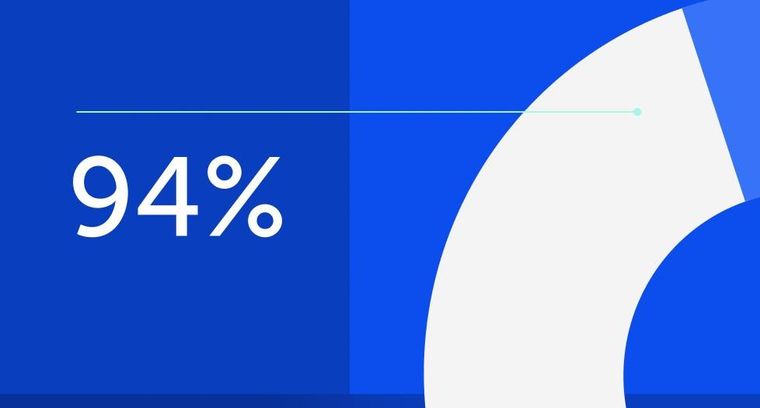
94% of researchers rate our articles as excellent or good
Learn more about the work of our research integrity team to safeguard the quality of each article we publish.
Find out more
REVIEW article
Front. Endocrinol., 19 September 2023
Sec. Bone Research
Volume 14 - 2023 | https://doi.org/10.3389/fendo.2023.1217579
This article is part of the Research TopicBone Cell Differentiation in Health and Disease, Volume IIView all 6 articles
Bone is a dynamic organ that, once formed, undergoes a constant remodeling process that includes bone resorption and synthesis. Osteoclasts and osteoblasts are primarily responsible for controlling this process. X-box binding protein 1 (XBP1), a transcription factor, affects the metabolism of bones in various ways. In recent years, numerous studies have revealed that XBP1 plays a vital role in bone metabolism, including osteoclast and osteoblast development, as well as in regulating immune cell differentiation that affects the immune microenvironment of bone remodeling. In this review, we highlight the regulatory mechanisms of XBP1 on osteoclasts and osteoblasts, how XBP1 affects the immune microenvironment of bone remodeling by influencing the differentiation of immune cells, and predict the possible future research directions of XBP1 to provide new insights for the treatment of bone-related metabolic diseases.
Bone is a rigid and dynamic organ that experiences a phenomenon referred to as remodeling (1, 2). In a typical state, there exists an equilibrium between the creation and breakdown of bone, overseen by osteoblasts and osteoclasts, respectively (3). Nevertheless, when these mechanisms become imbalanced, they can result in significant impairments to the structure and functionality of bone, consequently giving rise to various bone metabolic ailments, including osteoporosis, rheumatoid arthritis, and multiple myeloma (MM) (4–7).
Throughout the process of skeletal development, osteoblasts generate substantial quantities of extracellular matrix proteins. Endoplasmic reticulum stress (ER stress) is elicited by both endogenous and exogenous stimuli, leading to the activation of the unfolded protein response (UPR) (8, 9). In mammalian cells, three transmembrane proteins located in the endoplasmic reticulum serve as sensors for ER stress: protein kinase R-like endoplasmic reticulum kinase (PERK), activating transcription factor 6 (ATF6), and inositol-requiring enzyme 1α (IRE1α) (10). These proteins mediate the three branches of UPR signaling, namely PERK-eIF2α, ATF6, and IRE1α (11). Among these branches, the IRE1α/XBP1 pathway represents one of the most highly conserved signaling pathways throughout evolution (12). When IRE1α is activated, it facilitates the cleavage of unspliced XBP1 mRNA into spliced XBP1, which subsequently participates in the regulation of endoplasmic reticulum homeostasis, cell survival, angiogenesis, and other biological processes (13–15).
As a transcription factor, x-box binding protein 1 (XBP1) assumes a crucial function in the immune microenvironment of bone remodeling by promoting osteoclast formation (8, 16–18), osteoblast differentiation (19), and immune cell differentiation through diverse cytokines or signaling pathways (20).
XBP1, a key transcription factor, is crucial in the response of unfolded proteins under ER stress conditions and is associated with the pathogenesis of bone metabolic diseases (16, 21). Therefore, understanding the regulation of XBP1 in bone metabolism is crucial and may lead to new advances in treating various bone metabolic diseases. Current research on XBP1 has focused on the regulatory role in various tumor diseases, and little is known about its role in bone metabolic diseases. Herein, we summarize the critical roles of XBP1 in the differentiation of osteoclasts, osteoblasts, and immune cells, as well as in influencing the immune microenvironment of bone remodeling. In addition, the review also predicts possible future research directions of XBP1, providing new ideas for the treatment of various diseases.
It is a distinctive transcription factor of base-region leucine zipper (bZIP) that governs the regulation of human MHC class II genes (22, 23). Subsequent to this discovery, numerous investigations conducted approximately ten years later have demonstrated that XBP1 is a downstream component of IRE1α, a crucial transcription factor for the UPR in both invertebrates and vertebrates, and is indispensable for cellular survival in reaction to stressful stimuli (14, 24).
XBP1 can be produced by plasma cells (PCs), MM cells, and bone marrow stromal cells (BMSCs) (25, 26). XBP1 is highly expressed in PCs and alleviates ER Stress through UPR. XBP1 can also be induced in BMSCs in the MM microenvironment. Guoshuang Xu et al. found that XBP1 mRNA levels are significantly increased in BMSCs from MM patients compared to healthy donor. Meanwhile, they found that BMSCs with elevated levels of XBP1 protein induced more osteoclast formation (16).
XBP1 plays a role as a transcription factor not only in osteoclast differentiation but also in osteoblast differentiation. Initially, only literature using Northern-blot analysis demonstrated that XBP1 expresses during osteoblast differentiation, which PTH influences (27). A couple of years later, Takahide Tohmonda performed in vitro osteoblast differentiation assays using mouse embryonic fibroblasts (MEFs) and recombinant bone morphogenic protein 2 (BMP2) to detect the expression of early and late markers of osteoblast differentiation. These experimental results demonstrate that the IRE1α/XBP1 pathway is essential for BMP2-induced osteoblast differentiation (19).
In macrophages, the promotion of inflammatory cytokine production and M1-type macrophage polarization by XBP1 may play a role in osteoclast differentiation (28). Conversely, the activation of IRE1α/XBP1 signaling by estrogen can inhibit M1-type macrophage polarization (29). In CD4+ T cells, XBP1 can contribute to Th-cell polarization, leading to the secretion of various cytokines that impact osteoblast or osteoclast differentiation processes. Additionally, XBP1 in CD8+ T cells can regulate the expression of CD8+ T cell inhibitory receptors induced by cholesterol and induce T cell exhaustion. Furthermore, the activation of XBP1 by SIRT7 has been observed to selectively regulate the expression and secretion of cytokines, thereby exerting an influence on the process of bone remodeling. Within B cells, the inhibition of osteoclastogenesis can be achieved through the secretion of osteoprotegerin (OPG), which blocks the RANKL/RANK pathway. However, in MM PCs, the potential to promote osteoclastogenesis exists (30). Although XBP1 is abundantly expressed in PCs, the precise mechanism through which it impacts bone metabolism in this cell type remains unclear. It may affect the immune microenvironment of bone remodeling by influencing PCs differentiation and immunoglobulin (Ig) secretion.
Next, the review will discuss the role of XBP1 in regulating osteoclast differentiation, osteoblast differentiation, and affecting the immune microenvironment of bone metabolism, respectively (Figure 1).
Figure 1 Overview of the role of XBP1 in bone metabolism. During the process of osteoclast differentiation, the activation of endoplasmic reticulum stress by RANKL/RANK leads to the activation of IRE1α, resulting in slicing Xbp1u into mature Xbp1. X-box binding protein 1 (XBP1) can induce the formation of osteoclasts by promoting the transcription of Nfatc1 or by positively regulating the expression of the PTH/PTHrP pathway, which subsequently leads to bone resorption. Conversely, during osteoblast differentiation, XBP1 can be activated by various factors including proteasome inhibition (PI), bone morphogenic protein 2 (BMP2), lipopolysaccharide (LPS), and specific miRNAs such as Exo-miR-205-5p. XBP1 regulates the expression of Osterix, thereby facilitating osteoblast differentiation and bone formation, regulated by PTH. In macrophages, XBP1 can be activated by toll-like receptors (TLR), Annexin A2 (AnxA2), NF-κB, and estrogen. In T cells and B cells, XBP1 is highly expressed and thus may regulate bone metabolic processes. Yellow arrows represent increased osteoclastogenesis and blue arrows represent increased osteoblastogenesis.
The researchers conducted in vitro experiments to investigate the formation of osteoclasts using wild-type (WT) bone marrow macrophages (BMMs), recombinant soluble RANKL (sRANKL), and murine CSF1. The results demonstrated that the differentiation of osteoclasts under physiological conditions led to transient induction of ER stress (17). To further explore this phenomenon, the authors employed siRNA-mediated gene silencing to inhibit the IRE1α/XBP1 pathway in WT BMMs and conducted in vitro assays to assess osteoclast formation in these cells. The experimental results revealed that the IRE1α/XBP1 pathway plays a critical role in the regulation of osteoclast differentiation and that the termination of this pathway significantly inhibited osteoclast formation in vitro (17, 31).
The researchers discovered that the involvement of IRE1α/XBP1 in osteoclast formation can be attributed to a series of processes. The activation of IRE1α, triggered by ER stress induced by RANKL/RANK, leads to the splicing of XBP1u into mature XBP1. Notably, the Nfatc1 plays a crucial role in regulating osteoclast formation, and its promoter region contains potential binding sites for XBP1. Consequently, the direct binding of mature XBP1 to these sites stimulates the transcription of Nfatc1, thereby facilitating the promotion of osteoclast formation (Figure 2). Moreover, it was observed that ER stress was only transiently induced following RANKL stimulation, indicating that the role of the UPR in maintaining mature osteoclast function is limited (17, 32).
Figure 2 Regulation of XBP1 in Osteoclast Differentiation. RANKL/RANK can trigger endoplasmic reticulum stress (ER stress), which activates three typical branches of the unfolded protein response: PERK-eIF2α, ATF6, and IRE1α. Among them, IRE1α/XBP1 is the most evolutionarily conserved pathway, and IRE1α can splice Xbp1u into mature Xbp1. XBP1 can directly bind to the binding sites of NFATc1, a major regulator associated with osteoclast formation, to induce transcription of Nfatc1, thereby promoting osteoclast formation. In addition, XBP1 can also bind directly to the promoter of Pth1r to positively regulate PTH/PTHrP expression and participate in PTH-induced RANKL expression, which interacts with RANK, thereby inducing differentiation of osteoclast precursor into osteoclast.
Additionally, a substantial body of evidence has demonstrated that BMMs lacking IRE1α exhibit impaired ability to induce Nfatc1 after RANKL stimulation (17). Furthermore, in a study conducted by Lavinia Raimondi et al., pre-osteoclast cells (pOCs) were treated with MM cell-derived small extracellular vesicles (MM-EVs), revealing an increased expression of XBP1 in pOCs after 3 days of treatment with MM-EVs compared to untreated cells. Subsequently, the researchers proceeded to suppress the IRE1α/XBP1 pathway by employing a specific inhibitor of IRE1α. Remarkably, the application of MM-EVs in conjunction with the IRE1 inhibitor led to a significant decrease in the quantity of osteoclasts within Raw264.7 cells (33). These findings serve to further validate the significance of the IRE1α/XBP1 pathway in the process of osteoclast formation.
Furthermore, it has been postulated that parathyroid hormone (PTH) and parathyroid hormone-related protein (PTHrP) exert pivotal regulatory functions in bone development and homeostasis. The transcription of the PTH/PTH-related peptide receptor (PTH1R) is regulated by the IRE1α/XBP1 pathway, as evidenced by the direct interaction of XBP1 with the P2 promoter of Pth1r. Consequently, the IRE1α/XBP1 pathway exerts a positive influence on the expression of the PTH/PTHrP pathway, ultimately facilitating the upregulation of PTH-induced RANKL expression in osteoblasts. This, in turn, leads to the activation of osteoclastogenesis through the interaction between RANKL and RANK on osteoclasts (8, 34).
Osterix (Osx) is a novel zinc finger-containing transcription factor specifically expressed in all growing bones. It is viewed as a transcription factor essential for osteoblast differentiation (35). Without Osx, there is no cortical and trabecular bone formation via intramembranous or intracartilaginous osteogenesis. In the progression of osteoblast differentiation, osteoblast progenitors in intrachondral and interstitial cohesion of membranous bone elements differentiate into preosteoblasts through regulation by Runx2/Cbfa1, which in turn differentiate into mature osteoblasts in one or more steps and express characteristic osteoblast marker genes, a process that requires Osx (36, 37).
Takahide Tohmonda et al. discovered through several in vitro experiments that the osteoblast-specific transcription factor Osx is the direct target of XBP1, not Runx2. Researchers cultured multipotent, uncommitted MEFs and committed preosteoblasts in vitro and found that XBP1 regulates Osx expression by binding directly to the XBP1 binding site in the Osx promoter (38). Besides, using UPR signaling released by thapsigargin, a potent inducer of ER stress, could promote Osx transcription in BMP2-treated fibroblasts and osteoblast cell line MC3T3-E1, but this process was dependent on IRE1α/XBP1 (19). In addition, it has been shown that mutant reporter constructs analysis and a luciferase assay, based on data from the promoter region of Osx encoding a UPR element (TGACGTGG/A)-like sequence (TGAGCTGG) (39)and XBP1-binding sequence (ACGT) (40), reveals that the IRE1α/XBP1 pathway stimulates Osx expression and thus promotes the differentiation of preosteoblasts into osteoblasts (19).
Over the past decade, the medical community has introduced several new drugs for treating MM, of which proteasome inhibition (PI) is the most important class. PI has been shown to promote bone formation in vivo and vitro (41). Three PIs have been approved for the treatment of MM: bortezomib, carfilzomib, and ixazomib (42), which can reduce bone resorption and increase bone formation in MM patients (43, 44). Studies have shown that bortezomib counteracts the osteoclast regulators, RANKL and OPG, leading to osteoclast inhibition and reduced bone destruction. It also stimulates osteoblast differentiation, as evidenced by elevated bone-specific alkaline phosphatase (BSP) and osteocalcin (OCN) (45).
In vitro studies have provided evidence that myeloma cells possess the ability to hinder the differentiation of osteoblast precursors and trigger apoptosis in fully developed osteoblasts (46). A particular study demonstrated that the administration of bortezomib resulted in the expression of markers associated with osteogenesis, as observed through the assessment of calcium deposition in bortezomib-treated mouse BMSCs using Alizarin Red S (ARS) staining (47). Recent investigations involving ARS staining and Western blot analysis of IRE1α inhibitor-treated bortezomib-induced MSCs and MC3T3-E1 cells have indicated that the inhibition of XBP1 disrupts the osteogenic differentiation process induced by bortezomib (44). Moreover, the study revealed that the upregulation of XBP1 in MSCs and MC3T3-E1 cells through the utilization of Tet-On-inducible lentiviral vectors substantially augmented the expression of molecules associated with osteogenesis (48). Additionally, the authors observed that the inhibition of IRE1α/XBP1 effectively counteracted the bortezomib-induced bone formation in mice, as evidenced by micro CT analysis. These findings provide compelling evidence for the pivotal role of the XBP1 signaling pathway in bone formation induced by PI (44).
Periodontal ligament cells (PDLCs) are the cytological basis for periodontal tissue damage repair and regeneration (49), and their osteogenic potential is particularly critical. Disturbances in tissue homeostasis can initiate the osteogenic differentiation of PDLCs. RT-PCR of osteogenesis-induced PDLCs revealed that PDLCs undergo upregulation of ER stress levels during the osteogenic differentiation process and that XBP1 plays a role in it (50, 51).
BMP2 is a highly conserved functional protein of the TGF-β family, promoting osteogenesis (52, 53). It was shown by immunofluorescence that XBP1 highly expresses in human periodontal ligament cells (hPDLCs). At the same time, BMP2 could co-localize with XBP1 in hPDLCs and jointly improve the osteogenic ability of hPDLCs, suggesting that XBP1 has a positive role in the proliferation and osteogenesis of hPDLCs XBP1 have a positive role in both proliferation and osteogenesis of hPDLCs (54).
Lipopolysaccharide (LPS), a potent toll-like receptors (TLR) 4 agonist (55), has been shown to induce activation of the UPR in hPDLCs. When the UPR is activated, PERK, ATF6, and IRE1 are released from GRP78 to transduce the signal through the ER membrane to the cytoplasm and nucleus. Many studies have shown that LPS induces GRP78 expression in various cell types (56), activating the UPR. A previous study showed that qrt-PCR analysis of human periodontal ligament fibroblasts (hPDLFs) treated with LPS showed that LPS increased the expression of XBP1 mRNA level and promoted the splicing and activation of XBP1 in hPDLFs, thus participating in the regulation of the alveolar bone formation process (57).
It has also been shown in the literature that XBP1 is involved in a range of inflammation-related diseases by acting as a target gene for specific miRNAs. Exo-miR-205-5p inhibits inflammation in chronic periodontitis by targeting XBP1 (58). Whether it promotes osteoblast differentiation has not been reported.
Bone and immune cells co-exist in the bone marrow cavity and share a common progenitor cell and a diversity of regulatory molecules. Immune cells regulate bone homeostasis, and bone cells, in turn, influence the proliferation and differentiation of immune cells (59). Tissue injury triggers an immediate immune response, and the initial immune response consists primarily of the innate immune system, including neutrophils, mast cells, monocytes, and macrophages. The later immune response consists primarily of the adaptive immune system, including T cells and B cells. Immune cells have two main effects on bone regeneration: the anabolic function of acute inflammation and the catabolic function of chronic inflammation (60). The following focuses on the regulatory role of XBP1 in several common immune cells.
Macrophages are innate immune cells in almost all tissues and play a key role in maintaining normal tissue homeostasis. During acute inflammation, macrophages promote the restoration of tissue homeostasis by phagocytosing invading microorganisms, amplifying the inflammatory response, and recruiting extra immune cells (61). When tissue damage is cleared, macrophages promote tissue regeneration by secreting anti-inflammatory factors, recruiting progenitor cells, and producing growth factors. This functional plasticity of macrophages is defined as macrophage polarization (62). Macrophages during inflammation are referred to as M1-type macrophages, whereas macrophages active in tissue regeneration are M2-type macrophages (29, 63). Numerous studies have demonstrated the critical role of macrophages in abnormal bone metabolism.
T cells belong to the adaptive immune system with great specificity and immune memory for their targets (64). T cells are divided into two categories according to their T cell receptors: αβ T cells and γδ T cells. αβ T cells are subdivided into CD4-positive (CD4+) and CD8-positive (CD8+) T cells. CD4+ T cells, also known as T helper (Th) cells, are divided into several subpopulations, including Th1, Th2, Th9, Th17, Th22, and follicular helper T cells (Tfh) and Tregs, which can be indirectly involved in clearing infections by regulating the activity of other immune cells (65). While CD8+ T cells are known as cytotoxic T lymphocytes (CTLs), CTLs target virus-infected cells to induce their death (64), both of which have dual functions of promoting and inhibiting regeneration. γδ T cells, a small subpopulation of T cells (66), are thought to have a regeneration-promoting role, but their mechanisms are unknown. Thus, the regulatory effect of T cells in bone regeneration may be associated with T cell subsets, cytokines, and other agents (60).
B cells belong to the adaptive immune system and are characterized by their ability to differentiate into plasma cells to produce antibodies. These antibodies permeate extracellular spaces, where they protect against infection (67). After their generation, B cells assume various functions in immune regulation and host defense (68). The development of B cells occurs in the bone marrow (BM) from hematopoietic stem cells (HSCs), progressing through a sequence of stages involving common lymphoid progenitors (CLP), progenitor (pro), precursor (pre), and immature B cells. Subsequently, their migration from the BM is triggered, and upon activation, B lymphocytes can either become terminal B cells that secrete Ig-secreting PCs or antigen-specific memory B cells that may eventually reenter the BM (69). There is a large body of literature that shows that B lymphocytes, through the secretion of inflammatory cytokines, matrix metalloproteinases, and RANKL, as well as the production of pathogenic autoantibodies, play a skeletal disease role (70).
TLRs are a class of transmembrane proteins with a type I membrane structure, characterized by an ectodomain comprised of leucine-rich repeat sequences (LRRs) responsible for the recognition of pathogen-associated molecular patterns (PAMPs) (71, 72). In a scholarly study, the utilization of a Phos-tag reagent, which selectively binds to phosphorylated amino acid residues, was employed to investigate the activation of mouse J774 macrophages cultured in polyacrylamide gels containing Phos-tag acrylamide upon stimulation with the TLR agonist LPS. The presence of IRE1α was detected in these stimulated macrophages. Additionally, the activation of TLRs was also observed in primary macrophages derived from WT mice (C3H/HeOuJ) under LPS stimulation, as evidenced by the presence of TLR-activated XBP1 (20). XBP1 has been observed to fulfill a positive feedback function in macrophages, thereby preserving the integrity of the TLR pathway. Furthermore, the absence of Xbp1 in mice resulted in impaired secretion of inflammatory mediators, as evidenced by ELISA and RT-PCR assays conducted on TLR4 or TLR2 agonist-stimulated macrophages. This impairment serves as a demonstration of XBP1’s role in augmenting the production of pro-inflammatory cytokines through TLR activation. In line with this discovery, the activation of XBP1 was also found to synergistically enhance the regulation of cytokines by TLR in M1-type macrophages (20) (Figure 3).
Figure 3 Regulatory role of XBP1 in macrophages. In macrophages, XBP1 can be activated by toll-like receptor (TLR) and maintain the TLR pathway. Second, XBP1 can be activated by Annexin A2 (AnxA2), which is involved in inflammatory responses, and AnxA2 can also inhibit XBP1 maturation through the p38 MAPK pathway. In addition, XBP1 can be activated by NF-κB pathway, and in turn, XBP1 can enhance the expression, phosphorylation, and nuclear transport of NF-κB pathway. These processes can increase the formation of pro-inflammatory cytokines, which could potentially be involved in bone resorption. In contrast, XBP1 can also be activated by estrogen to increase anti-inflammatory cytokines and inhibit the expression of pro-inflammatory cytokines, which may ultimately play an essential role in bone formation.
Annexin A2 (AnxA2) is a multicompartmental compartmentalized protein. It is thought to mediate the linker protein of the membrane-associated protein complex to regulate the recruitment of leukocytes and the release of inflammatory mediators (73, 74). Currently, a large body of literature shows that AnxA2 is associated with ER stress and is located upstream of XBP1 involved in the inflammatory response (75). Some scholars have used siRNA technology to silence AnxA2, and transcript and protein levels of XBP1 were significantly reduced in mouse alveolar macrophage cell line (MH-S) and THP-1 cells with silenced AnxA2 after P.aeruginosa infection, indicating that AnxA2 deletion can impair P.aeruginosa-induced macrophage ER stress. Original studies have shown that AnxA2 promotes the production of pro-inflammatory cytokines (76) and may play a role in M1-type macrophages. Moreover, overexpression of XBP1 in MH-S stimulated the transcription of TNFα, IL-1β, and IL-6 in response to P.aeruginosa infection, suggesting that XBP1 could stimulate the transcription of pro-inflammatory cytokines. However, by measuring the levels of p38 and phospho-p38, it was found that AnxA2 could inhibit the maturation of XBP1 through the p38 MAPK pathway, thereby regulating the formation of inflammatory cytokines (75).
The nuclear factor NF-κB pathway has long been considered the classic pro-inflammatory signaling pathway, mainly based on the role of NF-κB in the expression of pro-inflammatory genes, including cytokines, chemokines, and adhesion molecules (77). NF-κB dimers can bind to related target DNA sequences, called κB sites, to regulate gene expression (78). A large body of literature shows that NF-κB is associated with ER stress (79). XBP1 expression was downregulated in MH-S cells using siRNA technology. qPCR, immunoblot analysis, and imaging analysis showed that XBP1 enhanced NF-κB pathway’s expression, phosphorylation, and nuclear translocation. At the same time, this pathway can enhance the formation of pro-inflammatory cytokines (75).
In contrast to the aforementioned findings, evidence suggests that the activation of IRE1α/XBP1 signaling by estrogen can impede M1-type macrophage polarization and potentially contribute to the anti-inflammatory mechanism. Estrogen, classified as a steroid hormone, assumes a crucial function in preserving immune homeostasis within the body (80). Predominantly present as estradiol (81), in vivo studies have demonstrated that estrogen can modulate the proliferation and differentiation of immune cells through its interaction with estrogen receptors located on these cells (82). Several researchers have administered estrogen stimulation exclusively to mouse macrophages and observed an upregulation in the expression of anti-inflammatory molecules, such as TGF-β and IL-10. Additionally, the activation of IRE1α signaling was significantly observed in macrophages following estrogen treatment, and this activation was hindered by the introduction of estrogen receptor antagonists. However, when both estrogen and IRE1α inhibitors were concurrently present, an upregulation in the expression of pro-inflammatory cytokines, including TNF-α and IL-6, was demonstrated. This experiment demonstrates that estrogen inhibits M1-type macrophage polarization by activating IRE1α/XBP1 signaling (29), thereby suppressing the inflammatory response.
Activated and differentiated helper T cells can secrete a large number of cytokines. It has been shown that after treatment with LPS and cecal ligation and puncture (CLP) in vivo and in vitro sepsis models, respectively, the ER stress levels and downstream markers increased in CD4+ T cells. At the same time, apoptosis of CD4+ T cells was significantly increased. This phenomenon was improved with the application of ER stress inhibitors (83). In addition, measuring the expression of IRE1α mRNA and protein in differentiated and reactivated Th2 cells in vitro using qPCR and Western blot showed that the IRE1α/XBP1 pathway is upregulated during helper T cell activation in vitro. Meanwhile, infecting C57BL/6 mice with the parasite Nippostrongylus brasiliensis demonstrated that the pathway is also active in vivo (84). Analysis of blood samples from patients with allergic rhinitis revealed that XBP1 might play an essential role in the development of Th2 polarization.
Cholesterol is a key constituent of membrane lipids and plasma compartments that can play a role in the anti-tumor response of T cells (85). It has been recently shown that microarray analysis of CD8+ T cells under different cholesterol treatment conditions revealed that XBP1 was highly upregulated in cholesterol-treated CD8+ T cells. Meanwhile, in vivo and in vitro experiments revealed that knocking down XBP1 significantly downregulated Pdcd1 mRNA expression in CD8+ T cells. Moreover, in the group without knockdown of XBP1 expression, cholesterol treatment increased PD-1 expression, thus demonstrating that XBP1 is an important regulator of cholesterol-induced CD8+ T cell inhibitory receptor expression and T cell exhaustion. T cell exhaustion is characterized by impaired effector cell function, sustained expression of inhibitory receptors, and a distinct transcriptional profile compared to functional effector cells or memory T cells (86). Both extrinsic negative regulatory pathways, including immunomodulatory cytokines, and intrinsic negative regulatory pathways play a crucial role in the development of exhaustion. Through the ChIP assay, the researchers discovered that cholesterol can stimulate the expression of XBP1, which subsequently modulates the expression of inhibitory receptors on CD8+ T cells, such as PD-1, and induces T cell exhaustion (87). It has been reported that advanced T-cell failure is associated with loss of cytotoxicity in CD4+ and CD8+ T cell subsets that produce IFN-γ, and early in vitro, studies have shown that IFN-γ directly inhibits myeloma cell growth, and preclinical models support a positive role for CD8+ T-cell failure in myeloma progression (88) (Figure 4).
Figure 4 XBP1 is upregulated in CD8+ T cells. Cholesterol can induce high expression of XBP1 on CD8+ T cells, which in turn regulates the expression of the inhibitory receptor PD-1 on CD8+ T cells and induces T cell depletion.CD8+ T cells can produce IFN-γ, which directly inhibits the growth of myeloma cell. And myeloma cells can express PD-L1 (ligand for the T-cell immunoreceptor PD-1), which can directly lead to T-cell exhaustion, which may play a key role in bone metabolism. SIRT7 also promotes the expression of XBP1 in CD8+ T-cells, which is involved in bone metabolism.
Sirtuins are a family of deacetylases that sense nutrients and regulate cellular homeostasis (89), of which SIRT7 is localized only in the nucleus and is associated with the maintenance of cellular homeostasis and adaptation to exogenous stress (90). Researchers found that knockdown SIRT7 significantly attenuated the expression of Tunicamycin (TM), an ER stress inducer, induced by these molecules. At the same time, overexpression of SIRT7 enhanced the upregulation of these molecules. Western blot and immunohistochemical staining analysis also demonstrated that SIRT7 could selectively activate the IRE1α/XBP1 branch (91). SIRT7 can promote the secretion of IRE1α-dependent tumor-promoting factors and alter the tumor immune microenvironment, thereby mediating tumorigenesis. It has been documented that SIRT7 is associated with the bone remodeling process (92).
There are two distinct subpopulations of mature B cells, namely B-1 and B-2. B-1 B cells are predominantly located in the peritoneal cavity and are responsible for producing systemic natural IgM. Conversely, B-2 cells primarily participate in conventional T-cell-dependent IgM and IgG responses in peripheral lymphoid organs, but they also have the capability to migrate to the intestinal lamina propria and generate IgA. The activation of B cells into antibody-secreting PCs depends on the nature of the antigen, which can occur through either a T-cell-independent (TI) or a T-cell-dependent (TD) mechanism (93).
XBP1 is of significant importance in the differentiation of PCs and the secretion of Igs. Substantial research has consistently demonstrated the elevated expression of XBP1 in PCs, while the absence of XBP1 in mice results in the absence of PCs (93). Additionally, during the transition from B cells to PCs, there is a specific activation of the IRE1α and ATF6 branches of the UPR (94). These findings undeniably establish the essential role of XBP1 in PC differentiation. The differentiation process of B cells into PCs is governed by two transcription factors, namely B lymphocyteeinduced maturation protein 1 (BLIMP1) and XBP1. During the progression of PC differentiation, XBP1 acts as a regulatory factor downstream of Blimp-1 (95). Previous studies have demonstrated a significant decrease in mRNA and protein levels of XBP1, ATF4, and ATF6 in bone marrow PCs upon the inactivation of Prdm1, which encodes BLIMP1. Additionally, a ChIPseq assay conducted on in vitro-generated plasmablasts revealed that Blimp1 has the ability to activate ATF6, a known inducer of XBP1 (39). On the other hand, it can promote the formation of the active mature form of XBP1 by directly regulating Ern1, which encodes IRE1 (96).
In bone metabolism, B lymphocytes and PCs play a significant role by producing various cytokines and chemokines. These molecules have the ability to directly influence osteoblasts and also contribute to the regulation of bone remodeling and regeneration by modulating the bone microenvironment (69). Existing literature has provided evidence that both B cells and osteoblasts are capable of secreting OPG, which acts by inhibiting osteoclastogenesis through the blockade of the RANKL/RANK interaction. Among these cell types, PCs are particularly noteworthy as they are the most potent producers of OPG (30). The significance of OPG secretion by B cells in maintaining bone homeostasis was clearly demonstrated through studies involving mice with B-cell deficiency and osteoporosis (97). However, it has been reported that B cells and B cell-derived PCs in MM possess the ability to facilitate osteoclastogenesis, potentially attributed to the direct osteoclastogenic impact of RANKL expression (98–100) or the indirect promotion of bone resorption through IL-7 secretion (101, 102). Furthermore, B lymphocytes that have been pretreated with LPS hinder osteoclastogenesis in rat BMSCs by activating Notch signaling (69). It is evident that B lymphocytes exhibit diverse and sometimes conflicting influences on bone metabolism, contingent upon the specific physiological and pathological situation.
In the context of bone metabolism, the UPR is initiated by the accumulation of misfolded proteins within the ER. Among the UPR pathways, the IRE1α/XBP1 is highly conserved. XBP1, a key component of this pathway, plays a critical regulatory role in bone metabolism. This article comprehensively elucidates the various roles of XBP1 in bone metabolism. Specifically, the involvement of XBP1 in osteoclast and osteoblast differentiation is now better understood. Notably, XBP1 has been shown to enhance osteoclastogenesis by binding to Nfatc1 in response to stimulation by RANKL/RANK. Additionally, XBP1 has the potential to indirectly enhance osteoclastogenesis by modulating the expression of RANKL on osteoblasts through positive regulation of PTH/PTHrP pathway. Moreover, XBP1 can facilitate osteoblast differentiation by promoting the expression of Osx. Furthermore, XBP1 can be activated by PI and actively participate in the process of bone formation. Notably, XBP1 may also exert its influence on periodontal tissues.
Interestingly, the regulatory effects of XBP1 on immune cells are multiple and often contradictory. In macrophages, XBP1 serves a dual function by facilitating the generation of pro-inflammatory cytokines and promoting macrophage polarization towards the M1 phenotype. Conversely, estrogen has the ability to activate XBP1, thereby inhibiting M1-type macrophage polarization. The impact of Th1 and Th2 cells on bone metabolism remains a subject of debate within the academic community. On one hand, Th1 cells have the capacity to activate osteoclasts, resulting in bone degradation. Conversely, both Th1 and Th2 cells produce a diverse array of cytokines that possess the ability to impede osteoclast differentiation, consequently promoting bone formation. Th17 cells have the ability to secrete active RANKL, thereby directly facilitating bone resorption. Considering the significant expression of XBP1 in T cells and its crucial involvement in bone metabolism, it is plausible to speculate that XBP1 may impact bone metabolism by influencing Th cells. Furthermore, XBP1 can modulate T cell dysfunction by regulating the expression of inhibitory receptors in CD8+ T cells and can be activated by SIRT7, potentially contributing to bone metabolism. In PCs, the expression of XBP1 is notably high. PCs have the capacity to significantly impact bone metabolism, both in terms of bone homeostasis and their potential to facilitate osteoclastogenesis. However, the precise molecular mechanism by which XBP1 regulates the aforementioned processes remains unclear.
In conclusion, further research is required to investigate: 1) the specific conditions that determine whether XBP1 activates osteoclast differentiation or osteoblast differentiation, and 2) the specific molecular mechanisms through which XBP1 regulates the differentiation of osteoblasts and osteoclasts by modulating immune cells. The current understanding of the relationship between XBP1 and bone metabolism is still limited. Exploring and comprehending the specific molecular mechanisms through which immune cells regulate bone metabolism via XBP1 could potentially complement and enhance therapeutic approaches and effectiveness in treating bone-related diseases. Consequently, investigating the regulatory function of XBP1 in bone metabolism may yield novel insights for clinical applications in this field.
NM and LZ made significant contributions to the conception and design of this article. WL, ZZ, and JJ contributed to the drafting of the article. YZ, YF and TX revised the manuscript. All authors have read and agreed to the published version of the manuscript.
This work was funded by the Science and Technology Research Project of Jilin Provincial Education Department (JJKH20221095KJ), the Key Discipline Construction Project of Tianjin Stomatological Hospital (No. 2022P01), Tianjin High-level Talents Development Program for the Health Fields (No. TJSQNYXXR-D2-114), and Tianjin Health Research Project (No. TJWJ2023MS034).
The authors declare that the research was conducted in the absence of any commercial or financial relationships that could be construed as a potential conflict of interest.
All claims expressed in this article are solely those of the authors and do not necessarily represent those of their affiliated organizations, or those of the publisher, the editors and the reviewers. Any product that may be evaluated in this article, or claim that may be made by its manufacturer, is not guaranteed or endorsed by the publisher.
1. Suda T, Takahashi. N, Udagawa N, Jimi E, Gillespie MT, Martin TJ. Modulation of osteoclast differentiation and function by the new members of the tumor necrosis factor receptor and ligand families. Endocr Rev (1999) 20(3):345–57. doi: 10.1210/edrv.20.3.0367
2. Teitelbaum. SL. Bone resorption by osteoclasts. Science (2000) 289:1504–8. doi: 10.1126/science.289.5484.1504
3. Doherty AH, Ghalambor CK, Donahue SW. Evolutionary physiology of bone: bone metabolism in changing environments. Physiol (Bethesda) (2015) 30:17–29. doi: 10.1152/physiol.00022.2014
4. Boyle WJ, Simonet. WS, Lacey DL. Osteoclast differentiation and activation. Nature (2003) 423:337–42. doi: 10.1038/nature01658
5. Cowan AJ, Green DJ, Kwok M, Lee S, Coffey DG, Holmberg LA, et al. Diagnosis and management of multiple myeloma: A review. Jama (2022) 327:464–77. doi: 10.1001/jama.2022.0003
6. Fischer V, Haffner-Luntzer M. Interaction between bone and immune cells: implications for postmenopausal osteoporosis. Semin Cell Dev Biol (2022) 123:14–21. doi: 10.1016/j.semcdb.2021.05.014
7. Komatsu N, Takayanagi H. Mechanisms of joint destruction in rheumatoid arthritis - immune cell-fibroblast-bone interactions. Nat Rev Rheumatol (2022) 18:415–29. doi: 10.1038/s41584-022-00793-5
8. Tohmonda T, Yoda M, Mizuochi H, Morioka H, Matsumoto M, Urano F, et al. The Ire1alpha-Xbp1 pathway positively regulates parathyroid hormone (Pth)/Pth-related peptide receptor expression and is involved in Pth-induced osteoclastogenesis. J Biol Chem (2013) 288:1691–5. doi: 10.1074/jbc.C112.424606
9. Oakes SA, Papa FR. The role of endoplasmic reticulum stress in human pathology. Annu Rev Pathol (2015) 10:173–94. doi: 10.1146/annurev-pathol-012513-104649
10. Chen Y, Brandizzi F. Ire1:Er stress sensor and cell fate executor. Trends Cell Biol (2013) 23:547–55. doi: 10.1016/j.tcb.2013.06.005
11. Li Y, Jiang W, Niu Q, Sun Y, Meng C, Tan L, et al. Eif2alpha-Chop-Bcl-2/Jnk and Ire1alpha-Xbp1/Jnk signaling promote apoptosis and inflammation and support the proliferation of Newcastle disease virus. Cell Death Dis (2019) 10:891. doi: 10.1038/s41419-019-2128-6
12. Maurel M, Chevet E, Tavernier J, Gerlo S. Getting ridd of Rna: Ire1 in cell fate regulation. Trends Biochem Sci (2014) 39:245–54. doi: 10.1016/j.tibs.2014.02.008
13. Zou J, Fei Q, Xiao H, Wang H, Liu K, Liu M, et al. Vegf-A promotes angiogenesis after acute myocardial infarction through increasing Ros production and enhancing Er stress-mediated autophagy. J Cell Physiol (2019) 234:17690–703. doi: 10.1002/jcp.28395
14. Chen S, Chen J, Hua X, Sun Y, Cui R, Sha J, et al. The emerging role of Xbp1 in cancer. BioMed Pharmacother (2020) 127:110069. doi: 10.1016/j.biopha.2020.110069
15. Pan Y, Liu. K, Ye Y, Fan M, Li X, Gou. F. Perspective study of upr signaling molecules as potential biomarkers in bone metabolism. Cell Signal (2023) 1(1):14–21.
16. Xu G, Liu K, Anderson J, Patrene K, Lentzsch S, Roodman GD, et al. Expression of Xbp1s in bone marrow stromal cells is critical for myeloma cell growth and osteoclast formation. Blood (2012) 119:4205–14. doi: 10.1182/blood-2011-05-353300
17. Tohmonda T, Yoda M, Iwawaki T, Matsumoto M, Nakamura M, Mikoshiba K, et al. Ire1alpha/Xbp1-mediated branch of the unfolded protein response regulates osteoclastogenesis. J Clin Invest (2015) 125:3269–79. doi: 10.1172/JCI76765
18. Huang W, Gong Y, Yan L. Er stress, the unfolded protein response and osteoclastogenesis: A review. Biomolecules (2023) 13:1050–68. doi: 10.3390/biom13071050
19. Tohmonda T, Miyauchi Y, Ghosh R, Yoda M, Uchikawa S, Takito J, et al. The Ire1alpha-Xbp1 pathway is essential for osteoblast differentiation through promoting transcription of osterix. EMBO Rep (2011) 12:451–7. doi: 10.1038/embor.2011.34
20. Martinon F, Chen X, Lee AH, Glimcher LH. Tlr activation of the transcription factor Xbp1 regulates innate immune responses in macrophages. Nat Immunol (2010) 11:411–8. doi: 10.1038/ni.1857
21. Harnoss JM, Le Thomas A, Shemorry A, Marsters SA, Lawrence DA, Lu M, et al. Disruption of Ire1alpha through its kinase domain attenuates multiple myeloma. Proc Natl Acad Sci U.S.A. (2019) 116:16420–9. doi: 10.1073/pnas.1906999116
22. Liou HC, Boothby. M, Finn PW, Davidon R, Nabavi N, Zeleznik-Le NJ, et al. A new member of the leucine zipper class of proteins that binds to the Hla Drα Promoter. Science (1990) 247(4950):1581–4. doi: 10.1126/science.2321018
23. Glimcher LH. Xbp1: the last two decades. Ann Rheum Dis (2010) 69 Suppl 1:I67–71. doi: 10.1136/ard.2009.119388
24. Read A, Schroder M. The unfolded protein response: an overview. Biol (Basel) (2021) 10:384–93. doi: 10.3390/biology10050384
25. Quwaider D, Corchete LA, Martin-Izquierdo M, Hernandez-Sanchez JM, Rojas EA, Cardona-Benavides IJ, et al. Rna sequencing identifies novel regulated Ire1-dependent decay targets that affect multiple myeloma survival and proliferation. Exp Hematol Oncol (2022) 11:18. doi: 10.1186/s40164-022-00271-4
26. Wohner M, Pinter T, Bonelt P, Hagelkruys A, Kostanova-Poliakova D, Stadlmann J, et al. The Xbp1-regulated transcription factor Mist1 restricts antibody secretion by restraining Blimp1 expression in plasma cells. Front Immunol (2022) 13:859598. doi: 10.3389/fimmu.2022.859598
27. Zambelli A, Mongiardini E, Villegas SN, Carri NG, Boot-Handford RP, Wallis GA. Transcription factor Xbp-1 is expressed during osteoblast differentiation and is transcriptionally regulated by parathyroid hormone (Pth). Cell Biol Int (2005) 29:647–53. doi: 10.1016/j.cellbi.2005.03.018
28. Shen Yu-Ting SY, Chen L-H. (2021). The Ire1α-Xbp1 pathway in endoplasmic reticulum stress is involved in the development of renal fibrosis by promoting the activation of M1-type macrophages. in: 14th Annual Meeting Of Chinese Society For Immunology, Chengdu, Sichuan Province, China.
29. Zhuo L, Wang S, Liu X, Chen B, Li X. Estradiol inhibits differentiation of mouse macrophage into A pro-inflammatory phenotype by upregulating the Ire1alpha-Xbp1 signaling axis. Nan Fang Yi Ke Da Xue Xue Bao (2022) 42:432–7. doi: 10.12122/j.issn.1673-4254.2022.03.17
30. Konnecke I, Serra A, El Khassawna T, Schlundt C, Schell H, Hauser A, et al. T and B cells participate in bone repair by infiltrating the fracture callus in A two-wave fashion. Bone (2014) 64:155–65. doi: 10.1016/j.bone.2014.03.052
31. Lee W-S, Jeong J-H, Lee E-G, Choi Y, Kim J-H, Kim H-R, et al. Tacrolimus regulates endoplasmic reticulum stress-mediated osteoclastogenesis and inflammation: in vitro and collagen-induced arthritis mouse model. Cell Biol Int (2018) 42:393–402. doi: 10.1002/cbin.10861
32. Iyer S, Adams DJ. Bone and the unfolded protein response: in sickness and in health. Calcified Tissue Int (2023) 113:96–109. doi: 10.1007/s00223-023-01096-x
33. Raimondi L, De Luca A, Fontana S, Amodio N, Costa V, Carina V, et al. Multiple myeloma-derived extracellular vesicles induce osteoclastogenesis through the activation of the Xbp1/Ire1α Axis. Cancers (2020) 12:2167–83. doi: 10.3390/cancers12082167
34. Dominicus CS, Patel V, Chambers JE, Malzer E, Marciniak SJ. Endoplasmic Reticulum Stress Signalling During Development. In: CLARKE, Clarke R. (ed.) The Unfolded Protein Response in Cancer. Switzerland: Humana Press (2019) 17–47. doi: 10.1007/978-3-030-05067-2_2
35. Liu Q, Li M, Wang S, Xiao Z, Xiong Y, Wang G. Recent advances of osterix transcription factor in osteoblast differentiation and bone formation. Front Cell Dev Biol (2020) 8:601224. doi: 10.3389/fcell.2020.601224
36. Nakashima K ZX, Kunkel G, Zhang Z, Deng JM, Behringer RR, De Crombrugghe B. The novel zinc finger-Containing transcription factor osterix is required for osteoblast differentiation and bone formation. Cell (2002) 108(1):17–29. doi: 10.1016/S0092-8674(01)00622-5
37. Amarasekara DS, Kim S, Rho J. Regulation of osteoblast differentiation by cytokine networks. Int J Mol Sci (2021) 22:2851–66. doi: 10.3390/ijms22062851
39. Yoshida H, Matsui. T, Yamamoto A, Okada T, Mori K. Xbp1 Mrna is induced by Atf6 and spliced by ire1 in response to Er stress to produce A highly active transcription factor. Cell (2001) 107(7):881–91. doi: 10.1016/S0092-8674(01)00611-0
40. Acosta-Alvear D, Zhou Y, Blais A, Tsikitis M, Lents NH, Arias C, et al. Xbp1 controls diverse cell type- and condition-specific transcriptional regulatory networks. Mol Cell (2007) 27:53–66. doi: 10.1016/j.molcel.2007.06.011
41. Giuliani N, Morandi F, Tagliaferri S, Lazzaretti M, Bonomini S, Crugnola M, et al. The proteasome inhibitor bortezomib affects osteoblast differentiation in vitro and in vivo in multiple myeloma patients. Blood (2007) 110:334–8. doi: 10.1182/blood-2006-11-059188
42. Fricker LD. Proteasome inhibitor drugs. Annu Rev Pharmacol Toxicol (2020) 60:457–76. doi: 10.1146/annurev-pharmtox-010919-023603
43. Gentile M OM, Vigna E, Corvatta L, Recchia AG, Morabito L, Morabito F, et al. Ixazomib for the treatment of multiple myeloma. Expert Opin Investig Drugs (2015) 24(9):1287–98. doi: 10.1517/13543784.2015.1065250
44. Zhang D, De Veirman K, Fan R, Jian Q, Zhang Y, Lei L, et al. Er stress arm Xbp1s plays A pivotal role in proteasome inhibition-induced bone formation. Stem Cell Res Ther (2020) 11:516. doi: 10.1186/s13287-020-02037-3
45. Terpos E, Sezer O, Croucher P, Dimopoulos MA. Myeloma bone disease and proteasome inhibition therapies. Blood (2007) 110:1098–104. doi: 10.1182/blood-2007-03-067710
46. Giuliani N, Rizzoli V, Roodman GD. Multiple myeloma bone disease: pathophysiology of osteoblast inhibition. Blood (2006) 108:3992–6. doi: 10.1182/blood-2006-05-026112
47. Zhang D, Fan R, Lei L, Lei L, Wang Y, Lv N, et al. Cell cycle exit during bortezomib-induced osteogenic differentiation of mesenchymal stem cells was mediated by Xbp1s-upregulated P21(Cip1) and P27(Kip1). J Cell Mol Med (2020) 24:9428–38. doi: 10.1111/jcmm.15605
48. Yang SY, Wei FL, Hu LH, Wang CL. Perk-Eif2alpha-Atf4 pathway mediated by endoplasmic reticulum stress response is involved in osteodifferentiation of human periodontal ligament cells under cyclic mechanical force. Cell Signal (2016) 28:880–6. doi: 10.1016/j.cellsig.2016.04.003
49. Gay IC, Chen S, Macdougall M. Isolation and characterization of multipotent human periodontal ligament stem cells. Orthod Craniofac Res (2007) 10:149–60. doi: 10.1111/j.1601-6343.2007.00399.x
50. Li L-F, Wen. Y, Jiang L, Zhu Y-Q. Study of endoplasmic reticulum stress response in osteogenic differentiation of human periodontal ligament cells. Shanghai J Stomatol (2017) 26:577–81. doi: 10.19439/j.sjos.2017.06.001
51. Cui Z, Qin R, Feng J, Liu Y, Zhou X, Qin X, et al. Xbp1s gene of endoplasmic reticulum stress enhances proliferation and osteogenesis of human periodontal ligament cells. Tissue Cell (2023) 83:102139–47. doi: 10.1016/j.tice.2023.102139
52. Xu Y, Yang Y, Hua Z, Li S, Yang Z, Liu Q, et al. Bmp2 immune complexes promote new bone formation by facilitating the direct contact between osteoclasts and osteoblasts. Biomaterials (2021) 275:120890. doi: 10.1016/j.biomaterials.2021.120890
53. Novak S, Madunic J, Shum L, Vucetic M, Wang X, Tanigawa H, et al. Pdgf inhibits Bmp2-induced bone healing. NPJ Regener Med (2023) 8:3. doi: 10.1038/s41536-023-00276-5
54. Zi-Wei C. Effects Of Lentivirus-Mediated Xbp1s On Proliferative And Osteogenic Capacity Of Periodontal Ligament Cells. Lanzhou, China: Master Thesis, Lanzhou University (2022).
55. Ciesielska A, Matyjek M, Kwiatkowska K. Tlr4 and Cd14 trafficking and its influence on Lps-induced pro-inflammatory signaling. Cell Mol Life Sci (2021) 78:1233–61. doi: 10.1007/s00018-020-03656-y
56. Zou X, Xu J, Yao S, Li J, Yang Y, Yang L. Endoplasmic reticulum stress-mediated autophagy protects against lipopolysaccharide-induced apoptosis in Hl-1 cardiomyocytes. Exp Physiol (2014) 99:1348–58. doi: 10.1113/expphysiol.2014.079012
57. Wang Y, Gong J, Zeng H, Liu R, Jin B, Chen L, et al. Lipopolysaccharide activates the unfolded protein response in human periodontal ligament fibroblasts. J Periodontol (2016) 87:E75–81. doi: 10.1902/jop.2015.150413
58. Kang L, Miao Y, Jin Y, Shen S, Lin X. Exosomal Mir-205-5p derived from periodontal ligament stem cells attenuates the inflammation of chronic periodontitis via targeting Xbp1. Immun Inflammation Dis (2023) 11:E743. doi: 10.1002/iid3.743
59. Arron JR, Cho Y. Bone versus immune system. Nature (2000) 408(6812):535–6. doi: 10.1038/35046196
60. Yang N, Liu Y. The role of the immune microenvironment in bone regeneration. Int J Med Sci (2021) 18:3697–707. doi: 10.7150/ijms.61080
61. Mosser DM, Edwards JP. Exploring the full spectrum of macrophage activation. Nat Rev Immunol (2008) 8:958–69. doi: 10.1038/nri2448
62. Pajarinen J, Lin T, Gibon E, Kohno Y, Maruyama M, Nathan K, et al. Mesenchymal stem cell-macrophage crosstalk and bone healing. Biomaterials (2019) 196:80–9. doi: 10.1016/j.biomaterials.2017.12.025
63. Munoz J, Akhavan NS, Mullins AP, Arjmandi BH. Macrophage polarization and osteoporosis: A review. Nutrients (2020) 12:2999–3014. doi: 10.3390/nu12102999
64. Wik JA, Skalhegg BS. T cell metabolism in infection. Front Immunol (2022) 13:840610. doi: 10.3389/fimmu.2022.840610
65. Ruterbusch M, Pruner KB, Shehata L, Pepper M. In vivo Cd4(+) T cell differentiation and function: revisiting the Th1/Th2 paradigm. Annu Rev Immunol (2020) 38:705–25. doi: 10.1146/annurev-immunol-103019-085803
66. Ribot JC, Lopes N, Silva-Santos B. Γδ T cells in tissue physiology and surveillance. Nat Rev Immunol (2021) 21:221–32. doi: 10.1038/s41577-020-00452-4
67. Hoffman W, Lakkis FG, Chalasani G. B cells, antibodies, and more. Clin J Am Soc Nephrol (2016) 11:137–54. doi: 10.2215/CJN.09430915
68. Tangye SG, Nguyen T, Deenick EK, Bryant VL, Ma CS. Inborn errors of human B cell development, differentiation, and function. J Exp Med (2023) 220:e20221105–23. doi: 10.1084/jem.20221105
69. Grcevic D, Sanjay A, Lorenzo J. Interactions of B-lymphocytes and bone cells in health and disease. Bone (2023) 168:116296. doi: 10.1016/j.bone.2021.116296
70. Zouali M. The emerging roles of B cells as partners and targets in periodontitis. Autoimmunity (2017) 50:61–70. doi: 10.1080/08916934.2016.1261841
72. Duan T, Du Y, Xing C, Wang HY, Wang RF. Toll-like receptor signaling and its role in cell-mediated immunity. Front Immunol (2022) 13:812774. doi: 10.3389/fimmu.2022.812774
73. Lim HI, Hajjar KA. Annexin A2 in fibrinolysis, inflammation and fibrosis. Int J Mol Sci (2021) 22:6836–49. doi: 10.3390/ijms22136836
74. Li YZ, Wang YY, Huang L, Zhao YY, Chen LH, Zhang C. Annexin A protein family in atherosclerosis. Clin Chim Acta (2022) 531:406–17. doi: 10.1016/j.cca.2022.05.009
75. Zhou CM, Luo LM, Lin P, Pu Q, Wang B, Qin S, et al. Annexin A2 regulates unfolded protein response via Ire1-Xbp1 axis in macrophages during P. Aeruginosa infection. J Leukoc Biol (2021) 110:375–84. doi: 10.1002/JLB.3A1219-686RR
76. Li R, Tan S, Yu M, Jundt MC, Zhang S, Wu M. Annexin A2 regulates autophagy in Pseudomonas aeruginosa infection through the Akt1-Mtor-Ulk1/2 signaling pathway. J Immunol (2015) 195:3901–11. doi: 10.4049/jimmunol.1500967
77. Lawrence T. The nuclear factor Nf-Kappab pathway in inflammation. Cold Spring Harb Perspect Biol (2009) 1:A001651. doi: 10.1101/cshperspect.a001651
78. Hayden MS, Ghosh S. Nf-Kappab in immunobiology. Cell Res (2011) 21:223–44. doi: 10.1038/cr.2011.13
79. Li W, Jin K, Luo J, Xu W, Wu Y, Zhou J, et al. Nf-kappab and its crosstalk with endoplasmic reticulum stress in atherosclerosis. Front Cardiovasc Med (2022) 9:988266. doi: 10.3389/fcvm.2022.988266
80. Notas G, Kampa M, Castanas E. G protein-coupled estrogen receptor in immune cells and its role in immune-related diseases. Front Endocrinol (Lausanne) (2020) 11:579420. doi: 10.3389/fendo.2020.579420
81. Watson CS, Jeng YJ, Kochukov MY. Nongenomic actions of estradiol compared with estrone and estriol in pituitary tumor cell signaling and proliferation. FASEB J (2008) 22:3328–36. doi: 10.1096/fj.08-107672
82. Mirandola L, Wade R, Verma R, Pena C, Hosiriluck N, Figueroa JA, et al. Sex-driven differences in immunological responses: challenges and opportunities for the immunotherapies of the third millennium. Int Rev Immunol (2015) 34:134–42. doi: 10.3109/08830185.2015.1018417
83. Lu G, Li Q, Liu J, Jia Y, Tang J, Zhang X. Inhibition of endoplasmic reticulum stress and the downstream pathways protects Cd4(+) T cells against apoptosis and immune dysregulation in sepsis. IUBMB Life (2022) 74:1070–80. doi: 10.1002/iub.2666
84. Pramanik J, Chen X, Kar G, Henriksson J, Gomes T, Park JE, et al. Genome-wide analyses reveal the Ire1a-Xbp1 pathway promotes T helper cell differentiation by resolving secretory stress and accelerating proliferation. Genome Med (2018) 10:76. doi: 10.1186/s13073-018-0589-3
85. Dessì S BB, Pulisci D, Spano O, Anchisi C, Tessitore L, Costelli P, et al. Cholesterol content in tumor tissues is inversely associated with high-Density lipoprotein cholesterol in serum in patients with gastrointestinal cancer. Cancer (1994) 73(2):253–8. doi: 10.1002/1097-0142(19940115)73:2<253::AID-CNCR2820730204>3.0.CO;2-F
87. Ma X, Bi E, Lu Y, Su P, Huang C, Liu L, et al. Cholesterol induces Cd8(+) T cell exhaustion in the tumor microenvironment. Cell Metab (2019) 30:143–156.E5. doi: 10.1016/j.cmet.2019.04.002
88. Minnie SA, Hill GR. Immunotherapy of multiple myeloma. J Clin Invest (2020) 130:1565–75. doi: 10.1172/JCI129205
89. Wang M, Lin H. Understanding the function of mammalian sirtuins and protein lysine acylation. Annu Rev Biochem (2021) 90:245–85. doi: 10.1146/annurev-biochem-082520-125411
90. Ianni A, Kumari P, Tarighi S, Simonet NG, Popescu D, Guenther S, et al. Sirt7-dependent deacetylation of Npm promotes P53 stabilization following uv-induced genotoxic stress. Proc Natl Acad Sci U.S.A. (2021) 118:e2015339118–28. doi: 10.1073/pnas.2015339118
91. Yi X, Wang H, Yang Y, Wang H, Zhang H, Guo S, et al. Sirt7 orchestrates melanoma progression by simultaneously promoting cell survival and immune evasion via Upr activation. Signal Transduct Target Ther (2023) 8:107. doi: 10.1038/s41392-023-01314-w
92. Fukuda M, Yoshizawa T, Karim MF, Sobuz SU, Korogi W, Kobayasi D, et al. Sirt7 has A critical role in bone formation by regulating lysine acylation of Sp7/Osterix. Nat Commun (2018) 9:2833. doi: 10.1038/s41467-018-05187-4
93. Conway KL, Kuballa P, Khor B, Zhang M, Shi HN, Virgin HW, et al. Atg5 regulates plasma cell differentiation. Autophagy (2013) 9:528–37. doi: 10.4161/auto.23484
94. Zhu H, Jiang C, Kaufman RJ, Li H, Singh N. In vitro stimulation of Ire1α/Xbp1-deficient B cells with Lps. Methods Mol Biol (2022) 2378:221–31. doi: 10.1007/978-1-0716-1732-8_14
95. Shaffer AL, Shapiro-Shelef M, Iwakoshi NN, Lee AH, Qian SB, Zhao H, et al. Xbp1, downstream of blimp-1, expands the secretory apparatus and other organelles, and increases protein synthesis in plasma cell differentiation. Immunity (2004) 21:81–93. doi: 10.1016/j.immuni.2004.06.010
96. Tellier J, Shi W, Minnich M, Liao Y, Crawford S, Smyth GK, et al. Blimp-1 controls plasma cell function through the regulation of immunoglobulin secretion and the unfolded protein response. Nat Immunol (2016) 17:323–30. doi: 10.1038/ni.3348
97. Li Y, Toraldo G, Li A, Yang X, Zhang H, Qian WP, et al. B cells and T cells are critical for the preservation of bone homeostasis and attainment of peak bone mass in vivo. Blood (2007) 109:3839–48. doi: 10.1182/blood-2006-07-037994
98. Choi Y, Woo KM, Ko SH, Lee YJ, Park SJ, Kim HM, et al. Osteoclastogenesis is enhanced by activated B cells but suppressed by activated Cd8(+) T cells. Eur J Immunol (2001) 31:2179–88. doi: 10.1002/1521-4141(200107)31:7<2179::AID-IMMU2179>3.0.CO;2-X
99. Manabe N, Kawaguchi H, Chikuda H, Miyaura C, Inada M, Nagai R, et al. Connection between B lymphocyte and osteoclast differentiation pathways. J Immunol (2001) 167:2625–31. doi: 10.4049/jimmunol.167.5.2625
100. Heider U, Langelotz C, Jakob C, Zavrski I, Fleissner C, Eucker J, et al. Expression of receptor activator of nuclear factor Kappab ligand on bone marrow plasma cells correlates with osteolytic bone disease in patients with multiple myeloma. Clin Cancer Res (2003) 9:1436–40.
101. Giuliani N, Colla S, Sala R, Moroni M, Lazzaretti M, La Monica S, et al. Human myeloma cells stimulate the receptor activator of nuclear factor-kappa B ligand (Rankl) in T lymphocytes: A potential role in multiple myeloma bone disease. Blood (2002) 100:4615–21. doi: 10.1182/blood-2002-04-1121
Keywords: XBP1, osteoclast, osteoblast, immune microenvironment, bone metabolism
Citation: Lv W, Zheng Y, Jiao J, Fu Y, Xu T, Zhang L, Zhang Z and Ma N (2023) The Role of XBP1 in bone metabolism. Front. Endocrinol. 14:1217579. doi: 10.3389/fendo.2023.1217579
Received: 10 May 2023; Accepted: 04 September 2023;
Published: 19 September 2023.
Edited by:
Nicole Horwood, University of East Anglia, United KingdomReviewed by:
Bettina Grötsch, University of Erlangen Nuremberg, GermanyCopyright © 2023 Lv, Zheng, Jiao, Fu, Xu, Zhang, Zhang and Ma. This is an open-access article distributed under the terms of the Creative Commons Attribution License (CC BY). The use, distribution or reproduction in other forums is permitted, provided the original author(s) and the copyright owner(s) are credited and that the original publication in this journal is cited, in accordance with accepted academic practice. No use, distribution or reproduction is permitted which does not comply with these terms.
*Correspondence: Li Zhang, emhhbmdfbGk5OUBqbHUuZWR1LmNu; Zheng Zhang, emhhbmd6aGVuZ0BuYW5rYWkuZWR1LmNu; Ning Ma, bWFuQGpsdS5lZHUuY24=
†These authors have contributed equally to this work and share first authorship
Disclaimer: All claims expressed in this article are solely those of the authors and do not necessarily represent those of their affiliated organizations, or those of the publisher, the editors and the reviewers. Any product that may be evaluated in this article or claim that may be made by its manufacturer is not guaranteed or endorsed by the publisher.
Research integrity at Frontiers
Learn more about the work of our research integrity team to safeguard the quality of each article we publish.