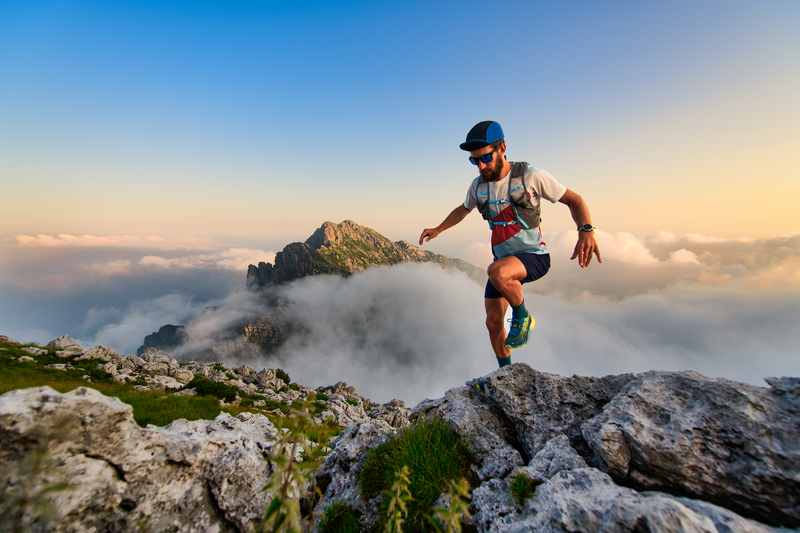
94% of researchers rate our articles as excellent or good
Learn more about the work of our research integrity team to safeguard the quality of each article we publish.
Find out more
HYPOTHESIS AND THEORY article
Front. Endocrinol. , 15 June 2023
Sec. Cardiovascular Endocrinology
Volume 14 - 2023 | https://doi.org/10.3389/fendo.2023.1205442
Research during the past decades has yielded numerous insights into the presence and function of lactate in the body. Lactate is primarily produced via glycolysis and plays special roles in the regulation of tissues and organs, particularly in the cardiovascular system. In addition to being a net consumer of lactate, the heart is also the organ in the body with the greatest lactate consumption. Furthermore, lactate maintains cardiovascular homeostasis through energy supply and signal regulation under physiological conditions. Lactate also affects the occurrence, development, and prognosis of various cardiovascular diseases. We will highlight how lactate regulates the cardiovascular system under physiological and pathological conditions based on evidence from recent studies. We aim to provide a better understanding of the relationship between lactate and cardiovascular health and provide new ideas for preventing and treating cardiovascular diseases. Additionally, we will summarize current developments in treatments targeting lactate metabolism, transport, and signaling, including their role in cardiovascular diseases.
Lactate is an important metabolite mainly generated through glycolysis (1–3). Conventional wisdom holds the opinion that lactate is a metabolic waste produced in normal cells only under hypoxic conditions, including intense exercise and ischemia (1–3). However, burgeoning evidence points out that lactate is not an innocuous bystander metabolite as traditionally viewed, but instead serves as a fuel source for the myocardium (4). In addition, lactate plays special roles in regulating vascular smooth muscle cells (VSMCs) (5), promoting angiogenesis (6, 7), regulating hemodynamics (8), and cardiac electrophysiological activity (9, 10), all of which are essential for the maintenance of cardiovascular homeostasis. In addition, the finding of histone lactoylation (11) and non-histone lactoylation (12) is attractive. In light of the recent finding that lactoyl-CoA may be found in the cardiac tissue of mice (13), it would be prudent to investigate its influence on heart biology.
For over 90 years, it has been observed that lactate can be produced in functioning mitochondria that have sufficient oxygen. This observation is referred to as the Warburg effect (1–3). Studies have recently reported that the Warburg effect occurs in various pathophysiological states of the cardiovascular system, including atrial fibrillation, pulmonary hypertension (PAH), and heart failure (HF), suggesting it has critical roles in cardiovascular disease (14–18). Furthermore, numerous clinical and preclinical studies have shown that lactate directly affects various cardiovascular diseases.
Targeting lactate metabolism has led to a breakthrough in treating some diseases, especially in the field of cancer (19). Some recent studies have indicated that targeting the metabolism, transport, and signaling of lactate may be a promising method for preventing and treating cardiovascular diseases (20). In this review, we summarize how lactate regulates the cardiovascular system, highlighting how it affects cardiovascular diseases. Furthermore, we attempt to provide readers with a systematic and objective understanding of the effect of lactate on cardiovascular health, proposing new ideas about the pathogenesis of cardiovascular diseases and their treatment by targeting lactate.
Lactate is mainly generated through glycolysis in most tissues of the human body, with the highest level of production detected in muscles (1–3). Under anaerobic conditions, pyruvate is reduced to lactate with nicotinamide adenine dinucleotide (NADH) and is subsequently fed into the Cori cycle as a substrate for gluconeogenesis. Glycolysis yields only two adenosine triphosphate (ATP) molecules and two lactate molecules per glucose without consuming any oxygen. Under aerobic conditions, pyruvate enters the Krebs cycle, producing abundant usable energy (approximately 25 ATP molecules per glucose) without lactate production (Figure 1).
Figure 1 Metabolism and transport of lactate under conditions of varying oxygen availability. DHAP dihydroxyacetone phosphate, F-6-P fructose-6-phosphate, F-1,6-2P fructose-1,6-bisphosphate, GAPDH glyceraldehyde-3-phosphate dehydrogenase, G-6-P glucose-6-phosphate, MCT monocarboxylate transporters, PFK-1 phosphofructokinase-1, TCA tricarboxylic acid cycle.
LDH mediates the bidirectional conversion of pyruvate and lactate and plays a crucial role in lactate metabolism (Figure 1) (1, 3, 19). LDH has two major isoforms—namely, LDHA and LDHB. LDHA predominantly catalyzes pyruvate reduction to lactate and couples NAD+ regeneration (21), whereas LDHB mostly converts lactate into pyruvate and couples NADH formation (21). Different combinations of LDHA and LDHB can entirely assemble into five tetrameric isozymes (LDH1, LDH2, LDH3, LDH4, and LDH5), which differ in their Km values for lactate and pyruvate, electrophoretic mobility, and sensitivity to pyruvate accumulation (22). Additionally, they differ in tissue expression; for instance, LDHA is the predominant isoform in skeletal muscles and other highly glycolytic tissues, whereas LDHB is the predominant isoform in the myocardium (21).
Monocarboxylate transporters (MCTs) can bidirectionally transport protons and monocarboxylate ions (lactate, pyruvate, and ketone body molecules), depending on the concentration of both, protons and monocarboxylate ions, in the environment and/or cellular context (23, 24).The MCTs belong to the SLC16 gene family, and different MCT subtypes synergistically maintain the lactate balance. In normal tissues, MCT1 is mainly responsible for lactate transmembrane transport and plays a role both in the import and export of lactate, which depends on the transmembrane gradient for lactic acid (24, 25). In cells that generate high lactic acid concentrations, MCT4 is primarily responsible for facilitating lactic acid and H+ export across cell membranes (24). MCT2 is very similar to MCT1, whereas MCT3 is functionally similar to MCT4 (3). MCT-mediated transmembrane transport is the foundation of the “lactate shuttle” (19, 26) which describes lactate’s function in the transmission of oxidative and gluconeogenic substrates and in cell signaling (19, 26).
Lactate can act as an extracellular ligand to conduct signals via G protein-coupled receptor 81 (GPR81). GPR81 is expressed in meningeal fibroblasts and adipocytes, where it inhibits lipolysis by decreasing the concentration of cyclic adenosine monophosphate e (27) and induces brain vascularization through ERK1/2 and Akt signaling (28). GPR81 also expresses in some tumor cells and sustains tumor growth and metastasis via triggering lactate-sensitive machinery (29). In recent years, researchers have determined that Gpr132, a member of the pH-sensing G protein-coupled receptor family, is a supplemental sensor/receptor for lactate and is highly expressed in macrophages (29). The lactate–Gpr132 axis stimulates the tumor–macrophage interplay to sustain breast cancer metastasis; nevertheless, its molecular mechanism requires further studies (29).
As the principal metabolite of glycolysis, lactate is a substrate for gluconeogenesis and energy metabolism and a signal molecule that regulates gene expression (11, 13, 30) and immune inflammation (11, 31–33) and promotes tumor growth (33–35). With an in-depth understanding of lactate’s regulatory function in tissues and organs, its effects on the cardiovascular system have gradually been revealed. Lactate can serve as energy source for the myocardium, regulate cardiac electrophysiological activity, modulate the function of VSMCs, and promote angiogenesis.
Under normal conditions, fatty acids are the main energy source for the heart (4, 36). However, when the heart is stressed by β-adrenergic stimulation (37–39), chronotropic challenge (40), increasing afterload (38, 41), or shock (42), lactate becomes the preferred fuel. The myocardium obtains 60–90% of its oxidizable carbon source from lactate (43–45). It is now generally accepted that dietary glucose is metabolized into lactate, which is then transported throughout the body and used to fuel the TCA cycle in tissues like the heart (46). It has been shown that the heart is the largest lactate-consuming organ in the body (47, 48). Almost all of the lactate absorbed by the myocardium is oxidized as fuel (39). Selective utilization of the fuel substrate is protective for the myocardium while meeting the energy demand (4). Interestingly, both embryonic and induced pluripotent stem cells can be differentiated into purified cardiomyocytes simply by growing them in glucose-free media containing lactate (49). Nevertheless, the diabetic myocardium constitutes an exception; in particular, compared to the control rat heart, the diabetic rat heart exhibits remarkably reduced lactate oxidation, which may cause an increase in the cytosolic NADH/NAD ratio (50). This observation indicates that diabetes causes specific inhibition of myocardial lactate oxidation, which may explain why patients with heart disease complicated by diabetes have a worse prognosis (50).
VSMCs, the main cellular components of the vasculature, are very important for the maintenance of vascular tension and the regulation of blood pressure (51–54). Recent studies have revealed that lactate promotes the proliferation (55), migration (56), and phenotype conversion of VSMCs (5). In an in vitro study, Kovacs et al. (57) observed that the promotion of lactate production resulted in a considerable increase in calpain activation in the pulmonary arterial smooth muscle cells (PASMCs) of patients with pulmonary arterial hypertension (PAH). Calpain inhibition prevents lactate-induced cell proliferation and reduces apoptosis. Furthermore, previous studies confirmed that the prevention of extracellular and intracellular lactate generation via downregulation of siRNA-PKM2 or LDHA inhibits the proliferation of human aortic VSMCs (16, 58).
The mobility of activated VSMCs is closely related to enhanced aerobic glycolysis (56, 58). Previous studies have shown for VSMC that the inhibition of glycolytic activity inhibits lactate production and migratory behavior via a compromised STAT3/HK2 signaling axis (56, 58). In vitro experiments conducted by Yang et al. revealed for the first time that lactate promotes the expression of synthetic VSMC markers instead of contractile markers when compared to lactate-free circumstances (5). Recent studies have also reported that lactate can inhibit arterial SMC contraction via Ca2+-activated K+ channels (KCa channels) (59, 60). Lactate regulates VSMCs through these pathways, suggesting a potential regulatory mechanism for vascular physiological function and pathological changes in the body.
The promotion of angiogenesis to ameliorate ischemia and hypoxia is beneficial for maintaining cardiovascular function in myocardial infarction (MI), ischemic cardiomyopathy (61), a compensatory period of myocardial hypertrophy (62), and chronic thromboembolic PAH (63, 64). Recent studies have confirmed that lactate promotes angiogenesis (6, 7, 65–69) (Figure 2). In preclinical experiments, exogenous supplementation or endogenous production of lactate promoted angiogenesis in brain tumors. In contrast, angiogenesis was impeded by inhibiting lactate production, by knocking down LDHA (70) or lactate transportation by targeting MCT1 (71–75).
Figure 2 Cellular pathways by which lactate regulates angiogenesis. ASCT2 alanine serine cysteine transporter 2, bFGF basic fibroblast growth factor, GLUT1 glucose transporter 1, HIF-1α hypoxia-inducible factor-1α, IL-8 interleukin-8, IκBα nuclear factor κB inhibitor-alpha, LDHA lactate dehydrogenase A, MCT1 monocarboxylate transporter 1, ME malic enzyme, NDRG3 N-Myc downstream-regulated gene 3, NF-κB nuclear factor κB, PHD prolyl-4-hydroxylase, VEGF vascular endothelial growth factor, VEGFR2 vascular endothelial growth factor receptor 2.
The mechanism by which lactate promotes angiogenesis can be partly explained by its effects on signal regulation. Vegran et al. performed both in vitro and in vivo experiments and reported that when lactate is transported into endothelial cells, it activates pro-angiogenic NF-κB/IL-8 signaling to promote angiogenesis (74). Previous studies showed that lactate inactivates prolyl-4-hydroxylase (PHD) and stabilizes the activation of hypoxia-inducible factor-1α, subsequently promoting angiogenesis by inducing the expression of vascular endothelial growth factor (VEGF)/VEGF receptor 2 and basic fibroblast growth factor (6, 66, 73, 76–78). Of note, lactate can indirectly promote angiogenesis by inducing macrophage secretion of VEGF-α (79, 80). Furthermore, recent studies have determined that lactate promotes angiogenesis via direct binding of N-Myc downstream-regulated gene 3 (NDRG3), an oxygen-regulated protein (5, 81). Lactate prevents NDRG3 from degrading and facilitates hypoxia-induced activation of the c-Raf/ERK pathway, which promotes angiogenesis. Inhibition of lactate production abolishes NDRG3-mediated angiogenesis (5, 81). Therefore, lactate may play a cardioprotective role via the promotion of angiogenesis, providing a new direction for the prevention and treatment of cardiovascular diseases presenting with ischemia and hypoxia.
Tissue metabolites regulating hemodynamics are well-documented (82). Under ischemic or hypoxic conditions, a considerably increased lactate production results in vasodilation with a marked reduction in vascular resistance (8, 60). Lactate also has pH-independent vasodilatory effects in animal coronary arteries (83–85). Nonetheless, Brazitikos et al. confirmed that neutralized lactate mediates acute hypoxia-induced vasodilation in the retina (86), suggesting that the vasodilatory effect may be due to lactate independent of pH change (87). Montoya et al.’s in vitro experiment revealed that lactate leads to coronary dilatation via the release of endothelial nitric oxide in the isolated perfused rat heart (8). Another in vivo experiment, conducted by Omar et al, showed that lactate might cause cGMP-mediated vasodilation in calf pulmonary arteries (88). Additionally, further studies have indicated that lactate promotes vasodilation, which is partly mediated by the activation of KCa channels in porcine coronary arteries (60).
The effects of lactate on vascular resistance and vasodilation are heterogeneous in different organs. Recent studies have shown that GPR81 agonists induce hypertension in rodents, which can be rescued by GPR81 inactivation (20, 89). The pressor effect has been associated with different effects on vascular resistance, which increases in the kidney but remains unchanged in the heart and hind limb (20, 89). This suggests that lactate may bridge metabolism and hemodynamics to maintain body homeostasis under ischemic or hypoxic conditions.
Lactate regulates the electrophysiological activity of the myocardium and is associated with arrhythmia (9, 10, 90). A possible explanation for this effect is how the lactate affects ion channel regulation (91). ATP-sensitive potassium (KATP) channels are inward-rectifying potassium channels that are widely distributed in cardiomyocytes, VSMCs, non-vascular smooth muscle cells, and nerve cells (92–94). Under physiological conditions, activation of the KATP channels plays a myocardial protective role; however, its continuous activation can lead to serious ventricular arrhythmias or even ventricular fibrillation (92–94). Keung et al. reported that intracellular application of lactate activates the KATP channels in guinea pig myocytes (95). Furthermore, Jin et al. confirmed that intracellular lactate induces the opening of the KATP channels in a dose-dependent manner in rabbit ventricular myocytes (91).
The fast sodium current (INa) is an essential ion channel on the membrane of the fast reactive myocardium and is an important cause of arrhythmia under pathological conditions (96). Lactate can modify INa by hyperpolarizing guinea pig ventricular myocytes, which may contribute to the development of ischemic arrhythmia (96, 97). Revealing the effects of lactate on cardiac electrophysiological properties will provide new insights into ischemic arrhythmia.
Various cardiovascular diseases are associated with elevated lactate concentrations, which often indicate a poor prognosis. Revealing the potential mechanism of lactate may provide a new understanding and lead to a breakthrough in preventing and treating cardiovascular diseases (Figure 3).
Figure 3 Implications of lactate in cardiovascular diseases. FFA free fatty acid, GPR81 G protein-coupled receptor 81, OSS oscillatory shear stress, PASMCs pulmonary arterial smooth muscle cells, PHD2 2-oxoglutarate-dependent prolyl-4-hydroxylase, SMCs smooth muscle cells.
Wall thickness is a marker of atherosclerotic plaque burden and is strongly associated with clinical events (98–100). The Atherosclerosis Risk in Communities (ARIC) carotid MRI study revealed a strong gradient correlation between lactate and wall thickness (101). In addition, recent studies have shown that lactate may reduce the risk of atherosclerosis by acting via a variety of different pathways.
First, plasma free fatty acid (FFA), an important risk factor for atherosclerosis, is mainly derived from triglyceride lipolysis in adipose tissues. Lactate activates GPR81 and suppresses lipolysis through insulin-induced antilipolytic effects in mouse, rat, and human adipocytes, and differentiated 3T3-L1 cells (27, 102, 103). GPR81-selective agonists can suppress lipolysis and FFA production in vitro and in mice without side effects (20, 104).
Second, areas of the vasculature affected by oscillatory shear stress (OSS) are more likely to develop into atherosclerotic lesions (105, 106). Physiologically relevant lactate doses can rescue OSS-induced reductions in GPR81 expression, and subsequent GPR81 activation can result in valuable atheroprotective effects in OSS-exposed endothelial cells (107).
Third, SMC proliferation is a key event in atherogenesis. MCT3 mRNA and protein expression are related to atherosclerosis severity. An impairment in lactate transport, arising from MCT3 inhibition, may result in enhanced SMC proliferation and promote of atherosclerosis (108). Lactate exerts an anti-atherosclerotic effect, which may, at least in part, explain the protective effects of moderate exercise against coronary heart disease. This observation suggests that regulation of lactate metabolism may be a potential method for preventing coronary heart disease.
Diabetic patients with acute MI (AMI) have been reported to have higher blood lactate concentrations than non-diabetic patients with AMI (4.54 ± 1.44 vs. 3.19 ± 1.005 mmol/L; P<0.05) (109). Blood lactate concentrations in AMI with diabetes are associated with an increased incidence of HF, severe arrhythmias, cardiogenic shock, and a higher mortality rate (109). Yang et al. reported that lactate concentrations, LDH levels, and MCT expression were greater in the ischemic zone than in non-ischemic tissues in a swine MI model (5). Whether the increase in lactate expression plays a protective role or is just a secondary change in AMI remains controversial. Zhang et al. induced AMI in rats and reported that pharmacological preconditioning with lactic acid and hydrogen-rich saline or lactic acid alone could rescue the infarct area, serum myocardial injury markers, and apoptotic index. This was achieved by creating conditions that mimic persistent tissue acidosis and allow for the selective generation of reactive oxygen species (110). However, Aresta et al. identified that increasing tissue lactate concentrations via repeated transient lactate exposure did not improve contractile recovery after a prolonged ischemic period in an isolated rat heart model (111). Therefore, more evidence is required to reveal the effects of lactate on AMI, which can be an indicator of prognosis.
It is generally acknowledged that elevated lactate concentrations are common in patients with HF and are related to poor outcomes (112–115). Several known conditions can cause lactate accumulation in patients with HF, including (1) the peripheral tissues lack blood and oxygen supply due to low cardiac output, vasoconstriction, hypoxemia, impairment in tissue perfusion, or inability of tissues to increase oxygen extraction (115–118) (2); adrenergic drive and neurohormonal activation, resulting in higher oxygen demand (113); and (3) diminished lactate clearance ability attributable to abnormal hepatic and renal functions (113, 118). An understanding of the causes of lactate accumulation is conducive to personalized treatment strategies.
Increased blood lactate concentrations (≥2 mmol/L) are correlated with a higher 1-year mortality rate in patients with acute HF (113). According to Kawase et al., elevated lactate levels (>3.2 mmol/L) on admission were related to worse in-hospital mortality (odds ratio, 2.14; 95% confidence interval [CI], 1.10–4.21; P=0.03) in patients with acute decompensated HF, either with or without acute coronary syndrome (119) This suggests that high lactate levels could also aid in stratifying the initial risk of early mortality. Gjesdal et al. reached a similar conclusion, reporting that the 30-day mortality rate was higher in MI patients complicated by HF who had a lactate level of ≥2.5 mmol/L than in other patients (112). All of the above mentioned results are based on studies that conducted a single lactate measurement. Biegus et al. investigated how persistent hyperlactatemia affects patients with HF (120) and examined 222 patients with elevated lactate levels. They observed that patients with persistent hyperlactatemia, defined as hyperlactatemia both on admission and after 24 h of hospitalization, had a higher rate of adverse events (e.g., HF worsening) than patients with transient hyperlactatemia. Additionally, persistent hyperlactatemia was an independent predictor of 1-year mortality (hazard ratio [HR], 2.5; 95% CI, 1.5–4.3; P<0.001) (120). In addition to the simple increase in lactate levels, Biegus et al. also observed that hyperlactatemia combined with intracellular iron deficiency significantly increased mortality compared to the control group (HR, 5.6; 95% CI, 2.2–14; P=0.0003) (114, 121). While hyperlactatemia is associated with a poor prognosis in patients with HF, the available evidence indicating that lactate is a risk factor for HF is difficult to prove because lactate is also an important energy source for the myocardium at rest and during stress (43–45). Danielle et al., using metabolomics to quantify blood metabolites from 110 patients, identified that failing hearts consumed more ketones and lactate (48). A pilot randomized controlled clinical trial identified that infusion of half-molar sodium lactate to patients with acute HF increased cardiac output (from 4.05 ± 1.37 L/min to 5.49 ± 1.9 L/min; P<0.01) and tricuspid annular plane systolic excursion (from 14.7 ± 5.5 mm to 18.3 ± 7 mm; P=0.02) without any detrimental effects on organ function (36). Furthermore, preclinical experiments revealed that in cardiac myocytes of rats with congestive HF, the MCT1 formation was significantly upregulated, and the lactate uptake rate was increased, which might promote myocardial energy metabolism and improve heart function (122, 123). Whether lactate is a protective or risk factor in patients with HF needs to be further verified.
Increasing evidence from clinical and preclinical experiments has shown that lactate contributes to the development of hypertension. In a study that included 5,554 participants from the ARIC study who had no diagnosed or subclinical hypertension at baseline, the mean plasma lactate concentrations were 0.8 mmol/L. Compared to the first quartile, the fourth quartile of plasma lactate concentrations was associated with a higher risk of hypertension at a median follow-up of 11.9 years (HR, 1.18; 95% CI, 1.07–1.31), even after adjustment for conventional risk factors (124). Moreover, Lian et al. reported that plasma lactate concentrations were significantly higher in patients with non-dipping hypertension than in those with dipping hypertension, which may contribute to greater targeted organ damage (125). Furthermore, animal experiments revealed that intravenous injection of sodium lactate at concentrations of 0.5 M or 2 M led to a prompt and short-term increase in blood pressure among normotensive Wistar rats and spontaneously hypertensive rats (126).
Lactate may promote an increase in blood pressure via the following pathways. First, lactate is a GPR81 agonist that induces hypertension in wild-type rodents via the endothelin system. Also, antagonism of the endothelin receptors can block the increase in blood pressure (20, 89). Second, lactate promotes an elevation of systemic arterial blood pressure by increasing central sympathetic activity. Marina et al. showed that brainstem hypoxia triggers lactate and ATP release in spontaneously hypertensive rats, promoting C1 neuron excitation in vitro and increasing sympathetic nerve activity and arterial blood pressure in vivo (127). Third, immunomodulation is crucial to the development of hypertension and hypertensive organ injury (128–130). Lactate is an important metabolite that contributes to immunomodulation, which may potentially elucidate why lactate promotes the occurrence of hypertension and target organ injury; nonetheless, further studies are required to confirm this (128, 131, 132). Furthermore, lactic acid can reduce extracellular pH via protons, leading to the activation of acid-sensing ion channels and reflexively increasing mean arterial pressure (133).
Using ultra-high-performance liquid chromatography coupled with high-resolution mass spectrometry, our team recently determined that serum lactate concentrations were higher in patients with PAH than in healthy controls (134). We also confirmed that the expression of glycolysis-related enzymes and LDH increased in a rat model of monocrotaline-induced PAH (134). Our results are consistent with the findings of other relevant studies, which reported that the Warburg effect is enhanced with increased lactate generation in PAH (135, 136). It is well documented that PASMC hyperproliferation is an important pathological basis of pulmonary vascular remodeling (137–140). Lactate not only promotes the proliferation of induced pluripotent stem cell-derived VSMCs in the human aorta (5) but also encourages PASMC proliferation and pulmonary vascular remodeling (57). Recent studies have reported that pulmonary vascular endothelial cells from patients with PAH have decreased PHD2 expression and that mice with endothelial cell-targeted disruption of the gene for PHD2 (EGLN1) develop obliterated vascular remodeling and complex lesions, similar to patients with PAH (141). Additionally, lactate can inhibit 2-oxoglutarate-dependent PHD, predominantly PHD2 (3, 66). These experiments suggest that lactate may promote the development of PAH via PHD2 inhibition. Recently, some researchers have focused on inhibiting the Warburg effect to develop new therapies for PAH (136, 142). However, prior to that, a more careful investigation of the mechanism of the Warburg effect and lactate in relation to the development of PAH is necessary (136).
As mentioned above, lactate affects the progression of cardiovascular diseases. Regulation of lactate production or signal transduction is a promising approach for cardiovascular disease therapeutics. Three pathways can directly influence lactate metabolism and signal transduction.
First, targeting LDH enzymes can directly affect lactate production. A variety of LDH-targeting compounds have been validated in preclinical models of cancer (19). Among these compounds, AT-101 is a nonselective LDH inhibitor, while galloflaavin, FX-11, and N-hydroxyindole-based compounds have been identified to preferentially inhibit LDHA than LDHB (19). Therefore, the efficacy of LDH inhibitors will depend on the expression of the LDH isoform in tissues and will be context-dependent.
Second, targeting MCTs may have considerable effects on lactate-dependent metabolic symbiosis, which is responsible for intracellular and extracellular lactate homeostasis. Several MCT inhibitors have been identified, such as α-cyano-4-hydroxycinnamate (143), organomercurials, and stilbene disulfonates (144), as well as other MCT inhibitors with higher selectivity, includingAR-C155858 (145), and the AstraZeneca compounds AZD3965 (targets MCT1 and MCT2) (146) 7-aminocarboxycoumarins (targets MCT1 and MCT4), SR13800 (targets MCT1) (147), and AZ93 (targets MCT4) (148). Currently, inhibition of MCT transport has been widely investigated in the field of cancer, and some breakthroughs have been achieved (149). Recent work has also shown that inhibiting lactate export by targeting MCT4 can mitigate isoproterenol-induced hypertrophy in cultured cardiomyocytes and in mice (149).
Third, targeting GPR81 is a potentially effective therapeutic approach, as it plays a signal transduction role by activating GPR81, thus indicating the potential of this receptor in controlling hypertension. Numerous studies have reported that lactate promotes hypertension via GPR81 (20, 89). Hence, theoretically, selective high-affinity antagonists of GPR81 have promise as antihypertensive drugs. Furthermore, GPR81 knockout mice ‘do not have any obvious difference in cardiovascular phenotype, indicating that pharmacological blockade of the receptor to antihypertensive might limit any important side effects (20). However, no such blockers are currently available to test the validity of this strategy.
Lactate metabolism plays an important role in regulating the cardiovascular system. Nonetheless, lactate’s mechanism of action at the molecular level in the cardiovascular system is not fully understood. New evidence indicates that lactate can extend its metabolic function to react with cells, tissues, or organs and that lactate regulates different cellular signaling pathways. Recent findings also suggest an important role for lactate in the heart. Growing both embryonic and induced pluripotent stem cells in lactate-supplemented, glucose-free medium allows for their differentiation into cardiomyocytes (49), indicating their critical role in the differentiation of cardiomyocytes. Isoproterenol-induced hypertrophy could be attenuated by inhibiting lactate export (149), suggesting a possible therapeutic strategy by targeting lactate. These findings not only aid us in obtaining a new understanding of how lactate regulates the cardiovascular system but also encourage us to re-examine the role of the Warburg effect in the cardiovascular system. Further exploration of how lactate regulates the cardiovascular system under physiological and pathological conditions and examining whether targeting lactate metabolism, transport, or signal transduction can be exploited as an effective protective strategy against cardiovascular diseases will be meaningful.
The original contributions presented in the study are included in the article/supplementary material. Further inquiries can be directed to the corresponding author.
PW and FL conceived the idea. PW wrote the manuscript. TZ, YH and PW collected and read the literature and revised the article. FL and ZF read through and corrected the manuscript. All authors contributed to the article and approved the submitted version.
This research was supported by grants from Hunan Provincial Natural Science Foundation of China [grant number 2021JJ40852 to FL]; National Natural Science Foundation of China [grant number 82100495 to FL]; Scientific Research Project of Hunan Provincial Health Commission [grant number 202203014009 to FL]; Scientific Research Launch Project for new employees of the Second Xiangya Hospital of Central South University [to FL]; China Postdoctoral Science Foundation [to FL]. Key Research and Development Program of Hunan Province of China [grant number 2021SK2004 to ZF].
We would like to express our gratitude to all those who helped us during the writing of this manuscript. Thanks to all the peer reviewers for their opinions and suggestions. We thank Jiaqi Liu for her support in making the illustrations as it appears in the figure.
The authors declare that the research was conducted in the absence of any commercial or financial relationships that could be construed as a potential conflict of interest.
All claims expressed in this article are solely those of the authors and do not necessarily represent those of their affiliated organizations, or those of the publisher, the editors and the reviewers. Any product that may be evaluated in this article, or claim that may be made by its manufacturer, is not guaranteed or endorsed by the publisher.
1. Ferguson BS, Rogatzki MJ, Goodwin ML, Kane DA, Rightmire Z, Gladden LB. Lactate metabolism: historical context, prior misinterpretations, and current understanding. Eur J Appl Physiol (2018) 118(4):691–728. doi: 10.1007/s00421-017-3795-6
2. Brown TP, Ganapathy V. Lactate/GPR81 signaling and proton motive force in cancer: role in angiogenesis, immune escape, nutrition, and warburg phenomenon. Pharmacol Ther (2020) 206:107451. doi: 10.1016/j.pharmthera.2019.107451
3. Ippolito L, Morandi A, Giannoni E, Chiarugi P. Lactate: a metabolic driver in the tumour landscape. Trends Biochem Sci (2019) 44(2):153–66. doi: 10.1016/j.tibs.2018.10.011
4. Abozguia K, Shivu GN, Ahmed I, Phan TT, Frenneaux MP. The heart metabolism: pathophysiological aspects in ischaemia and heart failure. Curr Pharm Des (2009) 15(8):827–35. doi: 10.2174/138161209787582101
5. Yang L, Gao L, Nickel T, Yang J, Zhou J, Gilbertsen A, et al. Lactate promotes synthetic phenotype in vascular smooth muscle cells. Circ Res (2017) 121(11):1251–62. doi: 10.1161/CIRCRESAHA.117.311819
6. Hunt TK, Aslam RS, Beckert S, Wagner S, Ghani QP, Hussain MZ, et al. Aerobically derived lactate stimulates revascularization and tissue repair via redox mechanisms. Antioxid Redox Signal (2007) 9(8):1115–24. doi: 10.1089/ars.2007.1674
7. Imre G. The role of increased lactic acid concentration in neovascularizations. Acta Morphol Hung. (1984) 32(2):97–103. doi: 10.1007/BF02385184
8. Montoya JJ, Fernández N, Monge L, Diéguez G, Villalón AL. Nitric oxide-mediated relaxation to lactate of coronary circulation in the isolated perfused rat heart. J Cardiovasc Pharmacol (2011) 58(4):392–8. doi: 10.1097/FJC.0b013e318226bcf7
9. Hiraoka M, Okamoto Y, Sano T. Electrophysiological effects of lactates in mammalian ventricular tissues. J Electrocardiol. (1981) 14(1):13–20. doi: 10.1016/S0022-0736(81)80023-4
10. Saman S, Opie LH. Mechanism of reduction of action potential duration of ventricular myocardium by exogenous lactate. J Mol Cell Cardiol (1984) 16(7):659–62. doi: 10.1016/S0022-2828(84)80629-X
11. Zhang D, Tang Z, Huang H, Zhou G, Cui C, Weng Y, et al. Metabolic regulation of gene expression by histone lactylation. Nature. (2019) 574(7779):575–80. doi: 10.1038/s41586-019-1678-1
12. Gaffney DO, Jennings EQ, Anderson CC, Marentette JO, Shi T, Schou Oxvig AM, et al. Non-enzymatic lysine lactoylation of glycolytic enzymes. Cell Chem Biol (2020) 27(2):206–13.e6. doi: 10.1016/j.chembiol.2019.11.005
13. Varner EL, Trefely S, Bartee D, von Krusenstiern E, Izzo L, Bekeova C, et al. Quantification of lactoyl-CoA (lactyl-CoA) by liquid chromatography mass spectrometry in mammalian cells and tissues. Open Biol (2020) 10(9):200187. doi: 10.1098/rsob.200187
14. Xiao Y, Peng H, Hong C, Chen Z, Deng X, Wang A, et al. PDGF promotes the warburg effect in pulmonary arterial smooth muscle cells via activation of the PI3K/AKT/mTOR/HIF-1α signaling pathway. Cell Physiol Biochem (2017) 42(4):1603–13. doi: 10.1159/000479401
15. Hu HJ, Zhang C, Tang ZH, Qu SL, Jiang ZS. Regulating the warburg effect on metabolic stress and myocardial fibrosis remodeling and atrial intracardiac waveform activity induced by atrial fibrillation. Biochem Biophys Res Commun (2019) 516(3):653–60. doi: 10.1016/j.bbrc.2019.06.055
16. Zhou Q, Xu J, Liu M, He L, Zhang K, Yang Y, et al. Warburg effect is involved in apelin-13-induced human aortic vascular smooth muscle cells proliferation. J Cell Physiol (2019) 234(9):14413–21. doi: 10.1002/jcp.28218
17. Rees ML, Subramaniam J, Li Y, Hamilton DJ, Frazier OH, Taegtmeyer H. A PKM2 signature in the failing heart. Biochem Biophys Res Commun (2015) 459(3):430–6. doi: 10.1016/j.bbrc.2015.02.122
18. Barron JT, Parrillo JE. Production of lactic acid and energy metabolism in vascular smooth muscle: effect of dichloroacetate. Am J Physiol (1995) 268(2 Pt 2):H713–9. doi: 10.1152/ajpheart.1995.268.2.H713
19. Doherty JR, Cleveland JL. Targeting lactate metabolism for cancer therapeutics. J Clin Invest. (2013) 123(9):3685–92. doi: 10.1172/JCI69741
20. Wallenius K, Thalén P, Björkman JA, Johannesson P, Wiseman J, Böttcher G, et al. Involvement of the metabolic sensor GPR81 in cardiovascular control. JCI Insight (2017) 2(19). doi: 10.1172/jci.insight.92564
21. Dawson DM, Goodfriend TL, Kaplan NO. LACTIC DEHYDROGENASES: FUNCTIONS OF THE TWO TYPES RATES OF SYNTHESIS OF THE TWO MAJOR FORMS CAN BE CORRELATED WITH METABOLIC DIFFERENTIATION. Science. (1964) 143(3609):929–33. doi: 10.1126/science.143.3609.929
22. Markert CL, Shaklee JB, Whitt GS. Evolution of a gene. multiple genes for LDH isozymes provide a model of the evolution of gene structure, function and regulation. Science. (1975) 189(4197):102–14. doi: 10.1126/science.1138367
23. Halestrap AP. The SLC16 gene family - structure, role and regulation in health and disease. Mol Aspects Med (2013) 34(2-3):337–49. doi: 10.1016/j.mam.2012.05.003
24. Halestrap AP. Monocarboxylic acid transport. Compr Physiol (2013) 3(4):1611–43. doi: 10.1002/cphy.c130008
25. Gandellini P, Giannoni E, Casamichele A, Taddei ML, Callari M, Piovan C, et al. miR-205 hinders the malignant interplay between prostate cancer cells and associated fibroblasts. Antioxid Redox Signal (2014) 20(7):1045–59. doi: 10.1089/ars.2013.5292
26. Brooks GA. Cell-cell and intracellular lactate shuttles. J Physiol (2009) 587(Pt 23):5591–600. doi: 10.1113/jphysiol.2009.178350
27. Ahmed K, Tunaru S, Tang C, Müller M, Gille A, Sassmann A, et al. An autocrine lactate loop mediates insulin-dependent inhibition of lipolysis through GPR81. Cell Metab (2010) 11(4):311–9. doi: 10.1016/j.cmet.2010.02.012
28. Morland C, Lauritzen KH, Puchades M, Holm-Hansen S, Andersson K, Gjedde A, et al. The lactate receptor, G-protein-coupled receptor 81/hydroxycarboxylic acid receptor 1: expression and action in brain. J Neurosci Res (2015) 93(7):1045–55. doi: 10.1002/jnr.23593
29. Roland CL, Arumugam T, Deng D, Liu SH, Philip B, Gomez S, et al. Cell surface lactate receptor GPR81 is crucial for cancer cell survival. Cancer Res (2014) 74(18):5301–10. doi: 10.1158/0008-5472.CAN-14-0319
30. Peter K, Rehli M, Singer K, Renner-Sattler K, Kreutz M. Lactic acid delays the inflammatory response of human monocytes. Biochem Biophys Res Commun (2015) 457(3):412–8. doi: 10.1016/j.bbrc.2015.01.005
31. Pucino V, Certo M, Bulusu V, Cucchi D, Goldmann K, Pontarini E, et al. Lactate buildup at the site of chronic inflammation promotes disease by inducing CD4(+) T cell metabolic rewiring. Cell Metab (2019) 30(6):1055–74.e8. doi: 10.1016/j.cmet.2019.10.004
32. Raychaudhuri D, Bhattacharya R, Sinha BP, Liu CSC, Ghosh AR, Rahaman O, et al. Lactate induces pro-tumor reprogramming in intratumoral plasmacytoid dendritic cells. Front Immunol (2019) 10:1878. doi: 10.3389/fimmu.2019.01878
33. Colegio OR, Chu NQ, Szabo AL, Chu T, Rhebergen AM, Jairam V, et al. Functional polarization of tumour-associated macrophages by tumour-derived lactic acid. Nature. (2014) 513(7519):559–63. doi: 10.1038/nature13490
34. Liu N, Luo J, Kuang D, Xu S, Duan Y, Xia Y, et al. Lactate inhibits ATP6V0d2 expression in tumor-associated macrophages to promote HIF-2α-mediated tumor progression. J Clin Invest. (2019) 129(2):631–46. doi: 10.1172/JCI123027
35. Updegraff BL, Zhou X, Guo Y, Padanad MS, Chen PH, Yang C, et al. Transmembrane protease TMPRSS11B promotes lung cancer growth by enhancing lactate export and glycolytic metabolism. Cell Rep (2018) 25(8):2223–33.e6. doi: 10.1016/j.celrep.2018.10.100
36. Nalos M, Leverve X, Huang S, Weisbrodt L, Parkin R, Seppelt I, et al. Half-molar sodium lactate infusion improves cardiac performance in acute heart failure: a pilot randomised controlled clinical trial. Crit Care (2014) 18(2):R48. doi: 10.1186/cc13793
37. Lopaschuk GD, Ussher JR, Folmes CD, Jaswal JS, Stanley WC. Myocardial fatty acid metabolism in health and disease. Physiol Rev (2010) 90(1):207–58. doi: 10.1152/physrev.00015.2009
38. Wyatt HL, Da Luz PL, Waters DD, Swan HJ, Forrester JS. Contrasting influences of alterations in ventricular preload and afterload upon systemic hemodynamics, function, and metabolism of ischemic myocardium. Circulation. (1977) 55(2):318–24. doi: 10.1161/01.CIR.55.2.318
39. Stanley WC. Myocardial lactate metabolism during exercise. Med Sci Sports Exerc. (1991) 23(8):920–4. doi: 10.1249/00005768-199108000-00006
40. Camici P, Marraccini P, Marzilli M, Lorenzoni R, Buzzigoli G, Puntoni R, et al. Coronary hemodynamics and myocardial metabolism during and after pacing stress in normal humans. Am J Physiol (1989) 257(3 Pt 1):E309–17. doi: 10.1152/ajpendo.1989.257.3.E309
41. Bourassa MG, Campeau L, Bois MA, Rico O. Myocardial lactate metabolism at rest and during exercise in ischemic heart disease. Am J Cardiol (1969) 23(6):771–7. doi: 10.1016/0002-9149(69)90370-1
42. Kline JA, Thornton LR, Lopaschuk GD, Barbee RW, Watts JA. Lactate improves cardiac efficiency after hemorrhagic shock. Shock. (2000) 14(2):215–21. doi: 10.1097/00024382-200014020-00023
43. Gertz EW, Wisneski JA, Stanley WC, Neese RA. Myocardial substrate utilization during exercise in humans. dual carbon-labeled carbohydrate isotope experiments. J Clin Invest. (1988) 82(6):2017–25. doi: 10.1172/JCI113822
44. Drake AJ, Haines JR, Noble MI. Preferential uptake of lactate by the normal myocardium in dogs. Cardiovasc Res (1980) 14(2):65–72. doi: 10.1093/cvr/14.2.65
45. Bertrand ME, Carre AG, Ginestet AP, Lefebvre JM, Desplanque LA, Lekieffre JP. Maximal exercise in normal subjects: changes in coronary sinus blood flow, contractility and myocardial extraction of FFA and lactate. Eur J Cardiol (1977) 5(6):481–91.
46. Hui S, Ghergurovich JM, Morscher RJ, Jang C, Teng X, Lu W, et al. Glucose feeds the TCA cycle via circulating lactate. Nature. (2017) 551(7678):115–8. doi: 10.1038/nature24057
47. Bartman CR, Weilandt DR, Shen Y, Lee WD, Han Y, TeSlaa T, et al. Slow TCA flux and ATP production in primary solid tumours but not metastases. Nature. (2023) 614(7947):349–57. doi: 10.1038/s41586-022-05661-6
48. Murashige D, Jang C, Neinast M, Edwards JJ, Cowan A, Hyman MC, et al. Comprehensive quantification of fuel use by the failing and nonfailing human heart. Science. (2020) 370(6514):364–8. doi: 10.1126/science.abc8861
49. Fuerstenau-Sharp M, Zimmermann ME, Stark K, Jentsch N, Klingenstein M, Drzymalski M, et al. Generation of highly purified human cardiomyocytes from peripheral blood mononuclear cell-derived induced pluripotent stem cells. PLoS One (2015) 10(5):e0126596. doi: 10.1371/journal.pone.0126596
50. Chatham JC, Gao ZP, Bonen A, Forder JR. Preferential inhibition of lactate oxidation relative to glucose oxidation in the rat heart following diabetes. Cardiovasc Res (1999) 43(1):96–106. doi: 10.1016/S0008-6363(99)00056-5
51. St Paul A, Corbett CB, Okune R, Autieri MV, Angiotensin II. Hypercholesterolemia, and vascular smooth muscle cells: a perfect trio for vascular pathology. Int J Mol Sci (2020) 21(12). doi: 10.3390/ijms21124525
52. Bennett MR, Sinha S, Owens GK. Vascular smooth muscle cells in atherosclerosis. Circ Res (2016) 118(4):692–702. doi: 10.1161/CIRCRESAHA.115.306361
53. Farina FM, Hall IF, Serio S, Zani S, Climent M, Salvarani N, et al. miR-128-3p is a novel regulator of vascular smooth muscle cell phenotypic switch and vascular diseases. Circ Res (2020) 126(12):e120–e35. doi: 10.1161/CIRCRESAHA.120.316489
54. Frismantiene A, Philippova M, Erne P, Resink TJ. Smooth muscle cell-driven vascular diseases and molecular mechanisms of VSMC plasticity. Cell Signal (2018) 52:48–64. doi: 10.1016/j.cellsig.2018.08.019
55. Zhou Y, Liu X, Huang C, Lin D. Lactate activates AMPK remodeling of the cellular metabolic profile and promotes the proliferation and differentiation of C2C12 myoblasts. Int J Mol Sci (2022) 23:13996. doi: 10.3390/ijms232213996
56. Heiss EH, Schachner D, Donati M, Grojer CS, Dirsch VM. Increased aerobic glycolysis is important for the motility of activated VSMC and inhibited by indirubin-3’-monoxime. Vascul Pharmacol (2016) 83:47–56. doi: 10.1016/j.vph.2016.05.002
57. Kovacs L, Cao Y, Han W, Meadows L, Kovacs-Kasa A, Kondrikov D, et al. PFKFB3 in smooth muscle promotes vascular remodeling in pulmonary arterial hypertension. Am J Respir Crit Care Med (2019) 200(5):617–27. doi: 10.1164/rccm.201812-2290OC
58. Kim JH, Bae KH, Byun JK, Lee S, Kim JG, Lee IK, et al. Lactate dehydrogenase-a is indispensable for vascular smooth muscle cell proliferation and migration. Biochem Biophys Res Commun (2017) 492(1):41–7. doi: 10.1016/j.bbrc.2017.08.041
59. Barron JT, Nair A. Lactate depresses sarcolemmal permeability of Ca2+ in intact arterial smooth muscle. Life Sci (2003) 74(5):651–62. doi: 10.1016/j.lfs.2003.07.011
60. Mori K, Nakaya Y, Sakamoto S, Hayabuchi Y, Matsuoka S, Kuroda Y. Lactate-induced vascular relaxation in porcine coronary arteries is mediated by Ca2+-activated k+ channels. J Mol Cell Cardiol (1998) 30(2):349–56. doi: 10.1006/jmcc.1997.0598
61. Li Z, Solomonidis EG, Meloni M, Taylor RS, Duffin R, Dobie R, et al. Single-cell transcriptome analyses reveal novel targets modulating cardiac neovascularization by resident endothelial cells following myocardial infarction. Eur Heart J (2019) 40(30):2507–20. doi: 10.1093/eurheartj/ehz305
62. Oka T, Akazawa H, Naito AT, Komuro I. Angiogenesis and cardiac hypertrophy: maintenance of cardiac function and causative roles in heart failure. Circ Res (2014) 114(3):565–71. doi: 10.1161/CIRCRESAHA.114.300507
63. Quarck R, Wynants M, Verbeken E, Meyns B, Delcroix M. Contribution of inflammation and impaired angiogenesis to the pathobiology of chronic thromboembolic pulmonary hypertension. Eur Respir J (2015) 46(2):431–43. doi: 10.1183/09031936.00009914
64. Deveza L, Choi J, Yang F. Therapeutic angiogenesis for treating cardiovascular diseases. Theranostics. (2012) 2(8):801–14. doi: 10.7150/thno.4419
65. García-Cañaveras JC, Chen L, Rabinowitz JD. The tumor metabolic microenvironment: lessons from lactate. Cancer Res (2019) 79(13):3155–62. doi: 10.1158/0008-5472.CAN-18-3726
66. Lu H, Dalgard CL, Mohyeldin A, McFate T, Tait AS, Verma A. Reversible inactivation of HIF-1 prolyl hydroxylases allows cell metabolism to control basal HIF-1. J Biol Chem (2005) 280(51):41928–39. doi: 10.1074/jbc.M508718200
67. Crowther M, Brown NJ, Bishop ET, Lewis CE. Microenvironmental influence on macrophage regulation of angiogenesis in wounds and malignant tumors. J Leukoc Biol (2001) 70(4):478–90. doi: 10.1189/jlb.70.4.478
68. Ghani QP, Wagner S, Hussain MZ. Role of ADP-ribosylation in wound repair. the contributions of Thomas k. hunt, MD. Wound Repair Regen. (2003) 11(6):439–44. doi: 10.1046/j.1524-475X.2003.11608.x
69. Beckert S, Farrahi F, Aslam RS, Scheuenstuhl H, Königsrainer A, Hussain MZ, et al. Lactate stimulates endothelial cell migration. Wound Repair Regen. (2006) 14(3):321–4. doi: 10.1111/j.1743-6109.2006.00127.x
70. Parra-Bonilla G, Alvarez DF, Alexeyev M, Vasauskas A, Stevens T. Lactate dehydrogenase a expression is necessary to sustain rapid angiogenesis of pulmonary microvascular endothelium. PloS One (2013) 8(9):e75984. doi: 10.1371/journal.pone.0075984
71. Morland C, Andersson KA, Haugen ØP, Hadzic A, Kleppa L, Gille A, et al. Exercise induces cerebral VEGF and angiogenesis via the lactate receptor HCAR1. Nat Commun (2017) 8:15557. doi: 10.1038/ncomms15557
72. Deng F, Zhou R, Lin C, Yang S, Wang H, Li W, et al. Tumor-secreted dickkopf2 accelerates aerobic glycolysis and promotes angiogenesis in colorectal cancer. Theranostics. (2019) 9(4):1001–14. doi: 10.7150/thno.30056
73. Sonveaux P, Copetti T, De Saedeleer CJ, Végran F, Verrax J, Kennedy KM, et al. Targeting the lactate transporter MCT1 in endothelial cells inhibits lactate-induced HIF-1 activation and tumor angiogenesis. PloS One (2012) 7(3):e33418. doi: 10.1371/journal.pone.0033418
74. Végran F, Boidot R, Michiels C, Sonveaux P, Feron O. Lactate influx through the endothelial cell monocarboxylate transporter MCT1 supports an NF-κB/IL-8 pathway that drives tumor angiogenesis. Cancer Res (2011) 71(7):2550–60. doi: 10.1158/0008-5472.CAN-10-2828
75. Zhou J, Liu T, Guo H, Cui H, Li P, Feng D, et al. Lactate potentiates angiogenesis and neurogenesis in experimental intracerebral hemorrhage. Exp Mol Med (2018) 50(7):1–12. doi: 10.1038/s12276-018-0113-2
76. Kumar VB, Viji RI, Kiran MS, Sudhakaran PR. Endothelial cell response to lactate: implication of PAR modification of VEGF. J Cell Physiol (2007) 211(2):477–85. doi: 10.1002/jcp.20955
77. Burns PA, Wilson DJ. Angiogenesis mediated by metabolites is dependent on vascular endothelial growth factor (VEGF). Angiogenesis. (2003) 6(1):73–7. doi: 10.1023/A:1025862731653
78. Xiong M, Elson G, Legarda D, Leibovich SJ. Production of vascular endothelial growth factor by murine macrophages: regulation by hypoxia, lactate, and the inducible nitric oxide synthase pathway. Am J Pathol (1998) 153(2):587–98. doi: 10.1016/S0002-9440(10)65601-5
79. Carmona-Fontaine C, Deforet M, Akkari L, Thompson CB, Joyce JA, Xavier JB. Metabolic origins of spatial organization in the tumor microenvironment. Proc Natl Acad Sci U S A. (2017) 114(11):2934–9. doi: 10.1073/pnas.1700600114
80. Constant JS, Feng JJ, Zabel DD, Yuan H, Suh DY, Scheuenstuhl H, et al. Lactate elicits vascular endothelial growth factor from macrophages: a possible alternative to hypoxia. Wound Repair Regen. (2000) 8(5):353–60. doi: 10.1111/j.1524-475X.2000.00353.x
81. Lee DC, Sohn HA, Park ZY, Oh S, Kang YK, Lee KM, et al. A lactate-induced response to hypoxia. Cell. (2015) 161(3):595–609. doi: 10.1016/j.cell.2015.03.011
82. Komaru T, Kanatsuka H, Shirato K. Coronary microcirculation: physiology and pharmacology. Pharmacol Ther (2000) 86(3):217–61. doi: 10.1016/S0163-7258(00)00057-7
83. Hester RK, Weiss GB, Willerson JT. Basis of pH-independent inhibitory effects of lactate on 45Ca movements and responses to KCl and PGF2 alpha in canine coronary arteries. Circ Res (1980) 46(6):771–9. doi: 10.1161/01.RES.46.6.771
84. Ishizaka H, Gudi SR, Frangos JA, Kuo L. Coronary arteriolar dilation to acidosis: role of ATP-sensitive potassium channels and pertussis toxin-sensitive G proteins. Circulation. (1999) 99(4):558–63. doi: 10.1161/01.CIR.99.4.558
85. Hayabuchi Y, Nakaya Y, Matsuoka S, Kuroda Y. Effect of acidosis on Ca2+-activated k+ channels in cultured porcine coronary artery smooth muscle cells. Pflugers Arch (1998) 436(4):509–14. doi: 10.1007/s004240050665
86. Brazitikos PD, Pournaras CJ, Munoz JL, Tsacopoulos M. Microinjection of l-lactate in the preretinal vitreous induces segmental vasodilation in the inner retina of miniature pigs. Invest Ophthalmol Vis Sci (1993) 34(5):1744–52.
87. Cross HR, Clarke K, Opie LH, Radda GK. Is lactate-induced myocardial ischaemic injury mediated by decreased pH or increased intracellular lactate? J Mol Cell Cardiol (1995) 27(7):1369–81. doi: 10.1006/jmcc.1995.0130
88. Omar HA, Mohazzab KM, Mortelliti MP, Wolin MS. O2-dependent modulation of calf pulmonary artery tone by lactate: potential role of H2O2 and cGMP. Am J Physiol (1993) 264(2 Pt 1):L141–5. doi: 10.1152/ajplung.1993.264.2.L141
89. Jones NK, Stewart K, Czopek A, Menzies RI, Thomson A, Moran CM, et al. Endothelin-1 mediates the systemic and renal hemodynamic effects of GPR81 activation. Hypertension. (2020) 75(5):1213–22. doi: 10.1161/HYPERTENSIONAHA.119.14308
90. Wissner SB. The effect of excess lactate upon the excitability of the sheep purkinje fiber. J Electrocardiol. (1974) 7(1):17–26. doi: 10.1016/S0022-0736(74)80004-X
91. Han J, So I, Kim EY, Earm YE. ATP-sensitive potassium channels are modulated by intracellular lactate in rabbit ventricular myocytes. Pflugers Arch (1993) 425(5-6):546–8. doi: 10.1007/BF00374883
92. Liss B, Roeper J. Molecular physiology of neuronal K-ATP channels (review). Mol Membr Biol (2001) 18(2):117–27.
93. Ashford ML, Sturgess NC, Trout NJ, Gardner NJ, Hales CN. Adenosine-5’-triphosphate-sensitive ion channels in neonatal rat cultured central neurones. Pflugers Arch (1988) 412(3):297–304. doi: 10.1007/BF00582512
94. Nichols CG, Ripoll C, Lederer WJ. ATP-sensitive potassium channel modulation of the guinea pig ventricular action potential and contraction. Circ Res (1991) 68(1):280–7. doi: 10.1161/01.RES.68.1.280
95. Keung EC, Li Q. Lactate activates ATP-sensitive potassium channels in guinea pig ventricular myocytes. J Clin Invest. (1991) 88(5):1772–7. doi: 10.1172/JCI115497
96. Tanaka H, Habuchi Y, Lu LL, Furukawa T, Morikawa J, Yoshimura M. Modulation of sodium current by lactate in guinea pig ventricular myocytes. Cardiovasc Res (1994) 28(10):1507–12. doi: 10.1093/cvr/28.10.1507
97. Hoffman BF, Rosen MR. Cellular mechanisms for cardiac arrhythmias. Circ Res (1981) 49(1):1–15. doi: 10.1161/01.RES.49.1.1
98. Wagenknecht L, Wasserman B, Chambless L, Coresh J, Folsom A, Mosley T, et al. Correlates of carotid plaque presence and composition as measured by MRI: the atherosclerosis risk in communities study. Circ Cardiovasc Imaging. (2009) 2(4):314–22. doi: 10.1161/CIRCIMAGING.108.823922
99. Saba L, Sanfilippo R, Pascalis L, Montisci R, Caddeo G, Mallarini G. Carotid artery wall thickness and ischemic symptoms: evaluation using multi-detector-row CT angiography. Eur Radiol (2008) 18(9):1962–71. doi: 10.1007/s00330-008-0962-5
100. Zureik M, Ducimetière P, Touboul PJ, Courbon D, Bonithon-Kopp C, Berr C, et al. Common carotid intima-media thickness predicts occurrence of carotid atherosclerotic plaques: longitudinal results from the aging vascular study (EVA) study. Arterioscler Thromb Vasc Biol (2000) 20(6):1622–9. doi: 10.1161/01.ATV.20.6.1622
101. Shantha GP, Wasserman B, Astor BC, Coresh J, Brancati F, Sharrett AR, et al. Association of blood lactate with carotid atherosclerosis: the atherosclerosis risk in communities (ARIC) carotid MRI study. Atherosclerosis. (2013) 228(1):249–55. doi: 10.1016/j.atherosclerosis.2013.02.014
102. Liu C, Wu J, Zhu J, Kuei C, Yu J, Shelton J, et al. Lactate inhibits lipolysis in fat cells through activation of an orphan G-protein-coupled receptor, GPR81. J Biol Chem (2009) 284(5):2811–22. doi: 10.1074/jbc.M806409200
103. Lafontan M, Langin D. Lipolysis and lipid mobilization in human adipose tissue. Prog Lipid Res (2009) 48(5):275–97. doi: 10.1016/j.plipres.2009.05.001
104. Sakurai T, Davenport R, Stafford S, Grosse J, Ogawa K, Cameron J, et al. Identification of a novel GPR81-selective agonist that suppresses lipolysis in mice without cutaneous flushing. Eur J Pharmacol (2014) 727:1–7. doi: 10.1016/j.ejphar.2014.01.029
105. Bai L, Shyy JYP. Shear stress regulation of endothelium: a double-edged sword. J Transl Int Med (2018) 6(2):58–61. doi: 10.2478/jtim-2018-0019
106. Chistiakov DA, Orekhov AN, Bobryshev YV. Effects of shear stress on endothelial cells: go with the flow. Acta Physiol (2017) 219(2):382–408. doi: 10.1111/apha.12725
107. Sun Z, Han Y, Song S, Chen T, Han Y, Liu Y. Activation of GPR81 by lactate inhibits oscillatory shear stress-induced endothelial inflammation by activating the expression of KLF2. IUBMB Life (2019) 71(12):2010–9. doi: 10.1002/iub.2151
108. Zhu S, Goldschmidt-Clermont PJ, Dong C. Inactivation of monocarboxylate transporter MCT3 by DNA methylation in atherosclerosis. Circulation. (2005) 112(9):1353–61. doi: 10.1161/CIRCULATIONAHA.104.519025
109. Aleksandar J, Vladan P, Markovic-Jovanovic S, Stolic R, Mitic J, Smilic T. Hyperlactatemia and the outcome of type 2 diabetic patients suffering acute myocardial infarction. J Diabetes Res (2016) 2016:6901345. doi: 10.1155/2016/6901345
110. Zhang G, Gao S, Li X, Zhang L, Tan H, Xu L, et al. Pharmacological postconditioning with lactic acid and hydrogen rich saline alleviates myocardial reperfusion injury in rats. Sci Rep (2015) 5:9858. doi: 10.1038/srep09858
111. Aresta F, Gerstenblith G, Weiss RG. Repeated, transient lactate exposure does not “precondition” rat myocardium. Can J Physiol Pharmacol (1997) 75(10-11):1262–6. doi: 10.1139/y97-138
112. Gjesdal G, Braun O, Smith JG, Scherstén F, Tydén P. Blood lactate is a predictor of short-term mortality in patients with myocardial infarction complicated by heart failure but without cardiogenic shock. BMC Cardiovasc Disord (2018) 18(1):8. doi: 10.1186/s12872-018-0744-1
113. Zymliński R, Biegus J, Sokolski M, Siwołowski P, Nawrocka-Millward S, Todd J, et al. Increased blood lactate is prevalent and identifies poor prognosis in patients with acute heart failure without overt peripheral hypoperfusion. Eur J Heart Fail (2018) 20(6):1011–8. doi: 10.1002/ejhf.1156
114. Biegus J, Zymliński R, Sokolski M, Jankowska EA, Banasiak W, Ponikowski P. Elevated lactate in acute heart failure patients with intracellular iron deficiency as identifier of poor outcome. Kardiol Pol (2019) 77(3):347–54. doi: 10.5603/KP.a2019.0014
115. Ander DS, Jaggi M, Rivers E, Rady MY, Levine TB, Levine AB, et al. Undetected cardiogenic shock in patients with congestive heart failure presenting to the emergency department. Am J Cardiol (1998) 82(7):888–91. doi: 10.1016/S0002-9149(98)00497-4
116. Metra M, Raddino R, Dei Cas L, Visioli O. Assessment of peak oxygen consumption, lactate and ventilatory thresholds and correlation with resting and exercise hemodynamic data in chronic congestive heart failure. Am J Cardiol (1990) 65(16):1127–33. doi: 10.1016/0002-9149(90)90326-V
117. Biegus J, Zymliński R, Sokolski M, Gajewski P, Banasiak W, Ponikowski P. Clinical, respiratory, haemodynamic, and metabolic determinants of lactate in heart failure. Kardiol Pol (2019) 77(1):47–52. doi: 10.5603/KP.a2018.0240
118. Adamo L, Nassif ME, Novak E, LaRue SJ, Mann DL. Prevalence of lactic acidaemia in patients with advanced heart failure and depressed cardiac output. Eur J Heart Fail (2017) 19(8):1027–33. doi: 10.1002/ejhf.628
119. Kawase T, Toyofuku M, Higashihara T, Okubo Y, Takahashi L, Kagawa Y, et al. Validation of lactate level as a predictor of early mortality in acute decompensated heart failure patients who entered intensive care unit. J Cardiol (2015) 65(2):164–70. doi: 10.1016/j.jjcc.2014.05.006
120. Biegus J, Zymliński R, Gajewski P, Sokolski M, Siwołowski P, Sokolska J, et al. Persistent hyperlactataemia is related to high rates of in-hospital adverse events and poor outcome in acute heart failure. Kardiol Pol (2019) 77(3):355–62. doi: 10.5603/KP.a2019.0030
121. Barge-Caballero E, Couto-Mallón D. Acute heart failure, iron deficiency, and hyperlactataemia: a high-risk combination. Kardiol Pol (2019) 77(3):327–8. doi: 10.5603/KP.2019.0050
122. Jóhannsson E, Lunde PK, Heddle C, Sjaastad I, Thomas MJ, Bergersen L, et al. Upregulation of the cardiac monocarboxylate transporter MCT1 in a rat model of congestive heart failure. Circulation. (2001) 104(6):729–34. doi: 10.1161/hc3201.092286
123. Evans RK, Schwartz DD, Gladden LB. Effect of myocardial volume overload and heart failure on lactate transport into isolated cardiac myocytes. J Appl Physiol (2003) 94(3):1169–76. doi: 10.1152/japplphysiol.00778.2002
124. Juraschek SP, Bower JK, Selvin E, Subash Shantha GP, Hoogeveen RC, Ballantyne CM, et al. Plasma lactate and incident hypertension in the atherosclerosis risk in communities study. Am J Hypertens (2015) 28(2):216–24. doi: 10.1093/ajh/hpu117
125. Lian H, Zhuo SQ, Tian XT, Liu FC. Increased plasma lactate level is associated with subclinical cardiovascular damage in patient with non-dipping hypertension. Clin Exp Hypertens (2016) 38(6):541–4. doi: 10.3109/10641963.2016.1174247
126. Wikander I, Roos T, Stakkestad A, Eriksson E. Sodium lactate elicits a rapid increase in blood pressure in wistar rats and spontaneously hypertensive rats. effect of pretreatment with the antipanic drugs clomipramine and alprazolam. Neuropsychopharmacology. (1995) 12(3):245–50. doi: 10.1016/0893-133X(94)00082-B
127. Marina N, Ang R, Machhada A, Kasymov V, Karagiannis A, Hosford PS, et al. Brainstem hypoxia contributes to the development of hypertension in the spontaneously hypertensive rat. Hypertension. (2015) 65(4):775–83. doi: 10.1161/HYPERTENSIONAHA.114.04683
128. Rodriguez-Iturbe B, Pons H, Johnson RJ. Role of the immune system in hypertension. Physiol Rev (2017) 97(3):1127–64. doi: 10.1152/physrev.00031.2016
129. Mattson DL. Immune mechanisms of salt-sensitive hypertension and renal end-organ damage. Nat Rev Nephrol. (2019) 15(5):290–300. doi: 10.1038/s41581-019-0121-z
130. Drummond GR, Vinh A, Guzik TJ, Sobey CG. Immune mechanisms of hypertension. Nat Rev Immunol (2019) 19(8):517–32. doi: 10.1038/s41577-019-0160-5
131. Lopez Gelston CA, Mitchell BM. Recent advances in immunity and hypertension. Am J Hypertens (2017) 30(7):643–52. doi: 10.1093/ajh/hpx011
132. Mikolajczyk TP, Guzik TJ. Adaptive immunity in hypertension. Curr Hypertens Rep (2019) 21(9):68. doi: 10.1007/s11906-019-0971-6
133. Farrag M, Drobish JK, Puhl HL, Kim JS, Herold PB, Kaufman MP, et al. Endomorphins potentiate acid-sensing ion channel currents and enhance the lactic acid-mediated increase in arterial blood pressure: effects amplified in hindlimb ischaemia. J Physiol (2017) 595(23):7167–83. doi: 10.1113/JP275058
134. Chen C, Luo F, Wu P, Huang Y, Das A, Chen S, et al. Metabolomics reveals metabolite changes of patients with pulmonary arterial hypertension in China. J Cell Mol Med (2020) 24(4):2484–96. doi: 10.1111/jcmm.14937
135. Humbert M, Guignabert C, Bonnet S, Dorfmüller P, Klinger JR, Nicolls MR, et al. Pathology and pathobiology of pulmonary hypertension: state of the art and research perspectives. Eur Respir J (2019) 53(1). doi: 10.1183/13993003.01887-2018
136. Thenappan T, Ormiston ML, Ryan JJ, Archer SL. Pulmonary arterial hypertension: pathogenesis and clinical management. BMJ (2018) 360:j5492. doi: 10.1136/bmj.j5492
137. Weiss A, Neubauer MC, Yerabolu D, Kojonazarov B, Schlueter BC, Neubert L, et al. Targeting cyclin-dependent kinases for the treatment of pulmonary arterial hypertension. Nat Commun (2019) 10(1):2204. doi: 10.1038/s41467-019-10135-x
138. Dai J, Zhou Q, Chen J, Rexius-Hall ML, Rehman J, Zhou G. Alpha-enolase regulates the malignant phenotype of pulmonary artery smooth muscle cells via the AMPK-akt pathway. Nat Commun (2018) 9(1):3850. doi: 10.1038/s41467-018-06376-x
139. Kurosawa R, Satoh K, Kikuchi N, Kikuchi H, Saigusa D, Al-Mamun ME, et al. Identification of celastramycin as a novel therapeutic agent for pulmonary arterial hypertension. Circ Res (2019) 125(3):309–27. doi: 10.1161/CIRCRESAHA.119.315229
140. Kikuchi N, Satoh K, Kurosawa R, Yaoita N, Elias-Al-Mamun M, Siddique MAH, et al. Selenoprotein p promotes the development of pulmonary arterial hypertension: possible novel therapeutic target. Circulation. (2018) 138(6):600–23. doi: 10.1161/CIRCULATIONAHA.117.033113
141. Dai Z, Li M, Wharton J, Zhu MM, Zhao YY. Prolyl-4 hydroxylase 2 (PHD2) deficiency in endothelial cells and hematopoietic cells induces obliterative vascular remodeling and severe pulmonary arterial hypertension in mice and humans through hypoxia-inducible factor-2α. Circulation. (2016) 133(24):2447–58. doi: 10.1161/CIRCULATIONAHA.116.021494
142. Michelakis ED, McMurtry MS, Wu XC, Dyck JR, Moudgil R, Hopkins TA, et al. Dichloroacetate, a metabolic modulator, prevents and reverses chronic hypoxic pulmonary hypertension in rats: role of increased expression and activity of voltage-gated potassium channels. Circulation. (2002) 105(2):244–50. doi: 10.1161/hc0202.101974
143. Halestrap AP, Denton RM. The specificity and metabolic implications of the inhibition of pyruvate transport in isolated mitochondria and intact tissue preparations by alpha-Cyano-4-hydroxycinnamate and related compounds. Biochem J (1975) 148(1):97–106. doi: 10.1042/bj1480097
144. Poole RC, Halestrap AP. Inhibition and labelling of the erythrocyte lactate transporter by stilbene disulphonates. Biochem Soc Trans (1990) 18(6):1245–6. doi: 10.1042/bst0181245
145. Le Floch R, Chiche J, Marchiq I, Naiken T, Ilc K, Murray CM, et al. CD147 subunit of lactate/H+ symporters MCT1 and hypoxia-inducible MCT4 is critical for energetics and growth of glycolytic tumors. Proc Natl Acad Sci U S A. (2011) 108(40):16663–8. doi: 10.1073/pnas.1106123108
146. Polański R, Hodgkinson CL, Fusi A, Nonaka D, Priest L, Kelly P, et al. Activity of the monocarboxylate transporter 1 inhibitor AZD3965 in small cell lung cancer. Clin Cancer Res (2014) 20(4):926–37. doi: 10.1158/1078-0432.CCR-13-2270
147. Doherty JR, Yang C, Scott KE, Cameron MD, Fallahi M, Li W, et al. Blocking lactate export by inhibiting the myc target MCT1 disables glycolysis and glutathione synthesis. Cancer Res (2014) 74(3):908–20. doi: 10.1158/0008-5472.CAN-13-2034
148. Corbet C, Bastien E, Draoui N, Doix B, Mignion L, Jordan BF, et al. Interruption of lactate uptake by inhibiting mitochondrial pyruvate transport unravels direct antitumor and radiosensitizing effects. Nat Commun (2018) 9(1):1208. doi: 10.1038/s41467-018-03525-0
Keywords: lactate, pathophysiology, cardiovascular disease, angiogenesis, signal transduction
Citation: Wu P, Zhu T, Huang Y, Fang Z and Luo F (2023) Current understanding of the contribution of lactate to the cardiovascular system and its therapeutic relevance. Front. Endocrinol. 14:1205442. doi: 10.3389/fendo.2023.1205442
Received: 13 April 2023; Accepted: 02 June 2023;
Published: 15 June 2023.
Edited by:
Guanghong Jia, University of Missouri, United StatesReviewed by:
Gabriel Komla Adzika, Xuzhou Medical University, ChinaCopyright © 2023 Wu, Zhu, Huang, Fang and Luo. This is an open-access article distributed under the terms of the Creative Commons Attribution License (CC BY). The use, distribution or reproduction in other forums is permitted, provided the original author(s) and the copyright owner(s) are credited and that the original publication in this journal is cited, in accordance with accepted academic practice. No use, distribution or reproduction is permitted which does not comply with these terms.
*Correspondence: Fei Luo, bHVvZmVpMDA1OEBjc3UuZWR1LmNu
Disclaimer: All claims expressed in this article are solely those of the authors and do not necessarily represent those of their affiliated organizations, or those of the publisher, the editors and the reviewers. Any product that may be evaluated in this article or claim that may be made by its manufacturer is not guaranteed or endorsed by the publisher.
Research integrity at Frontiers
Learn more about the work of our research integrity team to safeguard the quality of each article we publish.