determining daily food intake in teleost remains to be determined. To address this question, the present study addressed in depth the periprandial changes in serotonergic activity and its relationship to feeding regulatory neuropeptides in the brain of fed and non-fed rainbow trout. Since schedule feeding can adjust circadian changes in feeding regulatory systems (38), we assessed changes in 5HT and brain neuropeptides in groups of fish fed on two different daily feeding schedules.
2 Materials and methods
2.1 Fish
Immature rainbow trout (Oncorhynchus mykiss, Walbaum) weighing 92.4 ± 6.4 g, were obtained from a local hatchery (A Estrada, Spain), and transported to the facilities of the Faculty of Biology (University of Vigo). Fish were transferred to 100-L water tanks and began a 20-day acclimation period to laboratory conditions, i.e., 12L:12D light/dark photoperiod, controlled water temperature (13 ± 1°C) and continuously renewed, aerated and dechlorinated fresh water. Trout were fed daily at ZT2 (ZT0 = lights on) with commercial dry pellets (Dibaq-Diproteg SA, Spain; 48% crude protein, 14% carbohydrates, 25% crude fat, and 11.5% ash; 20.2 MJ/kg feed). All the experiments agreed with the European Union Council (2010/63/UE) and Spanish Government (RD 53/2013) Directives for the use of animals in research. The Animal Ethics Committee of the University of Vigo approved all the protocols.
2.2 Experimental design and sampling
Following acclimation, two cohorts of fish were randomly distributed into twenty experimental tanks (10 tanks per cohort, 25 individuals per tank), and were fed ad libitum for 10 days. Fish belonging to the first cohort received food 2 hours after lights on (zeitgeber time 2, ZT2), whereas those of the second cohort were fed at ZT6. This feeding protocol was applied to discriminate the possible influence of circadian fluctuations of the measured parameters on the changes occurring in relation to the daily feeding time. Each day the fish had visual access to the feeding operator for 5 seconds before the food was delivered. After the training period, trout from six tanks in each cohort were sampled at different times around the respective mealtime (MT): 90 min and 30 min before MT, just at MT (0 min), and 30, 90 and 240 min after MT. To ensure that all the fish of these groups had eaten food, stomach content was analyzed individually at the end of the experiment. The remaining four tanks from each cohort were not fed although a simulated feeding action was performed at mealtime. Trout of the unfed groups were subsequently sacrificed and sampled at 0, 30, 90 and 240 min, relative to the corresponding MT.
Prior to sampling, fish were anesthetized with 0.02% 2-phenoxyethanol (v/v, Merck) pre-diluted in 1 L of water and carefully added to the tank. Only 16 fish from each tank were used, with the remaining 9 fish being transferred to recovery tanks. This surplus of animals per experimental tank was established to avoid the incidence of dominance hierarchies, as previously reported (39). Blood samples were collected from the caudal peduncle by puncture with heparinized syringes. Plasma was obtained after blood centrifugation (6,000 g, 10 min at 4° C) and an aliquot of 50 µL was collected and immediately stored at -80° C for cortisol analysis. The remaining plasma volume was deproteinized with 0.6 M perchloric acid followed by centrifugation (14,000 g, 4 min at 4° C) and subsequent neutralization with 1 M potassium bicarbonate, thus frozen (-80° C) for subsequent biochemical determinations. Finally, fish were euthanized by decapitation and brain areas (telencephalon, diencephalon - including the hypothalamus - and hindbrain) were dissected as shown in Supplementary Figure 1. Tissues were frozen at -80°C until further analysis. Brain samples from ten fish were analyzed for monoamine content, while those from the remaining six fish were used for q-PCR assays.
2.3 Plasma cortisol and metabolites
Plasma cortisol levels were analyzed by a commercial enzyme-linked immunosorbent assay kit (Cayman Chemical Company, Ann Arbor, MI, USA), following the manufacturer’s indications. Plasma glucose levels were measured spectrophotometrically with a commercial kit (Spinreact, Barcelona, Spain).
2.4 Brain monoamines
The contents of 5HT and its main oxidative metabolite, the 5HIAA, in brain tissues were assessed by high performance liquid chromatography with electrochemical detection (HPLC-EC) according to Gesto et al. (40), with modifications. Brain tissues were homogenized by ultrasonic disruption in 0.4 mL of HPLC mobile phase and centrifuged (16,000 g, 10 min). Protein content in individual aliquots were estimated by the bicinchoninic acid method, and the remaining volume was filtered (0.2 µm, Millipore) and diluted 1:3 in mobile phase before injected into the HPLC system. This consisted of a Jasco PU-2080 Plus pump and AS-2057 autosampler, a 5-μm reverse phase analytical column (Kinetex C18 100 Å, 150 mm length × 4.6 mm diameter; Phenomenex) maintained at 25° C in a column oven (Jasco CO-4060), and an ESA Coulochem II detector. The detection system included a double analytical cell (M5011) with oxidation potentials set at +40 mV (first electrode) and +340 mV (second electrode). The mobile phase was composed of 63.9 mol/L NaH2PO4, 0.1 mol/L Na2EDTA, 0.80 mol/L sodium 1-octanesulfonate and 14% v/v methanol, and pH adjusted to 2.85 with o-phosphoric acid. Mobile phase was filtered, degassed under vacuum and pumped at a flow rate of 1.0 mL/min. The detection limits for 5HT and 5HIAA were 0.5 and 1.5 pg per injection, with a signal-to-noise ratio of 3. ChromNAV version 1.12 software (Jasco Corp.) was used for chromatogram acquisition and data integration.
2.5 Quantitative real time PCR (qPCR) assays
Total mRNA was individually extracted from brain samples according to the TRIzol® method (Life Technologies, Grand Island, NY, USA), and treated with DNAse (Promega, Madison, WI, USA). 1 μg total RNA was converted to cDNA with Superscript II reverse transcriptase (Promega) and random hexaprimers (Promega). To identify any genomic contamination in the RNA extract, negative control without reverse transcriptase was run for each sample.
The mRNA abundance was determined by real-time quantitative RT-PCR (q-PCR) using the iCycler iQ™ (BIO-RAD, Hercules, CA, USA). Assays were done in a total PCR volume of 15 μL, containing 2 μL of diluted cDNA (1:8), 7.5 μL of MAXIMA SYBR Green qPCR Mastermix (Life Technologies), 1.0 μL of each primer (0,2 µM final concentration), and 3.5 µL of RNAse-free MilliQ water. Primers were selected from sequences previously reported for rainbow trout and were obtained from Sigma (Table 1). The transcripts assayed were tph1, tph2, 5htr2c, 5htr1b, 5htr1a, npy, agrp1, pomca1, cartpt, crfb, and actb. The latter was used as a reference for relative quantification after it was found to show stable expression levels in tissues under the experimental conditions. Thermal cycling initiated by incubation at 95°C for 10 min using hot-start iTaq DNA polymerase activation; then, 35 qPCR steps were performed, each consisting of heating at 95°C for 15 s, 30 s at Tm-5° C and 30 s at 72°C. After the last PCR cycle, melting curves were systematically monitored (temperature gradient at 0.5°C/15s from Tm-5 to 94°C) to ensure that only one fragment from each sample was amplified. Samples lacking cDNA were run in parallel, as negative controls, to ensure primers accuracy. The efficiency of all transcripts was calculated and only values between 90% and 110% were accepted (R2 value was always higher than 0.985). The relative expression of the target genes was calculated following the Pfaffl method (41).
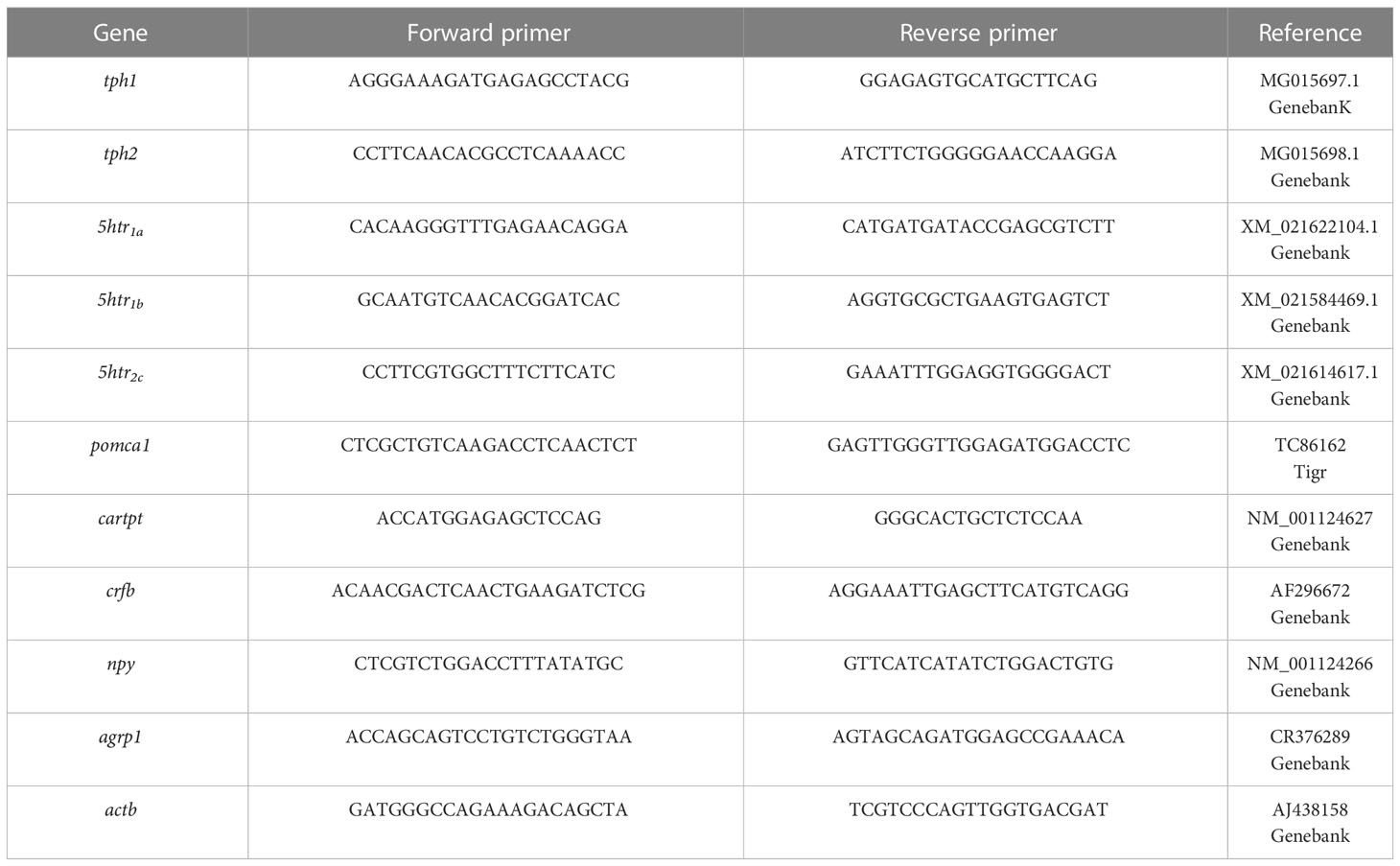
Table 1 Nucleotide sequences of the PCR primers used to evaluate mRNA abundance of transcript by RT-qPCR.
2.6 Statistical analysis
All data sets are shown as the mean ± standard error of the mean (SEM) and a significance level (α) of 0.05 was generally used. One-way ANOVA analysis was performed to assess differences among periprandial times for each parameter assayed within each cohort, ZT2 and ZT6. Post-prandial data (from 0 min to 240 min after MT) were analyzed by two-way ANOVA, which included feeding condition (fed/unfed) and time as main factors. When required, Tukey’s HSD was used for post hoc analysis. Statistical analyses were carried out with GraphPad Prism (version 9.0; GraphPad Software, San Diego, CA).
3 Results
Statistical results of the periprandial evolution of the different parameters in normally fed fish (one-way ANOVA), as well as comparisons between fed and non-fed during the postprandial time are included as supplementary material (Supplementary Tables 1, 2).
3.1 Plasma cortisol and glucose levels
Plasma cortisol and glucose levels are shown in Figure 1. Fish fed in the morning (ZT2) displayed elevated preprandial values, which gradually decreased until 240 min after MT. In contrast, cortisol levels in fish that were fed in the afternoon (ZT6; cohort 2) showed a significant increase at MT and decreased thereafter. Non-fed trout had higher cortisol levels that fed trout in both the ZT2 and ZT6 cohorts (+240 min: p<0.05 vs fed group). Plasma glucose levels in ZT2-fed trout initially decreased (-30 min before MT) and then transiently increased at +90 min. Those fed at ZT6 showed an increase in glucose levels at +30 min and +90 min, declining to basal levels at +240 min. Glucose levels in the unfed fish did not vary across the periprandial times.
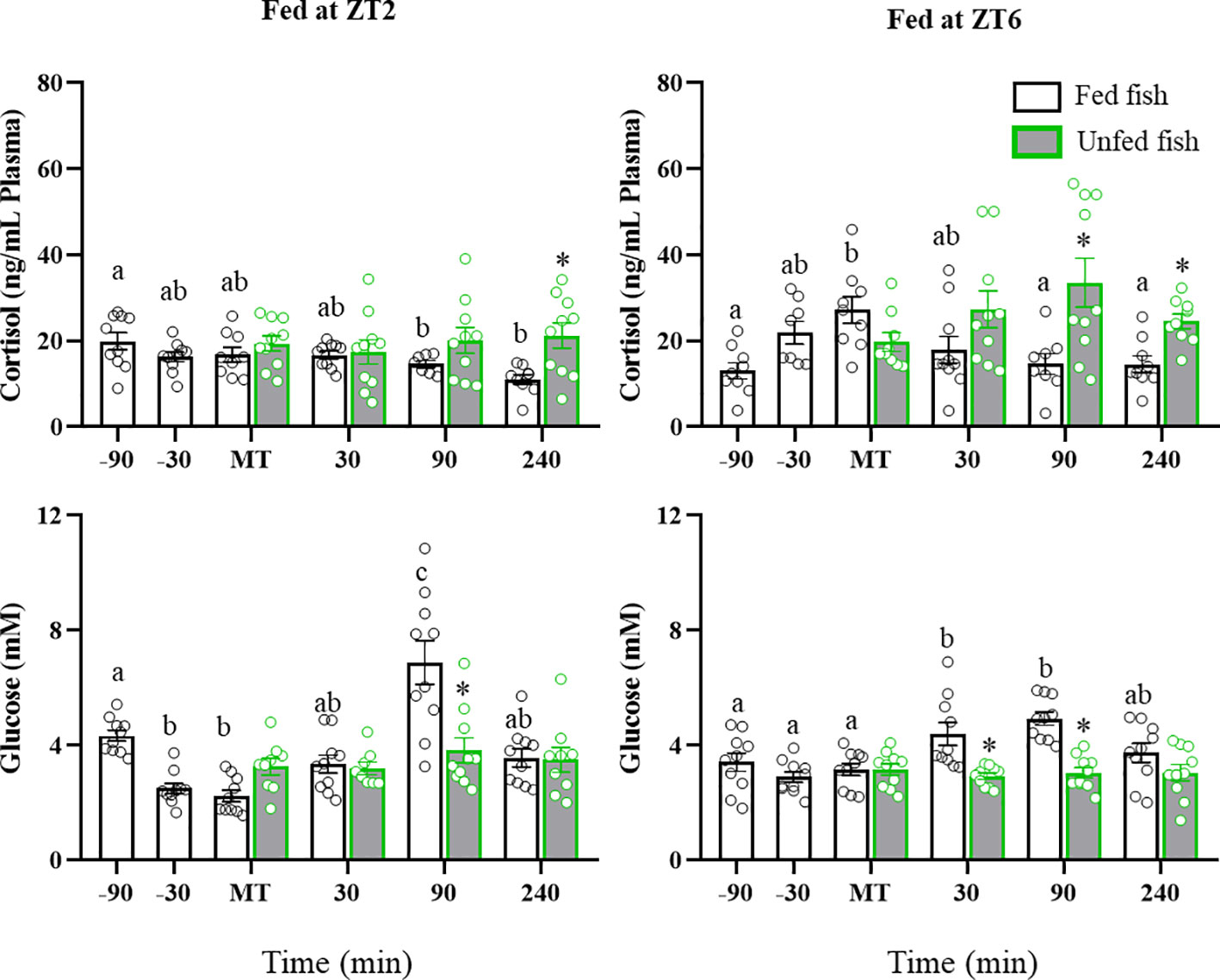
Figure 1 Periprandial changes and effect of fasting in plasma cortisol and glucose levels of rainbow trout normally fed at two different daily times (morning, ZT2, and afternoon, ZT6). The results are shown as the mean ± SEM of n=10 fish per group. Different letters indicate significant differences among times in the fed fish group. Asterisks indicate significant differences between non-fed and fed fish at each specific time (P<0.05).
3.2 mRNA abundance of food intake-related neuropeptides in diencephalon
Figure 2 shows the mRNA abundance of npy, agrp1, pomca1, cartpt and crfb in trout diencephalon. The preprandial time significantly affected npy and agrp1 mRNA abundance in ZT2-fed fish, showing a continuous drop from -90 min to MT. However, trout fed at ZT6 showed no preprandial changes in both peptides. After feeding, npy and agrp1 levels sharply decreased in both cohorts of fish, remaining low thereafter. In the non-fed trout, this effect was not observed initially, with its levels being higher at 30 min (npy and agrp1) and 90 min (npy) after MT compared to the respective fed groups. After 90 min (agrp1) and 240 min (npy) from feeding, transcript levels were similar in the fed and unfed groups. As for pomca1, a pre-MT decrease was observed in the ZT2 group (-30 min), followed in both the ZT2 and ZT6 groups by an increase that was maintained for 90 min after MT. The non-fed trout group showed no such an increase in pomca1, with values remaining stable throughout sampling. No significant periprandial changes for cartpt were found in both ZT2 and ZT6 fed fish, but an increase in non-fed fish (ZT2: +90 min; ZT6: +30 min; p<0.05 vs. respective fed groups).
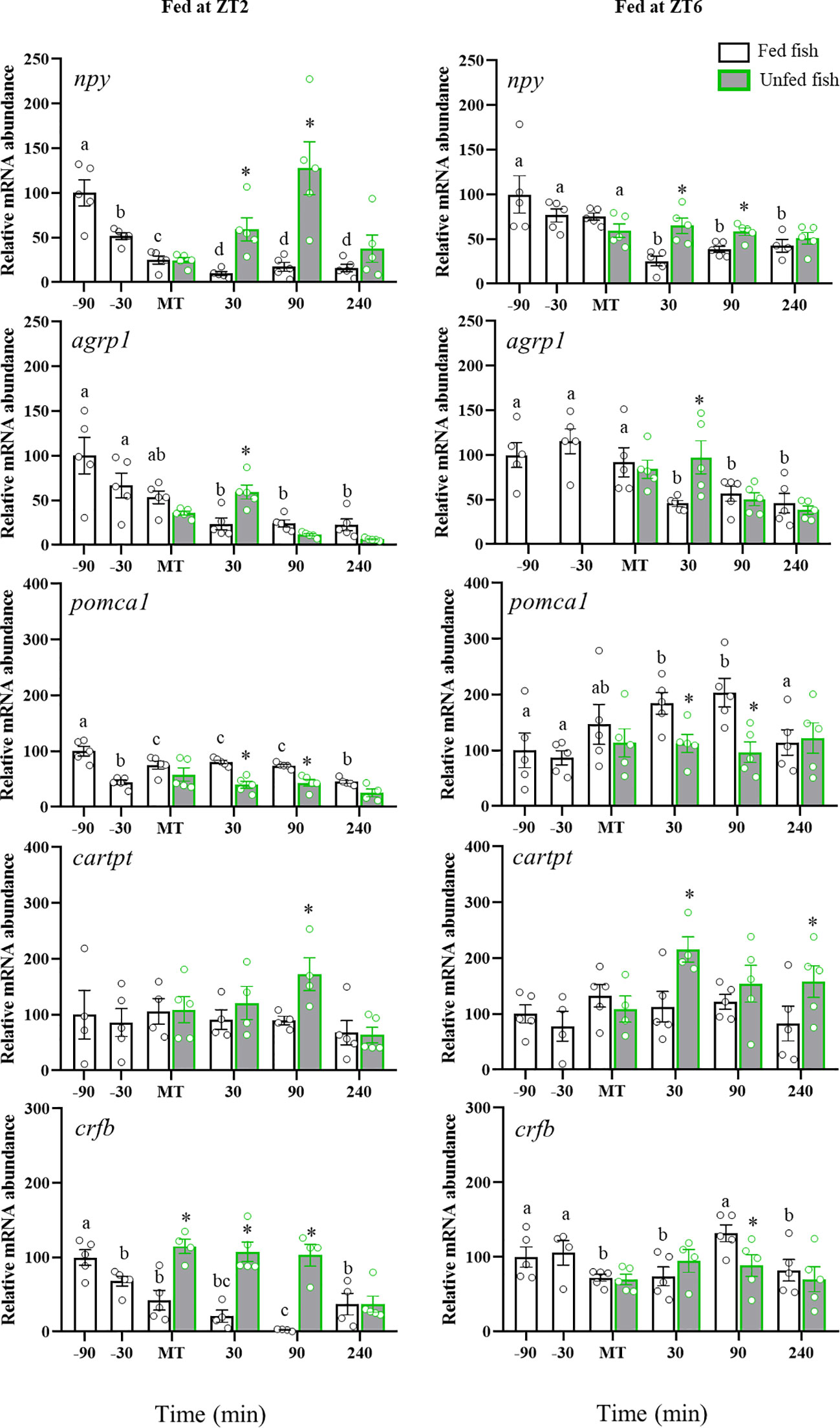
Figure 2 Periprandial changes and effect of fasting in mRNA abundance of regulatory neuropeptides in the diencephalon of rainbow trout normally fed at two different daily times (morning, ZT2, and afternoon, ZT6). The results are shown as the mean ± SEM of n=5 fish per group. Different letters indicate significant differences among times in the fed fish group. Asterisks indicate significant differences between non-fed and fed fish at each specific time (P<0.05).
The abundance of crfb in diencephalon showed a decreasing trend throughout most of the sampling time in ZT2-fed fish, which was most evident after the mealtime. In fish fed at ZT6, the post-feeding decline in crfb content was also noted, but more attenuated. Non-fed fish showed higher crfb levels from usual MT to +90 min as compared to fish fed at ZT2, whereas no differences were found between non-fed and ZT6-fed fish.
3.3 Periprandial changes in serotonergic activity and receptors
Figure 3 shows the mRNA abundance of tph1 and tph2 in the diencephalon and hindbrain of rainbow trout fed in ZT2 and ZT6, or in the respective sham-feeding groups. Fish from both cohorts showed significant time-dependent changes in diencephalic expression of tph1 with a sustained significant increase from 30 min before MT. Non-fed fish showed differential effects as a function of daily time. Thus, tph1 mRNA abundance was not affected in non-fed fish from the first cohort (ZT2), while those from the second cohort (ZT6) showed a significant decrease at 90 min after MT compared to the fed group (p=0.028).
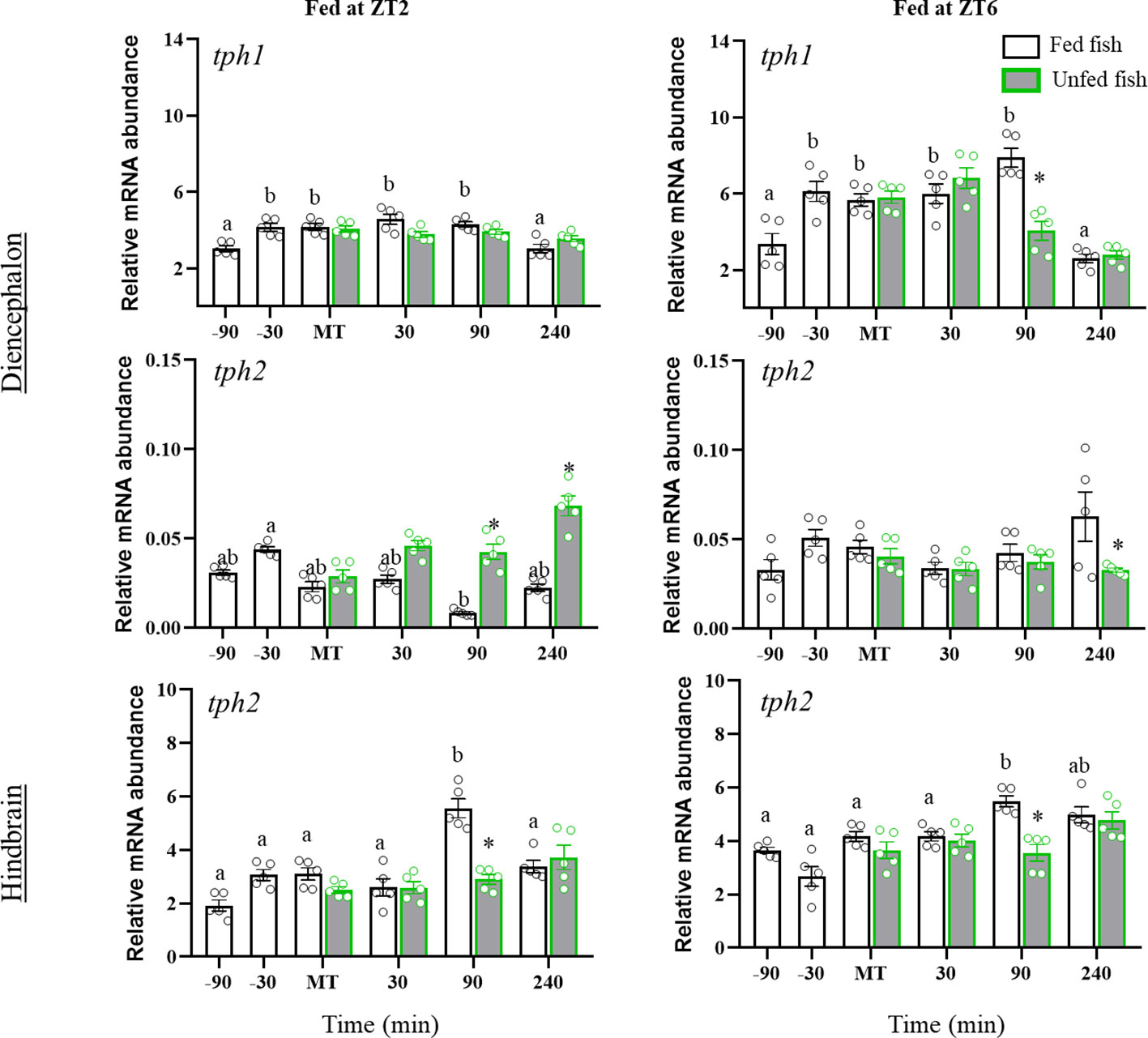
Figure 3 Periprandial changes in mRNA abundance of tryptophan hydroxylase 1 and 2 in diencephalon and tryptophan hydroxylase 2 in hindbrain of rainbow trout with two different feeding schedules, morning (ZT2) and afternoon (ZT6). The results are shown as the mean ± SEM of n=5 fish per group. Different letters indicate significant differences among times in the fed fish group. Asterisks indicate significant differences between non-fed and fed fish at each specific time (P<0.05).
Assessment of diencephalic tph2 mRNA abundance revealed minor periprandial changes, with significantly low tph2 mRNA levels at 90 min post-feeding in ZT2 fish, which increased in non-fed fish (from 90 to 240 min post-MT, p<0.05 vs. fed fish). Normally fed ZT6 cohort trout showed no significant periprandial fluctuations, whereas non-fed trout showed a decrease at 240 min post-MT. In the hindbrain, mean tph2 expression was significantly higher during the postprandial than the pre-prandial period in both ZT2 (P=0.010) and ZT6 (P<0.001) fish. The tph2 mRNA content increased transiently at 90 min post-MT in both cohorts, relative to pre-prandial and prandial values, followed by a decline to basal levels (+240 min). The postprandial increase in tph2 mRNA levels was not observed in non-fed fish of both cohorts (ZT2 and ZT6), so it was significantly lower at +90 min than in fed fish.
Figure 4 shows the tissue contents of 5HT and 5HIAA, as well as the 5HIAA/5HT ratio in the diencephalon. 5HT content increased significantly after feeding in fish from both cohorts (ZT2: p=0.005; ZT6: p<0.001 vs pre-prandial time), whereas no postprandial changes were detected in non-fed trout. Accordingly, 5HT levels were significantly lower at 30, 90 and 240 min (ZT2) or 90 min (ZT6) after MT in non-fed versus fed trout (ZT2: p<0.001; ZT6: p=0.007). Diencephalon 5HIAA content also increased after feeding time in both cohorts, reaching significance at 30 and 90 min after MT compared to previous values. Non-fed fish in the ZT2 cohort showed the same 5HIAA content profile as fed fish, whereas in the ZT6 cohort the levels of this metabolite were less in non-fed fish than in fed fish at 30 and 90 min after MT. As for the 5HIAA/5HT ratio, increased values were found 30 min before MT (ZT2) and at MT (ZT2 and ZT6), compared to previous sampling points, remaining elevated for at least 30 min in the postprandial period. Trout in the first cohort (ZT2) that were not fed showed lower values of the 5HIAA/5HT ratio at the MT than those fed, with these levels gradually increasing over the next 240 min. A similar effect was not observed in fish from the second cohort (ZT6).
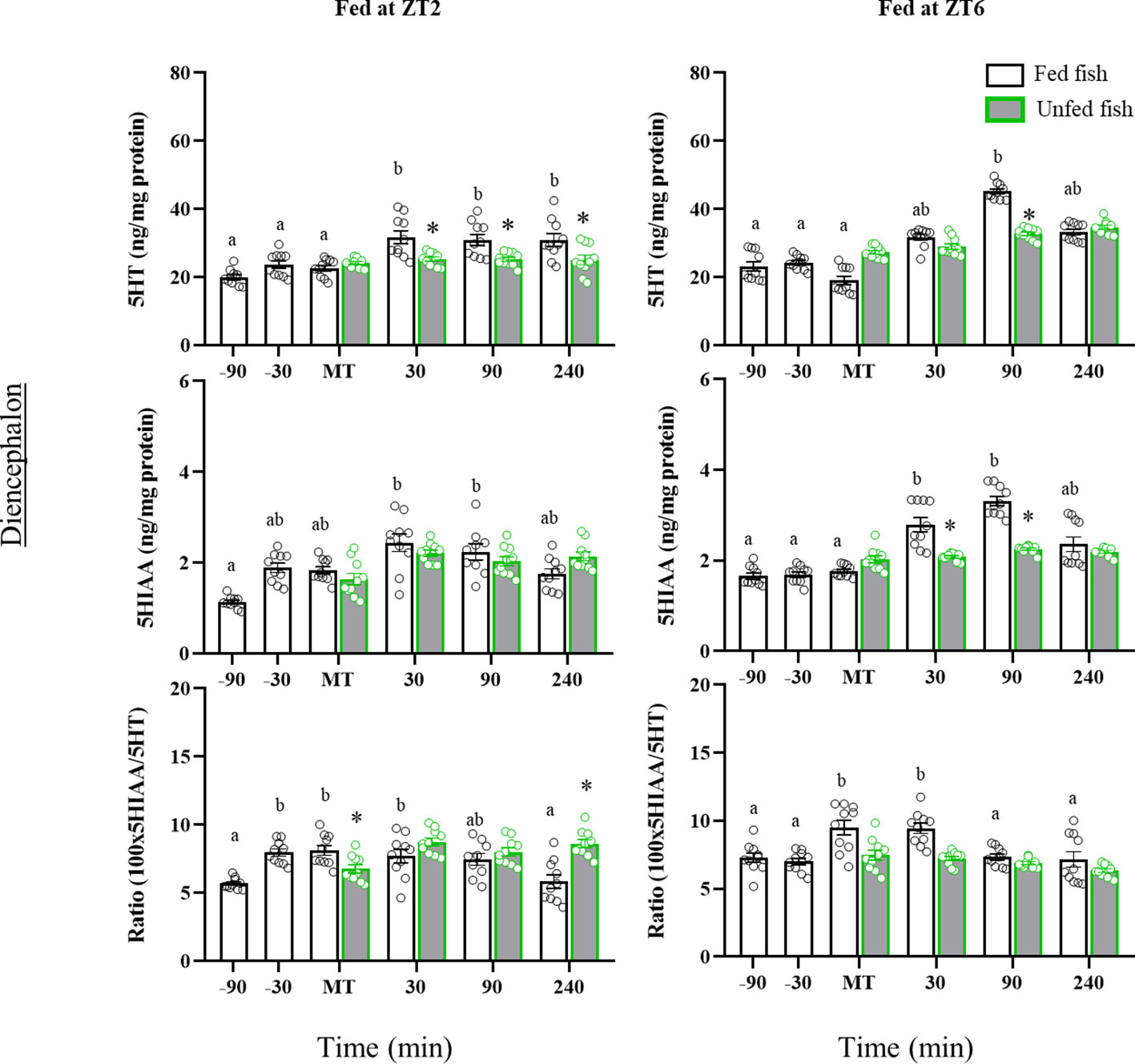
Figure 4 Periprandial changes and effects of fasting in the levels of serotonin (5HT) and 5-hydroxyindoleacetic acid (5HIAA), and the 5HIAA/5HT ratio in the diencephalon of rainbow trout with two different feeding schedules, morning (ZT2) and afternoon (ZT6). The results are shown as the mean ± SEM of n=10 fish per group. Different letters indicate significant differences among times in the fed fish group. Asterisk indicates significant differences between non-fed and fed fish at each specific time (P<0.05).
In the hindbrain (Figure 5), the 5HT content of the morning-fed fish (ZT2) increased in the postprandial period (30-, 90- and 240 min post-MT vs pre-prandial values, p=0.010). A post-prandial increase was also observed in the ZT6 group of fish (p=0.030) at 90 min and 240 min post-MT. In contrast, non-fed fish in both cohorts showed no significant periprandial changes in 5HT levels. Consequently, 5HT values were at some time lower in non-fed than in fed fish (90 min post-MT, p<0.05). The 5HIAA content in the hindbrain of morning-fed trout increased significantly before MT and remained elevated thereafter. Trout fed at ZT6 showed no significant variation throughout the entire sampling period. The 5HIAA content did not vary with time in the unfed fish of both cohorts, nor did it change relative to fed fish. The 5HIAA/5HT ratio in the hindbrain, increased transiently in MT in both cohorts, but returned to basal levels thereafter. Non-fed fish showed no such increase at MT, but 5HIAA/5HT gradually increased to significantly higher values 90 min after MT. A similar profile was not observed in fish from the second cohort (ZT6).
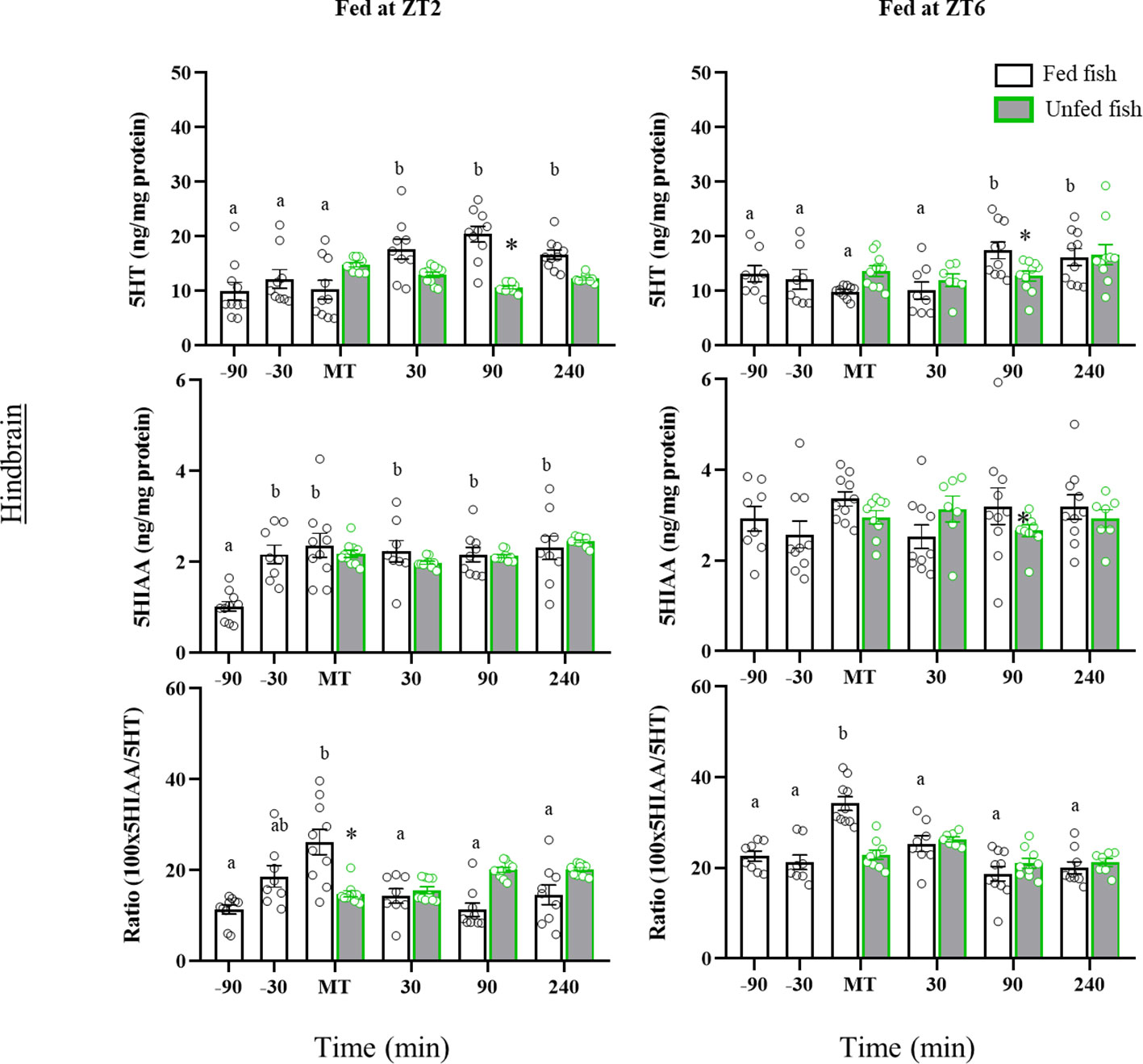
Figure 5 Periprandial changes and effects of fasting in the levels of serotonin (5HT) and 5-hydroxyindoleacetic acid (5HIAA), and the 5HIAA/5HT ratio in the hindbrain of rainbow trout with two different feeding schedules, morning (ZT2) and afternoon (ZT6). The results are shown as the mean ± SEM of n=10 fish per group. Different letters indicate significant differences among times in the fed fish group. Asterisk indicates significant differences between non-fed and fed fish at each specific time (P<0.05).
In the telencephalon, ZT2-fed trout showed significant postprandial decreases in 5HT (90- and 240 min post-MT) and 5HIAA (30-, 90- and 240-min post-MT) contents compared to earlier times (Figure 6). A decrease was also noted for 5HIAA in non-fed fish, but not for 5HT whose levels remained elevated (p<0.05 vs respective post-prandial times of fed fish). In contrast, fish fed normally in the afternoon (ZT6) had a transient prandial increase in the contents of 5HT (MT) and 5HIAA (MT and 30 min post-MT), which did not occur in non-fed fish. The periprandial profile of 5HIAA/5HT ratio values in the telencephalon was quite similar in both cohorts, with a gradual increase until the time of feeding (-30 min and MT in ZT2; MT in ZT6, p<0.05 vs. previous sampling points) and a decline to basal values after feeding. Non-fed fish lacked the increase in 5HIAA/5HT ratio shown for those fed at the time of feeding.
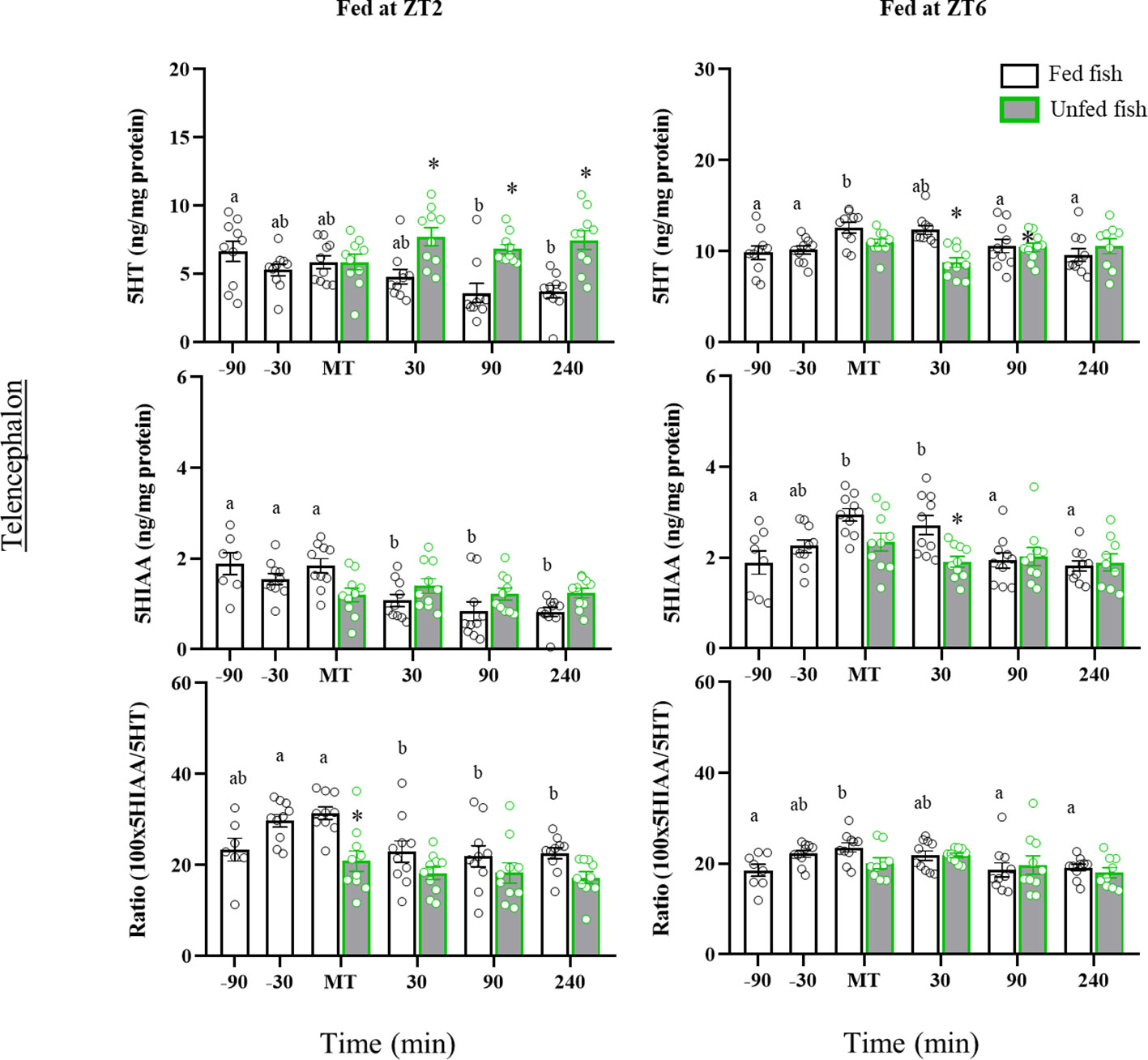
Figure 6 Periprandial changes and effects of fasting in the levels of serotonin (5HT) and 5-hydroxyindoleacetic acid (5HIAA), and the 5HIAA/5HT ratio in the telencephalon of rainbow trout with two different feeding schedules, morning (ZT2) and afternoon (ZT6). The results are shown as the mean ± SEM of n=10 fish per group. Different letters indicate significant differences among times in the fed fish group. Asterisk indicates significant differences between non-fed and fed fish at each specific time (P<0.05).
The mRNA abundance of 5HT receptors (5htr1a, 5htr2c and 5htr1b) was measured only at diencephalic level (Table 2). The expression of the genes evaluated did not change significantly throughout the periprandial period, despite a tendency of 5htr2c to increase and 5htr1a to decrease after MT in both cohorts. Non-fed fish showed 5htr2c mRNA abundance profile like that of fed fish in both cohorts, although a decrease was noted at +240 min in the ZT2 cohort compared to fed fish. Likewise, 5htr1b mRNA abundance showed a decrease at the end of the sampling period in non-fed fish (+90 min, +240 min, p<0.05 vs. fed fish) from both ZT2 and ZT6 cohorts.
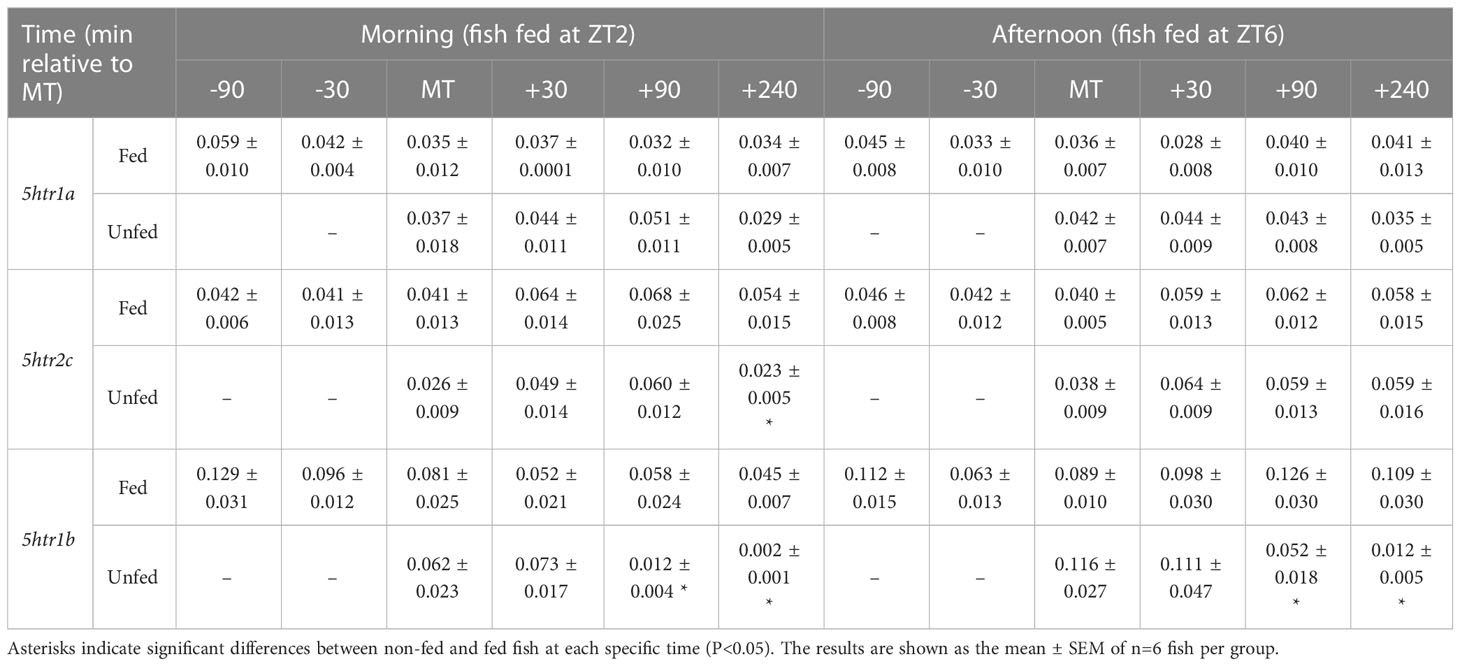
Table 2 Periprandial changes in mRNA abundance of diencephalic serotonin receptors of rainbow trout normally fed at two different daily times (morning, ZT2, and afternoon, ZT6) or unfed.
4 Discussion
4.1 Feeding influence on mRNA levels of neuropeptides in diencephalon
This study reports periprandial profiles in mRNA abundance of food intake-related neuropeptides in concert with serotonergic activity in the rainbow trout brain. The changes found in diencephalic neuropeptides are consistent with the role commonly attributed to them in the regulation of food intake in teleost fish. Thus, the highest mRNA abundance of the orexigenic neuropeptides (npy and agrp1) occurred before MT, decreasing shortly after feeding the fish, regardless of whether they were fed in the morning or in the afternoon. Meanwhile, mRNA levels of the anorexigenic neuropeptide pomca1 increased significantly at MT and remained elevated for the next four hours. In contrast, crfb showed a tendency to decrease after food intake, whereas no periprandial changes were found in cartpt, so the involvement of these two neuropeptides in the control of routine food intake seems to be of less relevance.
The literature in salmonids points to NPY as a short-term appetite regulator, usually as a hunger signal (42–44). In rainbow trout, treatment (icv) with NPY dose-dependently increased food intake at 2 to 3 hours (45), supporting an orexigenic role. In relation to mealtime, brain expression of npy has been shown to fluctuate widely, with increases occurring minutes before mealtime even when different feeding frequencies are scheduled throughout the day (46). In the same line, increases in npy mRNA levels in the hypothalamus of trout after 3 weeks of fasting have been reported (47). Our data are consistent with the orexigenic role of NPY in rainbow trout as a higher abundance of hypothalamic npy mRNA was found preprandially in both morning (ZT2) and afternoon (ZT6) fed fish, with smaller differences later. Thus, npy transcript levels decrease sharply 90 min before MT in the morning, but 30 min after MT in the afternoon, perhaps reflecting the interference between daily fluctuations in peptide transcription and feeding-related changes. Indeed, we previously reported that npy mRNA levels in trout are highest during the second half of the night gradually decreasing until the onset of the light phase (37). Consequently, the high transcript levels observed in the morning, i.e., 90 min before MT (30 min after turning on the lights) could reflect that npy mRNA abundance was still decreasing toward basal at the time the fish were fed (2 h after turning on the lights), an effect that was not found in fish fed in the afternoon. Meanwhile, non-fed fish from both cohorts did not show a postprandial decrease in npy mRNA levels but even an increase (in the morning) was found compared to preprandial values. This is in line with other fish species in which npy mRNA abundance up-regulates in the hypothalamus of food-deprived fish (48–50). Furthermore, in fed trout the decrease in npy expression in the diencephalon after feeding is simultaneous with the increase in blood glucose levels, which was not observed in fasted fish. This is consistent with the role of NPY-containing hypothalamic neurons in sensing metabolic state (i.e., glucose levels), as previously reported for trout (51) and another teleost (52–54).
Feeding also influenced agrp1 gene expression, with elevated levels of the transcript prior to MT and a decrease shortly after the meal. This fluctuation suggests that agrp1 has an orexigenic role in rainbow trout, consistent with other salmonids such as Atlantic salmon (55) and coho salmon (56). Meanwhile, agrp1 mRNA abundance in fasted trout remained transiently elevated (30 min after feeding) and decreased thereafter. This increase in fasting condition agrees with that reported in Atlantic salmon deprived of food for three days (55), although in other teleosts more days of food deprivation seem to be necessary for this increase to be observed (50, 57, 58). Changes in agrp1 mRNA abundance were similar in the morning and afternoon cohorts, suggesting that acclimation to a particular feeding schedule does not determine the expression profile of this peptide in rainbow trout. However, as for npy, basal level of agrp1 mRNA abundance was higher in the early morning than in the afternoon, consistent with peptide expression being affected by circadian fluctuations, as previously reported (37).
The melanocortin system seems to be a key player in the regulation of energy balance in fish (59). POMC neurons have been found in the hypothalamus of goldfish by in situ hybridization (59), while transcripts of the peptide have also been detected in the hindbrain of rainbow trout (51). Treatments with POMC-derived peptides, i.e., α-MSH or drugs acting on the MC4R clearly support that POMC plays an inhibitory role in food intake (6, 57). Changes in POMC transcript content during feeding are relatively consistent in salmonids, including the rainbow trout where pomca1 transcript levels increased after feeding (47) and respond to increased glucose levels (51). However, fasting-related changes are more inconsistent across species and time (55, 60). In rainbow trout, differential responses in POMC transcript variants to fasting were reported, with pomca1 being the only affected after 28-days food deprivation (61). Our data agree with the previous studies and show an increase in pomca1 mRNA abundance at MT and postprandially (+30 and +90 min). Meanwhile, non-fed trout showed no such increase, suggesting that feeding-related signals rapidly influence hypothalamic expression of pomca1 in rainbow trout.
Postprandial increases in cartpt mRNA abundance have been described in Atlantic salmon (60), goldfish (62), and catfish (63), among other teleost species, suggesting that this peptide acts as a short-term satiety signal (6). However, data in rainbow trout are scarce. This study shows that cartpt mRNA levels remained unchanged around the time of feeding, whereas they increased during the postprandial phase in trout that did not receive food. There are no clear reasons to explain this discrepancy, although it has been suggested that prandial changes in this peptide could be specific to the different cartpt genes identified in teleosts (64, 65). Even, a different role of the peptide in the short and long term has been suggested in some species (66, 67). Our present data do not support an inhibitory role of CART in food intake, but neither can it be ruled out that this peptide acts as a hunger signal under fasting conditions, which could be related to foraging (68).
CRF has been pointed as a negative regulator of food intake in teleost. In rainbow trout, studies showed that central CRF treatments stimulate the hypothalamic-pituitary-interrenal (HPI) axis to induce glucocorticoid (i.e., cortisol) secretion, which is further associated with anorectic effects (32, 34, 69). However, prandial changes in crf mRNA levels are scarcely known in salmonids and in general in teleost, whereas fasting decreased brain expression levels in other fish species (70, 71). In our study, crfb mRNA abundance enriched before the meal and down-regulated with the meal (ZT2 and ZT6), which is not clearly consistent with an anorexigenic role of this peptide. Furthermore, fasted fish did not have such a postprandial drop in crfb, but an increase was observed in ZT2-fed trout. Since changes in CRF are often related to fluctuations in HPI axis activity, cortisol levels in periprandial times were measured. We found an increase in cortisol levels before feed intake in trout ZT6-fed trout, which was not evident in those fed at ZT2 probably due to interference from elevated cortisol levels that in this species occur at the end of the night (72). In addition, cortisol values decreased postprandially in both fed cohorts, but not in the unfed fish. These data suggest that blood cortisol levels could be affected by feeding, increasing during mealtime in fed fish, and after mealtime in fish not fed at the expected time. This is consistent with other studies showing that the activity of HPI axis up-regulates during periods of fasting, increasing circulating cortisol levels (73, 74). Taken together, our data provide a similar picture during immediate fasting, which could be interpreted as an activation of the (eu)stress response linked to an alert before the arrival of food or to food seeking when it does not arrive at the expected time.
4.2 Periprandial changes of serotonergic activity in brain
In vertebrates, depolarization of serotonergic neurons is associated with increased 5HT synthesis to replenish the neurotransmitter in nerve terminals. This process involves the activation of TPH, the limiting enzyme of 5HT synthesis (12). Two TPH isoforms, TPH1 and TPH2, with different catalytic specificity and substrate affinity (75) are known in vertebrates. There is evidence that mammalian tph1 mRNA is distributed in peripheral tissues and the pineal gland, whereas tph2 expression is mainly restricted to the raphe nuclei of the brainstem (76, 77). However, in fish, the central serotonergic system extends to other brain structures, including hypothalamic and pretectal areas (26, 77). In rainbow trout, we have recently reported that both forms of tph are expressed in the central nervous system (27), albeit with important topographical differences. Whereas the major presence of tph2 is found in the raphe and reticular formation of the hindbrain, both tph1 and tph2 exist in the diencephalic nuclei, with tph1 restricted to the hypothalamic area. Our present data are consistent with the presence of both isoforms in the diencephalon, whereas tph2 mRNA transcripts were only detected in the hindbrain. Expression levels of tph were negligible in the telencephalon, consistent with the absence of 5HT-producing cells in forebrain areas (27).
In this study, significant periprandial changes in 5HT content were noticed in brain regions of rainbow trout that are key in feeding regulation. In both the hindbrain and diencephalon, 5HT content increased shortly after feeding in fed fish, but not in non-fed fish, in which 5HT content was lower. In addition, a postprandial increase in 5HIAA levels in the diencephalon (30 and 90 min after feeding) was observed in both morning and afternoon fed fish, which was interpreted as an increased utilization of 5HT associated with the postprandial period. Diencephalic serotonergic activation is also supported by a higher abundance of tph1 mRNA, the most prevalent TPH isoform in this region. In contrast, tph2 mRNA levels decreased after the meal, although expression levels of this enzyme in the diencephalon were very low, suggesting that its contribution to serotonergic functionality is restricted. Overall, the changes in diencephalic serotonergic activity agree with previous pharmacological studies in rainbow trout in which increases in central 5HT (29, 33, 69) were associated with inhibition of food intake. Our data suggest that the inhibitory role of 5HT occurs at a time after feeding, i.e., the amine may act as a satiety signal. The fact that non-fed fish showed no alterations in periprandial 5HT levels and had a less consistent postprandial increase in 5HIAA levels than fed fish further supports the functional involvement of 5HT in postprandial satiety.
The release of monoaminergic neurotransmitters into the synaptic cleft occurs in discrete bursts and the amine is then reuptaken into the terminals and metabolized. Consequently, short-term changes in tissue contents of 5HT and 5HIAA respond to alterations in neuronal release, with the 5HIAA/5HT ratio being considered a valuable parameter to define synaptic mobilization of the neurotransmitter (40, 78, 79). However, neuronal turnover depends on the influence of several parameters, such as neurotransmitter synthesis, transport, reuptake, and degradation, which could be regulated independently. Thus, neuronal activation may result in simultaneous increases or decreases in 5HT and 5HIAA contents that do not translate into ratio alterations, so changes in these parameters should be analyzed individually. In our study, serotonergic activity was elevated at the time of food intake, as suggested by the increased values of the 5HIAA/5HT ratio, then remaining high for a long time, as showed by enhanced 5HIAA content in both the diencephalon and hindbrain. This suggests that prandial activation of serotonergic neurons is rapid and quite prolonged in time, possibly extending into the post-absorptive satiety phase.
Feeding is a complex behavioral process that also affects foraging and develops in anticipation of the next meal in the form of increased motor activity, social interactions, or the accentuation of hierarchies, among others (80, 81). The so-called meal anticipatory activity is a typical example of scheduled daily pre-feeding behavior, which in teleost is under circadian control (38, 82). Strikingly, we observed that either tph1 mRNA abundance (ZT2 and ZT6 cohorts) or the 5HIAA/5HT ratio (ZT2 cohort) increased before the time of feeding (-30 min, compared to previous values), suggesting that diencephalic serotonergic activity already increases before the fish receives food. These results are novel relative to previous pharmacological studies and point to diencephalic 5HT being involved not only in the cessation of feeding, but also in its initiation. Our data point to a possible circadian influence driving an increase in serotonergic activity prior to feeding; however, the non-inclusion of a randomly fed group in the study precludes drawing conclusions in this regard. There is also no previous information linking the preprandial increase in hypothalamic serotonergic activity to other feeding-related behaviors. However, administration of enhancers of 5HTergic activity results in increased locomotor activity in zebrafish (83), while brain 5HT is involved in complex social processes, such as hierarchy formation, aggression, and social defeat (84), which may be exacerbated prior to feeding when animals are most active. In cavefish, it has been proposed that two neighboring hypothalamic populations of serotonergic neurons play dissimilar roles, one related to satiety and the other modulating foraging and aggression (85, 86), with these behaviors likely to be enhanced prior to feeding. Therefore, it is possible that serotonergic neurons at diencephalic locations are involved in pre-feeding behavior in teleost fish, although the specific mechanisms are unknown.
Serotonergic activity in the hindbrain also increased before the meal (ZT2-fed fish) or during the meal (ZT6-fed fish), showing increased levels of 5HIAA and/or an increased 5HIAA/5HT ratio. In addition, tph2 mRNA abundance was significantly increased in the hindbrain during the postprandial phase. This region contains clusters of neurons homologous to mammalian raphe nuclei, which intensely express tph2 transcripts (27). TPH2 enzyme activity is known to be more susceptible to be influenced by substrate availability than TPH1 (87), so tryptophan influx during feeding could lead to increased 5HT synthesis at sites with elevated TPH2 activity, such as mammalian raphe nuclei (76) or the hindbrain of fish (15). Serotonergic neurons in the teleost raphe region project extensively to hypothalamic areas (77), making this region susceptible to alterations in 5HT related to substrate availability. Therefore, food and the resulting increase in tryptophan availability could also affect hypothalamic 5HT content and release through its effects on 5HT biosynthesis in neuronal projections originating from the raphe. Thus, our data are consistent with a possible contribution of 5HT from raphe neurons to hypothalamic projections, which could be restricted to the postprandial time when 5HT is involved in the cessation of food intake.
The projections of dorsal and ventral 5HT neuronal populations from the hindbrain and diencephalon reach distinct forebrain groups. The changes detected in our study in serotonergic parameters in the telencephalon were relatively distinct from those found in the diencephalon. While food intake was followed (0 and 30 min) by higher levels of 5HT and 5HIAA in the afternoon-fed fish, an increase in the 5HIAA/5HT ratio was also evident at the time of feeding, but not before feeding, in the morning-fed fish. Non-fed fish did not show such an increase, so it appears that mealtime-associated signals trigger 5HTergic neuronal activation in this region. In addition, there was a decrease in 5HTergic activity after feeding in trout that ate at their feeding time, which did not appear in trout that did not receive food. These data rule out a role for telencephalic 5HT as a satiety-related signal, although it may have a role in those not receiving food at the expected time. Several 5HT receptors such as the subtypes 5HT2A, 5HT1A, 5HT1B and 5HT2C are expressed in fish forebrain, implicating the amine in different physiological processes (88). Our results support a role of 5HT in the forebrain distinct from that related to satiety and may include processes such as the management of sensory information related to food, locomotor activity associated with foraging or related to the reward system (89), although this deserves further investigation.
4.3 Linking changes in brain 5HT and feeding control
Overall, the serotonergic parameters analyzed in our study indicate that feeding time is coupled with increased 5HT abundance in somatodendritic and projection areas harboring neuropeptides related to regulation of food intake (Figure 7). Additionally, we monitored changes in periprandial mRNA abundance of three 5HT receptor subtypes, 5HTR1A, 5HTR1B, and 5HTR2C, which have been shown in mammals to prominently regulate food intake (10, 11, 90). No changes in 5HT receptor mRNA abundance were found in fed trout, limiting any conclusions about a possible relationship with food intake regulation at prandial and postprandial times. In fish, mechanistic studies are not as precise as in mammals, but treatments with 5HT or drugs that stimulate 5HTergic signaling, such as fluoxetine or d-fenfluramine, reduced the rate of food intake in goldfish (28, 31) and rainbow trout (29, 30). As for the 5HT receptors mediating this action, the most consistent results point to an involvement of the 5HTR2C subtype, whose agonist activation produces a decrease in food intake along with an increase in pomc expression in the hypothalamus of rainbow trout (33, 91). This is in agreement with mammalian data, where POMC neurons contains 5htr2c receptors whose activation largely determines the anorectic effect of the amine. In our study, a reduction in 5htr2c mRNA levels was found in fasted compared to fed fish, which was very late (+240 min) relative to the timing of feeding and was observed only in the morning cohort of fish. In fed fish, some tendency for a postprandial increase was found in both cohorts, but without statistical significance. Therefore, these results are still too weak to predict an involvement of these receptors in the regulation of daily feed intake in rainbow trout, which requires further studies.
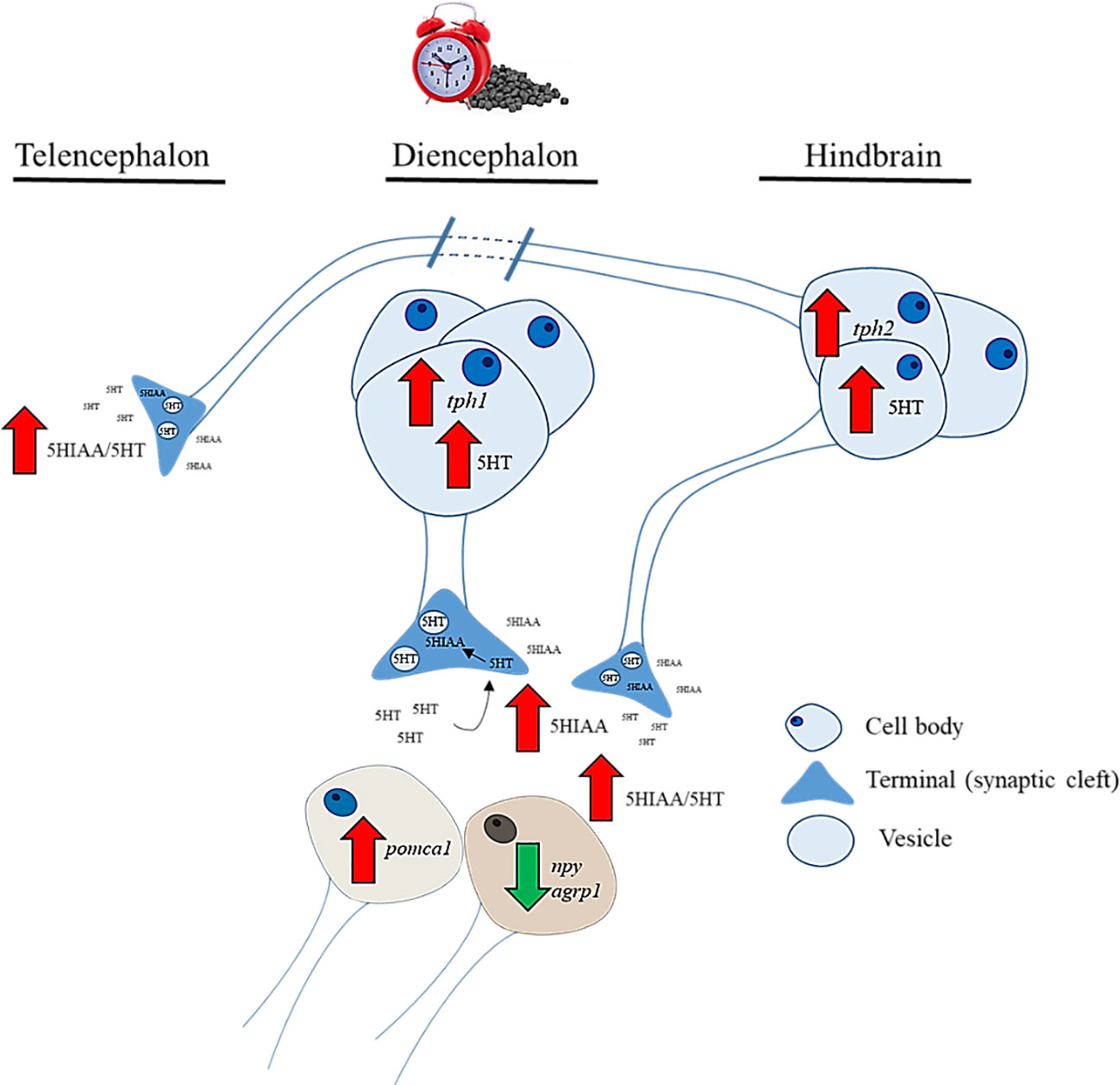
Figure 7 Graphical summary about the regional changes in brain serotonin functioning during feeding time in rainbow trout and proposed action on hypothalamic neuropeptides involved in food intake regulation.
Treatment with 5HTR1A receptor agonists did not alter feed intake in rainbow trout (33), which is in line with the lack of periprandial changes we have found in this receptor in fed trout. There was also no change in 5htr1b mRNA abundance in fed trout, whereas non-fed trout showed a significant down-regulation in receptor expression at 90 and 240 min after the expected feeding time, which was concomitant with a certain attenuation of serotonergic activity. In mammals, 5htr1b is expressed in NPY/AGRP neurons of the arcuate nucleus, and its activation leads to neuronal hyperpolarization (21) and anorexigenic effects. With this in mind, it appears that delayed decrease in 5htr1b expression in fasted trout could act as a facilitating mechanism for hunger signals once the feeding period was exceeded and there was no access to food. Although existing pharmacological evidence in trout does not allow linking a modulatory action of 5HT on npy expression in hypothalamus (30), the increase in serotonin produced by fluoxetine injections increased such expression in goldfish (31), so this possibility should not be ruled out.
In summary, our results reveal that rainbow trout acclimated to two daily feeding schedules develop a complex serotonergic response that may, in part, be influenced by circadian inputs related with the time at which the food is provided. In addition, feeding induces anticipatory activity affecting neuropeptides systems and the serotonergic system at the diencephalic level. In relation to 5HT, the results point to an inhibitory role of this neurotransmitter in food intake probably acting as a satiety-related signal, which could be mediated by interaction with hypothalamic neuropeptides. The results also suggest additional roles of 5HT during the preprandial phase in which serotonergic activity increases. Further research on 5HT neuronal networks and links with other neurotransmitter systems is necessary to expand our knowledge of the involvement of the brain 5HT in feeding behavior in fish.
Data availability statement
The datasets presented in this study can be found in online repositories. The names of the repository/repositories and accession number(s) can be found in the article/Supplementary Material.
Ethics statement
The animal study was approved by Animal Ethics Committee of the University of Vigo. The study was conducted in accordance with the local legislation and institutional requirements.
Author contributions
MC and JM conceived and designed the experiments. MC, RC, and ML-P carried out the experiments and analyzed the samples. MC, JM, MA, JC-R, and JLS interpreted the data and drafted the manuscript. Funding acquisition and project administration: JLS and JM. All authors contributed to manuscript revision, read, and approved the submitted version.
Funding
This research was supported by grants from Spanish Agencia Estatal de Investigación and European Fund of Regional Development (PID2022-136288OB-C31), and Xunta de Galicia (Consolidación e estructuración de unidades de investigación competitivas do SUG, ED431B 2019/37). MC was a recipient of predoctoral (FPI Program, Spanish Ministerio de Ciencia e Innovación, BES-2017-079708) and postdoctoral (Xunta de Galicia, ED481B-2022-082) fellowships.
Conflict of interest
The authors declare that the research was conducted in the absence of any commercial or financial relationships that could be construed as a potential conflict of interest.
The handling editor NP declared a past co-authorship with the author JLS.
Publisher’s note
All claims expressed in this article are solely those of the authors and do not necessarily represent those of their affiliated organizations, or those of the publisher, the editors and the reviewers. Any product that may be evaluated in this article, or claim that may be made by its manufacturer, is not guaranteed or endorsed by the publisher.
Supplementary material
The Supplementary Material for this article can be found online at: https://www.frontiersin.org/articles/10.3389/fendo.2023.1241019/full#supplementary-material
References
1. Rui L. Brain regulation of energy balance and body weight. Rev Endocr Metab Disord (2013) 14(4):387–407. doi: 10.1007/s11154-013-9261-9
2. Sobrino-Crespo C, Perianes-Cachero A, Puebla-Jiménez L, Barrios V, Arilla-Ferreiro E. Peptides and food intake. Front Endocrinol (2014) 5:58. doi: 10.3389/fendo.2014.00058
3. Volkoff H. The neuroendocrine regulation of food intake in fish: A review of current knowledge. Front Neurosci (2016) 10:540. doi: 10.3389/fnins.2016.00540
4. Morton GJ, Cummings DE, Baskin DG, Barsh GS, Schwartz MW. Central nervous system control of food intake and body weight. Nature (2006) 443:289–95. doi: 10.1038/nature05026
5. Waterson MJ, Horvath TL. Neuronal regulation of energy homeostasis: beyond the hypothalamus and feeding. Cell Metab (2015) 22:962–70. doi: 10.1016/j.cmet.2015.09.026
6. Delgado MJ, Cerdá-Reverter JM, Soengas JL. Hypothalamic integration of metabolic, endocrine, and circadian signals in fish: Involvement in the control of food intake. Front Neurosci (2017) 11:354. doi: 10.3389/fnins.2017.00354
7. Soengas JL, Cerdá-Reverter JM, Delgado MJ. Central regulation of food intake in fish: An evolutionary perspective. J Mol Endocrinol (2018) 60:171–99. doi: 10.1530/JME-17-0320
8. Vera LM, De Pedro N, Gómez-Milán E, Delgado MJ, Sánchez-Muros MJ, Madrid JA, et al. Feeding entrainment of locomotor activity rhythms, digestive enzymes and neuroendocrine factors in goldfish. Physiol Behav (2007) 90:518–24. doi: 10.1016/j.physbeh.2006.10.017
9. Tecott LH. Serotonin and the orchestration of energy balance. Cell Metab (2007) 6(5):352–61. doi: 10.1016/j.cmet.2007.09.012
10. Sohn JW, Elmquist JK, Williams KW. Neuronal circuits that regulate feeding behavior and metabolism. Trends Neurosci (2013) 36:504–12. doi: 10.1016/j.tins.2013.05.003
11. Donovan MH, Tecott LH. Serotonin and the regulation of mamMalian energy balance. Front Neurosci (2013) 7:36. doi: 10.3389/fnins.2013.00036
12. Höglund E, Øverli Ø., Winberg S. Tryptophan metabolic pathways and brain serotonergic activity: A comparative review. Front Endocrinol (Lausanne) (2019) 10:158. doi: 10.3389/fendo.2019.00158
13. Sangkuhl K, Klein TE, Altman RB. Selective serotonin reuptake inhibitors pathway. Pharmacogenet Genomics (2009) 19(11):907–9. doi: 10.1097/FPC.0b013e32833132cb
14. Boadle-Biber MC. Regulation of serotonin synthesis. Prog Biophys Mol Biol (1993) 60(1):1–15. doi: 10.1016/0079-6107(93)90009-9
15. Gaspar P, Lillesaar C. Probing the diversity of serotonin neurons. Philos Trans R Soc Lond B Biol Sci (2012) 367(1601):2382–94. doi: 10.1098/rstb.2011.0378
16. Rozenblit-Susan S, Chapnik N, Genzer Y, Froy O. Serotonin suppresses food anticipatory activity and synchronizes the food-entrainable oscillator during time-restricted feeding. Behav Brain Res (2016) 297:150–4. doi: 10.1016/j.bbr.2015.10.019
17. Barnes NM, Sharp T. A review of central 5-HT receptors and their function. Neuropharmacology (1999) 38:1083–152. doi: 10.1016/S0028-3908(99)00010-6
18. Xu Y, Jones JE, Kohno D, Williams KW, Lee CE, Choi MJ, et al. 5-HT2CRs Expressed by pro-opiomelanocortin neurons regulate energy homeostasis. Neuron (2008) 60:582–9. doi: 10.1016/j.neuron.2008.09.033
19. Doslikova B, Garfield AS, Shaw J, Evans ML, Burdakov D, Billups B, et al. 5-HT2C Receptor agonist anorectic efficacy potentiated by 5-HT1B receptor agonist coapplication: an effect mediated via increased proportion of pro-opiomelanocortin neurons activated. J Neurosci (2013) 33:9800–4. doi: 10.1523/JNEUROSCI.4326-12.2013
20. Clemett DA, Punhani T, Duxon MS, Blackburn TP, Fone KC. Immunohistochemical localisation of the 5-HT2C receptor protein in the rat CNS. Neuropharmacology (2000) 39(1):123–32. doi: 10.1016/s0028-3908(99)00086-6
21. Heisler LK, Jobst EE, Sutton GM, Zhou L, Borok E, Thornton-Jones Z, et al. Serotonin reciprocally regulates melanocortin neurons to modulate food intake. Neuron (2006) 51:239–49. doi: 10.1016/j.neuron.2006.06.004
22. Balthasar N, Dalgaard LT, Lee CE, Yu J, Funahashi H, Williams T, et al. Divergence of melanocortin pathways in the control of food intake and energy expenditure. Cell (2005) 123(3):493–505. doi: 10.1016/j.cell.2005.08.035
23. Zhou Y, Liang XF, Yuan X, Li J, He Y, Fang L, et al. Neuropeptide Y stimulates food intake and regulates metabolism in grass carp, Ctenopharyngodon idellus. Aquaculture (2013) 380–383:52–61. doi: 10.1016/j.aquaculture.2012.11.033
24. Yao T, He J, Cui Z, Wang R, Bao K, Huang Y, et al. Central 5-HTR2C in the control of metabolic homeostasis. Front Endocrinol (2021) 12:694204. doi: 10.3389/fendo.2021.694204
25. Voigt J-P, SChade R, Fink H, Hörtnagl H. Role of 5-HT1A receptors in the control of food intake in obese Zucker rats of different ages. Pharmacol Biochem Behav (2002) 72:403–9. doi: 10.1016/S0091-3057(01)00763-8
26. Frankenhuis-van den Heuvel THM, Nieuwenhuys R. Distribution of serotonin-immunoreactivity in the diencephalon and mesencephalon of the trout, Salmo gairdneri – Cellbodies, fibres and terminals. Anat Embryol (Berl) (1984) 169:193–204. doi: 10.1007/BF00303149
27. Chivite M, Leal E, Míguez JM, Cerdá-Reverter JM. Distribution of two isoforms of tryptophan hydroxylase in the brain of rainbow trout (Oncorhynchus mykiss). An in situ hybridization study. Brain Struct Funct (2021) 226(7):2265–78. doi: 10.1007/s00429-021-02322-8
28. De Pedro N, Pinillos ML, Valenciano AI, Alonso-Bedate M, Delgado MJ. Inhibitory effect of serotonin on feeding behaviour in goldfish: Involvement of CRF. Peptides (1998) 19:505–11. doi: 10.1016/S0196-9781(97)00469-5
29. Ruibal C, Soengas JL, Aldegunde M. Brain serotonin and the control of food intake in rainbow trout (Oncorhynchus mykiss): Effects of changes in plasma glucose levels. J Comp Physiol A Neuroethol. Sensory Neural Behav Physiol (2002) 188:479–84. doi: 10.1007/s00359-002-0320-z
30. Mancebo MJ, Ceballos FC, Pérez-Maceira J, Aldegunde M. Hypothalamic neuropeptide Y (NPY) gene expression is not affected by central serotonin in the rainbow trout (Oncorhynchus mykiss). Comp Biochem Physiol – A Mol Integr Physiol (2013) 166:186–90. doi: 10.1016/j.cbpa.2013.05.034
31. Mennigen JA, Harris EA, Chang JP, Moon TW, Trudeau VL. Fluoxetine affects weight gain and expression of feeding peptides in the female goldfish brain. Regul Pept (2009) 155:99–104. doi: 10.1016/j.regpep.2009.01.001
32. Ortega VA, Lovejoy DA, Bernier NJ. Appetite-suppressing effects and interactions of centrally administered corticotropin-releasing factor, urotensin I and serotonin in rainbow trout (Oncorhynchus mykiss). Front Neurosci (2013) 7:196. doi: 10.3389/fnins.2013.00196
33. Pérez-Maceira JJ, Mancebo MJ, Aldegunde M. The involvement of 5-HT-like receptors in the regulation of food intake in rainbow trout (Oncorhynchus mykiss). Comp Biochem Physiol – C Toxicol Pharmacol (2014) 161:1–6. doi: 10.1016/j.cbpc.2013.12.003
34. Pérez-Maceira JJ, Otero-Rodiño C, Mancebo MJ, Soengas JL, Aldegunde M. Food intake inhibition in rainbow trout induced by activation of serotonin 5-HT2C receptors is associated with increases in POMC, CART and CRF mRNA abundance in hypothalamus. J Comp Physiol B Biochem Syst Environ Physiol (2016) 186:313–21. doi: 10.1007/s00360-016-0961-9
35. Kehoe AS, Volkoff H. Cloning and characterization of neuropeptide Y (NPY) and cocaine and amphetamine regulated transcript (CART) in Atlantic cod (Gadus morhua). Comp Biochem Physiol – A Mol Integr Physiol (2007) 146:451–61. doi: 10.1016/j.cbpa.2006.12.026
36. Murashita K, Kurokawa T, Ebbesson LOE, Stefansson SO, Rønnestad I. Characterization, tissue distribution, and regulation of agouti-related protein (AgRP), cocaine- and amphetamine-regulated transcript (CART) and neuropeptide Y (NPY) in Atlantic salmon (Salmo salar). Gen Comp Endocrinol (2009) 162:160–71. doi: 10.1016/j.ygcen.2009.03.015
37. Naderi F, Hernández-Pérez J, Chivite M, Soengas JL, Míguez JM, López-Patiño MA. Involvement of cortisol and sirtuin1 during the response to stress of hypothalamic circadian system and food intake-related peptides in rainbow trout, Oncorhynchus mykiss. Chronobiol Int (2018) 35:1122–41. doi: 10.1080/07420528.2018.1461110
38. López-Olmeda JF, Tartaglione EV, de la Iglesia HO, Sánchez-Vázquez FJ. Feeding entrainment of food-anticipatory activity and per1 expression in the brain and liver of zebrafish under different lighting and feeding conditions. Chronobiol. Int (2010) 27:1380–400. doi: 10.3109/07420528.2010.501926
39. Gesto M, López-Patiño MA, Hernández J, Soengas JL, Míguez JM. Gradation of the stress response in rainbow trout exposed to stressors of different severity: the role of brain serotonergic and dopaminergic systems. J Neuroendocrinol (2015) 27(2):131–41. doi: 10.1111/jne.12248
40. Gesto M, Lopez-Patino MA, Hernandez J, Soengas JL, Miguez JM. The response of brain serotonergic and dopaminergic systems to an acute stressor in rainbow trout: a time course study. J Exp Biol (2013) 216:4435–42. doi: 10.1242/jeb.091751
41. Pfaffl MW. A new mathematical model for relative quantification in real-time RT-PCR. Nucleic Acids Res (2001) 29:e45. doi: 10.1093/nar/29.9.e45
42. Silverstein JT, Breininger J, Baskin DG, Plisetskaya EM. Neuropeptide Y-like gene expression in the salmon brain increases with fasting. Gen Comp Endocrinol (1998) 220:78–87. doi: 10.1006/gcen.1998.7058
43. Rønnestad I, Gomes AS, Murashita K, Angotzi R, Jönsson E, Volkoff H. Appetite-controlling endocrine systems in teleosts. Front Endocrinol (2017) 8:73. doi: 10.3389/fendo.2017.00073
44. Tolås I, Kalananthan T, Gomes AS, Lai F, Norland S, Murashita K, et al. Regional expression of NPY mRNA paralogs in the brain of Atlantic salmon (Salmo salar, l.) and response to fasting. Front Physiol (2021) 12:720639. doi: 10.3389/fphys.2021.720639
45. Aldegunde M, Mancebo M. Effects of neuropeptide Y on food intake and brain biogenic amines in the rainbow trout (Oncorhynchus mykiss). Peptides (2006) 27(4):719–27. doi: 10.1016/j.peptides.2005.09.014
46. Nahayat M, Zakeri M, Salati AP, Qasemi SA. Changes in the neuropeptide y mrna expression in Oncorhynchus mykiss at different feeding frequencies. Aquaculture Nutr (2022) 2022:9. doi: 10.1155/2022/2080639
47. Gong N, Björnsson BT. Leptin signaling in the rainbow trout central nervous system is modulated by a truncated leptin receptor isoform. Endocrinology (2014) 155:2445–55. doi: 10.1210/en.2013-2131
48. De Pedro N, Björnsson BT. Regulation of food intake by neuropeptides and hormones. In: Houlihan D, Boujard T, Jobling M, editors. Food Intake in Fish Oxford: Blackwell Science (2001). p. 269–96.
49. Volkoff H, Canosa LF, Unniappan S, Cerdá-Reverter JM, Bernier NJ, Kelly SP, et al. Neuropeptides and the control of food intake in fish. Gen Comp Endocrinol (2005) 142(1-2):3–19. doi: 10.1016/j.ygcen.2004.11.001
50. Wei R, Zhou C, Yuan D, Wang T, Lin F, Chen H, et al. Characterization, tissue distribution and regulation of neuropeptide Y in Schizothorax prenanti. J. Fish Biol (2014) 85:278–91. doi: 10.1111/jfb.12413
51. Conde-Sieira M, Agulleiro MJ, Aguilar AJ, Míguez JM, Cerdá-Reverter JM, Soengas JL. Effect of different glycaemic conditions on gene expression of neuropeptides involved in control of food intake in rainbow trout; Interaction with stress. J Exp Biol (2010) 213:3858–65. doi: 10.1242/jeb.048439
52. Narnaware YK, Peter RE. Influence of diet composition on food intake and neuropeptide Y (NPY) gene expression in goldfish brain. Regul Pept (2002) 103:75–83. doi: 10.1016/S0167-0115(01)00342-1
53. Riley LG, Walker AP, Dorough CP, Schwandt SE, Grau EG. Glucose regulates ghrelin, neuropeptide Y, and the GH/IGF-I axis in the tilapia, Oreochromis mossambicus. Comp Biochem Physiol A Mol Integr Physiol (2009) 154(4):541–6. doi: 10.1016/j.cbpa.2009.08.018
54. Tuziak SM, Rise ML, Volkoff H. An investigation of appetite-related peptide transcript expression in Atlantic cod (Gadus morhua) brain following a Camelina sativa meal-supplemented feeding trial. Gene (2014) 550(2):253–63. doi: 10.1016/j.gene.2014.08.039
55. Kalananthan T, Murashita K, Rønnestad I, Ishigaki M, Takahashi K, Silva MS, et al. Hypothalamic agrp and pomc mRNA Responses to Gastrointestinal Fullness and Fasting in Atlantic salmon (Salmo salar, L.). Front Physiol (2020) 11:61. doi: 10.3389/fphys.2020.00061
56. Kim JH, Leggatt RA, Chan M, Volkoff H, Devlin RH. Effects of chronic growth hormone overexpression on appetite-regulating brain gene expression in coho salmon. Mol Cell Endocrinol (2015) 413:178–88. doi: 10.1016/j.mce.2015.06.024
57. Cerdá-Reverter JM, Peter RE. Endogenous melanocortin antagonist in fish: Structure, brain mapping, and regulation by fasting of the goldfish agouti-related protein gene. Endocrinology (2003) 144:4552–61. doi: 10.1210/en.2003-0453
58. Agulleiro MJ, Cortés R, Leal E, Ríos D, Sánchez E, Cerdá-Reverter JM. Characterization, tissue distribution and regulation by fasting of the agouti family of peptides in the sea bass (Dicentrarchus labrax). Gen Comp Endocrinol (2014) 205:251–9. doi: 10.1016/j.ygcen.2014.02.009
59. Cerdá-Reverter JM, Agulleiro MJ, Guillot R R, Sánchez E, Ceinos R, Rotllant J. Fish melanocortin system. Eur J Pharmacol (2011) 660:53–60. doi: 10.1016/j.ejphar.2010.10.108
60. Valen R, Jordal AEO, Murashita K, Rønnestad I. Postprandial effects on appetite-related neuropeptide expression in the brain of Atlantic salmon, Salmo salar. Gen Comp Endocrinol (2011) 171:359–66. doi: 10.1016/j.ygcen.2011.02.027
61. Leder EH, Silverstein JT. The pro-opiomelanocortin genes in rainbow trout (Oncorhynchus mykiss): Duplications, splice variants, and differential expression. J Endocrinol (2006) 188:355–63. doi: 10.1677/joe.1.06283
62. Volkoff H, Peter RE. Interactions between orexin A, NPY and galanin in the control of food intake of the goldfish, Carassius auratus. Regul Pept (2001) 101:59–72. doi: 10.1016/S0167-0115(01)00261-0
63. Peterson BC, Waldbieser GC, Riley LG, Upton KR, Kobayashi Y, Small BC. Pre- and postprandial changes in orexigenic and anorexigenic factors in channel catfish (Ictalurus punctatus). Gen Comp Endocrinol (2012) 176:231–9. doi: 10.1016/j.ygcen.2012.01.022
64. Gomes AS, Jordal AE, Olsen K, Harboe T, Power DM, Rønnestad I. Neuroendocrine control of appetite in Atlantic halibut (Hippoglossus hippoglossus): changes during metamorphosis and effects of feeding. Comp Biochem Physiol A Mol Integr Physiol (2015) 3:116–25. doi: 10.1016/j.cbpa.2015.01.009
65. Kalananthan T, Gomes AS, Lai F, Tolås I, Jordal A-EO, Norland S, et al. Brain distribution of 10 cart transcripts and their response to 4 days of fasting in Atlantic salmon (Salmo salar l.). Front Mar Sci (2021) 8:763766. doi: 10.3389/fmars.2021.763766
66. Mechaly AS, Richardson E, Rinkwitz S. Activity of etv5a and etv5b genes in the hypothalamus of fasted zebrafish is influenced by serotonin. Gen Comp Endocrinol (2017) 246:233–40. doi: 10.1016/j.ygcen.2016.12.013
67. Zhang X, Gao Y, Tang N, Qi J, Wu Y, Hao J, et al. One evidence of cocaine- and amphetamine-regulated transcript (CART) has the bidirectional effects on appetite in Siberian sturgeon (Acipenser baerii). Fish Physiol Biochem (2018) 44:411–22. doi: 10.1007/s10695-017-0444-2
68. Volkoff H, Peter RE. Effects of CART peptides on food consumption, feeding and associated behaviors in the goldfish, Carassius auratus: Actions on neuropeptide Y- and orexin A-induced feeding. Brain Res (2000) 887:125–33. doi: 10.1016/S0006-8993(00)03001-8
69. Ortega VA, Renner KJ, Bernier NJ. Appetite-suppressing effects of ammonia exposure in rainbow trout associated with regional and temporal activation of brain monoaminergic and CRF systems. J Exp Biol (2005) 208:1855–66. doi: 10.1242/jeb.01577
70. Wang T, Cheng YZ, Liu ZP, Long XH. Effects of light intensity on husbandry parameters, digestive enzymes and whole-body composition of juvenile Epinephelus coioides reared in artificial sea water. Aquac. Res (2015) 46:884–96. doi: 10.1111/are.12241
71. Qi J, Tang N, Wu Y, Chen H, Wang S, Wang B, et al. The transcripts of CRF and CRF receptors under fasting stress in Dabry's sturgeon (Acipenser dabryanus Dumeril). Gen Comp Endocrinol (2019) 280:200–8. doi: 10.1016/j.ygcen.2019.05.005
72. Hernández-Pérez J, Naderi F, Chivite M, Soengas JL, Míguez JM, López-Patiño MA. Influence of stress on liver circadian physiology. A study in rainbow trout, Oncorhynchus mykiss, as fish model. Front Physiol (2019) 10:611. doi: 10.3389/fphys.2019.00611
73. Kelley KM, Haigwood JT, Perez M, Galima MM. Serum insulin-like growth factor binding proteins (IGFBPs) as markers for anabolic/catabolic condition in fishes. Comp Biochem Physiol – B: Biochem Mol (2001) 129(2-3):229–36. doi: 10.1016/S1096-4959(01)00314-1
74. Barcellos LJG, Marqueze A, Trapp M, Quevedo RM, Ferreira D. The effects of fasting on cortisol, blood glucose and liver and muscle glycogen in adult jundiá Rhamdia quelen. Aquaculture. (2010) 300(1–4):231–6. doi: 10.1016/j.aquaculture.2010.01.013
75. Walther DJ, Bader M. A unique central tryptophan hydroxylase isoform. Biochem Pharmacol (2003) 66:1673–80. doi: 10.1016/S0006-2952(03)00556-2
76. Panula P, Chen YC, Priyadarshini M, Kudo H, Semenova S, Sundvik M, et al. The comparative neuroanatomy and neurochemistry of zebrafish CNS systems of relevance to human neuropsychiatric diseases. Neurobiol Dis (2010) 40:46–57. doi: 10.1016/j.nbd.2010.05.010
77. Lillesaar C. The serotonergic system in fish. J Chem Neuroanat (2011) 41(4):294–308. doi: 10.1016/j.jchemneu.2011.05.009
78. Øverli Ø., Harris CA, Winberg S. Short-term effects of fights for social dominance and the establishment of dominant-subordinate relationships on brain monoamines and cortisol in rainbow trout. Brain Behav Evol (1999) 54:263–75. doi: 10.1159/000006627
79. López-Patiño MA, Skrzynska AK, Naderi F, Mancera JM, Míguez JM, Martos-Sitcha JA. High stocking density and food deprivation increase brain monoaminergic activity in gilthead sea bream (Sparus aurata). Anim (Basel) (2021) 1(6):1503. doi: 10.3390/ani11061503
80. Kulczykowska E, Sánchez-Vázquez FJ. Neurohormonal regulation of feed intake and response to nutrients in fish: aspects of feeding rhythm and stress. Aqua Rep (2010) 41(5):654–67. doi: 10.1111/j.1365-2109.2009.02350.x
81. Backström T, Winberg S. Serotonin coordinates responses to social stress-what we can learn from fish. Front Neurosci (2017) 11:595. doi: 10.3389/fnins.2017.00595
82. Sánchez-Vázquez FJ, Tabata M. Circadian rhythms of demand-feeding and locomotor activity in rainbow trout. J Fish Biol (1998) 52:255–67. doi: 10.1006/jfbi.1997.0576
83. Herculano AM, Maximino C. Serotonergic modulation of zebrafish behavior: Towards a paradox. Prog Neuropsychopharmacol Biol Psychiatry (2014) 55:50–66. doi: 10.1016/j.pnpbp.2014.03.008
84. Winberg S, Thörnqvist PO. Role of brain serotonin in modulating fish behavior. Curr Zool (2016) 62:317–23. doi: 10.1093/cz/zow037
85. Elipot Y, Hinaux H, Callebert J, Rétaux S. Evolutionary shift from fighting to foraging in blind cavefish through changes in the serotonin network. Curr Biol (2013) 23:1–10. doi: 10.1016/j.cub.2012.10.044
86. Rétaux S, Elipot Y. Feed or fight: A behavioral shift in blind cavefish. Commun Integr Biol (2013) 6:2. doi: 10.4161/cib.23166
87. McKinney J, Knappskog PM, Haavik J. Different properties of the central and peripheral forms of human tryptophan hydroxylase. J Neurochem (2005) 2(2):311–20. doi: 10.1111/j.1471-4159.2004.02850.x
88. Schneider H, Fritzky L, Williams J, Heumann C, Yochum M, Pattar K, et al. Cloning and expression of a zebrafish 5-HT2C receptor gene. Gene (2012) 502:108–17. doi: 10.1016/j.gene.2012.03.070
89. Chivite M, Comesaña S, Calo J, Soengas JL, Conde-Sieira M. Endocannabinoid receptors are involved in enhancing food intake in rainbow trout. Horm Behav (2022) 146:105277. doi: 10.1016/j.yhbeh.2022.105277
90. Lam DD, Przydzial MJ, Ridley SH, Yeo GSH, Rochford JJ, O’Rahilly S, et al. Serotonin 5-HT2C receptor agonist promotes hypophagia via downstream activation of melanocortin 4 receptors. Endocrinology (2008) 149:1323–8. doi: 10.1210/en.2007-1321
Keywords: feeding regulation, serotonin, neuropeptides, hypothalamus, brain, trout, teleost
Citation: Chivite M, Ceinos RM, Cerdá-Reverter JM, Soengas JL, Aldegunde M, López-Patiño MA and Míguez JM (2023) Unraveling the periprandial changes in brain serotonergic activity and its correlation with food intake-related neuropeptides in rainbow trout Oncorhynchus mykiss. Front. Endocrinol. 14:1241019. doi: 10.3389/fendo.2023.1241019
Received: 15 June 2023; Accepted: 08 August 2023;
Published: 24 August 2023.
Edited by:
Nuria de Pedro, Complutense University of Madrid, SpainReviewed by:
Suraj Unniappan, University of Saskatchewan, CanadaNicholas J. Bernier, University of Guelph, Canada
Copyright © 2023 Chivite, Ceinos, Cerdá-Reverter, Soengas, Aldegunde, López-Patiño and Míguez. This is an open-access article distributed under the terms of the Creative Commons Attribution License (CC BY). The use, distribution or reproduction in other forums is permitted, provided the original author(s) and the copyright owner(s) are credited and that the original publication in this journal is cited, in accordance with accepted academic practice. No use, distribution or reproduction is permitted which does not comply with these terms.
*Correspondence: Jesús M. Míguez, am1taWd1ZXpAdXZpZ28uZ2Fs