- 1Center of Infectious Diseases, West China Hospital, Sichuan University, Chengdu, China
- 2West China School of Medicine, Sichuan University, Chengdu, China
- 3West China College of Stomatology, Sichuan University, Chengdu, China
- 4Key Laboratory of Birth Defects and Related Diseases of Women and Children of MOE, State Key Laboratory of Biotherapy, West China Second University Hospital, Sichuan University, Chengdu, China
- 5NHC Key Laboratory of Chronobiology, Sichuan University, Chengdu, China
- 6Sichuan Birth Defects Clinical Research Center, West China Second University Hospital, Sichuan University, Chengdu, China
Background: Nonalcoholic fatty liver disease (NAFLD) is a common chronic liver disease that affects approximately one-quarter of the global population and is becoming increasingly prevalent worldwide. The lack of current noninvasive tools and efficient treatment is recognized as a significant barrier to the clinical management of these conditions. Extracellular vesicles (EVs) are nanoscale vesicles released by various cells and deliver bioactive molecules to target cells, thereby mediating various processes, including the development of NAFLD.
Scope of review: There is still a long way to actualize the application of EVs in NAFLD diagnosis and treatment. Herein, we summarize the roles of EVs in NAFLD and highlight their prospects for clinical application as a novel noninvasive diagnostic tool as well as a promising therapy for NAFLD, owing to their unique physiochemical characteristics. We summarize the literatures on the mechanisms by which EVs act as mediators of intercellular communication by regulating metabolism, insulin resistance, inflammation, immune response, intestinal microecology, and fibrosis in NAFLD. We also discuss future challenges that must be resolved to improve the therapeutic potential of EVs.
Major conclusions: The levels and contents of EVs change dynamically at different stages of diseases and this phenomenon may be exploited for establishing sensitive stage-specific markers. EVs also have high application potential as drug delivery systems with low immunogenicity and high biocompatibility and can be easily engineered. Research on the mechanisms and clinical applications of EVs in NAFLD is in its initial phase and the applicability of EVs in NAFLD diagnosis and treatment is expected to grow with technological progress.
1 Introduction
Nonalcoholic fatty liver disease (NAFLD) has become the most common chronic liver disorder affecting approximately 25% of the global adult population, which prevalence varies from 13.5% in Africa to 31.8% in the Middle East (1), causing a growing global burden of liver diseases (2). NAFLD encompasses a disease continuum from nonalcoholic fatty liver (NAFL) to nonalcoholic steatohepatitis (NASH), which is characterized by necroinflammation and faster fibrosis progression than NAFL (3). Patients with NAFLD are prone to developing cirrhosis and hepatocellular carcinoma (HCC), making NAFLD the most rapidly growing cause of liver transplantation in HCC patients, with an 11.8-fold increase during 2002-2016 (4, 5). NAFLD has become the most rapidly growing contributor to liver mortality and morbidity (6). Drugs such as glucagon-like peptide-1 (GLP-1) agonists, pioglitazone, and sodium-dependent glucose transporter 2 (SGLT2) inhibitors are currently available for the treatment of obesity and type 2 diabetes mellitus(T2DM), but there is currently no FDA-approved drug therapy for NASH (3). A healthy lifestyle and weight management remain central to the prevention and treatment of NAFLD (7).
The pathophysiology of NASH is multifactorial, involving genetic and epigenetic factors, insulin resistance (IR), adipose-derived hormones, over-nutrition, and microbiome-related factors that are not well understood, and several studies have reported that extracellular vesicles (EVs) play a significant role in the development of NAFLD (3, 8, 9). EVs act as intercellular mediators and participate in metabolic regulation, inflammatory and immune responses, intestinal microecological balance, and fibrotic processes. Therefore, understanding the mechanism of EVs is of great significance for improving the diagnosis and treatment of NAFLD. In this review, we summarize the roles of EVs in NAFLD and highlight their utility as diagnostic and therapeutic tools in NAFLD.
2 The characteristic of extracellular vesicles
An EV is a membranous vesicle derived from the cellular membrane systems of living cells with lipid bilayer membranes (10). EVs were first observed in plasma in 1967 (11). Since then, it has been established that EVs can be isolated from a variety of body fluids (12–16) and be released by almost all types of cells (17–20). Various proteins, lipids, DNA, RNA, and metabolic products, which have been proven to regulate gene expression and signaling pathways in cells, can function as cargo for EVs (21, 22). The biosynthesis of EVs may be regulated by EV cargoes bind trafficking effectors, which enrich cargoes in endosomal and plasma membrane patches, and cause the endosomal membrane to bud into the lumen of the endosome, leading to the formation of intraluminal vesicles as early endosomes mature into late endosomal multivesicular body (23). Depending on their source, size, and function, EVs can be divided into three types—exosomes, microvesicles, and apoptotic bodies (Figure 1) (24, 25). Exosomes are the smallest EVs with a diameter of 40-120 nm and are formed due to exocytosis. Microvesicles are derived from cellular budding and have a larger size of 50-1000 nm. Both exosomes and microvesicles play a role in intercellular communication. Apoptotic bodies are always derived from dead cells and are the largest of EVs with a size of 500-2000 nm. Different surface modifications of EVs, such as exosomes, can make it have different functions, so as to achieve targeted drug delivery and in vivo imaging and tracking. Specific membrane proteins functionalized on the surface of exosomes, such as tetraspanins proteins (CD63, CD81, CD9), lactadherin, lysosome-associated membrane protein-2B, and glycosyl phosphatidylinositol, as well as different surface modification strategies such as genetic engineering, interact with the receptor system of target cells, which is involved in the regulation of physiological functions of various organ systems (26).
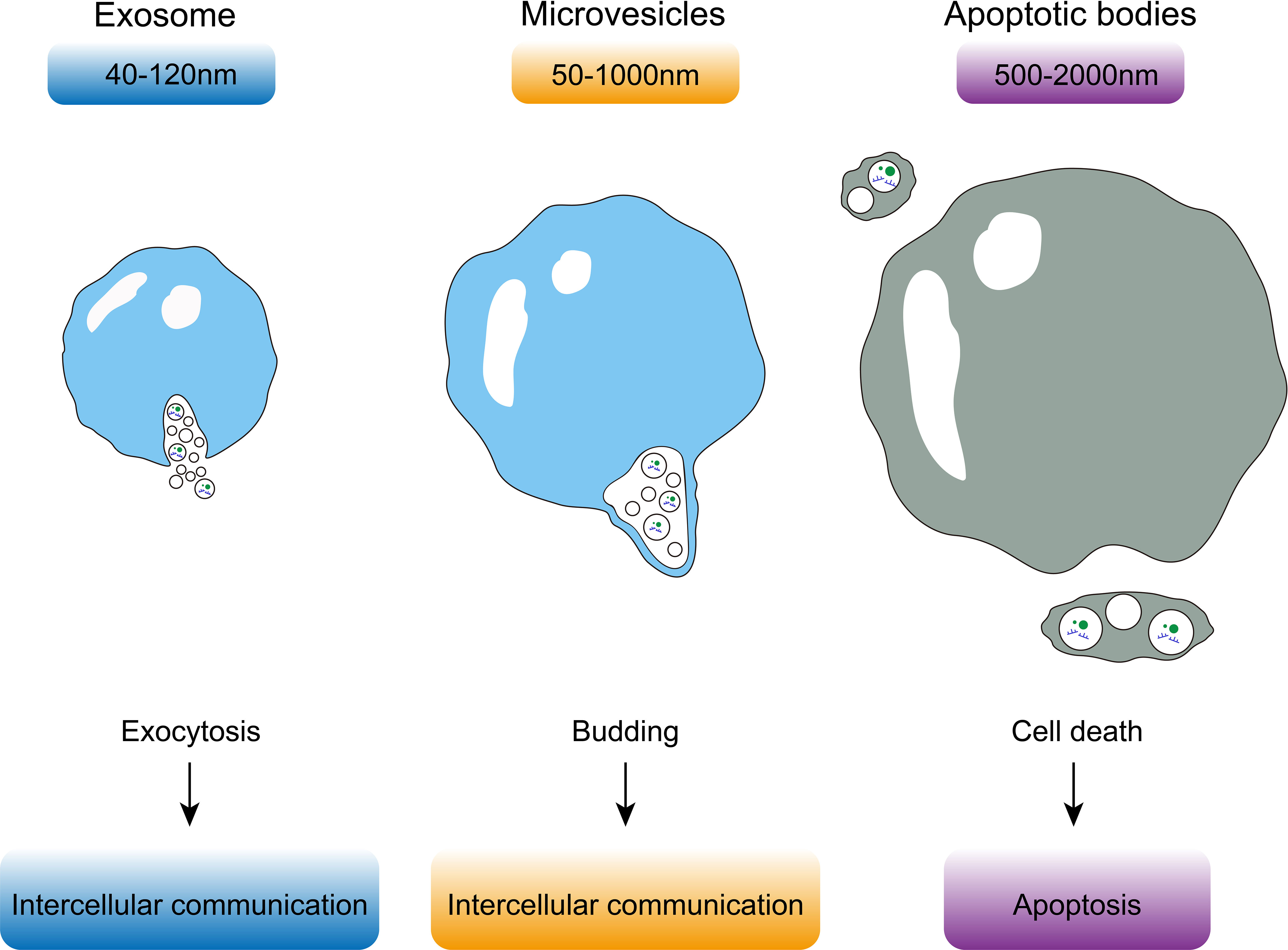
Figure 1 Exosomes are the smallest EVs with a diameter of 40-120 nm and are formed due to exocytosis. Microvesicles are derived from cellular budding and have a larger size of 50-1000 nm. Apoptotic bodies are always derived from dead cells and are the largest of EVs with a size of 500-2000 nm.
3 The role of EVs in NAFLD
EVs contain specific molecules on their surface that can induce signal transduction in specific cells by recognizing target cells and binding to cell-specific receptors or fusing with the target cell membrane and transferring the cargo into their cytoplasm to regulate the physiological activities of cells (Figure 2) (21). Based on different cell sources, EVs participate not only in normal physiological processes but also in disease processes (27–29). Consequently, EVs have potential application value as diagnostic biomarkers (30). In the liver, EVs not only play an important role in mediating signal transduction in liver cells but also affect metabolic pathways in liver cells associated with apoptosis of hepatocytes, inflammation, liver fibrosis, and the development of NAFLD (Table 1) (55, 56).
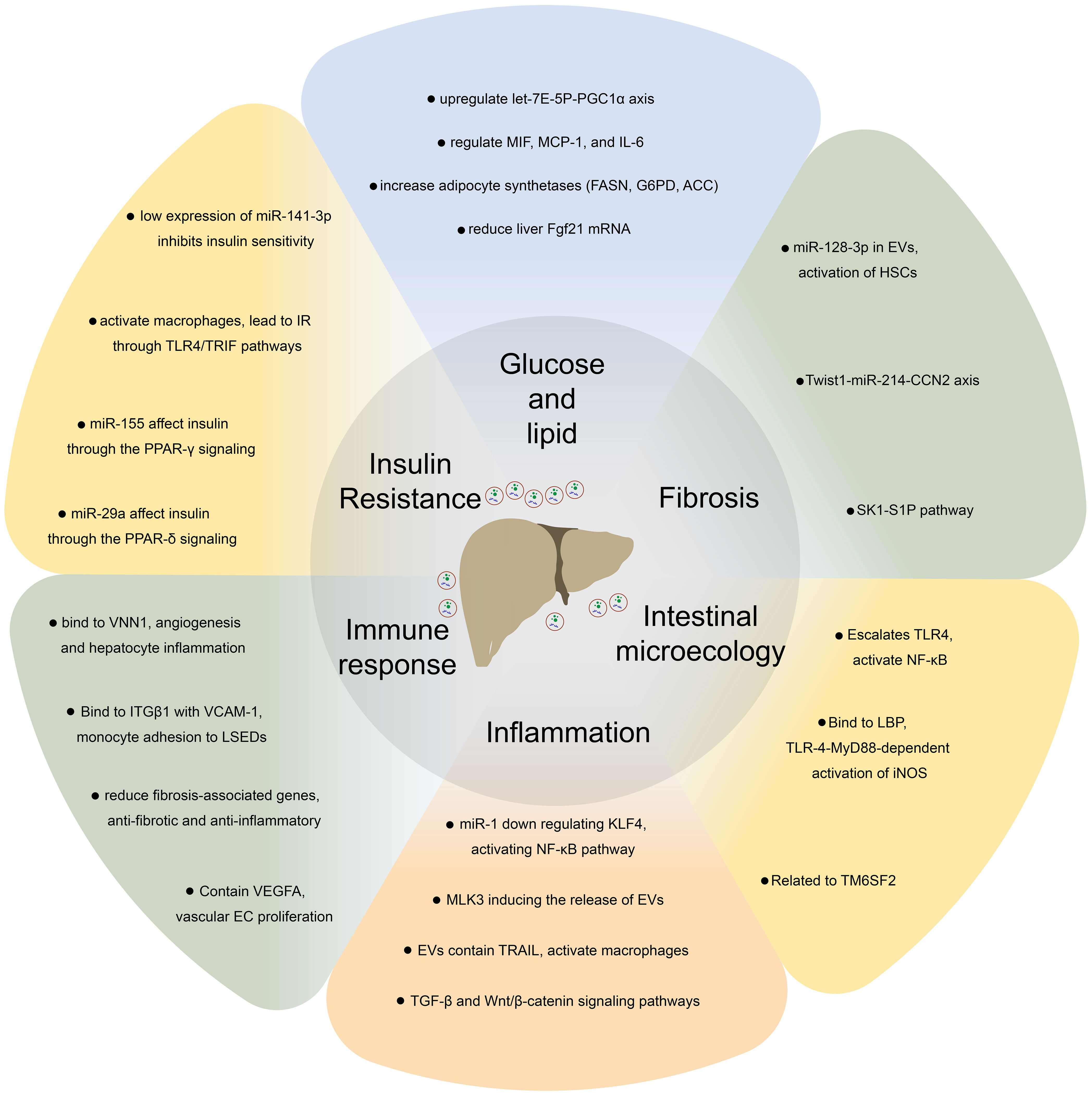
Figure 2 EVs regulate glucose and lipid metabolism, fibrosis, intestinal microecology, inflammation, immune response, insulin resistance and other processes by recognizing target cells and binding to cell-specific receptors or fusion with the target cell membrane to achieve substance transport and induce intracellular signal transduction, thus, participate in the pathophysiological process of non-alcoholic fatty liver disease.
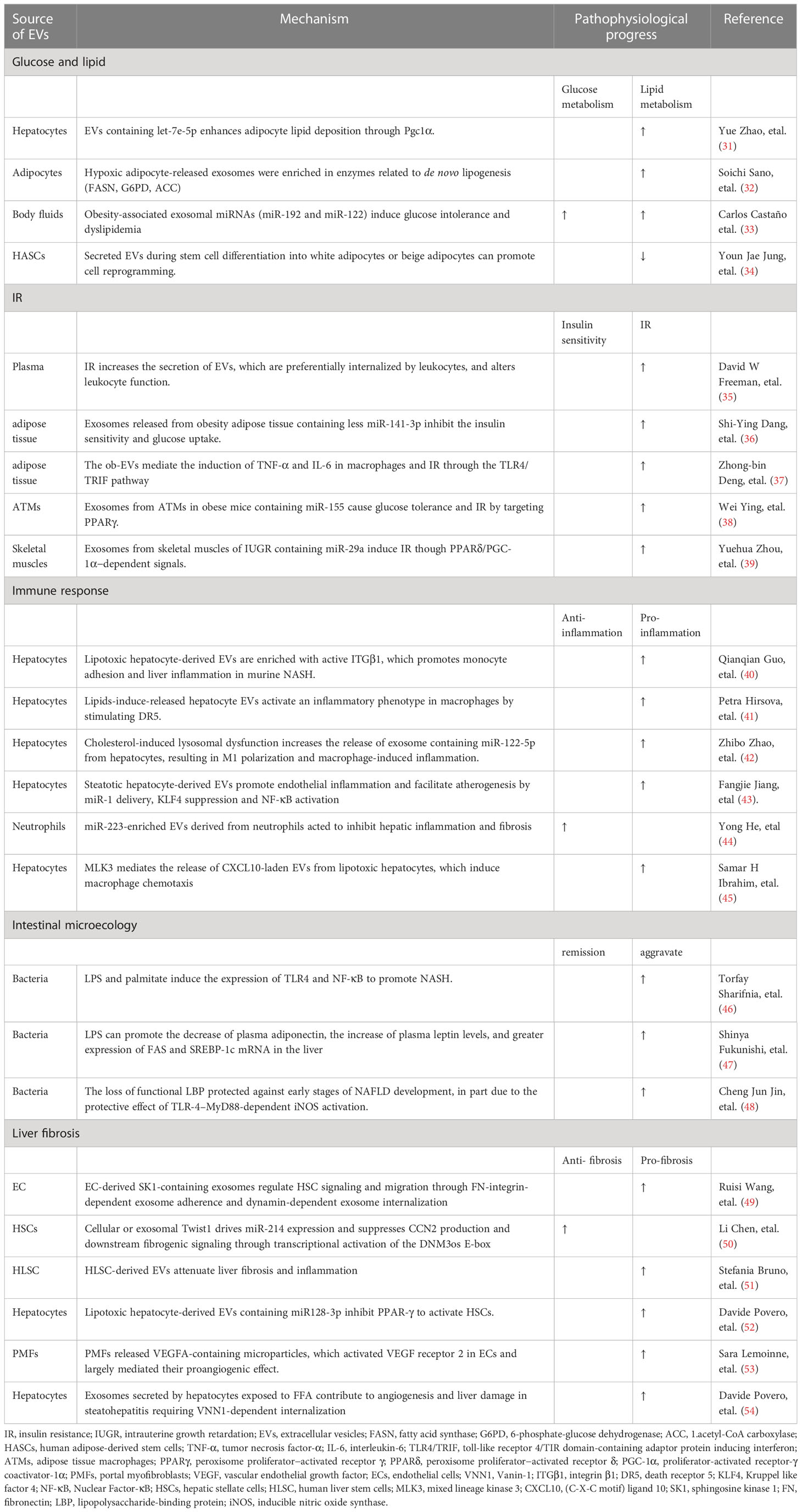
Table 1 Mechanisms of development of nonalcoholic fatty liver disease related to extracellular vesicles.
3.1 Glucose and lipid metabolism
Patients with NAFLD often have glucose and lipid metabolic disorders, which have been proven to be regulated by EVs and are associated with multiple pathways (57–59). Some research report that obese individuals have higher levels of circulating EVs than normal-weight individuals (60, 61). While, the role of EVs in regulating the glucose and lipid metabolism is multifarious. The possible mechanism may be the diversity of contents carried by exosomes, including non-coding RNAs and cytokines, which play biological functions.
A recent report indicated that adipocyte-derived EVs may induce hepatitis and cirrhosis by regulating adipose tissue homeostasis, interfering with normal signaling pathways, and causing metabolic dysfunction (22). EVs play a significant role in lipid redistribution in metabolic organs such as the liver, adipose tissues, and muscles under lipid overload. The study found that EVs levels increased in response to acute lipid overload and these EVs containing let-7e-5p fuse with adipocytes to promote adipocyte regeneration by upregulating the let-7E-5p-PGC1α axis (31). In a relatively hypoxic environment, the secretion of EVs derived from 3T3-L1 adipocytes increased. Proteomic analysis revealed 231 protein components in these EVs, including a variety of lipogenic enzymes such as fatty acid synthase (FASN), 6-phosphate-glucose dehydrogenase (G6PD), and acetyl-CoA carboxylase (ACC), which promote fat synthesis and increase adipocyte load (32). Adipocyte-derived EVs act as adipocytokines that regulate the secretion of cytokines from adjoining cells in response to a variety of stimuli. They activate macrophages and promote the synthesis and release of macrophage colony-stimulating factor, interleukin-6 (IL-6), and tumor necrosis factor-α (TNF-α), which aggravate IR, destroy gluconeogenesis in liver tissue and promote liver inflammation by adjusting the release of macrophage migration inhibitory factor (MIF), macrophage chemoattractant protein-1 (MCP-1) and IL-6 (62). EVs isolated from human adipose-derived stem cells (HASCs) generated during beige adipogenic differentiation can differentiate HASCs into beige and brown adipocytes. EVs derived from beige/brown adipocytes have beneficial effects on the browning of the white adipose tissue (22, 34). A study by Thomou et al. (63) showed that circulating EVs isolated from adipose tissue-specific miRNA knockout mice contain decreased miRNAs, and circulating miRNA levels are almost completely restored after transplantation of white/brown adipose tissue. These miRNAs play a role in improving glucose tolerance and reducing fibroblast growth factor 21(Fgf21) mRNA in hepatocytes. Another study showed that EVs containing let-7b-5p activated TGF-β-let-7b-5p signaling pathway in hepatocytes, reducing mitochondrial oxidative phosphorylation and suppressing white-to-beige fat conversion, that promoted high-fat diet (HFD)-induced steatosis and obesity (64). In summary, EVs can regulate glucose and lipid metabolism and NAFLD development by regulating gene expression or cell-specific signaling pathways. By blocking specific signaling pathways in or receptors on target cells, we can modulate the effects of EV cargo. The findings of the studies discussed above may provide new insights for the research and development of novel drugs in the future.
3.2 Insulin resistance
IR is closely related to liver steatosis and can also predict the development of NAFLD (65). Recent studies have revealed that EVs are easily internalized by cells and cause functional changes in specific tissues, regulating insulin signaling in other tissues (35). Adipocyte-derived exosomes can cause IR. The first study that identified the role of adipocyte-EVs in IR was performed in a mice model of obesity (37). EVs released by adipocytes can activate macrophages, which can induce IR through toll-like receptor 4/TIR domain-containing adaptor protein inducing interferon-β (TLR4/TRIF) pathways (37). Dang et al. (36) proposed that IR in obese individuals is highly correlated with the low expression of miR-141-3p in exosomes secreted by adipose tissues. EVs released by adipose tissue from obese mouse models can mediate crosstalk between adipose tissues and macrophages. Additionally, some studies have suggested that insulin sensitivity is related to macrophages that reside within adipose tissue (ATMs). Another study on ATMs found that miR-155 in exosomes released by ATMs was overexpressed in an obese mouse model (66). And it is reported that miR-29a was overexpressed in exosomes derived from ATMs in obese mouse models (38). miR-155 and miR-29a are key mediators s in the peroxisome proliferation-activated receptor-γ (PPAR-γ) and PPAR-δ signaling pathways, respectively. Both PPAR-γ and PPAR-δ have been identified as targets of miRNAs that regulate IR (39). The studies suggest that ATMs can impair insulin sensitivity by secreting exosomes containing specific miRNAs, leading to the inhibition of glucose uptake and directly affecting the insulin levels in an organism. Anja Fuchs et al. (67) found that systemic IR in people with obesity and NAFLD is associated with increased plasma PAI-1 concentrations and both plasma and subcutaneous abdominal adipose tissue derived exosomes. In addition, gut microbial-derived EVs can also influence glucose metabolism by regulating IR (68). It was found that fecal-derived EVs induced IR and poor glucose tolerance in high-fat diet (HFD)-fed mice compared to conventional diet-fed mice (69). In summary, EVs have been implicated in the development of IR and understanding the molecular mechanisms by which they confer IR may be effective in the prevention or treatment of NAFLD.
3.3 Immune response
The persistent inflammatory response is an important cause of the transition from simple fatty liver disease to severe liver injury, such as steatohepatitis and cirrhosis, which is related to the immune response (70). Immune regulation, including innate and adaptive immunity, is crucial to the pathogenesis of NAFLD. Innate immune cells in the liver include Kupffer cells, dendritic cells, natural killer (NK) cells, innate lymphoid cells, invariant NKT cells, and mucosal-associated invariant T cells, which form the first line of defense against invading organisms and environmental challenges. The hepatic innate immune response plays a prominent role in the progression of liver disease; therefore, it is an important driving force in NAFLD (71). Increasing evidence suggests the role of lymphocyte-mediated adaptive immunity as a factor promoting liver inflammation, including the role of B cells and CD4+T and CD8+T cells in sustaining NASH progression (72).
3.3.1 Innate immunity
Patients with NAFLD show increased levels of EVs derived from macrophages and NK cells. The levels of EVs derived from immune cells can be used to assess the extent of chronic liver disease, which is related to the enhancement of innate immune function during the development of NAFLD (73). EVs promote pathological angiogenesis and fibrosis in NASH by transporting a variety of mediators including growth factors, hedgehog molecules, proteins, and miRNAs (74). A study by Pover et al. (54) showed that under saturated lipotoxicity, the caspase8-caspase3-ROCK1 pathway in hepatocytes is activated, releasing EVs containing a large amount of Vanin-1, which can reinforce the internalization of EVs, initiate the migration of endothelial cells (ECs), and promote the generation of new small blood vessels, resulting in hepatocyte inflammation. Lemoinne et al. (53) showed that EVs carrying vascular endothelial growth factor A (VEGF-A) can be released by activated portal myofibroblasts and bind to VEGF-A receptors on vascular EC to promote vascular ECs and ductal hyperplasia. Vascular ECs co-cultured with steatotic hepatocytes or treated with steatotic hepatocyte-derived EVs decreased Kruppel-like factor 4 release, which activated the intracellular NF-κB pathway and significantly increased pro-inflammatory factor release (43).
Lipotoxic liver cells can release EVs that contain various macrophage chemokines and active mediators. Protein mass spectrometry of EVs showed that EVs contain many damage-associated molecular patterns, which can activate the inflammatory response in mammals (75). Lipid molecules can promote NAFLD by activating cytokines in hepatocytes. To activate the death receptor 5 (DR5) of hepatocytes through non-ligand-dependent pathways, the secretion of EVs in hepatocytes increase. TNF-related apoptosis-inducing ligand (TRAIL) on the surface of the EVs activates DR5-RIP1-NF-κB signaling pathway in macrophages to increase the secretion of IL-1β and IL-6 (41), which aggravates inflammation in hepatocytes. EVs carrying miR-122-5p secreted by hepatocytes can stimulate the secretion of pro-inflammatory factors and polarize hepatic macrophages into the M1 phenotype (42). Studies also have reported that EVs can mediated the macrophages, which is supposed to play an important role in the regulation of fibrosis (76, 77). Several studies have demonstrated that NAFLD are associated with exosomes derived from or transferred to macrophages (78). For example, exosomal miRNA-411-5p derived from M2 macrophages plays an inhibitory role in HSCs activation during NASH progression by inhibiting its target gene CAMSAP1 (79). Furthermore, the exosomes released by lipotoxic hepatocytes can be ingested by macrophages, resulting in activation of M1 macrophages and hepatic inflammation by regulating the Rictor/Akt/FoxO1 signaling pathway (80). Hepatocyte-derived EVs can also promote monocyte adhesion via an integrin β1-dependent mechanism to induce an inflammatory response (40). Besides, under the inflammation or mechanical stimulation, the hepatic stellate cells (HSCs) activated and participate in the formation of liver fibrosis through the proliferation and secretion of the extracellular matrix. One possible mechanism for this transformation is through the upregulation and release of miR128-3p by EVs under lipotoxicity caused by increased free fatty acids in hepatocytes (64). These hepatocyte-derived EVs are internalized by HSCs and inhibit PPAR-γ in quiescent HSCs to facilitate phenotypic conversion (52). When HSCs were exposed to miR128-3p-deficient EVs, a higher PPAR-γ level and reduced proliferation and migration were observed. EVs containing connective tissue growth factor or miR214 promote the phenotypic transformation of activated HSCs (81). This is a possible mechanism of the translation from NAFLD to liver fibrosis and NASH. However, some studies suggest that EVs derived from hepatocytes can significantly downregulate the expression of genes related to fibrosis and have anti-inflammatory and anti-fibrotic effects. Neutrophil-derived miR-223 with high apolipoprotein E expression can be taken up by hepatocytes to limit the progression of steatosis to NASH (44). Fibrosis-related genes were significantly downregulated in immune-deficient NASH mice (methionine-choline-deficient diet-induced) that were treated with human hepatocyte-derived EVs (51). EVs from human liver stem cells are believed to slow down the symptoms of fibrosis and inflammation by regulating gene expression in liver cells. Therefore, the role of EVs in innate immunity appears to be dynamic and must be further investigated.
3.3.2 Adaptive immunity
The current concept is that innate immunity represents a key element in development of NAFLD, however, adaptive immunity is increasingly being recognized as an additional factor of NAFLD (72). NASH is characterized by increased levels of liver and circulating IFN-γ-producing CD4+ T cells (82). CD4+ T cells can differentiate into T helper 17 cells that release IL-17. IL-17 can promote M1-type macrophage polarization and exacerbate the liver inflammatory response to accelerate NAFLD progression (83). Mice lacking CD8+ T cells and NKT cells are protected from steatosis and NASH when fed with a choline-deficient HFD, which is associated with reduced production of LIGHT by CD8+T cells and NKT cells (84). Adaptive immunity and innate immunity are not completely independent, and there is an interplay between the two. Sun et al. (85) showed that OX40 was a key regulator of intrahepatic innate and adaptive immunity and mediated two-way signals and promotes both pro-inflammatory monocytes and macrophages, as well as T cell function, resulting in the development of NASH. By promoting NK cell activation, lymphocytes stimulate the secretion of IL-15 and IL-18 by macrophages, thereby modulating the progression of steatohepatitis and fibrogenesis (86). In conclusion, adaptive immune responses are crucial in the progression of NAFLD. EVs have been proven to be key factors in mediating adaptive immune responses by playing roles in antigen presentation, T-cell activation, T-cell polarization to regulatory T-cells, and immune suppression (87). Therefore, EVs play a role in NAFLD through the modulation of adaptive immunity.
3.4 Inflammation
Recently, many studies have found that EV levels significantly increase in NASH mice models (54, 75). HFD promotes the release of EVs, and the number of EVs increases in a time-dependent manner (88). MiR-1 in hepatocyte-derived EVs is an important factor in the promotion of endothelial inflammation. EVs aggravate not only endothelial inflammation but also atherosclerosis by delivering miR-1, which induces the inhibition of Kruppel like factor 4 (KLF4) and activation of the NF-κB pathway (43). In addition to promoting inflammation in endothelial cells, EVs also mediate inflammation through macrophages. Several studies have shown that the aggregation of Kupffer cells is closely related to hepatocyte-derived EV levels (75, 89–91), suggesting that EVs mediate the inflammatory response in liver damage by inducing chemotaxis of macrophages. In lipotoxic hepatocytes, the activated mixed lineage kinase 3 pathway promotes EV secretion by upregulating c-Jun N-terminal kinase. The secreted EVs further mediate chemotaxis of macrophages by releasing C-X-C motif ligand 10 (CXCL10) via binding to C-X-C receptor-3 (CXCR-3) and promoting macrophage-associated hepatic inflammation (45). Garcia-Martinez, et al. (92) found higher levels of Mitochondrial DNA (mtDNA) in EVs of mice and patients with NASH, with concurrent increase in hepatocyte-specific marker that activate toll-like receptor 9 (TLR9). TLR9 can mediate inflammation, thereby contributing to the transition from simple steatosis to steatohepatitis. Another study has shown that the mechanism of released EVs is related to the activation of the DR5 signaling pathway and the activation of macrophages by TRAIL of the released EVs to promote a metabolic response (41). Ferrante et al. (93) analyzed EVs shed by adipocytes from obese people and confirmed that adipocyte-derived EVs participate in transforming growth factor (TGF)-β and Wnt/β-catenin signaling pathways through miRNAs, which promote inflammation and fibrosis. In summary, the lipotoxicity in hepatocytes promotes the release of EVs, and increased EVs mediate the inflammatory response by enabling intercellular interaction.
3.5 Intestinal microecology
Intestinal microorganisms produce a variety of proteins and bile acids, participate in bidirectional communication along the enterohepatic axis, and regulate intestinal microecology. Damage to gut microflora balance, such as changes in intestinal microflora composition and intestinal bacterial metabolites, plays an important role in regulating the development of NAFLD (58). Many bacteria-derived molecules, including nucleic acids, proteins, polysaccharides, and glycolipids, exist in microbe-derived EVs (21). These EVs not only support the survival of bacteria by delivering virulence factors and nutrients but also participate in the regulation of multiple signaling pathways in host cells (94). They influence NAFLD by regulating glucose and fat metabolism, immune responses, and redox balance (95).
Bacterial EVs can trigger multiple metabolic cascades and immune responses (95). Bacteria-derived EVs contain and transfer lipopolysaccharide (LPS), enter hepatocytes via the biliary tract, portal vein, and enterohepatic axis, and aggravate NAFLD. These EVs can induce liver inflammation by activating the TLR4-TRIF-GBPs signaling pathway (96) or delivering LPS into the cytosol of host cells to activate caspase-11, which regulates the immune response (97). Compared to patients with NAFLD, patients with NASH show a significant increase in LPS and free fatty acid (FFA), as well as an increase in TLR4 mRNA and interferon regulatory factor 3 (IRF-3) in the myeloid differentiation factor 88-independent signaling pathway. In addition, when using small interfering RNA-mediated TLR4 inhibitors, the inductive effect of LPS on NF-κB was weakened, suggesting that LPS can affect the TLR4-mediated NF-κB signaling pathway (46). TLR4 activates downstream signaling pathways that stimulate the release of cytokines and chemokines, leading to liver damage (47).
LPS-binding protein (LBP) and CD14 also participate in recognizing LPS, which is increased in NASH and NAFLD patients. LBP knockout in mice and subsequent prevention of LPS and TLR4 binding improved lipid metabolism in mice, protecting them from developing NAFLD under HFD conditions (48), suggesting that LBP is a crucial factor in NAFLD development. LBP and LPS levels have been shown to be associated with the development of NASH and fibrosis (98). Short RNA (sRNA) from bacteria-derived EVs can participate in regulating the innate immune response in host animals (99). Thus, damage to intestinal microecology can promote NAFLD by regulating intestinal bacteria-derived EVs containing sRNA or LPS.
As discussed above, gut microbiota-derived EVs may affect NAFLD through different mechanisms. Therefore, augmentation of beneficial gut microbes is a potential therapeutic approach. Previous studies have found that probiotics, prebiotics and other products can improve the condition of NAFLD patients (100). For example, ingestion of Lactobacillus acidophilus La5 and Bifidobacterium lactis Bb12 improved liver enzyme, serum total cholesterol, and LDL cholesterol levels in patients with NAFLD (101). Prebiotics significantly reduced TNF-α, CRP, liver enzymes, and steatosis in patients (102). Considering the potential benefits of probiotic transplantation and prebiotics in the treatment of NAFLD, combined with the role of EVs in NAFLD, we believe that this will be a direction of great research potential in the future, but further in-depth research is still needed.
3.6 Liver fibrosis
In addition to promoting inflammation, EV levels can influence liver fibrosis. Studies have shown that EVs can induce the activation of HSCs (52, 56, 103) and transmit information between liver cells and HSCs (104). Many studies have confirmed that HSC activation and proliferation are closely related to liver fibrosis (105). A study showed that miR-128-3p in EVs plays a crucial role in HSC activation, indicating that hepatocyte-derived EVs can mediate HSC activation through endocytosis (52). Additionally, HSCs can deliver connective tissue growth factor (CCN2) via the secretion of EVs. Besides CCN2, Twist1 and miR-214, which comprise the Twist1-miR-214-CCN2 axis in HSCs, also mediate fibrosis through delivery by EVs (50). Moreover, the migration of HSCs is affected by EC-derived EVs containing sphingosine kinase 1 (SK1), which mediates HSC chemotaxis through the SK1-S1P pathway (49). Besides, Studies have reported that PTEN has been proved to play an important role in the fibrosis in kidney (106) and liver (107) and highly related to exosome. For example, lipotoxic hepatocytes exosome transplantation aggravated the degree of PTEN-induced expression of putative protein kinase 1 (PINK1) mediated mitophagy suppression, steatohepatitis, lipidosis, and fibrosis in the livers of NAFLD mice with cirrhosis (108). Research have found that transfer of circDIDO1 mediated by MSC-isolated exosomes can suppress HSC activation through the miR-141-3p/PTEN/AKT pathway to suppress the proliferation, reduce pro-fibrotic markers, and induce apoptosis as well as cell cycle arrest in HSCs (109). The lipotoxic hepatocyte-derived exosomal miR-1297 could promote the activation and proliferation of HSCs through the PTEN/PI3K/AKT signaling pathway, accelerating the progression of NAFLD (110). In conclusion, as mediators of communication between cells, EVs play a significant role in the development of liver fibrosis by interacting with HSCs in different ways, such as by regulating specific signaling pathways.
4 Applications of EVs in NAFLD
We summarized the ways in which EVs play an important role in glucose and lipid metabolism, insulin residence, immune response, inflammation, intestinal microecology, and fibrosis in NAFLD. Several studies investigating the biological mechanisms of EVs have addressed their utility in the diagnosis and treatment of complex pathologies. Owing to the complex cargo and delivery functions of EVs, they can be used as part of a multicomponent diagnostic strategy for disease detection and as a targeting vehicle for disease therapy (111). Herein, we discuss the potential diagnostic and therapeutic applications of EVs.
4.1 Diagnostic utility of EVs in NAFLD
There is currently no reliable method to diagnose or stage NAFLD except via invasive liver biopsy. Some studies have shown that the components in circulating EVs, such as RNAs and proteins, provide new evidence for the diagnosis of NAFLD and NASH (112), suggesting the potential of liquid biopsy as a noninvasive and accurate approach to diagnose and monitor NAFLD (113, 114). Therefore, EVs as biomarkers can be measured in body fluids and may be a promising noninvasive method for diagnosing NAFLD, overcoming some limitations of surgical biopsy (25, 112). For example, miR-135a-3p-enriched EVs have been proven to be an accurate and sensitive biomarker in NAFLD. It has been shown that the amount of circulating EVs was significantly increased after 8 weeks L-amino acid defined diet, and miRNA-122 and miR-192 are enriched in circulating EVs in NAFLD (115, 116). Therefore, the EV levels change at the early stage of NAFLD, and can be traced to identify the latent development of potential fatty liver disease at an early stage; this may be valuable for the early diagnosis of NAFLD. The contents in EVs also change dynamically at different stages during the progression of NAFLD (25) and can be exploited for identifying biomarkers for sensitively monitoring the progression of NAFLD (117). Newman et al. (118) found a stable predictive performance for total cell-free RNA and EV derived miR-128-3p in health people, NAFL and NASH patients. Therefore, EV-derived miRNA biomarkers can robustly distinguish patients with NAFL and NASH and show the severity of NAFLD.
In addition to NAFLD, EVs have been used as biomarkers in liquid biopsies for cancer diagnosis, monitoring, and prognosis (119, 120). The development of engineered EVs as individualized imaging diagnostic reagents and for facilitating targeted therapy has been proposed (121). Many exosome sensing technologies including exosome chips, EV array, and proteomic platforms, are designed to detect EVs in cancers, and CD26, CD81, and CD10 have been proposed as markers for the detection of hepatic damage associated with liver cancer (120, 122–124).
In recent years, researchers have also found that the composition of circulating exosome content in peripheral blood may be significantly changed in obese patients after bariatric surgery, and the content of circulating exosomes may be used as a serological marker to evaluate the prognosis of bariatric surgery (125–127). For example, it is reported that the microRNA content of circulating adipocyte-derived exosomes isolated from the peripheral blood are significantly modified following gastric bypass bariatric surgery and these changes are correlated to improvements in IR post-surgery (128).Another study found that total circulating EVs and hepatocyte-derived EVs are elevated in NAFLD and decrease following NAFLD resolution due to weight loss surgery, which may be new biomarkers for NAFLD resolution and response to weight loss surgery (129). In conclusion, the changes in the types and quantities of peripheral exosome contents may be used as a new indicator to evaluate the efficacy of preoperative and postoperative bariatric surgery.
4.2 Therapeutic utility of EVs in NAFLD
EVs have potential benefits as key mediators of cell therapy because of their advantageous features of product stability, immune tolerability, effectiveness in systemic delivery, and efficacy enhancement (130).
Currently, many studies have explored the therapeutic application of EVs (131). These include the use of mesenchymal stem cell (MSC)-derived EVs in the treatment of SARS-CoV-2-associated pneumonia (132) and the use of ticagrelor to decrease the release of procoagulant EVs from activated platelets to treat patients with myocardial infarction (133). EV-based antitumor and antibacterial vaccines have shown good safety and tolerance in patients with advanced melanoma and non-small cell lung cancer (134). Some EV cargos alleviate NAFLD.
After treatment with MSC exosomes, the levels of blood glucose and insulin, volume of visceral fat, number of lipid droplets, ballooning degeneration in liver tissue, and NAFLD activity score decreased in NASH mice. MSC exosomes can alleviate fatty liver in NASH mice and promote M2 polarization of macrophages (our unpublished data). A melanocorticosterone type 4 receptor knockout NASH mouse model challenged with LPS showed that treatment with MSC-derived EVs had anti-inflammatory and anti-fibrotic effects (135). Many studies indicate that the development of drugs to inhibit the expression of certain genes or signaling pathways with EVs participation may prevent lipid deposition and fibrosis (70, 136, 137). For example, the ROCK1 inhibitor fasudil can effectively block lipotoxicity-induced EV release in mouse models and prevent NASH progression in vivo (41).
As natural carriers of functional small RNA and proteins, EVs also have high application potential as drug delivery systems with low immunogenicity and high biocompatibility for chemotherapy (138, 139). In addition, EVs can be engineered to enhance bioactivity and targeting ability, avoid undesired and unnecessary cell toxicity, and enhance therapeutic effects (140). Zhang et al. found that compared with chemotherapy alone, umbilical cord-derived macrophage exosomes loaded with cisplatin significantly increased cytotoxicity in drug-resistant ovarian cancer cells (A2780/DDP and A2780 cells) (141), and TNF-α-loaded EV-based vehicles enhanced cancer-targeting under a magnetic field and suppressed tumor growth in murine melanoma subcutaneous models (142). Studies have also shown that exosomes loaded with doxorubicin have the same efficacy as doxorubicin and prevent cardiotoxicity (143). Therefore, EVs are capable of safe and efficient drug delivery and provide a viable alternative to conventional drug delivery in NAFLD.
Synthetic exosome mimics have been fabricated as therapeutic tools for drug delivery and have been reported to have therapeutic effects (144). However, most of these studies are in the laboratory research stage; therefore, it is also necessary to establish reliable assays to assess the therapeutic potential of EVs and further develop them into formal potency tests for promoting the clinical applicability of EVs (145).
5 Limitation
The limitations of this study include three aspects below. Firstly, most of our research results are from the laboratory, clinical research data is insufficient. Secondly, current standards of EV detection methods are not consolidated, so it is necessary to further test and standardize the detection technology. Thirdly, current studies are limited to published articles, while ongoing studies are not included. So, it is supposed to track the updated research results.
6 Conclusion
EVs contain various biological molecules, including proteins, nucleic acids, and lipids; they play an important role in intercellular communication in various biological processes, including the development and progression of diseases such as NAFLD. EVs participate in different signaling pathways to regulate the initiation and progression of NAFLD. As natural carriers of biological molecules, EVs have potential advantages in the treatment of NAFLD. Circulating EVs have been considered potential diagnostic and prognostic biomarkers fin NAFLD. Exploring the precise mechanism of EVs in NAFLD will help us identify new biomarkers. Research on the mechanisms and clinical applications of EVs in NAFLD is in its initial phase and the applicability of EVs in NAFLD diagnosis and treatment is expected to grow with technological progress.
Author contributions
YX, J-CC, and WJ are responsible for collecting and sorting the literature and writing the paper. Y-HL, YH, C-HL, EC and HT are responsible for supplementing, revising and improving content. HZ and DW are responsible for guidance and proofreading. All authors contributed to the article and approved the submitted version.
Funding
This research was supported by 1.3.5 project for disciplines of excellence, West China Hospital, Sichuan University (No. ZYGD20009), the Science and Technological Supports Project of Sichuan Province, China (No. 2022YFS0338), Natural Science Foundation of Sichuan Province (No. 2022NSFSC0732), Post-Doctor Research Project of West China Hospital of Sichuan University (No. 2023HXBH112 and No. 2020HXBH079).
Conflict of interest
The authors declare that the research was conducted in the absence of any commercial or financial relationships that could be construed as a potential conflict of interest.
Publisher’s note
All claims expressed in this article are solely those of the authors and do not necessarily represent those of their affiliated organizations, or those of the publisher, the editors and the reviewers. Any product that may be evaluated in this article, or claim that may be made by its manufacturer, is not guaranteed or endorsed by the publisher.
References
1. Younossi ZM, Koenig AB, Abdelatif D, Fazel Y, Henry L, Wymer M. Global epidemiology of nonalcoholic fatty liver disease-meta-analytic assessment of prevalence, incidence, and outcomes. Hepatology (2016) 64(1):73–84. doi: 10.1002/hep.28431
2. Estes C, Razavi H, Loomba R, Younossi Z, Sanyal AJ. Modeling the epidemic of nonalcoholic fatty liver disease demonstrates an exponential increase in burden of disease. Hepatology (2018) 67(1):123–33. doi: 10.1002/hep.29466
3. Powell EE, Wong VW, Rinella M. Non-alcoholic fatty liver disease. Lancet (2021) 397:2212–24. doi: 10.1016/S0140-6736(20)32511-3
4. Wong RJ, Cheung R, Ahmed A. Nonalcoholic steatohepatitis is the most rapidly growing indication for liver transplantation in patients with hepatocellular carcinoma in the U.S. Hepatology (2014) 59(6):2188–95. doi: 10.1002/hep.26986
5. Younossi Z, Stepanova M, Ong JP, Jacobson IM, Bugianesi E, Duseja A, et al. Nonalcoholic steatohepatitis is the fastest growing cause of hepatocellular carcinoma in liver transplant candidates. Clin Gastroenterol Hepatol (2019) 17(4):748–755.e3. doi: 10.1016/j.cgh.2018.05.057
6. Paik JM, Golabi P, Younossi Y, Mishra A, Younossi ZM. Changes in the global burden of chronic liver diseases from 2012 to 2017: the growing impact of NAFLD. Hepatology (2020) 72(5):1605–16. doi: 10.1002/hep.31173
7. Yoshioka N, Ishigami M, Watanabe Y, Sumi H, Doisaki M, Yamaguchi T, et al. Effect of weight change and lifestyle modifications on the development or remission of nonalcoholic fatty liver disease: sex-specific analysis. Sci Rep (2020) 10(1):481. doi: 10.1038/s41598-019-57369-9
8. Ipsen DH, Tveden-Nyborg P. Extracellular vesicles as drivers of non-alcoholic fatty liver disease: small particles with big impact. Biomedicines (2021) 9(1):93. doi: 10.3390/biomedicines9010093
9. Huang DQ, El-Serag HB, Loomba R. Global epidemiology of NAFLD-related HCC: trends, predictions, risk factors and prevention. Nat Rev Gastroenterol Hepatol (2021) 18(4):223–38. doi: 10.1038/s41575-020-00381-6
10. Hernández A, Arab JP, Reyes D, Lapitz A, Moshage H, Bañales JM, et al. Extracellular vesicles in NAFLD/ALD: from pathobiology to therapy. Cells (2020) 9(4):817. doi: 10.3390/cells9040817
11. Wolf P. The nature and significance of platelet products in human plasma. Br J Haematol (1967) 13(3):269–88. doi: 10.1111/j.1365-2141.1967.tb08741.x
12. De Broe M, Wieme R, Roels F. Letter: membrane fragments with koinozymic properties released from villous adenoma of the rectum. Lancet (1975) 2(7946):1214–5. doi: 10.1016/S0140-6736(75)92709-9
13. Benz EW Jr., Moses HL. Small, virus-like particles detected in bovine sera by electron microscopy. J Natl Cancer Inst (1974) 52(6):1931–4. doi: 10.1093/jnci/52.6.1931
14. Dalton AJ. Microvesicles and vesicles of multivesicular bodies versus "virus-like" particles. J Natl Cancer Inst (1975) 54(5):1137–48. doi: 10.1093/jnci/54.5.1137
15. Stegmayr B, Ronquist G. Promotive effect on human sperm progressive motility by prostasomes. Urol Res (1982) 10(5):253–7. doi: 10.1007/BF00255932
16. Aalberts M, van Dissel-Emiliani FM, van Adrichem NP, van Wijnen M, Wauben MH, Stout TA, et al. Identification of distinct populations of prostasomes that differentially express prostate stem cell antigen, annexin A1, and GLIPR2 in humans. Biol Reprod (2012) 86(3):82. doi: 10.1095/biolreprod.111.095760
17. Bobrie A, Colombo M, Raposo G, Théry C. Exosome secretion: molecular mechanisms and roles in immune responses. Traffic (2011) 12(12):1659–68. doi: 10.1111/j.1600-0854.2011.01225.x
18. Chaput N, Théry C. Exosomes: immune properties and potential clinical implementations. Semin Immunopathol (2011) 33(5):419–40. doi: 10.1007/s00281-010-0233-9
19. Simons M, Raposo G. Exosomes–vesicular carriers for intercellular communication. Curr Opin Cell Biol (2009) 21(4):575–81. doi: 10.1016/j.ceb.2009.03.007
20. Théry C, Ostrowski M, Segura E. Membrane vesicles as conveyors of immune responses. Nat Rev Immunol (2009) 9(8):581–93. doi: 10.1038/nri2567
21. Yáñez-Mó M, Siljander PRM, Andreu Z, Bedina Zavec A, Borràs FE, Buzas EI, et al. Biological properties of extracellular vesicles and their physiological functions. J Extracellular Vesicles (2015) 4(1):27066. doi: 10.3402/jev.v4.27066
22. Li CJ, Fang QH, Liu ML, Lin JN. Current understanding of the role of adipose-derived extracellular vesicles in metabolic homeostasis and diseases: communication from the distance between cells/tissues. Theranostics (2020) 10(16):7422–35. doi: 10.7150/thno.42167
23. Dixson AC, Dawson TR, Di Vizio D, Weaver AM. Context-specific regulation of extracellular vesicle biogenesis and cargo selection. Nat Rev Mol Cell Biol (2023) 24:454–76. doi: 10.1038/s41580-023-00576-0
24. Borges FT, Reis LA, Schor N. Extracellular vesicles: structure, function, and potential clinical uses in renal diseases. Braz J Med Biol Res (2013) 46(10):824–30. doi: 10.1590/1414-431X20132964
25. Ban LA, Shackel NA, McLennan SV. Extracellular vesicles: a new frontier in biomarker discovery for non-alcoholic fatty liver disease. Int J Mol Sci (2016) 17(3):376. doi: 10.3390/ijms17030376
26. Salunkhe S, Dheeraj, Basak M, Chitkara D, Mittal A. Surface functionalization of exosomes for target-specific delivery and in vivo imaging & tracking: strategies and significance. J Control Release (2020) 326:599–614. doi: 10.1016/j.jconrel.2020.07.042
27. Becker A, Thakur BK, Weiss JM, Kim HS, Peinado H, Lyden D. Extracellular vesicles in cancer: cell-to-Cell mediators of metastasis. Cancer Cell (2016) 30(6):836–48. doi: 10.1016/j.ccell.2016.10.009
28. Robbins PD, Morelli AE. Regulation of immune responses by extracellular vesicles. Nat Rev Immunol (2014) 14(3):195–208. doi: 10.1038/nri3622
29. Maus RLG, Jakub JW, Nevala WK, Christensen TA, Noble-Orcutt K, Sachs Z, et al. Human melanoma-derived extracellular vesicles regulate dendritic cell maturation. Front Immunol (2017) 8:358. doi: 10.3389/fimmu.2017.00358
30. Zhou X, Xie F, Wang L, Zhang L, Zhang S, Fang M, et al. The function and clinical application of extracellular vesicles in innate immune regulation. Cell Mol Immunol (2020) 17(4):323–34. doi: 10.1038/s41423-020-0391-1
31. Zhao Y, Zhao MF, Jiang S, Wu J, Liu J, Yuan XW, et al. Liver governs adipose remodelling via extracellular vesicles in response to lipid overload. Nat Commun (2020) 11(1):719. doi: 10.1038/s41467-020-14450-6
32. Sano S, Izumi Y, Yamaguchi T, Yamazaki T, Tanaka M, Shiota M, et al. Lipid synthesis is promoted by hypoxic adipocyte-derived exosomes in 3T3-L1 cells. Biochem Biophys Res Commun (2014) 445(2):327–33. doi: 10.1016/j.bbrc.2014.01.183
33. Castaño C, Kalko S, Novials A, Párrizas M. Obesity-associated exosomal miRNAs modulate glucose and lipid metabolism in mice. Proc Natl Acad Sci U.S.A. (2018) 115(48):12158–63. doi: 10.1073/pnas.1808855115
34. Jung YJ, Kim HK, Cho Y, Choi JS, Woo CH, Lee KS, et al. Cell reprogramming using extracellular vesicles from differentiating stem cells into white/beige adipocytes. Sci Adv (2020) 6(13):eaay6721. doi: 10.1126/sciadv.aay6721
35. Freeman DW, Noren Hooten N, Eitan E, Green J, Mode NA, Bodogai M, et al. Altered extracellular vesicle concentration, cargo, and function in diabetes. Diabetes (2018) 67(11):2377–88. doi: 10.2337/db17-1308
36. Dang SY, Leng Y, Wang ZX, Xiao X, Zhang X, Wen T, et al. Exosomal transfer of obesity adipose tissue for decreased miR-141-3p mediate insulin resistance of hepatocytes. Int J Biol Sci (2019) 15(2):351–68. doi: 10.7150/ijbs.28522
37. Deng ZB, Poliakov A, Hardy RW, Clements R, Liu C, Liu Y, et al. Adipose tissue exosome-like vesicles mediate activation of macrophage-induced insulin resistance. Diabetes (2009) 58(11):2498–505. doi: 10.2337/db09-0216
38. Liu T, Sun YC, Cheng P, Shao HG. Adipose tissue macrophage-derived exosomal miR-29a regulates obesity-associated insulin resistance. Biochem Biophys Res Commun (2019) 515(2):352–8. doi: 10.1016/j.bbrc.2019.05.113
39. Zhou Y, Gu P, Shi W, Li J, Hao Q, Cao X, et al. MicroRNA-29a induces insulin resistance by targeting PPARδ in skeletal muscle cells. Int J Mol Med (2016) 37(4):931–8. doi: 10.3892/ijmm.2016.2499
40. Guo Q, Furuta K, Lucien F, Gutierrez Sanchez LH, Hirsova P, Krishnan A, et al. Integrin β(1)-enriched extracellular vesicles mediate monocyte adhesion and promote liver inflammation in murine NASH. J Hepatol (2019) 71(6):1193–205. doi: 10.1016/j.jhep.2019.07.019
41. Hirsova P, Ibrahim SH, Krishnan A, Verma VK, Bronk SF, Werneburg NW, et al. Lipid-induced signaling causes release of inflammatory extracellular vesicles from hepatocytes. Gastroenterology (2016) 150(4):956–67. doi: 10.1053/j.gastro.2015.12.037
42. Zhao Z, Zhong L, Li P, He K, Qiu C, Zhao L, et al. Cholesterol impairs hepatocyte lysosomal function causing M1 polarization of macrophages via exosomal miR-122-5p. Exp Cell Res (2020) 387(1):111738. doi: 10.1016/j.yexcr.2019.111738
43. Jiang F, Chen Q, Wang W, Ling Y, Yan Y, Xia P. Hepatocyte-derived extracellular vesicles promote endothelial inflammation and atherogenesis via microRNA-1. J Hepatol (2020) 72(1):156–66. doi: 10.1016/j.jhep.2019.09.014
44. He Y, Rodrigues RM, Wang X, Seo W, Ma J, Hwang S, et al. Neutrophil-to-hepatocyte communication via LDLR-dependent miR-223-enriched extracellular vesicle transfer ameliorates nonalcoholic steatohepatitis. J Clin Invest (2021) 131(3):e141513. doi: 10.1172/JCI141513
45. Ibrahim SH, Hirsova P, Tomita K, Bronk SF, Werneburg NW, Harrison SA, et al. Mixed lineage kinase 3 mediates release of c-X-C motif ligand 10-bearing chemotactic extracellular vesicles from lipotoxic hepatocytes. Hepatology (2016) 63(3):731–44. doi: 10.1002/hep.28252
46. Sharifnia T, Antoun J, Verriere TGC, Suarez G, Wattacheril J, Wilson KT, et al. Hepatic TLR4 signaling in obese NAFLD. Am J Physiology-Gastrointestinal Liver Physiol (2015) 309(4):G270–8. doi: 10.1152/ajpgi.00304.2014
47. Fukunishi S, Sujishi T, Takeshita A, Ohama H, Tsuchimoto Y, Asai A, et al. Lipopolysaccharides accelerate hepatic steatosis in the development of nonalcoholic fatty liver disease in zucker rats. J Clin Biochem Nutr (2014) 54(1):39–44. doi: 10.3164/jcbn.13-49
48. Jin CJ, Engstler AJ, Ziegenhardt D, Bischoff SC, Trautwein C, Bergheim I. Loss of lipopolysaccharide-binding protein attenuates the development of diet-induced non-alcoholic fatty liver disease in mice. J Gastroenterol Hepatol (2017) 32(3):708–15. doi: 10.1111/jgh.13488
49. Wang R, Ding Q, Yaqoob U, de Assuncao TM, Verma VK, Hirsova P, et al. Exosome adherence and internalization by hepatic stellate cells triggers sphingosine 1-phosphate-dependent migration. J Biol Chem (2015) 290(52):30684–96. doi: 10.1074/jbc.M115.671735
50. Chen L, Chen R, Kemper S, Charrier A, Brigstock DR. Suppression of fibrogenic signaling in hepatic stellate cells by Twist1-dependent microRNA-214 expression: role of exosomes in horizontal transfer of Twist1. Am J Physiol Gastrointest Liver Physiol (2015) 309(6):G491–9. doi: 10.1152/ajpgi.00140.2015
51. Bruno S, Pasquino C, Herrera Sanchez MB, Tapparo M, Figliolini F, Grange C, et al. HLSC-derived extracellular vesicles attenuate liver fibrosis and inflammation in a murine model of non-alcoholic steatohepatitis. Mol Ther (2020) 28(2):479–89. doi: 10.1016/j.ymthe.2019.10.016
52. Povero D, Panera N, Eguchi A, Johnson CD, Papouchado BG, de Araujo Horcel L, et al. Lipid-induced hepatocyte-derived extracellular vesicles regulate hepatic stellate cell via microRNAs targeting PPAR-γ. Cell Mol Gastroenterol Hepatol (2015) 1(6):646–663.e4. doi: 10.1016/j.jcmgh.2015.07.007
53. Lemoinne S, Cadoret A, Rautou PE, El Mourabit H, Ratziu V, Corpechot C, et al. Portal myofibroblasts promote vascular remodeling underlying cirrhosis formation through the release of microparticles. Hepatology (2015) 61(3):1041–55. doi: 10.1002/hep.27318
54. Povero D, Eguchi A, Niesman IR, Andronikou N, de Mollerat du Jeu X, Mulya A, et al. Lipid-induced toxicity stimulates hepatocytes to release angiogenic microparticles that require vanin-1 for uptake by endothelial cells. Sci Signal (2013) 6(296):ra88. doi: 10.1126/scisignal.2004512
55. Eguchi A, Feldstein AE. Extracellular vesicles in non-alcoholic and alcoholic fatty liver diseases. Liver Res (2018) 2(1):30–4. doi: 10.1016/j.livres.2018.01.001
56. Szabo G, Momen-Heravi F. Extracellular vesicles in liver disease and potential as biomarkers and therapeutic targets. Nat Rev Gastroenterol Hepatol (2017) 14(8):455–66. doi: 10.1038/nrgastro.2017.71
57. Kim A, Shah AS, Nakamura T. Extracellular vesicles: a potential novel regulator of obesity and its associated complications. Children (Basel) (2018) 5(11):152. doi: 10.3390/children5110152
58. Geng Y, Faber KN, de Meijer VE, Blokzijl H, Moshage H. How does hepatic lipid accumulation lead to lipotoxicity in non-alcoholic fatty liver disease? Hepatol Int (2021) 15:21–35. doi: 10.1007/s12072-020-10121-2
59. Farrell GC, Haczeyni F, Chitturi S. Pathogenesis of NASH: how metabolic complications of overnutrition favour lipotoxicity and pro-inflammatory fatty liver disease. Adv Exp Med Biol (2018) 1061:19–44. doi: 10.1007/978-981-10-8684-7_3
60. Elfeky O, Longo S, Lai A, Rice GE, Salomon C. Influence of maternal BMI on the exosomal profile during gestation and their role on maternal systemic inflammation. Placenta (2017) 50:60–9. doi: 10.1016/j.placenta.2016.12.020
61. Stepanian A, Bourguignat L, Hennou S, Coupaye M, Hajage D, Salomon L, et al. Microparticle increase in severe obesity: not related to metabolic syndrome and unchanged after massive weight loss. Obes (Silver Spring) (2013) 21(11):2236–43. doi: 10.1002/oby.20365
62. Kranendonk ME, Visseren FL, van Herwaarden JA, Nolte-'t Hoen EN, de Jager W, Wauben MH, et al. Effect of extracellular vesicles of human adipose tissue on insulin signaling in liver and muscle cells. Obes (Silver Spring) (2014) 22(10):2216–23. doi: 10.1002/oby.20847
63. Thomou T, Mori MA, Dreyfuss JM, Konishi M, Sakaguchi M, Wolfrum C, et al. Adipose-derived circulating miRNAs regulate gene expression in other tissues. Nature (2017) 542(7642):450–5. doi: 10.1038/nature21365
64. Koenen MT, Brandt EF, Kaczor DM, Caspers T, Heinzmann ACA, Fischer P, et al. Extracellular vesicles from steatotic hepatocytes provoke pro-fibrotic responses in cultured stellate cells. Biomolecules (2022) 12(5):698. doi: 10.3390/biom12050698
65. Watt MJ, Miotto PM, De Nardo W, Montgomery MK. The liver as an endocrine organ-linking NAFLD and insulin resistance. Endocr Rev (2019) 40(5):1367–93. doi: 10.1210/er.2019-00034
66. Ying W, Riopel M, Bandyopadhyay G, Dong Y, Birmingham A, Seo JB, et al. Adipose tissue macrophage-derived exosomal miRNAs can modulate in vivo and in vitro insulin sensitivity. Cell (2017) 171(2):372–384.e12. doi: 10.1016/j.cell.2017.08.035
67. Fuchs A, Samovski D, Smith GI, Cifarelli V, Farabi SS, Yoshino J, et al. Associations among adipose tissue immunology, inflammation, exosomes and insulin sensitivity in people with obesity and nonalcoholic fatty liver disease. Gastroenterology (2021) 161(3):968–981.e12. doi: 10.1053/j.gastro.2021.05.008
68. Erridge C, Attina T, Spickett CM, Webb DJ. A high-fat meal induces low-grade endotoxemia: evidence of a novel mechanism of postprandial inflammation. Am J Clin Nutr (2007) 86(5):1286–92. doi: 10.1093/ajcn/86.5.1286
69. Choi Y, Kwon Y, Kim DK, Jeon J, Jang SC, Wang T, et al. Gut microbe-derived extracellular vesicles induce insulin resistance, thereby impairing glucose metabolism in skeletal muscle. Sci Rep (2015) 5:15878. doi: 10.1038/srep15878
70. Wang H, Mehal W, Nagy LE, Rotman Y. Immunological mechanisms and therapeutic targets of fatty liver diseases. Cell Mol Immunol (2021) 18(1):73–91. doi: 10.1038/s41423-020-00579-3
71. Cai J, Zhang XJ, Li H. Role of innate immune signaling in non-alcoholic fatty liver disease. Trends Endocrinol Metab (2018) 29(10):712–22. doi: 10.1016/j.tem.2018.08.003
72. Sutti S, Albano E. Adaptive immunity: an emerging player in the progression of NAFLD. Nat Rev Gastroenterol Hepatol (2020) 17(2):81–92. doi: 10.1038/s41575-019-0210-2
73. Kornek M, Lynch M, Mehta SH, Lai M, Exley M, Afdhal NH, et al. Circulating microparticles as disease-specific biomarkers of severity of inflammation in patients with hepatitis c or nonalcoholic steatohepatitis. Gastroenterology (2012) 143(2):448–58. doi: 10.1053/j.gastro.2012.04.031
74. Povero D, Feldstein AE. Novel molecular mechanisms in the development of non-alcoholic steatohepatitis. Diabetes Metab J (2016) 40(1):1–11. doi: 10.4093/dmj.2016.40.1.1
75. Hirsova P, Ibrahim SH, Verma VK, Morton LA, Shah VH, LaRusso NF, et al. Extracellular vesicles in liver pathobiology: small particles with big impact. Hepatology (2016) 64(6):2219–33. doi: 10.1002/hep.28814
76. Jiao B, An C, Du H, Tran M, Wang PA-O, Zhou DA-O, et al. STAT6 deficiency attenuates myeloid fibroblast activation and macrophage polarization in experimental folic acid nephropathy. Cells (2021) 10(11):3057. doi: 10.3390/cells10113057
77. An C, Jiao BA-OX, Du H, Tran M, Song B, Wang P, et al. Jumonji domain-containing protein-3 (JMJD3) promotes myeloid fibroblast activation and macrophage polarization in kidney fibrosis. Br J Pharmacol (2023), 1476–5381. doi: 10.1111/bph.16096
78. Shen M, Shen Y, Fan X, Men R, Ye T, Yang L. Roles of macrophages and exosomes in liver diseases. Front Med (Lausanne) (2020) 7:583691. doi: 10.3389/fmed.2020.583691
79. Wan Z, Yang X, Liu X, Sun Y, Yu P, Xu F, et al. M2 macrophage-derived exosomal microRNA-411-5p impedes the activation of hepatic stellate cells by targeting CAMSAP1 in NASH model. iScience (2022) 25(7):104597. doi: 10.1016/j.isci.2022.104597
80. Liu XL PQ, Cao HX, Xin FZ, Zhao ZH, Yang RX, Zeng J, et al. Lipotoxic hepatocyte-derived exosomal MicroRNA 192-5p activates macrophages through Rictor/Akt/Forkhead box transcription factor O1 signaling in nonalcoholic fatty liver disease. Hepatology (2020) 72(2):454–69. doi: 10.1002/hep.31050
81. Newman LA, Sorich MJ, Rowland A. Role of extracellular vesicles in the pathophysiology, diagnosis and tracking of non-alcoholic fatty liver disease. J Clin Med (2020) 9(7):2032. doi: 10.3390/jcm9072032
82. Ferreyra Solari NE, Inzaugarat ME, Baz P, De Matteo E, Lezama C, Galoppo M, et al. The role of innate cells is coupled to a Th1-polarized immune response in pediatric nonalcoholic steatohepatitis. J Clin Immunol (2012) 32(3):611–21. doi: 10.1007/s10875-011-9635-2
83. Yang Y, Han CY, Guan QB, Ruan SL. [Interleukin-17-mediated inflammation promotes nonalcoholic fatty liver disease in mice with regulation of M1-type macrophage polarization]. Zhonghua Gan Zang Bing Za Zhi (2018) 26(12):916–21. doi: 10.3760/cma.j.issn.1007-3418.2018.12.008
84. Wolf MJ, Adili A, Piotrowitz K, Abdullah Z, Boege Y, Stemmer K, et al. Metabolic activation of intrahepatic CD8+ T cells and NKT cells causes nonalcoholic steatohepatitis and liver cancer via cross-talk with hepatocytes. Cancer Cell (2014) 26(4):549–64. doi: 10.1016/j.ccell.2014.09.003
85. Sun G, Jin H, Zhang C, Meng H, Zhao X, Wei D, et al. OX40 regulates both innate and adaptive immunity and promotes nonalcoholic steatohepatitis. Cell Rep (2018) 25(13):3786–3799.e4. doi: 10.1016/j.celrep.2018.12.006
86. Tosello-Trampont A, Surette FA, Ewald SE, Hahn YS. Immunoregulatory role of NK cells in tissue inflammation and regeneration. Front Immunol (2017) 8:301. doi: 10.3389/fimmu.2017.00301
87. Zhang B, Yin Y, Lai RC, Lim SK. Immunotherapeutic potential of extracellular vesicles. Front Immunol (2014) 5:518. doi: 10.3389/fimmu.2014.00518
88. Li J, Liu H, Mauer AS, Lucien F, Raiter A, Bandla H, et al. Characterization of cellular sources and circulating levels of extracellular vesicles in a dietary murine model of nonalcoholic steatohepatitis. Hepatol Commun (2019) 3(9):1235–49. doi: 10.1002/hep4.1404
89. Ibrahim SH, Hirsova P, Gores GJ. Non-alcoholic steatohepatitis pathogenesis: sublethal hepatocyte injury as a driver of liver inflammation. Gut (2018) 67(5):963–72. doi: 10.1136/gutjnl-2017-315691
90. Liao CY, Song MJ, Gao Y, Mauer AS, Revzin A, Malhi H. Hepatocyte-derived lipotoxic extracellular vesicle sphingosine 1-phosphate induces macrophage chemotaxis. Front Immunol (2018) 9:2980. doi: 10.3389/fimmu.2018.02980
91. Sato K, Kennedy L, Liangpunsakul S, Kusumanchi P, Yang Z, Meng F, et al. Intercellular communication between hepatic cells in liver diseases. Int J Mol Sci (2019) 20(9):2180. doi: 10.3390/ijms20092180
92. Garcia-Martinez I, Santoro N, Chen Y, Hoque R, Ouyang X, Caprio S, et al. Hepatocyte mitochondrial DNA drives nonalcoholic steatohepatitis by activation of TLR9. J Clin Invest (2016) 126(3):859–64. doi: 10.1172/JCI83885
93. Ferrante SC, Nadler EP, Pillai DK, Hubal MJ, Wang Z, Wang JM, et al. Adipocyte-derived exosomal miRNAs: a novel mechanism for obesity-related disease. Pediatr Res (2015) 77(3):447–54. doi: 10.1038/pr.2014.202
94. Anand D, Chaudhuri A. Bacterial outer membrane vesicles: new insights and applications. Mol Membrane Biol (2016) 33(6-8):125–37. doi: 10.1080/09687688.2017.1400602
95. Ji Y, Yin Y, Li Z, Zhang W. Gut microbiota-derived components and metabolites in the progression of non-alcoholic fatty liver disease (NAFLD). Nutrients (2019) 11(8):1712. doi: 10.3390/nu11081712
96. Villard A, Boursier J, Andriantsitohaina R. Bacterial and eukaryotic extracellular vesicles and non-alcoholic fatty liver disease: new players in the gut-liver axis? Am J Physiol Gastrointest Liver Physiol (2021) 320:G485–95. doi: 10.1152/ajpgi.00362.2020
97. Gu L, Meng R, Tang Y, Zhao K, Liang F, Zhang R, et al. Toll-like receptor 4 signaling licenses the cytosolic transport of lipopolysaccharide from bacterial outer membrane vesicles. Shock (2019) 51(2):256–65. doi: 10.1097/SHK.0000000000001129
98. Pang J, Xu W, Zhang X, Wong GL, Chan AW, Chan HY, et al. Significant positive association of endotoxemia with histological severity in 237 patients with non-alcoholic fatty liver disease. Aliment Pharmacol Ther (2017) 46(2):175–82. doi: 10.1111/apt.14119
99. Koeppen K, Hampton TH, Jarek M, Scharfe M, Gerber SA, Mielcarz DW, et al. A novel mechanism of host-pathogen interaction through sRNA in bacterial outer membrane vesicles. PloS Pathog (2016) 12(6):e1005672. doi: 10.1371/journal.ppat.1005672
100. Davani-Davari D, Negahdaripour M, Karimzadeh I, Seifan M, Mohkam M, Masoumi SJ, et al. Prebiotics: definition, types, sources, mechanisms, and clinical applications. Foods (2019) 8(3):92. doi: 10.3390/foods8030092
101. Nabavi S, Rafraf M, Somi MH, Homayouni-Rad A, Asghari-Jafarabadi M. Effects of probiotic yogurt consumption on metabolic factors in individuals with nonalcoholic fatty liver disease. J Dairy Sci (2014) 97(12):7386–93. doi: 10.3168/jds.2014-8500
102. Malaguarnera M, Vacante M, Antic T, Giordano M, Chisari G, Acquaviva R, et al. Bifidobacterium longum with fructo-oligosaccharides in patients with non alcoholic steatohepatitis. Dig Dis Sci (2012) 57(2):545–53. doi: 10.1007/s10620-011-1887-4
103. Hernández A, Reyes D, Geng Y, Arab JP, Cabrera D, Sepulveda R, et al. Extracellular vesicles derived from fat-laden hepatocytes undergoing chemical hypoxia promote a pro-fibrotic phenotype in hepatic stellate cells. Biochim Biophys Acta Mol Basis Dis (2020) 1866(10):165857. doi: 10.1016/j.bbadis.2020.165857
104. Lee YS, Kim SY, Ko E, Lee JH, Yi HS, Yoo YJ, et al. Exosomes derived from palmitic acid-treated hepatocytes induce fibrotic activation of hepatic stellate cells. Sci Rep (2017) 7(1):3710. doi: 10.1038/s41598-017-03389-2
105. Higashi T, Friedman SL, Hoshida Y. Hepatic stellate cells as key target in liver fibrosis. Adv Drug Delivery Rev (2017) 121:27–42. doi: 10.1016/j.addr.2017.05.007
106. An C, Jiao B, Du H, Tran M, Zhou D, Wang Y. Myeloid PTEN deficiency aggravates renal inflammation and fibrosis in angiotensin II-induced hypertension. J Cell Physiol (2022) 237(1):983–91. doi: 10.1002/jcp.30574
107. Nguyen Huu T, Park J, Zhang Y, Duong Thanh H, Park I, Choi JM, et al. The role of oxidative inactivation of phosphatase PTEN and TCPTP in fatty liver disease. Antioxidants (Basel) (2023) 12(1):120. doi: 10.3390/antiox12010120
108. Luo X, Xu ZX, Wu JC, Luo SZ, Xu MY. Hepatocyte-derived exosomal miR-27a activateshepatic stellate cells through the inhibitionof PINK1-mediated mitophagy in MAFLD. Mol Ther Nucleic Acids (2021) 26:1241–54. doi: 10.1016/j.omtn.2021.10.022
109. Ma L, Wei J, Zeng Y, Liu J, Xiao E, Kang Y, et al. Mesenchymal stem cell-originated exosomal circDIDO1 suppresses hepatic stellate cell activation by miR-141-3p/PTEN/AKT pathway in human liver fibrosis. Drug Delivery (2022) 29(1):440–53. doi: 10.1080/10717544.2022.2030428
110. Luo X, Luo SZ, Xu ZX, Zhou C, Li ZH, Zhou XY, et al. Lipotoxic hepatocyte-derived exosomal miR-1297 promotes hepatic stellate cell activation through the PTEN signaling pathway in metabolic-associated fatty liver disease. World J Gastroenterol (2021) 27(14):1419–34. doi: 10.3748/wjg.v27.i14.1419
111. Kalluri R, LeBleu VS. The biology, function, and biomedical applications of exosomes. Science (2020) 367(6478):eaau6977. doi: 10.1126/science.aau6977
112. Jiang WY, Xun YH. [Value of detection of extracellular vesicles in the diagnosis of nonalcoholic fatty liver disease]. Zhonghua Gan Zang Bing Za Zhi (2020) 28(1):92–6. doi: 10.3760/cma.j.issn.1007-3418.2020.01.022
113. Shabangu CS, Huang JF, Hsiao HH, Yu ML, Chuang WL, Wang SC, et al. Liquid biopsy for the diagnosis of viral hepatitis, fatty liver steatosis, and alcoholic liver diseases. Int J Mol Sci (2020) 21(10):3732. doi: 10.3390/ijms21103732
114. Povero D, Yamashita H, Ren W, Subramanian MG, Myers RP, Eguchi A, et al. Characterization and proteome of circulating extracellular vesicles as potential biomarkers for NASH. Hepatol Commun (2020) 4(9):1263–78. doi: 10.1002/hep4.1556
115. Jiang H, Qian Y, Shen Z, Liu Y, He Y, Gao R, et al. Circulating microRNA−135a−3p in serum extracellular vesicles as a potential biological marker of non−alcoholic fatty liver disease. Mol Med Rep (2021) 24(1):498. doi: 10.3892/mmr.2021.12137
116. Povero D, Eguchi A, Li H, Johnson CD, Papouchado BG, Wree A, et al. Circulating extracellular vesicles with specific proteome and liver microRNAs are potential biomarkers for liver injury in experimental fatty liver disease. PloS One (2014) 9(12):e113651. doi: 10.1371/journal.pone.0113651
117. Tryndyak VP, Latendresse JR, Montgomery B, Ross SA, Beland FA, Rusyn I, et al. Plasma microRNAs are sensitive indicators of inter-strain differences in the severity of liver injury induced in mice by a choline- and folate-deficient diet. Toxicol Appl Pharmacol (2012) 262(1):52–9. doi: 10.1016/j.taap.2012.04.018
118. Newman LA, Useckaite Z, Johnson J, Sorich MJ, Hopkins AM, Rowland A, et al. Selective isolation of liver-derived extracellular vesicles redefines performance of miRNA biomarkers for non-alcoholic fatty liver disease. Biomedicines (2022) 10(1):195. doi: 10.3390/biomedicines10010195
119. Ma YS, Yang XL, Xin R, Liu JB, Fu D. Power and promise of exosomes as clinical biomarkers and therapeutic vectors for liquid biopsy and cancer control. Biochim Biophys Acta Rev Cancer (2021) 1875(1):188497. doi: 10.1016/j.bbcan.2020.188497
120. Li W, Li C, Zhou T, Liu X, Liu X, Li X, et al. Role of exosomal proteins in cancer diagnosis. Mol Cancer (2017) 16(1):145. doi: 10.1186/s12943-017-0706-8
121. Wu P, Zhang B, Ocansey DKW, Xu W, Qian H. Extracellular vesicles: a bright star of nanomedicine. Biomaterials (2021) 269:120467. doi: 10.1016/j.biomaterials.2020.120467
122. He M, Zeng Y. Microfluidic exosome analysis toward liquid biopsy for cancer. J Lab Autom (2016) 21(4):599–608. doi: 10.1177/2211068216651035
123. Conde-Vancells J, Rodriguez-Suarez E, Gonzalez E, Berisa A, Gil D, Embade N, et al. Candidate biomarkers in exosome-like vesicles purified from rat and mouse urine samples. Proteomics – Clin Appl (2010) 4(4):416–25. doi: 10.1002/prca.200900103
124. Santiago-Dieppa DR, Steinberg J, Gonda D, Cheung VJ, Carter BS, Chen CC. Extracellular vesicles as a platform for 'liquid biopsy' in glioblastoma patients. Expert Rev Mol Diagn (2014) 14(7):819–25. doi: 10.1586/14737159.2014.943193
125. Brandao BB, Lino M, Kahn CR. Extracellular miRNAs as mediators of obesity-associated disease. J Physiol (2022) 600(5):1155–69. doi: 10.1113/JP280910
126. Han H, Wang L, Du H, Jiang J, Hu C, Zhang G, et al. Expedited biliopancreatic juice flow to the distal gut benefits the diabetes control after duodenal-jejunal bypass. Obes Surg (2015) 25(10):1802–9. doi: 10.1007/s11695-015-1633-7
127. Bae YU, Kim Y, Lee H, Kim H, Jeon JS, Noh H, et al. Bariatric surgery alters microRNA content of circulating exosomes in patients with obesity. Obes (Silver Spring) (2019) 27(2):264–71. doi: 10.1002/oby.22379
128. Hubal MJ, Nadler EP, Ferrante SC, Barberio MD, Suh JH, Wang J, et al. Circulating adipocyte-derived exosomal MicroRNAs associated with decreased insulin resistance after gastric bypass. Obes (Silver Spring) (2017) 25(1):102–10. doi: 10.1002/oby.21709
129. Nakao Y, Amrollahi P, Parthasarathy G, Mauer AS, Sehrawat TS, Vanderboom P, et al. Circulating extracellular vesicles are a biomarker for NAFLD resolution and response to weight loss surgery. Nanomedicine (2021) 36:102430. doi: 10.1016/j.nano.2021.102430
130. Marbán E. The secret life of exosomes: what bees can teach us about next-generation therapeutics. J Am Coll Cardiol (2018) 71(2):193–200. doi: 10.1016/j.jacc.2017.11.013
131. Alice G, Silvia P, Cristiano C, Francesca R, Marzia B. Biophotonics for diagnostic detection of extracellular vesicles. Adv Drug Delivery Rev (2021) 174:229–49. doi: 10.1016/j.addr.2021.04.014
132. Raghav A, Khan ZA, Upadhayay VK, Tripathi P, Gautam KA, Mishra BK, et al. Mesenchymal stem cell-derived exosomes exhibit promising potential for treating SARS-CoV-2-Infected patients. Cells (2021) 10(3):587. doi: 10.3390/cells10030587
133. Gasecka A, Nieuwland R, van der Pol E, Hajji N, Ćwiek A, Pluta K, et al. P2Y12 antagonist ticagrelor inhibits the release of procoagulant extracellular vesicles from activated platelets. Cardiol J (2019) 26(6):782–9. doi: 10.5603/CJ.a2018.0045
134. Barile L, Vassalli G. Exosomes: therapy delivery tools and biomarkers of diseases. Pharmacol Ther (2017) 174:63–78. doi: 10.1016/j.pharmthera.2017.02.020
135. Watanabe T, Tsuchiya A, Takeuchi S, Nojiri S, Yoshida T, Ogawa M, et al. Development of a non-alcoholic steatohepatitis model with rapid accumulation of fibrosis, and its treatment using mesenchymal stem cells and their small extracellular vesicles. Regener Ther (2020) 14:252–61. doi: 10.1016/j.reth.2020.03.012
136. Luo X, Li H, Ma L, Zhou J, Guo X, Woo SL, et al. Expression of STING is increased in liver tissues from patients with NAFLD and promotes macrophage-mediated hepatic inflammation and fibrosis in mice. Gastroenterology (2018) 155(6):1971–1984.e4. doi: 10.1053/j.gastro.2018.09.010
137. Chen L, Brenner DA, Kisseleva T. Combatting fibrosis: exosome-based therapies in the regression of liver fibrosis. Hepatol Commun (2019) 3(2):180–92. doi: 10.1002/hep4.1290
138. Yang B, Chen Y, Shi J. Exosome biochemistry and advanced nanotechnology for next-generation theranostic platforms. Adv Mater (2019) 31(2):e1802896. doi: 10.1002/adma.201802896
139. Ding J, Wang J, Chen J. Exosomes as therapeutic vehicles in liver diseases. Ann Transl Med (2021) 9(8):735. doi: 10.21037/atm-20-5422
140. Gurunathan S, Kang MH, Kim JH. A comprehensive review on factors influences biogenesis, functions, therapeutic and clinical implications of exosomes. Int J Nanomedicine (2021) 16:1281–312. doi: 10.2147/IJN.S291956
141. Zhang X, Liu L, Tang M, Li H, Guo X, Yang X. The effects of umbilical cord-derived macrophage exosomes loaded with cisplatin on the growth and drug resistance of ovarian cancer cells. Drug Dev Ind Pharm (2020) 46(7):1150–62. doi: 10.1080/03639045.2020.1776320
142. Zhang Z, Dombroski JA, King MR. Engineering of exosomes to target cancer metastasis. Cell Mol Bioeng (2020) 13(1):1–16. doi: 10.1007/s12195-019-00607-x
143. Toffoli G, Hadla M, Corona G, Caligiuri I, Palazzolo S, Semeraro S, et al. Exosomal doxorubicin reduces the cardiac toxicity of doxorubicin. Nanomedicine (Lond) (2015) 10(19):2963–71. doi: 10.2217/nnm.15.118
144. Li SP, Lin ZX, Jiang XY, Yu XY. Exosomal cargo-loading and synthetic exosome-mimics as potential therapeutic tools. Acta Pharmacol Sin (2018) 39(4):542–51. doi: 10.1038/aps.2017.178
Keywords: extracellular vesicles (EV), NAFLD, diagnosis, treatment, mechanisms
Citation: Jiang W, Xu Y, Chen J-C, Lee Y-H, Hu Y, Liu C-H, Chen E, Tang H, Zhang H and Wu D (2023) Role of extracellular vesicles in nonalcoholic fatty liver disease. Front. Endocrinol. 14:1196831. doi: 10.3389/fendo.2023.1196831
Received: 30 March 2023; Accepted: 21 June 2023;
Published: 18 July 2023.
Edited by:
Isabel Rodriguez Amado, International Iberian Nanotechnology Laboratory (INL), PortugalReviewed by:
Hao Du, UCONN Health, United StatesBaihai Jiao, University of Connecticut Health Center, United States
Copyright © 2023 Jiang, Xu, Chen, Lee, Hu, Liu, Chen, Tang, Zhang and Wu. This is an open-access article distributed under the terms of the Creative Commons Attribution License (CC BY). The use, distribution or reproduction in other forums is permitted, provided the original author(s) and the copyright owner(s) are credited and that the original publication in this journal is cited, in accordance with accepted academic practice. No use, distribution or reproduction is permitted which does not comply with these terms.
*Correspondence: Hua Zhang, aHVhemgwMTA4QDEyNi5jb20=; Dongbo Wu, ZG9uZ2JvaHVheGlAc2N1LmVkdS5jbg==
†These authors have contributed equally to this work