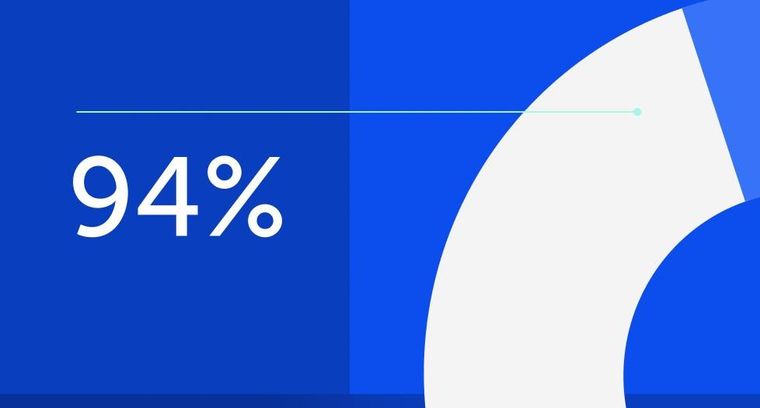
94% of researchers rate our articles as excellent or good
Learn more about the work of our research integrity team to safeguard the quality of each article we publish.
Find out more
REVIEW article
Front. Endocrinol., 30 June 2023
Sec. Gut Endocrinology
Volume 14 - 2023 | https://doi.org/10.3389/fendo.2023.1192216
This article is part of the Research TopicEmerging Roles of the Gut Microbiota in the Pathogenesis of Metabolic Disorders - Volume IIView all 8 articles
Thyroid disorders are clinically characterized by alterations of L-3,5,3’,5’-tetraiodothyronine (T4), L-3,5,3’-triiodothyronine (T3), and/or thyroid-stimulating hormone (TSH) levels in the blood. The most frequent thyroid disorders are hypothyroidism, hyperthyroidism, and hypothyroxinemia. These conditions affect cell differentiation, function, and metabolism. It has been reported that 40% of the world’s population suffers from some type of thyroid disorder and that several factors increase susceptibility to these diseases. Among them are iodine intake, environmental contamination, smoking, certain drugs, and genetic factors. Recently, the intestinal microbiota, composed of more than trillions of microbes, has emerged as a critical player in human health, and dysbiosis has been linked to thyroid diseases. The intestinal microbiota can affect host physiology by producing metabolites derived from dietary fiber, such as short-chain fatty acids (SCFAs). SCFAs have local actions in the intestine and can affect the central nervous system and immune system. Modulation of SCFAs-producing bacteria has also been connected to metabolic diseases, such as obesity and diabetes. In this review, we discuss how alterations in the production of SCFAs due to dysbiosis in patients could be related to thyroid disorders. The studies reviewed here may be of significant interest to endocrinology researchers and medical practitioners.
Thyroid dysfunction is a worldwide health problem affecting an average of 20 million Americans (1, 2). The most common conditions related to thyroid dysfunction are hypothyroidism (overt and subclinical) and hyperthyroidism (overt and subclinical) (3, 4). These conditions are often associated with the development of other pathologies (5) such as depression (6) and chronic metabolic conditions like type 1 diabetes and type 2 diabetes mellitus (4, 7). Importantly, thyroid disorders are common during pregnancy (8). The prevalence of hypothyroidism during pregnancy varies between 2.5% and 11% (9). The main cause of thyroid disorders is iodine malnutrition (iodine deficiency or excess). However, genetics, smoking, alcohol consumption, infections, drugs, and gender are also important factors influencing thyroid disease development (1, 10). Maintaining a healthy lifestyle, manage thyroid and metabolic diseases, and having a balanced gut microbiome depend on appropriate nutrition (11). In this review, we discuss how having a healthy gut microbiota will facilitate the availability of essential minerals for the thyroid gland, such as iodine (12). Microbial fermentation transforms the dietary fiber into short-chain fatty acids (SCFAs) (13–15), which have beneficial effects on the host (16, 17). SCFAs enhance ATP synthesis affecting cell metabolism and supplying the gut epithelium with energy (18), and controlling the immune system (19), for example, by generating a more tolerogenic environment (20–22). Dysbiosis, or changes in the composition of the intestinal microbiota, can have an impact on the metabolism and the production of SCFAs, which can influence disease progression (23), among them are thyroid diseases. This review also provides new perspectives on SCFAs detection for improving the quality of patients’ lives.
Thyroid hormones (THs) L-3,5,3’,5’-tetraiodothyronine (T4) and L-3,5,3’-triiodothyronine (T3) are biological molecules that contain iodine in their structure and play crucial roles in mammal physiology like growth, neuronal development, reproduction, and cell energy metabolism (24). THs are synthesized and secreted by the thyroid gland, an endocrine gland located in the front of the neck (24, 25). Thyroid cells, called thyrocytes, are arranged into spherical structures forming the thyroid follicles, which are functional units of the thyroid gland. Each follicle surrounds a colloid mainly composed of a glycoprotein named thyroglobulin (Tg), which is the precursor of THs (24, 25). For THs synthesis, thyrocytes actively uptake inorganic iodide (I-) from circulation by its active transport through the Na+/I- symporter (NIS), which is located at the basolateral side of the follicles (26). Then, I- diffuses through the cytosol towards the apical membrane, and it passively crosses to the apical side of the plasma membrane through several molecules, including Pendrin and the human apical iodide transporter (IT) (27, 28). On the apical side of the plasma membrane are located the nicotinamide adenine dinucleotide phosphate (NADPH) oxidase (NOX) family of oxidoreductase enzymes, dual oxidase 1 (DUOX1) and dual oxidase 2 (DUOX2) (29–31). The primary purpose of these enzymes is to protect thyrocytes from oxidative stress as they generate hydrogen peroxide (H2O2) (30, 32). This action is not trivial because once I- has been transported into the colloid, the thyroperoxidase (TPO) enzyme (in the presence of H2O2) catalyzes its oxidation (generation of I2) and its subsequent covalent incorporation into a tyrosyl residue at Tg (32, 33). The products of this reaction are 3-iodotyrosine or monoiodothyronine (MIT) and 3,5-diiodotyrosine or diiodotyrosine (DIT). The TPO enzyme can combine two DIT residues to form T4 and one DIT residue with one MIT residue to form T3, both reactions occur in the presence of H2O2 as oxidizing agent (32). When the thyroid gland is stimulated by TSH, the Tg is endocytosed by the thyrocytes into endosomes, which fuse with lysosomes causing the release of lysosomal enzymes that catalyze the hydrolysis of Tg residues into T4 and T3 and later will be released T4 and T3 into the bloodstream, where the majority of the THs produced in a ratio around 10:1 (T4/T3), are transported coupled to proteins such as thyroxine-binding globulin (TBG), transthyretin (TTR), and human serum albumin (HSA) (30, 33–36) (Figure 1).
Figure 1 Thyroid hormone synthesis. The thyroid gland is composed of thyroid follicles which are constituted by thyrocytes, surrounding the colloid that contains thyroglobulin (Tg) the precursor of THs. Thyrocytes uptake the inorganic iodide (I-) through the Na+/I- symporter (NIS), located at the basolateral membrane, diffuse and cross towards the apical membrane by the anion transporter Pendrin and the human apical iodine transporter (IT). In the apical membrane, are located the dual oxidase 1 and 2 (DUOX) enzymes which are NADPH oxidases generating hydrogen peroxide (H2O2). Then in the colloid the thyroperoxidase (TPO) catalyzes I- oxidation and its covalent incorporation into a tyrosyl residue at the Tg, producing monoiodotyrosine (MIT) and 3,5-diiodotyrosine (DIT) all in the presence of H2O2. TPO also combines two DIT residues to form T4 and one DIT residue with one MIT residue to form T3 (coupling reaction). Finally, THs are endocytosed by the thyrocytes into endosomes, hydrolyzed and later released as T4 and T3 into the bloodstream. Created with Biorender.com.
T4 comprises the majority of the THs produced and is considered the reserve hormone, whereas T3 is the main active biological TH (24, 37–39). THs’ production and release into the circulation are tightly regulated by the Hypothalamus-Pituitary-Thyroid (HPT) axis. Here, neurons at the hypothalamic paraventricular nucleus produce and secrete the thyrotropin-releasing hormone (TRH), which prompts the pituitary to release thyroid-stimulating hormone (TSH) (40). TSH promotes the release of T4 and T3 into the bloodstream by binding to its receptors located at the thyroid gland, stimulating the THs secretion and NIS, Tg, and TPO gene expression (40, 41) (Figure 2). An increase of THs in the bloodstream triggers negative feedback in the HPT axis, decreasing the production of TSH (42). T4 is converted into T3 that binds its nuclear receptor to down regulate the transcription of TSH mRNA (24, 38, 43). The conversion of T4 into T3 is mediated by deiodinases 1, 2 and 3 (D1, D2 and D3 respectively), which are selenoproteins with iodothyronine deiodinase capacity (44). In general, D1 and D2 convert T4 into T3 and reverse T3 (rT3; 3,3′,5′-triiodothyronine) into T2 (3,3′-diiodothyronine). D3 inactivates T4 by converting it into rT3 or T2 (44). Even though THs are lipophilic molecules, they are uptaken from the bloodstream by passive transport (37, 45). They can also, entry into cells by several transporters, such as monocarboxylate transporters (MCT), particularly MCT 1, 4, 8, and 10, and sodium-coupled monocarboxylate transporter 1 (SMCT1) (44). Additionally, organic anion transporters (OATP1A2 and OATP1C1) and L-type transporters 1 and 2 (LAT1/2) have also been identified as active transporters of THs (37, 45). THs can exert a genomic action via intracellular receptors for T3 (TRs), which are nuclear proteins known as TRα and TRβ, that act as transcription factors modulating gene expression (46, 47). TRs recognize specific nucleotide sequences of DNA called thyroid hormone response elements (TREs) (46, 47). These receptors are known to form complex structures with other proteins, such as the retinoic acid receptor (RXR) (46, 47). In addition to their effects on gene regulation, THs exert non-genomic mechanisms of action by binding to the transmembrane protein αVβ3 integrin, which interacts with extracellular matrix proteins (48), initiating an intracellular signaling cascade through the phospholipase C (PLC) pathway and the Cα protein, which activates MAPK proteins regulated by extracellular signals (48). Once activated, MAPK is translocated to the nucleus and phosphorylates the TRβa receptor, thereby modulating gene expression (49).
Figure 2 Regulation of thyroid hormone secretion and synthesis by the hypothalamus, pituitary, and thyroid gland axis (HPT axis). The hypothalamus produces and secretes thyrotropin-releasing hormone (TRH), which induces the release thyroid-stimulating hormone (TSH) by the pituitary gland. TSH stimulates the synthesis and production of T3 and T4 in the bloodstream. An increase of T3 and T4 serum levels activates a negative feedback loop over the hypothalamus and pituitary gland decreasing the production of TRH and TSH, thereby reducing the plasmatic levels of thyroid hormones (THs) (40, 42).
Thyroid disorders are conditions in which the function of the thyroid gland is impaired, and they are clinically characterized by alterations in blood THs levels (1). Hypothyroidism and hyperthyroidism are common health problems whose prevalence has been reported in 110 nations worldwide (1). In Europe, 4.94% of hypothyroidism and 1.72% of hyperthyroidism remain undiagnosed (3). A Brazilian longitudinal study showed an incidence of 1.98% for overt hypothyroidism, 0.19% for overt hyperthyroidism, 3.99% for subclinical hypothyroidism, and 0.54% for subclinical hyperthyroidism, showing a higher incidence of hypothyroidism in the country even when is a country with adequate iodine intake (10).
Hyperthyroidism, clinically diagnosed by high T3 and T4 and low TSH blood levels (50), is characterized by heat sensitivity, weight loss, changes in appetite, irritability, and poor fertility (50). Among the risk factors for developing hyperthyroidism are nutritional selenium and iodine status, family history of thyroid conditions like Grave’s disease (GD), and other chronic illnesses, like pernicious anemia, primary adrenal insufficiency, and recent pregnancy, which increase the predisposition to thyroiditis (51). In America and Europe, the prevalence of overt hyperthyroidism ranges from 0.5% to 1% (3, 52). Although several therapies are available, the most effective is the use of antithyroid drugs that interfere with THs synthesis, radioiodine therapy, and surgery to eliminate a portion of the gland; however, this treatment may result in permanent hypothyroidism (53, 54). Moreover, subclinical hyperthyroidism is characterized by THs normal levels with below-normal TSH values, causing important clinical consequences like cardiovascular disease and bone loss (55).
Hypothyroidism is diagnosed by low blood levels of T3 and T4, with high blood TSH levels (56). Typical symptoms include fatigue, cold sensitivity, constipation, weight gain, and even more severe consequences, such as hyperlipidemia (50), most of which are consequence of a reduced metabolic rate (50, 57). One of the main causes of hypothyroidism is insufficient iodine consumption. Hashimoto’s thyroiditis (HT) is the most prevalent autoimmune thyroid condition characterized by the development of anti-thyroid antibodies, is also described as a frequent cause of hypothyroidism (58, 59). Interestingly, iodine-rich foods can serve as stressors for the thyroid gland by increasing iodine levels inside the gland and causing the production of reactive oxygen species (ROS). This results in the development of autoantibodies directed against the gland, such as TPO antibodies (TPO-Ab+), which is the most common trait used for the diagnosis of HT (58, 59). Globally, HT primarily affects men (approximately a 4-fold increase). However, there are variations across areas with varied economic status, which are more common in low and middle-income regions, particularly in Africa (58, 59). HT is the most common cause of subclinical hypothyroidism (60), which is characterized by normal THs levels with elevated TSH levels (4, 61). Subclinical hypothyroidism is a mild condition that could go unnoticed by the patient (60).
Hypothyroxinemia (HTX) is a frequent worldwide thyroid condition that is clinically relevant during pregnancy due to the essential role of maternal T4 for fetal development (62). HTX is clinically defined as low levels of blood T4 with normal T3 and TSH levels (62). Gestational HTX is 200 times more frequent than congenital hypothyroidism (63, 64). It has been reported that early in pregnancy, the maternal thyroid gland requires more iodine to supply mother and fetal THs (8). This THs demand can be a stress factor for the maternal thyroid gland, and the mother will compensate by reducing the level of T4 to maintain normal T3 levels. If this condition persists, the pregnant woman can develop hypothyroidism (8, 62, 65). Factors associated with HTX incidence are iodine deficiency, stress, smoking, and particulate matter (PM2.5) exposure in the air (66, 67).
Gestational HTX has been linked to harmful effects on fetal development (68) due to the pivotal role of maternal T4 on fetal neurodevelopment (8, 62, 65). The neurodevelopmental disorders in the offspring associated with these conditions are schizophrenia, autism, bipolar disorder, and attention-deficit/hyperactivity disorder (ADHD), as well as impairments like low intelligence quotient (IQ), neurocognitive disabilities, and auditory impairments (68–74). In humans, the consequences of gestational HTX are concentrated in the CNS; however, in animal models, the consequences surpass the CNS, reaching the immune response (75, 76). The offspring gestated in HTX (HTX-offspring) have an increased predisposition to develop more severe and premature autoimmune disease in comparison to the euthyroid-gestated offspring (75). Thus, thyroid disorders are a major global health problem, and they are particularly important in pregnancy and childhood for their impact on CNS development (77). The prevalence of numerous types of thyroid disorders calls for the development of novel and less invasive treatments and additional support for diagnosis. Appropriate iodine nutrition is essential for thyroid health, and regardless of cultures, ethnicities, and/or socioeconomic status, people can be affected by iodine deficiency or excess (77, 78). However, it has been difficult to make a comparison between different countries and draw conclusions about several aspects of thyroid diseases due to differences in diagnostic thresholds, assay sensitivities, population selection, and variations in iodine intake (1).
Many essential nutrients must be consumed in the diet; for example, iodine is an essential element for the function of all mammals’ thyroid glands and as is part of the structure of THs (79). Therefore, iodine nutrition determines thyroid function worldwide (1). Deficiency or excess of iodine in the diet can have severe consequences for fetal development, impairing central nervous system (CNS) function and body growth (80). In addition, in both infants and adults, the lack of iodine can strongly affect metabolic processes, generating hypothyroidism and goiter, whereas iodine excess can trigger thyroiditis, hyperthyroidism, and hypothyroidism (81). Consumption of foods containing iodine is the only way to obtain this micronutrient, which is present in foods such as seaweed, marine fish, and seafood (82, 83). However, historically, iodine deficiency has been supplied in many countries through the fortification of foods with massive consumption, such as table salt (78). Although this fortification succeeded in restoring normal levels of iodine intake in several countries that adhered to iodination policies. The western diet includes a low dietary fiber intake along with a high amount of ultra-processed foods containing high amounts of refined sugars, saturated fats, trans fats, salt, and alcohol (78). These components of processed foods are harmful to health and contribute to the development of diseases such as obesity, diabetes (84), metabolic syndrome (85), cardiovascular disease (86), dysbiosis (87), cancer (88), and other inflammatory diseases like inflammatory bowel disease (IBD) and asthma (89). Regarding iodine status in people who follow a western diet, some studies have indicated that normal iodine intake values decrease in this type of diet by not including key foods such as seaweed or fish, which also affects other essential micronutrients such as vitamins, selenium, iron, among others (90). On the other hand, some studies indicate that iodine intake does not decrease because of the high amounts of iodized salt used in fast and ultra-processed food preparations, but it could even deliver an excess intake of iodine, which is harmful when combined with excess fat (91). In either case, the amount of iodine ingested is not adequate, which is detrimental, especially since it is not known whether the current supplementation of table salt is beneficial. In addition, this intake is coupled with low-quality nutrients and elements (92), which not only harm the role of iodine in the body but can also affect the proper functioning of other organs besides the thyroid and cause diseases such as those previously mentioned. Therefore, more studies are required in this field because this type of diet could have more serious consequences than those currently known (93).
Diet can modulate the maturation of the microbiota (94, 95) by consuming whole grains, fruits, and vegetables that provide antioxidants and fiber (96). Dietary fiber is one of the most important elements for the nutrition of microorganisms present in the intestinal microbiota, as well as for the fermentation of these compounds, by producing beneficial metabolites such as, vitamins, precursors of neurotransmitters, among others (18, 97). The metabolites produced by the intestinal microbiota can be classified as: 1) metabolites derived directly from the metabolism of dietary compounds; 2) metabolites synthesized by the host and transformed from the gut microbiota; 3) metabolites produced de novo by the gut microbiota (98). The metabolites modulated by dietary compounds are bile acids, branched-chain amino acids, trimethylamine, tryptophan, indole metabolites and SCFAs (98). Low fiber intake might affect intestinal barrier integrity and immunity (99). Fiber is an essential nutrient (11), described as a carbohydrate polymer with ten or more monomeric units that digestive enzymes cannot hydrolyze and could have a prebiotic effect (100). Dietary fibers and their different structures can elicit different microbial responses and the production of metabolites, such as SCFAs, which are one of the most studied (101, 102). SCFAs are carbohydrates with 1-6 carbon atoms. These are the final products of microbial carbohydrate fermentation by bacteria with polysaccharide-degrading enzymes, producing 90-95% of the most abundant SCFAs subtypes in the colon, including acetate (C2), propionate (C3), and butyrate (C4) (103, 104). These metabolites participate in various cellular processes and are important for maintaining intestinal and immune homeostasis (105).
Mothers’ health and the microbiota inherited by their offspring through gestational time, birth method, and breastfeeding influence their later adult health status (106–108). Besides, the human gut microbiota is constantly changing and maturing with its host (109). Environmental factors such as antibiotics, dietary supplements, probiotics, hygiene, and contact with pets in early life influence the offspring’s microbiota, disrupting microbial homeostasis, and increasing the risk of developing several diseases (110, 111). Subsequently, the composition, quantity, and activity of these microbial communities along the entire digestive tract can vary depending on circadian networks related to intestinal IgA (112), diet, host age, or preferential localization in the intestinal tract due to changes in pH and oxygen levels (113–115). The microbiota varies along the digestive tract, and the oral cavity is remarkably diverse and abundant (116). In mammals, the stomach harbors the lowest concentration of bacteria per gram of content (101 cells/gram), followed by an increase in concentration from the duodenum to the ileum (103 to 107 cells/gram) and a substantial concentration in the colon (1012 cells/gram) (117, 118). A balanced microbiota helps in the proper development of physiological functions such as nutrient transport in the small intestine (119). Due to its anatomical location, the small intestinal microbiota has been difficult to research, despite being essential for the synthesis and assimilation of essential micronutrients, including vitamin K and B12, bile acid physiology, and digestion of carbohydrates and fats (120, 121). Most of the microbiota present in the small intestine resembles the oral cavity microbiota, with a low abundance of strict anaerobes and the most enriched bacterial species, including Veionella, Rothia, Streptococcus, Actinomyces, Clostridium, and Lactobacillus species (122, 123). The colon is inhabited by trillions of microbes that include at least 1000 distinct species that contribute enzymes that enhance human metabolism (124).
The microbiota from the colon lives in an anaerobic enviroment coevolving with their host and can transmit genetic and metabolic characteristics to individuals (125). Within the principal metabolites produced by the this microbiota are acetate, propionate and butyrate principal SCFAs that are produced in a molar ratio of 60:20:20 respectively (Figure 3) (97, 126, 129). Regarding SCFAs, acetate production has been characterized in species such as Coprococcus sp. Strain L2-50 is dependent on the activities of butyrate kinase, acetate kinase, and butyryl-CoA: acetate-CoA transferase (130). The abundance of Ruminococcus and Ruminiclostridium genera has also been linked to fecal acetate production, where the majority is processed and absorbed in the liver, and the remaining amount of fecal acetate can be correlated with serum acetate levels (131). The pathways involved in acetate production are pyruvate via acetyl-CoA and the Wood-Ljungdahl pathway (132). Acetate can also be used as a substrate by butyrate-producers like B. fibrisolvens that have enzymes like butyril-CoA:acetate-CoA transferase; therefore, its contribution can favor butyrate production (130).
Figure 3 Thyroid function related to changes in gut microbiota. The figure shows how thyroid diseases influence the gut or vice-versa. Studies in individuals with normal THs levels or in euthyroidism (1), they share a gut microbiota in balance (2), with a normal production of SCFAs being acetate, propionate and butyrate produced in a molar ratio of 60:20:20 respectively (126), and when their microbiota is compared with the microbiota of patients with thyroid disorders (3), these patients shows intestinal dysbiosis with an increase in the abundance of pathogenic bacteria there is an increase in the abundance of pathogenic bacteria with a reduced abundance of commensal bacteria and reduced abundance of commensals that share functions such as SCFAs production, as it has been found in patients with Grave’s disease (127, 128).
Propionate-producing bacteria harbor key enzymes for the succinate pathway or propanediol pathway (133), and the primary genera producing propionate include Bacteroides, Ruminococcus, Veionella, Lactobacillus, and Propionibacterium (131, 133, 134), using carbohydrates, organic acids, and amino acids as primary sources (133). The predominant bacteria in the colon belong to the phylum Firmicutes (135), including most members of the Clostridia class (135), such as Clostridia cluster XIV and Faecalibacteria (136), Bacteroidetes, and Proteobacteria (135), the majority of Gram-positive Firmicutes, which make up between 5-14 percent of the total bacteria found in healthy human feces, are colonic butyrate producers (137–139). The main producer of this metabolite are Clostridium cluster IV (Faecalibacterium prausnitzii) and Clostridium cluster XIVa (Eubacterium rectale/Roseburia spp.) (137, 138). Butyrate can be produced by the condensation of two molecules of acetyl-CoA with a later reduction to butyryl-CoA (132). Other pathways that include bacterial butyryl coenzyme A (CoA): acetate-CoA transferase and acetate kinase activities are also involved (130), using and as substrates carbohydrates, organic acids, glutamate, and lysine (133).
SCFAs are essential fecal anions absorbed in the intestinal epithelia that stimulate Na+-dependent fluid absorption via a cyclic AMP-independent process (140). Non-saturable diffusion of the undissociated acid (HSCFA) can occur through coupled electroneutral NaCl- transport proteins expressed in the intestinal epithelia, enabling the exchange of Cl− and HCO3-, as well as acetate−, propionate−, and butyrate− (141).
The expression of transporters involved in the effective entry of SCFAs is critical for their local and systemic beneficial effects (142). Colonocytes preferentially obtain SCFAs via low-affinity H+ dependent monocarboxylate transporters (MCTs) or sodium-coupled monocarboxylate transporters (SMCTs) (142). SMCTs are high-affinity transporters, among them, SMCT1 (SLC5A8) and SMCT2 (SLC5A12) are expressed in the apical membrane and have electrogenic and electroneutral transport functions that regulate the luminal concentrations of SCFAs (143). Along the length of the human gut, MCT1 and MCT4 expression vary, increasing towards the distal colon (144). The transporter MCT4 (SLC16A3) present in the basolateral membrane of colonocytes, and the transporter MCT1 (SLC16A1), present in both the apical and basolateral membranes of colonocytes, are the principal transporters of SCFAs (143). Previous studies have shown that dietary fiber content may regulate the expression of MCT1, as inadequate expression levels of this transporter can be found in low-fiber diets (145). Fiber fermentation products, such as butyrate, play a critical role in regulating MCT1 gene transcription and the stability of its transcripts (146), but the expression of SMCT1 has been directly related to the modulation of the intestinal microorganisms’ producers of SCFAs (143). In vitro studies showed that in the presence of enteric pathogens such as Escherichia coli, the entry of butyrate can be altered not only by the modulation of Na+/H+ and Cl-/HCO3- exchange but also by reducing the expression of the MCT1 transporter (147). Furthermore, since MCT1 has a basolateral distribution in enterocyte membranes, it can affect the efflux of SCFAs and monocarboxylate metabolites and the maintenance of colonic homeostasis (148, 149).
SCFAs can facilitate signaling events involving G protein-coupled receptors (GPRs), on the cell surface (150, 151). Acetate, propionate, and butyrate are among the ligands shared by some GPRs (152, 153). Within these receptors, we can find GPR109a (also known as hydroxycarboxylic acid receptor 2 or HCA2) expressed in the apical side of intestinal cells, adipose tissue, and immune cells (154), and in different tissues and cells of mammals can be found the free fatty acid receptor 2 (FFAR2; also known as GPR43) and the free fatty acid receptor 3 (FFAR3; also known as GPR41) (155, 156). The production of SCFAs by the gut microbiota can modulate immune cell responses (157). Different immune cells, such as neutrophils and leukocytes, predominate the expression of FFAR2 (158, 159) and have been described to be involved in the inhibition of inflammatory pathways (152, 153), and protective effects by diminishing the susceptibility to bacterial infections with Klebsiella pneumoniae, Citrobacter rodentium, and Staphylococcus aureus, but also by viruses such as respiratory syncytial and influenza viruses (159). Its protective effects can be compromised by inadequate fiber intake or a deficiency of FFAR2 (160). FFAR3 is expressed in neurons that allow neuronal links (155), blood vessel endothelial cells, and adipose tissues (158). FFAR2/3, besides regulating the immune and nervous systems, is also found in enteroendocrine cells (155). Moreover, they can activate signaling pathways related to energy metabolism, appetite control, adipogenesis, and intestinal health (156, 161), in addition to inducing signaling pathways that involve cytoprotective roles by involving nuclear erythroid 2- related factor 2 (Nrf2), contributing to the maintenance of redox homeostasis under physiological conditions, and suppressing carcinogenesis by antiproliferative properties (162).
Intestinal fermentation of carbohydrates results in physiological concentrations of SCFAs between 10 and 100 mM in the gut lumen (163). SCFAs, such as butyrate and propionate, protect intestinal barrier integrity by influencing the expression of MUC2 mRNA levels (164). This effect is advantageous because butyrate at physiological concentrations cannot reach the epithelial stem cells in the intestinal crypts (165), since the majority is used as an energy source by differentiated intestinal epithelial cells of the villus (166, 167). It could also act as a proliferation suppressor and affect epithelial renewal, an effect not observed for propionate and acetate (165). Propionate can generate motor activity in the intestinal epithelia by enhancing the colonic flux of Cl- (168). Therefore, a reduced production of SCFAs led to an increased release of lipopolysaccharide (LPS) into the bloodstream, as it has been observed in patients with hypothyroidism (169). The increase in bloodstream LPS could be related to increased thyroglobulin protein levels (170). Consequently, dysbiosis, and a decreased phylum of Firmicutes along with increased Bifidobacterium and Lactobacillus contribute to the inflammation in AITD (171).
The intestinal microbiota has a substantial impact on energy homeostasis through SCFAs, which can act directly as the primary source of the energy produced in eukaryotic cells in the through major biochemical pathways, such as the tricarboxylic acid (TCA) cycle, oxidative phosphorylation (OXPHOS), and mitochondrial fatty acid β-oxidation (FAO) (172). FAO is a key source of energy for fatty acids metabolism based on the individual’s cellular energy requirements (173). Butyrate is the principal SCFA involved in the maintenance of energy homeostasis. When glucose is unavailable, fatty acids serve as the body’s main source of energy (174). Butyrate was found to increase FAO in keratinocytes (99) due to its ability to regulate FAO-related enzymes such as acyl-CoA dehydrogenase. In colonocytes, butyrate has a function as an energy source rather than an inhibitor of histone deacetylases (HDAC) supporting energy homeostasis and preventing autophagy (166). The mechanism of butyrate can be due to its incorporation to the TCA cycle (especially in citrate) and OXPHOS, producing Acetyl-coenzyme A (acetyl-CoA) to generate ATP (99, 165, 175). Butyrate can produce metabolic reprogramming and affect the behavior of immune cells. In macrophages, butyrate increases OXPHOS for alternative cell activation and anti-inflammatory effects (176). Moreover, it induces the peripheral inducible T regulatory cells (iTreg) by the transformation of butyrate by acyl-CoA synthetase short-chain family member 2 (ACSS2) producing butyryl-CoA (BCoA), which plays a critical role in enhancing FAO stress (177). Additionally, butyrate has been linked to the gut-brain neuronal circuit, improving plasma lipid metabolism and insulin sensitivity, promoting fat oxidation and activating brown adipose tissue (BAT) by inhibiting orexigenic neuron NPY activity in the hypothalamus (178). White adipocytes also take part in several immunological and metabolic processes (179). These cells express SCFA receptors, and it has been suggested that adipocyte metabolism of adipocytes may be influenced by these products (156, 158). In vitro studies have shown that SCFAs may affect glucose metabolism by increasing glucose uptake, fatty acid synthesis (180). It has been reported that, oral administration of propionate and butyrate can improve glucose and insulin tolerance in rats (181, 182). Additionally, several studies conducted in mice orally fed with acetate or butyrate showed a reducion in body fat content due to improved energy expenditure and fat oxidation (181, 183). The evidence available in the literature strongly suggests that SCFAs play a beneficial role in host metabolism; however, there are not enough in vivo studies conducted in humans to support the physiological effects of SCFAs. More studies are needed to understand the impact of SCFAs on glucose and fat metabolism and insulin sensitivity, particularly in adipose tissue.
The majority of immune cells, including B cells, CD4 T cells, CD8 T cells, macrophages, dendritic cells (DCs), innate lymphoid cells (ILCs), and polymorphonuclear leukocytes, express one or more of the GPRs. Thus, SCFAs can bind to these receptors on immune cells to modulate their function. CD4 regulatory T cells (Tregs) are a subset of CD4 T cells that express FoxP3 and/or produce IL-10 and are responsible for the regulation of proinflammatory IFN-ϒ producing Thelper-1 (Th1) and IL-17 producing Th17 cells. The intestine has a large Treg population that maintains homeostasis at the mucosal surfaces, where intestinal bacteria and metabolites play an important role in the generation or maintenance of this Treg population. SCFAs are one of the major bacterial products linked to the expansion and suppressive function of Treg (184, 185). For example, SCFAs from chloroform-resistant fecal bacteria were established as the main bacterial metabolite responsible for the induction of Tregs and suppression of inflammation in a murine model of colitis (186, 187).
Although the precise mechanism through which SCFAs influence Tregs is unknown, SCFAs are thought to enhance the suppressive function of Treg cells (184, 185). Administration of dietary fibers, SCFAs, and gut bacteria with SCFAs-producing ability has been shown to induce Treg cells, and it has been implicated in this by histone deacetylation function of SCFA. HDACs can modify chromatin structure and control genes by removing acetyl groups from specific lysine residues in histone and non-histone proteins (188), which is linked to the regulation of the expression of FoxP3 and IL-10 genes during steady state. Thus, the regulatory vs. inflammatory effects of SCFAs might be context dependent and in steady state, SCFAs will promote the expansion of regulatory cells such as Tregs; however, during chronic inflammatory stages or at super high levels, they might promote a proinflammatory Th1/Th17 response. SCFAs can influence the functions of APCs by modulating their cytokine production and antigen presentation function through GPR and HDAC activation. Butyrate can affect differentiation, maturation, and function of DCs (189–191).
SCFAs can also regulate Treg expansion and function indirectly through modulation of antigen presenting cells (APCs), such as DCs and macrophages. Consequently, during the steady state, SCFAs can boost Treg expansion and function. However, SCFAs have also been shown to induce pro-inflammatory Th1 and Th17 cells during an active immune response. Park et al, showed that SCFAs can induce both effectors as well as Tregs through histone modification (192, 193).
Butyrate, can signal through the receptor for SCFAs GPR109a in macrophages promoting the expansion of Tregs by DCs (194). Similarly, intestinal macrophages treated with butyrate showed reduced levels of LPS induced nitric oxide, IL-6, and IL-12 (195), and can also induce IL-10 producing regulatory B cells, especially IL-10+ IgM+ CD138high plasma cells (196), both ex-vivo and in-vivo. SCFAs, particularly butyrate and propionate, can enhance class-switch DNA recombination at low doses but decrease at higher doses, suggesting a dual dose-dependent effect of SCFAs on B cell class switch (197). These studies highlight that SCFAs, especially butyrate can have significant effects on B cell function and antibody production. Besides these immune subsets, SCFAs can also influence ILCs by increasing ILC-3 activity while downregulating ILC-2 activity, which has been discussed in detail elsewhere (19). Thus, SCFAs can regulate the generation and function of most of the immune cell population; however, further studies are required to understand the precise mechanism through which SCFAs regulate different immune subsets.
According to (198), dysbiosis is an imbalance between commensal and pathogenic microorganisms in the microbiome. Dysbiosis can be divided into three main categories: first as a bloom of pathobionts or low relative abundance of bacteria caused by abnormalities in the intestinal ecosystem; second as the loss of commensals due to microbial death or deduced bacterial proliferation; and third as a reduction of alpha-diversity or richness of species within a site associated with different pathologies (199).
Changes in the composition and balance of the main intestinal microorganisms are associated with an increased risk of obesity (200), IBD (201), and the pathogenesis of irritable bowel syndrome (202). Recent studies have shown a connection between the gut-thyroid axis due to the role of gut microbiota in thyroidal metabolism (203). Patients treated for Hypo or Hyperthyroidism share gut microbiota traits that are consistently associated with diseases such as depression, migraine, gout, type 2 diabetes, cardiac diseases, food allergies, constipation, and IBD (204).
The intestinal microbiota is not the only one that can be affected; in a study performed on patients with high TSH levels and insulin resistance, their oral microbiome showed more bacterial richness and prevalence of microorganisms from the genera Granulicatella, Treponema, and Streptobacillus, with functional profiles related to carbohydrate metabolism, nucleotide, amino acid and energy metabolism (205). Thyroid hormones play a key role in several signaling pathways (40), and are implicated in regulating body temperature by stimulating fatty acid β-oxidation, mitochondrial respiration, biogenesis, and autophagy activity in brown adipose tissue (BAT) and white adipose tissue (WAT) (206, 207), may influence free fatty acid (FFA) and its uptake in the liver (208). More specifically, FT4 and TSH have also been implicated in weight regulation (209), as it has been observed how HDL cholesterol level and waist circumference in patients with metabolic syndrome have been linked to thyroid function (210). Thyroid dysfunction is also prevalent in obese subjects and is an important risk factor for cardiovascular disease and diabetes (211). In a study, it was shown that among thyroid disorders, 27.6% of patients had type 2 diabetes (T2D) and 38.7% had type 1 diabetes (T1D) (4). A cross-sectional study reported an increased risk of overt hypothyroidism and TPOAb positivity among adult Iranian overweight and obese individuals (212).
Dietary fiber intake is associated with a reduced risk of non-communicable chronic diseases (213), and the American Diabetes Association (ADA) recommendations have been associated with significantly lower blood pressure in adult patients with T1D (214), whose pathogenesis involves the action of autoreactive T cells through islet ß-cells, which can be regulated by acetate (150). In the cases of T2D and obesity, both conditions show complex alterations in SCFAs levels, leading to diverse outcomes. For instance, increased butyrate production improves insulin sensitivity, whereas aberrant propionate production or absorption increases the risk of T2D (215). Demonstrating that SCFAs balance is also necessary. On the other hand, obesity is a disease with increasing prevalence, and it is characterized by an excessive accumulation of white adipose tissue that leads to negative effects on health (179, 216). It has been described that both, the composition of the gut microbiota and the availability of THs play a key role in the regulation of metabolism and whole-body energy expenditure (18, 217). Therefore, it is believed that gut microbiota products, particularly SCFAs, together with THs may modulate the progression of diseases like obesity through modifications of the host’s metabolism. The majority of WAT is made up of white adipocytes, a type of cell that is particularly adept at storing energy, primarily in the form of triglycerides. White adipocytes modulate several immunological and metabolic processes through the secretion of various hormones, cytokines, and adipokines (179). It has been shown that the SCFA receptors GPR41 and GPR43, both of which may regulate cell metabolism and function, are expressed by white adipocytes (156, 158). In line with this, it has been described that SCFAs mediate energy balance through the adipose tissue by increasing energy expenditure and fat oxidation (180, 218). Furthermore, T3 regulates the whole machinery responsible for the balance between lipogenesis and lipolysis in WAT (219). Nevertheless, the link between SCFAs, thyroid function, and obesity remains under discussion. It has been described that the microbiota from animals fed a western diet (high fat and high sugar diet) together with sedentarism result in a predominance of Firmicutes over the phyla accompanied by a lower degree of diversity when compared to a low fat, high polysaccharide diet (220). Obesity-induced changes in the microbiota enrichment may thus be suggested as an additional factor to explain the reported correlation between thyroid diseases and obesity.
Once established that a patient suffers from type 1 or 2 diabetes, prediabetes and gestational diabetes mellitus, a fundamental approach in the therapy is a change in lifestyle, and the ADA recommends not only physical activity, smoking cessation, and psychosocial care, but also medical nutrition therapy, emphasizing nutrient-dense carbohydrate sources high in fiber (221). With recommended fiber intake of 14 g per 1,000 kcal (222). It has been described that insulin secretion by pancreatic islets was related to increased degradation of GABA modulated by 4-aminobutanoate degradation V microbial pathway, the pathway of 4-aminobutanoate (GABA) degradation pathway have been related to the production of butyrate and acetate, associated with the abundance of species like Eubacterium rectale and Roseburia intestinalis (215). In addition, human islet ß-cell function can be beneficially changed by propionate as an acute effect to protect these cells from apoptotic stimuli (105). A study conducted on monozygotic twin pairs reported a negative correlation between fecal SCFAs and adiposity parameters, and individuals with visceral obesity had a lower abundance of Bacteroides and Collinsella (223). Interestingly, Jiang et al., reported a decrease in Collinsella but not in Bacteroides in individuals with Graves’ disease (224). In a case-control study, it was found that most patients with metabolic syndrome (37%) had subclinical hypothyroidism (SCH) more frequently than overt hypothyroidism (12%) and overt hyperthyroidism (2%) (225). Currently, most case-control studies have reported an association between obesity and high levels of SCFAs but not gut microbiota richness, at least at the phylum level (226). Additional efforts and studies are needed to understand how the thyroid-gut axis participates in the development of metabolic disorders such as obesity, diabetes, and metabolic syndrome.
An unbalanced microbiota present in the small intestine could have an impact on gastrointestinal function, such as the absorption of key elements for thyroid hormone production or even other nutrients important for fetal growth in pregnant women. Alterations in thyroid gland metabolism caused by hypothyroidism or levothyroxine therapy, as well as diabetes mellitus, are significant contributors to the development of dysbiosis characterized by an increase in bacterial colonization in the small bowel, known as small intestinal bacterial overgrowth syndrome (SIBO), a condition with symptoms such as abdominal discomfort and pain, diarrhea, and weight loss, primarily detected by the lactulose hydrogen breath test (LHBT) where lactulose is a disaccharide that reaches the colon before its absorption, or by the glucose hydrogen breath test (GHBT), where glucose is a monosaccharide absorbed in the first portion of the small intestine (227, 228). These tests have been used to detect SIBO in patients with overt hypothyroidism, revealing an increased incidence of abdominal distention, and treatment of this condition showed improved gastrointestinal symptoms (229). Positive LHBT and GHBT test results were found in pregnant women with subclinical hypothyroidism, a common condition during pregnancy. These patients showed increased levels of TPOAb compared to patients without SIBO; however, it is still unclear whether the dysfunctional flora and its metabolites play a role in inflammatory and immune pathways (228). The relationship between thyroid hormones and intestinal motility is important because it could lead to dysbiosis, as it has been observed in hypothyroidism and its association with altered gastrointestinal motility, which increases the risk of developing SIBO (230).
SCFAs are important for maintaining the health status of the host; however, new studies related to the gut thyroid axis have shown interest in the composition of the intestinal microbiota, and the effect of the products of these metabolites on thyroid disorders remains unknown. Because of the direct or indirect effects of the gut microbiota and its metabolites, they should be considered in the pathogenesis of thyroid disorders (231). In patients with subclinical hypothyroidism, receiving different doses of L-thyroxine showed variations in the relative abundance of certain species from genera with hydrolytic activities, such as the hydrolysis of glucoronate and sulfated iodothyronines like Odoribacter, and Alistipes (203). The treatment prescribed for this condition is based on hormone replacement, and at least 3.8% of patients are chronic levothyroxine users (232). As the gastrointestinal system is crucial for the absorption of levothyroxine (233), little is known about the effect of these chronic treatments on the intestinal microbiota of patients (232). In addition, the microbiota of women with subclinical and overt hypothyroidism has been evaluated, and both studies found an altered abundance of Prevotella genera (234–236), and altered abundances of other commensals and opportunistic pathogens have also been observed in metabolic diseases (Table 1). In hypothyroid Hashimoto’s thyroiditis, is hypothesized that intestinal microbes play a role in triggering the pathogenesis of this autoimmune thyroid disorder (255, 256). Various studies have shown marked dysbiosis in these patients, with reduced bacterial richness and diversity (257), for example, Ishaq et al. found a reduced abundance of Prevotella and Dialister, with an important overgrowth of Bacteroides, Escherichia-Shigella and Parasutterella genera, with the increased prevalence of opportunistic gut bacteria Bacteroides and other species in HT patients (255). Su et al. found a decreased abundance of Prevotella and Fecalibacterium, Bacteroides, and Lachnoclostridium genera, which were enriched in healthy patients, indicating that the composition of the microbiome could be related to the clinical parameters of these patients (256). Animal studies suggest that the microbiota from hypothyroidism patients can cause changes in the thyroid function of mice, which could be related to the reduced abundance of SCFAs-producing bacteria (169). The microbial metabolic processes (energy, carbohydrate, and amino acid metabolism) performed by beneficial microorganisms in patients with HT are affected by the reduced relative abundance of commensals (258), and it is unknown how the levels of SCFAs are in these patients. Where female patients tend to show a different dysbiosis certain microorganisms were more abundant than in male patients, indicating that gender is a factor that should be considered in the dysbiosis found in thyroid diseases (258) (Table 2).
Table 1 Microbial changes related to subclinical and overt hypothyroidism, and the relation of the altered microbiota to metabolic diseases.
Table 2 Microbial changes related to Hashimoto’s Thyroiditis, and the relation of the altered microbiota to metabolic diseases.
In patients with Graves’ disease (GD), an autoimmune disease of the thyroid gland, a clearly altered composition of the gut microbiota has been described (272). At the phylum level, GD patients had a significantly lower proportion of Firmicutes and a significantly higher proportion of Bacteroidetes compared with the controls. This indicates that thyroid hormones may also affect the composition and function of the microbiota, which in turn leads to changes in a person’s weight (224). At the genus level, GD patients had an altered abundance of Faecalibacterium, Bacteroides, Prevotella, and Bifidobacterium and lower numbers of Blautia, Subdoligranulum, Eubacterium species, compared to controls. The functional prediction has revealed that Blautia may be an important microbe in certain metabolic pathways that occur in the hyperthyroid state (224). Another study showed an altered and significantly decreased abundance of metabolic abilities in fatty acid biosynthesis, creatinine degradation, pyruvate fermentation to hexanol, anaerobic energy metabolism, and gluconeogenesis (128). These patients with GD showed reduced levels of SCFAs (127, 128), which could be related to the susceptibility of these patients to intestinal epithelial cell injury and increased intestinal permeability by alterations in the tight junctions in the intestinal epithelial cells, according to studies in patients with GD who had significantly higher serum levels of serum LPS, intestinal fatty acid-binding protein (I-FABP), zonulin, and D-lactate (273).
Other authors have related the increased abundance of Bacteroides to its ability to produce SCFAs other than butyric acid, including succinate, propionate, and acetate, indicating that it might be implicated in the impairment of intestinal barrier function because these metabolites do not induce mucin production (224), resulting in the release of a large number of pro-inflammatory factors outside the intestine and causing immune dysfunction (224). A study evaluating the combination of the treatment for GD, methimazole (MI) in combination with potential prebiotic berberine supplementation, showed an improved abundance of beneficial species like Lactococcus lactis while decreasing the abundance of pathogenic bacteria like Enterobacter hormaechei and Chryseobacterium indologenes, and also improved FT3 and TSH indices to normal levels, relating TSH to Faecalibacterium prausnitzii and enterobactin biosynthesis, and with Lactococcus lactis were positively correlated with the three metabolic pathways of vitamin K2 synthesis, whereas MI alone restored only FT3 and had no modifications on the microbiota (274), demonstrating the importance of evaluating the combination of treatments that could provide benefits for the host microbiota and how to ameliorate the symptoms of the disease. Other studies found an increase in pathogenic bacteria and opportunistic pathogens in these patients, which are related to significant differences in the reduced abundance of acetic, propionic, and butyric acids with an increased abundance of cholate and chenodeoxycholate (128). Even when these metabolites were detected in silico (275), their identification is considered a potential biomarker for the disease. In both autoimmune thyroid diseases (AITDs), besides showing differences in the core microbioma of GD and HT patients, in both were found concrete bacteria that could be related to their reduced tolerance to self-antigens (276) (Figure 3). Therefore, evidence suggests a key role of microbiota status in thyroid diseases, where metabolic changes and dysbiosis are related to an altered composition of SCFAs producers and an increased abundance of pathogens (Table 3).
Table 3 Microbial changes related to Hyperthyroidism and Grave’s Disease, and the relation of the altered microbiota to metabolic diseases.
There is limited information available of the thyroid gut-axis besides knowing the dysbiosis found in thyroid disorders as it was described in the previous section, highlighting new findings related to the content of SCFA of these patients. Nevertheless, little is known about how SCFA affect thyroid function or how they cooperate (289). However, it is important to revisit that a primary contribution of SCFA’s to cell homeostasis is the regulation of HDAC (251), especially butyrate and propionate can inhibit HDAC (184), preventing the removal of acetyl groups on certain lysine residues in histones and non-histone proteins that control gene expression (188). Other described epigenetically active mechanisms used by SCFAs are histone butyrylation (by the conversion of butyrate to butyryl-CoA) or propionylation (by the converting propionate to propionyl-CoA) (290). Histone acetylation may be crucial for the control of hormone-mediated transcriptional regulation (291), and some studies have shown that butyrate can regulate thyroid hormone receptors levels through the acetylation of chromatin-associated proteins in rat cells (292–294), promoting the expression of the growth hormone (GH) which is regulated by T3 and retinoic acid receptors, as result of the accumulation of hyperacetylated histones (291). Moreover, it has been described that the treatment of these animals with n-butyrate, could modulate gene expression by increasing the binding capacity of T3 on nuclear thyroid hormone receptors in the rat liver (295).
Even when the in vivo and in vitro studies about the evaluation of thyroid hormone receptors and butyrate here mentioned are at least 40 years old. It is important that new studies of the gut-thyroid axis, could generate new information that laid the foundations for the role of the microbiota and its metabolites in thyroid function, which is quite useful for developing potential treatments for thyroid-associated diseases (296), especially considering that autoimmune diseases like HT have increased inflammation that could be related to dysbiosis that leads to altered levels of SCFAs (297), and the bacterial metabolites have an important role in maintaining a balance the pro- and anti-inflammatory environment (184).
Given the great importance of SCFAs as bacterial metabolites produced by components of the microbiota and their relevance in processes including regulation of metabolism, immune system development and activation, improving gut barrier function, and their role in the occurrence of metabolic diseases such as thyroid diseases (132), it is essential to have one or more robust methods to measure the levels of these molecules in biological samples such as feces, luminal contents, or serum. Evidence has suggested that for detecting SCFAs, it is necessary to perform a meticulous analysis of these SCFAs with an accurate extraction and sample preparation process to guarantee the correct SCFAs profiling (298, 299). In this context, several methods of SCFAs extraction, purification, and treatment of biological samples have been described, including techniques of sample derivatization and methods of purification to obtain a fast sample preparation (300). The unique physicochemical properties of SCFAs, such as their high polarity, low vapor pressure, high volatility, and complex matrix of biological samples, require highly sensitive, selective, and accurate methods for their determination (299). In this sense, diverse methods have been developed to detect SCFAs in biological samples (300), including separation-based techniques such as gas chromatography (GC), high-performance liquid chromatography (HPLC), or mass spectrometry (MS), and their hyphenation with MS (GC/MS or HPLC/MS) the most common methods (301–305). Nevertheless, techniques such as capillary electrophoresis (CE) or nuclear magnetic resonance (NMR) are frequently used (306, 307). Within the analytical methods for SCFAs, the GC remains the most commonly used technique because of its high resolution and sensitivity (299), in addition to the advantage of being able to be attached to a flame ionization detector (FID) (308, 309) or to a mass spectrometer (MS) (303, 310–312), that can further enhance the selectivity and sensitivity of the method. However, the HPLC technique is also favored for its sensitivity and ability to handle complex samples (313), as well as the advantages of coupling fluorescence, ultraviolet–visible light (UV–VIS) (304), electrochemical detection (ECD) (314) and MS detectors (315, 316). Both methods involve the separation of SCFAs based on their physical and chemical properties, followed by the detection and quantification of these metabolites. Although the GC/MS and HPLC/MS methods offer excellent SCFAs analysis alternatives, they often require a sample chemical derivatization process with specific techniques according to the method to be used, to improve their volatility and/or retention properties in the column of equipment (300). However, it is necessary to carry out an extraction and purification process before SCFAs derivatization and analysis because for some samples the detection of small concentrations of SCFAs can be challenging due to high concentration of other biological constituents, which complicates the precision and reproducibility of the method and results in instrumental contamination, which makes it challenging to analyze and quantify SCFAs. As a result, it is crucial to perform a selective extraction and correct derivatization of SCFAs.
Several physical pretreatments are used to enhance and accelerate SCFAs extraction processes, such as filtration, ultrafiltration, or centrifugation, which are fast and simple, but, despite the rapidity of these extraction methods, have the drawback that a large number of impurities may also be extracted, leading to incomplete separation of the SCFAs and therefore contaminating the chromatographic column reducing its useful life (310, 317, 318). The use of steam distillation and vacuum distillation, which are carried out at low temperatures and pressure, are other methods for the separation of SCFAs, however, over a long period can generate the possibility of a loss of volatile acids (319, 320). One extraction strategy widely used is the acidification of these compounds, which enhances the hydrophobicity and therefore facilitates extraction with apolar organic solvents, which could be complemented with a liquid-liquid extraction (LLE) (321, 322). In this same context, extraction in the absence of solvent employing a solid phase microextraction device (SPME) taking advantage of the volatility of SCFAs is a technique that is increasingly used due to its speed, selectivity, and sensitivity (308, 312).
On the other hand, as previously mentioned, a frequently used approach for the quantification of SCFAs involves chemical derivatization followed by analysis using separation-based techniques. The derivatization strategies allow the change in the SCFAs properties and have been used to enhance the compound’s separations and increased resolution allowing for more accurate and sensitive measurement. The most common techniques for the derivatization of SCFAs are the conversion of SCFAs to their corresponding fatty acid esters or the corresponding methyl esters, derivatives that enhance their volatility and improve detection by gas chromatography (GC) or mass spectrometry (MS) (300, 323). In contrast, converting SCFAs to their corresponding 2,4-dinitrophenylhydrazine derivatives or Trifluoroacetic acid (TFA) treatment are derivatization strategies used to be easily quantified using high-performance liquid chromatography (HPLC) (304, 324). It is important to note that each derivatization technique has its own set of advantages, and the choice of an adequate method will depend on the specific application and the analytical requirements. Finally, another alternative SCFAs determination method is enzymatic detection. This technique involves the use of specific enzymes to convert SCFAs into a detectable product, which can then be quantified using various methods, such as UV spectrophotometry. In addition, the direct detection method for SCFAs is the ELISA technique (Enzyme-Linked Immunosorbent Assay), which utilizes antibodies specific to the SCFAs (325). Although ELISA is a rapid and simple method, however it is less sensitive than GC, HPLC, or MS. It is important to note that the choice of detection method will depend on the specific application, the type of sample, and the desired sensitivity and specificity. Furthermore, the use of derivatization techniques, as discussed earlier, can enhance the detection and analysis of SCFAs. In conclusion, the detection of SCFAs is crucial for understanding their role in various physiological and pathological processes and the development of new therapies. The advancement of analytical techniques, along with the increasing recognition of the importance of SCFAs, will likely continue to drive new insights into their detection and characterization.
Even though SCFAs have been studied in the context of thyroid disorders, there is limited supporting evidence. Gas chromatography-mass spectrometry (GC-MS) measurements of SCFAs in patients with Grave’s disease revealed decreased levels of acetic, propionic, and butyric acid (127, 128). However, more studies in other thyroid disorders are needed, although it has been suggested that SCFAs may indirectly influence thyroid function by affecting gut microbiota and immune function (326). Given that butyrate, which plays a role in regulating the immune system and has anti-inflammatory effects that could be potentially beneficial for people with AITDs such as Hashimoto’s thyroiditis (327).
Although there is ongoing research to identify new biomarkers for thyroid diseases, and SCFAs may be among the compounds studied in this context, more investigation is needed to understand the relationship between SCFAs and thyroid disorders.
Thyroid disorders are involved for the development of diseases or chronic metabolic conditions such as type 1 diabetes and type 2 diabetes. Iodine nutrition is considered the main cause of thyroid disorders’ development. In this context, adequate nutrition can be a protective factor to avoid the development of thyroid disease and improve thyroid function. Key nutrients like iodine, selenium, and iron uptake, can be affected by an alteration of the gut microbiota homeostasis, influencing the host’s systemic metabolism. Therefore, THs availability and a balanced gut microbiota are key for the regulation of metabolism. Thyroid disorders are more common in women, and the relationship between gut microbiota, thyroid conditions during pregnancy, and their effects on the offspring’s immune system is far from being completely understood. What is known is that dysbiosis is often observed in thyroid disorders, but the enzymatic and metabolic pathways affected by the abundance or depletion of certain microorganisms in these conditions need to be described. Here we show that there is no strong research demonstrating the impact of SCFAs on thyroid function, even though there is no doubt that SCFAs have beneficial effects on important organs, tissues, and cells, considering that SCFAs allowing chemical communication both locally at the intestinal mucosa and systemically to the rest of the organs. In this work, it is described how a low abundance of beneficial organisms correlated with altered SCFAs production, highlighting the importance of SCFAs detection as an evaluation tool for the patient’s gut microbiota’s environment and the need for more research on metabolites linking gut dysbiosis and thyroid function to improve the patient’s life quality.
Conception, design, illustrations of Figures 2, 3, drafting of the manuscript (MM-L). MM-L, AM, AR, EG-M, MR-R, OA-M, OV, SB, YD and MO wrote sections of the manuscript. CR, MO, AM and FM-G contributed to the literature review and manuscript revision. AK, CM contributed with manuscript revision. All authors contributed to the article and approved the submitted version.
This work was supported by Agencia Nacional de Investigación y Desarrollo (ANID) - Millennium Science Initiative Program, Millennium Institute on Immunology and Immunotherapy (ICN09_016 / ICN 2021_045; former P09/016-F). Agencia Nacional de Investigación y Desarrollo (ANID) -Fondo de Fomento al Desarrollo Científico y Tecnológico ID20I10082 (SB); FONDECYT 1191300 (CR), 11200764 (FM-G), 11201113 (YD); ANID Becas Doctorado Nacional 21211419 (MM-L), 21202085 (EG-M).
Millennium Institute of Immunology and Immunotherapy (ICN09_016 / ICN 2021_045; former P09/016-F).
The authors declare that the research was conducted in the absence of any commercial or financial relationships that could be construed as a potential conflict of interest.
All claims expressed in this article are solely those of the authors and do not necessarily represent those of their affiliated organizations, or those of the publisher, the editors and the reviewers. Any product that may be evaluated in this article, or claim that may be made by its manufacturer, is not guaranteed or endorsed by the publisher.
1. Taylor P, Albrecht D, Scholz A, Gutierrez-Buey G, Lazarus J, Dayan C, et al. Global epidemiology of hyperthyroidism and hypothyroidism. Nat Rev Endocrinol (2018) 14(5):301–16. doi: 10.1038/nrendo.2018.18
2. ATA. Prevalence and impact of thyroid disease. American thyroid association (2022). Available at: https://www.thyroid.org/media-main/press-room/.
3. Madariaga A, Santos Palacios S, Guillén-Grima F, Galofré J. The incidence and prevalence of thyroid dysfunction in Europe: a meta-analysis. J Clin Endocrinol Metab (2014) 99(3):923–31. doi: 10.1210/jc.2013-2409
4. Nearmeen R, Ghada S. Prevalence, risks, and comorbidity of thyroid dysfunction: a cross-sectional epidemiological study. Egyptian J Internal Med (2020) 31(4):635–41. doi: 10.4103/ejim.ejim_22_19
5. Hennessey J, Garber J, Woeber K, Cobin R, Klein I. American Association of clinical endocrinologists and American college of endocrinology position statement on thyroid dysfunction case finding. Endocrine Pract (2016) 22(2):262–70. doi: 10.4158/EP151038.PS
6. Kafle B, Khadka B, Tiwari M. Prevalence of thyroid dysfunction among depression patients in a tertiary care centre. J Nepal Med Assoc (2020) 58(229):654–8. doi: 10.31729/jnma.5296
7. Shun CB, Donaghue KC, Phelan H, Twigg SM, Craig ME. Thyroid autoimmunity in type 1 diabetes: systematic review and meta-analysis. Diabetic Med (2014) 31(2):126–35. doi: 10.1111/dme.12318
8. Alexander E, Pearce E, Brent G, Brown R, Chen H, Dosiou C, et al. 2017 guidelines of the American thyroid association for the diagnosis and management of thyroid disease during pregnancy and the postpartum. Thyroid (2017) 27(3):315–89. doi: 10.1089/thy.2016.0457
9. Gupta P, Jain M, Verma V, Gupta N. The study of prevalence and pattern of thyroid disorder in pregnant women: a prospective study. Cureus (2021) 13(7):1–7. doi: 10.7759/cureus.16457
10. Benseñor I, Sgarbi J, Castro C, Almeida B, de Fátima M, da Conceição C, et al. Incidence of thyroid diseases: results from the Brazilian longitudinal study of adult health (ELSA-brasil). Arch Endocrinol Metab (2021) 65(4):468–78. doi: 10.20945/2359-3997000000348
11. Valdes A, Walter J, Segal E, Spector T. Role of the gut microbiota in nutrition and health. BMJ (2018) 361:36–44. doi: 10.1136/bmj.k2179
12. Knezevic J, Starchl C, Berisha AT, Amrein K. Thyroid-gut-axis: how does the microbiota influence thyroid function? Nutrients (2020) 12(6):1–16. doi: 10.3390/nu12061769
13. FAO/WHO. Proposal for a definition and methods if anlysis for dietary fibre content. 17th ed Vol. Vol. 5. Horwitz Ed., editor. Rome: Official Methods of Analysis of AOAC International (2004). Available at: http://www.fao.org/tempref/codex/Meetings/CCNFSDU/ccnfsdu26/nf2603ae.pdf.
14. Layden B, Angueira A, Brodsky M, Durai V, Lowe W. Short chain fatty acids and their receptors: new metabolic targets. Trans Res (2013) 161(3):131–40. doi: 10.1016/j.trsl.2012.10.007
15. Morrison D, Preston T. Formation of short chain fatty acids by the gut microbiota and their impact on human metabolism. Gut Microbes (2016) 7(3):189–200. doi: 10.1080/19490976.2015.1134082
16. Grundy M, Edwards C, Mackie A, Gidley M, Butterworth P, Ellis P. Re-evaluation of the mechanisms of dietary fibre and implications for macronutrient bioaccessibility, digestion and postprandial metabolism. Br J Nutr (2016) 116(5):816–33. doi: 10.1017/S0007114516002610
17. Holscher HD. Dietary fiber and prebiotics and the gastrointestinal microbiota. Gut Microbes (2017) 8(2):172–84. doi: 10.1080/19490976.2017.1290756
18. LeBlanc J, Chain F, Martín R, Bermúdez-Humarán L, Courau S, Langella P. Beneficial effects on host energy metabolism of short-chain fatty acids and vitamins produced by commensal and probiotic bacteria. Microbial Cell Factories (2017) 16(1):1–10. doi: 10.1186/s12934-017-0691-z
19. Kim CH. Control of lymphocyte functions by gut microbiota-derived short-chain fatty acids. Cell Mol Immunol (2021) 18(5):1161–71. doi: 10.1038/s41423-020-00625-0
20. Nastasi C, Fredholm S, Willerslev-Olsen A, Hansen M, Bonefeld C, Geisler C, et al. Butyrate and propionate inhibit antigen-specific CD8+ T cell activation by suppressing IL-12 production by antigen-presenting cells. Sci Rep (2017) 7(1):1–10. doi: 10.1038/s41598-017-15099-w
21. Sun M, Wu W, Chen L, Yang W, Huang X, Ma C, et al. Microbiota-derived short-chain fatty acids promote Th1 cell IL-10 production to maintain intestinal homeostasis. Nat Commun (2018) 9(1):1–15. doi: 10.1038/s41467-018-05901-2
22. Yang W, Yu T, Huang X, Bilotta A, Xu L, Lu Y, et al. Intestinal microbiota-derived short-chain fatty acids regulation of immune cell IL-22 production and gut immunity. Nat Commun (2020) 11(1):1–18. doi: 10.1038/s41467-020-18262-6
23. Montanari C, Parolisi S, Borghi E, Putignani L, Bassanini G, Zuvadelli J, et al. Dysbiosis, host metabolism, and non-communicable diseases: trialogue in the inborn errors of metabolism. Front Physiol (2021) 12:716520. doi: 10.3389/fphys.2021.716520
24. Mondal S, Raja K, Schweizer U, Mugesh G. Chemistry and biology in the biosynthesis and action of thyroid hormones. Angewandte Chemie - Int Edition (2016) 55(27):7606–30. doi: 10.1002/anie.201601116
25. van der Spek AH, Fliers E, Boelen A. The classic pathways of thyroid hormone metabolism. Mol. Cell. Endocrinol (2017) 458:29–38. doi: 10.1016/j.mce.2017.01.025
26. Ravera S, Reyna-Neyra A, Ferrandino G, Amzel L, Carrasco N. The Sodium/Iodide symporter (NIS): molecular physiology and preclinical and clinical applications. Annu Rev Physiol (2017) 79:261–89. doi: 10.1146/annurev-physiol-022516-034125
27. Rodriguez A-M, Perron B, Lacroix L, Caillou B, Leblanc G, Schlumberger M, et al. Identification and characterization of a putative human iodide transporter located at the apical membrane of thyrocytes. J Clin Endocrinol Metab (2002) 87(7):3500–3. doi: 10.1210/jcem.87.7.8797
28. Bizhanova A, Kopp P. Minireview: the sodium-iodide symporter NIS and pendrin in iodide homeostasis of the thyroid. Endocrinology (2009) 150(3):1084–90. doi: 10.1210/en.2008-1437
29. Yoshihara A, Hara T, Kawashima A, Akama T, Tanigawa K, Wu H, et al. Regulation of dual oxidase expression and H2O2 production by thyroglobulin. Thyroid (2012) 22(10):1054–62. doi: 10.1089/thy.2012.0003
30. Carvalho D, Dupuy C. Role of the NADPH oxidases DUOX and NOX4 in thyroid oxidative stress. Eur Thyroid J (2013) 2(3):160–7. doi: 10.1159/000354745
31. de Faria C, Fortunato R. The role of dual oxidases in physiology and cancer. Genet Mol Biol (2020) 43(1):1–9. doi: 10.1590/1678-4685/GMB-2019-0096
32. Szanto I, Pusztaszeri M, Mavromati M. H2O2 metabolism in normal thyroid cells and in thyroid tumorigenesis: focus on NADPH oxidases. Antioxidants (2019) 8(5):1–21. doi: 10.3390/antiox8050126
33. Ruf J, Carayon P. Structural and functional aspects of thyroid peroxidase. Arch Biochem Biophysics (2006) 445(2):269–77. doi: 10.1016/j.abb.2005.06.023
34. Dillmann WH. Cellular action of thyroid hormone. In: Thyroid California, US: Mary Ann Liebert, Inc., vol. Vol. 12. (2002). doi: 10.1089/105072502760143809
35. Carvalho D, Dupuy C. Thyroid hormone biosynthesis and release. Mol Cell Endocrinol (2017) 458:6–15. doi: 10.1016/j.mce.2017.01.038
36. Gomes-Lima C, Wartofsky L, Burman K. Can reverse T3 assay be employed to guide T4 vs. T4/T3 therapy in hypothyroidism? Front Endocrinol (2019) 10:856. doi: 10.3389/fendo.2019.00856
37. Braun D, Schweizer U. Thyroid hormone transport and transporters. In: Vitamins and hormones, 1st ed, vol. 106. Germany: Elsevier Inc (2018). doi: 10.1016/bs.vh.2017.04.005
38. Yen P. Physiological and molecular basis of thyroid hormone action. Physiol Rev (2001) 81(3):1097–142. doi: 10.1152/physrev.2001.81.3.1097
39. Singh B, Yen P. A clinician’s guide to understanding resistance to thyroid hormone due to receptor mutations in the TRα and TRβ isoforms. Clin Diabetes Endocrinol (2017) 3(1):1–11. doi: 10.1186/s40842-017-0046-z
40. Brent GA. Mechanisms of thyroid hormone action. J Clin Invest (2012) 122(9):3035–43. doi: 10.1172/JCI60047
41. Korta P, Pocheć E. Glycosylation of thyroid-stimulating hormone receptor. Endokrynologia Polska (2019) 70(1):86–93. doi: 10.5603/EP.a2018.0077
42. Feldt-Rasmussen U, Effraimidis G, Klose M. The hypothalamus-pituitary-thyroid (HPT)-axis and its role in physiology and pathophysiology of other hypothalamus-pituitary functions. Mol Cell Endocrinol (2021) 525:1–12. doi: 10.1016/j.mce.2021.111173
43. Luongo C, Dentice M, Salvatore D. Deiodinases and their intricate role in thyroid hormone homeostasis. Nat Rev Endocrinol (2019) 15(8):479–88. doi: 10.1038/s41574-019-0218-2
44. Germain D, Galton V, Hernandez A. Miniereview: defining the roles of the lodothyronine deiodinases: current concepts and challenges. Endocrinology (2009) 150(3):1097–107. doi: 10.1210/en.2008-1588
45. Groeneweg S, Van Geest F, Peeters R, Heuer H, Visser W. Thyroid hormone transporters. Endocrine Rev (2019) 41(2):146–201. doi: 10.1210/endrev/bnz008
46. Bernal J. Thyroid hormone receptors in brain development and function. Nat Clin Pract Endocrinol Metab (2007) 3(3):249–59. doi: 10.1038/ncpendmet0424
47. de Oliveira M, Luvizotto R, Olimpio R, Sibio M, Silva C, Conde S, et al. Modulation of thyroid hormone receptors, TRα and TRβ, by using different doses of triiodothyronine (T3) at different times. Arq Bras Endocrinol Metab (2013) 57(5):368–74. doi: 10.1590/s0004-27302013000500006
48. Bergh J, Lin H, Lansing L, Mohamed S, Davis F, Mousa S, et al. Integrin αVβ3 contains a cell surface receptor site for thyroid hormone that is linked to activation of mitogen-activated protein kinase and induction of angiogenesis. Endocrinology (2005) 146(7):2864–71. doi: 10.1210/en.2005-0102
49. Hiroi Y, Kim H, Ying H, Furuya F, Huang Z, Simoncini T, et al. Rapid nongenomic actions of thyroid hormone. Proc Natl Acad Sci USA (2006) 103(38):14104–9. doi: 10.1073/pnas.0601600103
50. Baskin H, Duick D, Guttler R, Kaplan M, Segal R, Hamilton C, et al. American Association of clinical endocrinologists medical guidelines for clinical practice for the evaluation and treatment of American association of clinical endocrinologists medical guidelines for clinical practice. Endocrine Pract (2002) 8(6):457–69. doi: 10.4158/1934-2403-8.6.457
51. Winther K, Rayman M, Bonnema S, Hegedüs L. Selenium in thyroid disorders [[/amp]]mdash; essential knowledge for clinicians. Nat Rev Endocrinol (2020) 16(3):165–76. doi: 10.1038/s41574-019-0311-6
52. Hollowell J, Staehling N, Flanders D, Hannon H, Gunter E, Spencer C, et al. Serum TSH, T4, and thyroid antibodies in the united states population, (1988 to 1994): national health and nutrition examination survey (NHANES III). J Clin Endocrinol Metab (2002) 87(2):489–99. doi: 10.1210/jcem.87.2.8182
53. Agboola-Abu C, Kuku S. Experience in the use of radioactive iodine therapy for hyperthyroidism in Nigerian patients. a study of twenty-two patients. West Afr J Med (2003) 22(4):324–8. doi: 10.4314/wajm.v22i4.28057
54. De Leo S, Lee SY, Braverman L. Hyperthyroidism. Lancet (2016) 388(10047):906–18. doi: 10.1016/S0140-6736(16)00278-6
55. Biondi B, Cooper DS. Subclinical hyperthyroidism. N Engl J Med (2018) 378(25):2411–9. doi: 10.1056/NEJMcp1709318
56. Chaker L, Bianco A, Jonklaas J, Peeters R. Hypothyroidism. Lancet (2017) 390(10101):1550–62. doi: 10.1016/S0140-6736(17)30703-1
57. Zimmermann M, Boelaert K. Iodine deficiency and thyroid disorders. Lancet Diabetes Endocrinol (2015) 3(4):286–95. doi: 10.1016/S2213-8587(14)70225-6
58. Ragusa F, Fallahi P, Elia G, Gonnella D, Paparo SR, Giusti C, et al. Hashimotos’ thyroiditis: epidemiology, pathogenesis, clinic and therapy. Best Pract Res: Clin Endocrinol Metab (2019) 33(6):101367. doi: 10.1016/j.beem.2019.101367
59. Hu X, Chen Y, Shen Y, Tian R, Sheng Y, Que H. Global prevalence and epidemiological trends of hashimoto’s thyroiditis in adults: a systematic review and meta-analysis. Front Public Health (2022) 10:1020709. doi: 10.3389/fpubh.2022.1020709
60. Biondi B, Cappola AR, Cooper DS. Subclinical hypothyroidism: a review. JAMA - J Am Med Assoc (2019) 322, Issue 2:153–160). doi: 10.1001/jama.2019.9052
61. Yoo WS, Chung HK. Subclinical hypothyroidism: prevalence, health impact, and treatment landscape. Endocrinol Metab (2021) 36(3):500–13. doi: 10.3803/ENM.2021.1066
62. Negro R, Soldin O, Obregon M, Stagnaro-Green A. Hypothyroxinemia and pregnancy. Endocr Pract (2011) 17(3):422–9. doi: 10.4158/EP10309.RA
63. Lavado-Autric R, Ausó E, García-Velasco JV, Del Carmen Arufe M, Escobar del Rey F, Berbel P, et al. Early maternal hypothyroxinemia alters histogenesis and cerebral cortex cytoarchitecture of the progeny. J Clin Invest (2003) 111(7):1073–82. doi: 10.1172/JCI200316262
64. Moleti M, Trimarchi F, Vermiglio F. Doubts and concerns about isolated maternal hypothyroxinemia. J Thyroid Res (2011) 2011:1–7. doi: 10.4061/2011/463029
65. López-Muñoz E, Mateos-Sánchez L, Mejía-Terrazas G, Bedwell-Cordero S. Hypothyroidism and isolated hypothyroxinemia in pregnancy, from physiology to the clinic. Taiwanese J Obstet Gynecol (2019) 58(6):757–63. doi: 10.1016/j.tjog.2019.09.005
66. Dosiou C, Medici M. Isolated maternal hypothyroxinemia during pregnancy: knowns and unknowns. Eur J Endocrinol (2017) 176(1):R21–38. doi: 10.1530/EJE-16-0354
67. Ghassabian A, Pierotti L, Basterrechea M, Chatzi L, Estarlich M, Fernández-Somoano A, et al. Association of exposure to ambient air pollution with thyroid function during pregnancy. JAMA Network Open (2019) 2(10):1–15. doi: 10.1001/jamanetworkopen.2019.12902
68. Chen Y, Xue F. The impact of gestational hypothyroxinemia on the cognitive and motor development of offspring. J Maternal-Fetal Neonatal Med (2020) 33(11):1940–5. doi: 10.1080/14767058.2018.1529749
69. Román GC, Ghassabian A, Bongers-Schokking JJ, Jaddoe VWV, Hofman A, de Rijke YB, et al. Association of gestational maternal hypothyroxinemia and increased autism risk. Ann Neurol (2013) 74(5):733–42. doi: 10.1002/ana.23976
70. Päkkilä F, Männistö T, Pouta A, Hartikainen A, Ruokonen A, Surcel H, et al. The impact of gestational thyroid hormone concentrations on ADHD symptoms of the child. J Clin Endocrinol Metab (2014) 99(1):1–8. doi: 10.1210/jc.2013-2943
71. Modesto T, Tiemeier H, Peeters R, Jaddoe V, Hofman A, Verhulst F, et al. Maternal mild thyroid hormone insufficiency in early pregnancy and attention-deficit/Hyperactivity disorder symptoms in children. JAMA Pediatr (2015) 169(9):838–45. doi: 10.1001/jamapediatrics.2015.0498
72. Gyllenberg D, Sourander A, Heljä-Marja S, Hinkka-Yli-Salomäki S, McKeague I, Brown A. Hypothyroxinemia during gestation and offspring schizophrenia in a national birth cohort. Biol Psychiatry (2016) 79(12):962–70. doi: 10.1016/j.biopsych.2015.06.014
73. Levie D, Korevaar T, Bath S, Dalmau-Bueno A, Murcia M, Espada M, et al. Thyroid function in early pregnancy, child IQ, and autistic traits: a meta-analysis of individual participant data. J Clin Endocrinol Metab (2018) 103(8):2967–79. doi: 10.1210/jc.2018-00224
74. Spann M, Cheslack-Postava K, Brown A. The association of serologically documented maternal thyroid conditions during pregnancy with bipolar disorder in offspring. Bipolar Disord (2020) 22(6):621–8. doi: 10.1111/bdi.12879
75. Haensgen H, Albornoz E, Opazo M, Bugueño K, Jara Fernández E, Binzberger R, et al. Gestational hypothyroxinemia affects its offspring with a reduced suppressive capacity impairing the outcome of the experimental autoimmune encephalomyelitis. Front Immunol (2018) 9:1257(JUN). doi: 10.3389/fimmu.2018.01257
76. González-Madrid E, Rangel-Ramírez M, Mendoza-León M, Álvarez-Mardones O, González P, Kalergis A, et al. Risk factors from pregnancy to adulthood in multiple sclerosis outcome. Int J Mol Sci (2022) 23(13):1–25. doi: 10.3390/ijms23137080
77. Biban B, Lichiardopol C. Iodine deficiency, still a global problem? Curr Health Sci J (2017) 43(2):103–11. doi: 10.12865/CHSJ.43.02.01
78. Hatch-McChesney A, Lieberman H. Iodine and iodine deficiency: a comprehensive review of a re-emerging issue. Nutrients (2022) 14(3473):1–11. doi: 10.3390/nu14173474
79. Schomburg L, Köhrle J. On the importance of selenium and iodine metabolism for thyroid hormone biosynthesis and human health. Mol Nutr Food Res (2008) 52, Issue 11:1235–1246). doi: 10.1002/mnfr.200700465
80. Brough L. Iodine intake for pregnant and breastfeeding women and their infants remains a global concern. J Nutr (2021) 151, Issue 12:3604–3605). doi: 10.1093/jn/nxab364
81. Winder M, Kosztyła Z, Boral A, Kocełak P, Chudek J. The impact of iodine concentration disorders on health and cancer. Nutrients (2022) 14, Issue 11:1–17. doi: 10.3390/nu14112209
82. Fordyce FM. Database of the iodine content of food and diets populated with data from published literature (2003). Available at: nora.nerc.ac.uk/id/eprint/8354/.
83. Smyth P. Iodine, seaweed, and the thyroid. Eur Thyroid J (2021) 10, Issue 2:101–108). doi: 10.1159/000512971
84. Rakhra V, Galappaththy L, Bulchandani S, Cabandugama P. Obesity and the Western diet: how we got here. Missouri Med (2020) 117(6):536–8.
85. Więckowska-Gacek A, Mietelska-Porowska A, Wydrych M, Wojda U. Western Diet as a trigger of alzheimer’s disease: from metabolic syndrome and systemic inflammation to neuroinflammation and neurodegeneration. In: Ageing research reviews, vol. Vol. 70. Poland: Elsevier Ireland Ltd (2021). doi: 10.1016/j.arr.2021.101397
86. Siri-Tarino P, Sun Q, Hu F, Krauss R. Saturated fat, carbohydrate, and cardiovascular disease. Am J Clin Nutr (2010) 91, Issue 3:502–509. doi: 10.3945/ajcn.2008.26285
87. Martinez-Medina M, Denizot J, Dreux N, Robin F, Billard E, Bonnet R, et al. Western Diet induces dysbiosis with increased e coli in CEABAC10 mice, alters host barrier function favouring AIEC colonisation. Gut (2014) 63(1):116–24. doi: 10.1136/gutjnl-2012-304119
88. Fiolet T, Srour B, Sellem L, Kesse-Guyot E, Allès B, Méjean C, et al. Consumption of ultra-processed foods and cancer risk: results from NutriNet-santé prospective cohort. BMJ (2018) 360:1–11. doi: 10.1136/bmj.k322
89. Christ A, Lauterbach M, Latz E. Western Diet and the immune system: an inflammatory connection. Immunity (2019) 51(5):794–811. doi: 10.1016/j.immuni.2019.09.020
90. Venturelli S, Leischner C, Helling T, Burkard M, Marongiu L. Vitamins as possible cancer biomarkers: significance and limitations. Nutrients (2021) 13(11):1–23. doi: 10.3390/nu13113914
91. Han H, Xin P, Zhao L, Xu J, Xia Y, Yang X, et al. Excess iodine and high-fat diet combination modulates lipid profile, thyroid hormone, and hepatic LDLr expression values in mice. Biol Trace Element Res (2012) 147(1–3):233–9. doi: 10.1007/s12011-011-9300-x
92. Vega-Vega O, Fonseca-Correa J, Mendoza-De la Garza A, Rincón-Pedrero R, Espinosa-Cuevas A, Baeza-Arias Y, et al. Contemporary dietary intake: too much sodium, not enough potassium, yet sufficient iodine: the SALMEX cohort results. Nutrients (2018) 10(7):1–11. doi: 10.3390/nu10070816
93. Shi Z. Gut microbiota: an important link between western diet and chronic diseases. Nutrients (2019) 11(10):1–3. doi: 10.3390/nu11102287
94. Derrien M, Alvarez A, de Vos W. The gut microbiota in the first decade of life. Trends Microbiol (2019) 27(12):997–1010. doi: 10.1016/j.tim.2019.08.001
95. Leeming E, Louca P, Gibson R, Menni C, Spector T, Le Roy C. The complexities of the diet-microbiome relationship: advances and perspectives. Genome Med (2021) 13(1):1–14. doi: 10.1186/s13073-020-00813-7
96. Bellastella G, Scappaticcio L, Caiazzo F, Tomasuolo M, Carotenuto R, Caputo M, et al. Mediterranean Diet and thyroid: an interesting alliance. In: Nutrients. Switzerland: MDPI, vol. 14 (19). (2022). doi: 10.3390/nu14194130
97. Rowland I, Gibson G, Heinken A, Scott K, Swann J, Thiele I, et al. Gut microbiota functions: metabolism of nutrients and other food components. Eur J Nutr (2018) 57(1):1–24. doi: 10.1007/s00394-017-1445-8
98. Feng W, Liu J, Cheng H, Zhang D, Tan Y, Peng C. Dietary compounds in modulation of gut microbiota-derived metabolites. Front Nutr (2022) 9(939571). doi: 10.3389/fnut.2022.939571
99. Trompette A, Pernot J, Perdijk O, Alqahtani R, Domingo J, Camacho-Muñoz D, et al. Gut-derived short-chain fatty acids modulate skin barrier integrity by promoting keratinocyte metabolism and differentiation. Mucosal Immunol (2022) 15(5):908–26. doi: 10.1038/s41385-022-00524-9
100. McKeown N, Fahey G, Slavin J, Van Der Kamp J. Fibre intake for optimal health: how can healthcare professionals support people to reach dietary recommendations? BMJ (2022) 1:1–8. doi: 10.1136/bmj-2020-054370
101. Thursby E, Juge N. Introduction to the human gut microbiota. Biochem J (2017) 474(11):1823–36. doi: 10.1042/BCJ20160510
102. Tuncil Y, Thakkar R, Arioglu-Tuncil S, Hamaker B, Lindemann S. Subtle variations in dietary-fiber fine structure differentially influence the composition and metabolic function of gut microbiota. MSphere (2020) 5(3):1–6. doi: 10.1128/msphere.00180-20
103. Cummings J, Pomare E, Branch H, Naylor C, Macfarlane G. Short chain fatty acids in human large intestine, portal, hepatic and venous blood. Gut (1987) 265–267:1221–7. doi: 10.1136/gut.28.10.1221
104. Ríos-Covián D, Ruas-Madiedo P, Margolles A, Gueimonde M, De los Reyes-Gavilán C, Salazar N. Intestinal short chain fatty acids and their link with diet and human health. Front Microbiol (2016) 7:185. doi: 10.3389/fmicb.2016.00185
105. Pingitore A, Chambers E, Hill T, Maldonado I, Liu B, Morrison D, et al. The diet-derived short chain fatty acid propionate improves beta-cell function in humans and stimulates insulin secretion from human islets in vitro. Diabetes Obes Metab (2017) 19(2):257–65. doi: 10.1111/dom.12811
106. DiGiulio D, Callahan B, McMurdie P, Costello E, Lyell D, Robaczewska A, et al. Temporal and spatial variation of the human microbiota during pregnancy. Proc Natl Acad Sci USA (2015) 112(35):11060–5. doi: 10.1073/pnas.1502875112
107. Smid M, Ricks N, Panzer A, McCoy A, Azcarate-Peril M, Keku T, et al. Maternal gut microbiome biodiversity in pregnancy. Am J Perinatol (2018) 35(1):24–30. doi: 10.1055/s-0037-1604412
108. Younes J, Lievens E, Hummelen R, van der Westen R, Reid G, Petrova M. Women and their microbes: the unexpected friendship. Trends Microbiol (2018) 26(1):16–32. doi: 10.1016/j.tim.2017.07.008
109. Roswall J, Olsson L, Kovatcheva-Datchary P, Nilsson S, Tremaroli V, Simon MC, et al. Developmental trajectory of the healthy human gut microbiota during the first 5 years of life. Cell Host Microbe (2021) 29(5):765–76.e3. doi: 10.1016/j.chom.2021.02.021
110. Tamburini S, Shen N, Wu HC, Clemente J. The microbiome in early life: implications for health outcomes. Nat Med (2016) 22(7):713–22. doi: 10.1038/nm.4142
111. Kim H, Sitarik A, Woodcroft K, Johnson C, Zoratti E. Birth mode, breastfeeding, pet exposure, and antibiotic use: associations with the gut microbiome and sensitization in children. Curr Allergy Asthma Rep (2019) 19(4):17–9. doi: 10.1007/s11882-019-0851-9
112. Penny H, Domingues R, Krauss M, Melo-Gonzalez F, Lawson M, Dickson S, et al. Rhythmicity of intestinal IgA responses confers oscillatory commensal microbiota mutualism. Sci Immunol (2022) 7(75):eabk2541. doi: 10.1126/sciimmunol.abk2541
113. Friedman E, Bittinger K, Esipova T, Hou L, Chau L, Jiang J, et al. Microbes vs. chemistry in the origin of the anaerobic gut lumen. Proc Natl Acad Sci USA (2018) 115(16):4170–5. doi: 10.1073/pnas.1718635115
114. Ruan W, Engevik M, Spinler J, Versalovic J. Healthy human gastrointestinal microbiome: composition and function after a decade of exploration. Digestive Dis Sci (2020) 65(3):695–705. doi: 10.1007/s10620-020-06118-4
115. de Vos W, Tilg H, Van Hul M, Cani P. Gut microbiome and health: mechanistic insights. Gut (2022) 71(5):1020–32. doi: 10.1136/gutjnl-2021-326789
116. Segata N, Haake S, Mannon P, Lemon K, Waldron L, Gevers D, et al. Composition of the adult digestive tract bacterial microbiome based on seven mouth surfaces, tonsils, throat and stool samples. Genome Biol (2012) 13(6):1–18. doi: 10.1186/gb-2012-13-6-r42
117. Sekirov I, Russell S, Antunes C, Finlay B. Gut microbiota in health and disease. Physiol Rev (2010) 90(3):859–904. doi: 10.1152/physrev.00045.2009
118. Mailhe M, Ricaboni D, Vitton V, Gonzalez J, Bachar D, Dubourg G, et al. Repertoire of the gut microbiota from stomach to colon using culturomics and next-generation sequencing. BMC Microbiol (2018) 18(1):1–11. doi: 10.1186/s12866-018-1304-7
119. Kiela PR, Ghishan FK. Physiology of intestinal absorption and secretion. Best Pract Res Clin Gastroenterol (2016) 30(2):145–59. doi: 10.1016/j.bpg.2016.02.007
120. Tuddenham S, Sears C. The intestinal microbiome and health. Curr Opin Infect Disr (2015) 28(5):464–70. doi: 10.1097/QCO.0000000000000196
121. Kastl A, Terry N, Wu G, Albenberg L. The structure and function of the human small intestinal microbiota: current understanding and future directions. Cmgh (2020) 9(1):33–45. doi: 10.1016/j.jcmgh.2019.07.006
122. Villmones H, Haug E, Ulvestad E, Grude N, Stenstad T, Halland A, et al. Species level description of the human ileal bacterial microbiota. Sci Rep (2018) 8(1):1–9. doi: 10.1038/s41598-018-23198-5
123. Ruigrok R, Collij V, Sureda P, Klaassen M, Bolte L, Jansen B, et al. The composition and metabolic potential of the human small intestinal microbiota within the context of inflammatory bowel disease. J Crohn’s Colitis (2021) 15(8):1326–38. doi: 10.1093/ecco-jcc/jjab020
124. Vipperla K, O’Keefe SJ. The microbiota and its metabolites in colonic mucosal health and cancer risk. Nutr Clin Pract (2012) 27(5):624–35. doi: 10.1177/0884533612452012
125. Bäckhed F, Ley R, Sonnenburg J, Peterson D, Gordon J. Host-bacterial mutualism in the human intestine. Science (2005) 307(5717):1915–20. doi: 10.1126/science.1104816
126. Venegas DP, de la Fuente MK, Landskron G, González MJ, Quera R, Dijkstra G, et al. Short chain fatty acids (SCFAs)mediated gut epithelial and immune regulation and its relevance for inflammatory bowel diseases. Front Immunol (2019) 10:277(MAR). doi: 10.3389/fimmu.2019.00277
127. Su X, Yin X, Liu Y, Yan X, Zhang S, Wang X, et al. Gut dysbiosis contributes to the imbalance of treg andTh17 cells in graves’ disease patients by propionic acid. J Clin Endocrinol Metab (2020) 105(11):3526–47. doi: 10.1210/clinem/dgaa511
128. Zhu Q, Hou Q, Huang S, Ou Q, Huo D, Vázquez-Baeza Y, et al. Compositional and genetic alterations in graves’ disease gut microbiome reveal specific diagnostic biomarkers. ISME J (2021) 15(11):3399–411. doi: 10.1038/s41396-021-01016-7
129. Miller TL, Wolin MJ. Pathways of acetate, propionate, and butyrate formation by the human fecal microbial flora. Appl Environ Microbiol (1996) 62(5):1589–92. doi: 10.1128/aem.62.5.1589-1592.1996
130. Duncan S, Barcenilla A, Stewart C, Pryde S, Flint H. Acetate utilization and butyryl coenzyme a (CoA): acetate-CoA transferase in butyrate-producing bacteria from the human large intestine. Appl Environ Microbiol (2002) 68(10):5186–90. doi: 10.1128/AEM.68.10.5186-5190.2002
131. Yamamura R, Nakamura K, Kitada N, Aizawa T, Shimizu Y, Nakamura K, et al. Associations of gut microbiota, dietary intake, and serum short-chain fatty acids with fecal short-chain fatty acids. Biosci Microbiota Food Health (2020) 39(1):11–7. doi: 10.12938/BMFH.19-010
132. Koh A, de Vadder F, Kovatcheva-Datchary P, Bäckhed F. From dietary fiber to host physiology: short-chain fatty acids as key bacterial metabolites. Cell (2016) 165(6):1332–45. doi: 10.1016/j.cell.2016.05.041
133. Louis P, Flint H. Formation of propionate and butyrate by the human colonic microbiota. Environ Microbiol (2017) 19(1):29–41. doi: 10.1111/1462-2920.13589
134. Hosseini E, Grootaert C, Verstraete W, van de Wiele T. Propionate as a health-promoting microbial metabolite in the human gut. Nutr Rev (2011) 69(5):245–58. doi: 10.1111/j.1753-4887.2011.00388.x
135. Eckburg P, Bik E, Bernstein C, Purdom E, Dethlefsen L, Sargent M, et al. Diversity of the human intestinal microbial flora. Science (2005) 10(308):1635–8. doi: 10.1126/science.1110591
136. Gill S, Pop M, DeBoy R, Eckburg P, Turnbaugh P, Samuel B, et al. Metagenomic analysis of the human distal gut microbiome. Science (2006) 312(5778):1355–9. doi: 10.1126/science.1124234
137. Louis P, Flint H. Diversity, metabolism and microbial ecology of butyrate-producing bacteria from the human large intestine. FEMS Microbiol Lett (2009) 294(1):1–8. doi: 10.1111/j.1574-6968.2009.01514.x
138. Hippe B, Zwielehner J, Liszt K, Lassl C, Unger F, Haslberger A. Quantification of butyryl CoA:acetate CoA-transferase genes reveals different butyrate production capacity in individuals according to diet and age. FEMS Microbiol Lett (2011) 316(2):130–5. doi: 10.1111/j.1574-6968.2010.02197.x
139. Fu X, Liu Z, Zhu C, Mou H, Kong Q. Nondigestible carbohydrates, butyrate, and butyrate-producing bacteria. Crit Rev Food Sci Nutr (2019) 59(S1):S130–52. doi: 10.1080/10408398.2018.1542587
140. Binder HJ. Role of colonic short-chain fatty acid transport in diarrhea. Annu Rev Physiol (2009) 72:297–313. doi: 10.1146/annurev-physiol-021909-135817
141. Stumpff F. A look at the smelly side of physiology: transport of short chain fatty acids. Pflugers Archiv Eur J Physiol (2018) 470(4):571–98. doi: 10.1007/s00424-017-2105-9
142. Silva Y, Bernardi A, Frozza R. The role of short-chain fatty acids from gut microbiota in gut-brain communication. Front Endocrinol (2020) 11:25. doi: 10.3389/fendo.2020.00025
143. Sivaprakasam S, Bhutia Y, Yang S, Ganapathy V. Short-chain fatty acid transporters: role in colonic homeostasis. Compr Physiol (2018) 8(1):229–314. doi: 10.1002/cphy.c170014
144. Gill R, Saksena S, Alrefai W, Sarwar Z, Goldstein J, Carroll R, et al. Expression and membrane localization of MCT isoforms along the length of the human intestine. Am J Physiol - Cell Physiol (2005) 289(4 58-4):846–52. doi: 10.1152/ajpcell.00112.2005
145. Ganapathy V, Thangaraju M, Prasad P, Martin P, Singh N. Transporters and receptors for short-chain fatty acids as the molecular link between colonic bacteria and the host. Curr Opin Pharmacol (2013) 13(6):869–74. doi: 10.1016/j.coph.2013.08.006
146. Cuff M, Lambert D, Shirazi-Beechey S. Substrate-induced regulation of the human colonic monocarboxylate transporter, MCT1. J Physiol (2002) 539(2):361–71. doi: 10.1113/jphysiol.2001.014241
147. Borthakur A, Gill R, Hodges K, Ramaswamy K, Hecht G, Dudeja P. Enteropathogenic escherichia coli inhibits butyrate uptake in caco-2 cells by altering the apical membrane MCT1 level. Am J Physiol - Gastrointest Liver Physiol (2006) 290(1):30–5. doi: 10.1152/ajpgi.00302.2005
148. Cuff M, Shirazi-Beechey S. The human monocarboxylate transporter, MCT1: genomic organization and promoter analysis. Biochem Biophys Res Commun (2002) 292(4):1048–56. doi: 10.1006/bbrc.2002.6763
149. Iwanaga T, Takebe K, Kato I, Karaki S, Kuwahara A. Cellular expression of monocarboxylate transporters (MCT) in the digestive tract of the mouse, rat, and humans, with special reference to slc5a8. Biomed Res (2006) 27(5):243–54. doi: 10.2220/biomedres.27.243
150. Mariño E, Richards J, McLeod K, Stanley D, Yap YA, Knight J, et al. Gut microbial metabolites limit the frequency of autoimmune T cells and protect against type 1 diabetes. Nat Immunol (2017) 18(5):552–62. doi: 10.1038/ni.3713
151. Priyadarshini M, Kotlo K, Dudeja P, Layden B. Role of short chain fatty acid receptors in intestinal physiology and pathophysiology. Compr Physiol (2017) 8(3):1091–115. doi: 10.1002/cphy.c170050
152. Sun M, Wu W, Liu Z, Cong Y. Microbiota metabolite short chain fatty acids, GPCR, and inflammatory bowel diseases. J Gastroenterol (2017) 52(1):1–8. doi: 10.1007/s00535-016-1242-9
153. Park B, Kang J, Paudel S, Park S, Park B, Han S, et al. Novel GPR43 agonists exert an anti-inflammatory effect in a colitis model. Biomol Ther (2022) 30(1):48–54. doi: 10.4062/biomolther.2021.078
154. Thangaraju M, Cresci G, Liu K, Ananth S, Gnanaprakasam J, Browning D, et al. GPR109A is a G-protein-coupled receptor for the bacterial fermentation product butyrate and functions as a tumor suppressor in colon. Cancer Res (2009) 69(7):2826–32. doi: 10.1158/0008-5472.CAN-08-4466
155. Nøhr M, Pedersen M, Gille A, Egerod K, Engelstoft M, Husted A, et al. GPR41/FFAR3 and GPR43/FFAR2 as cosensors for short-chain fatty acids in enteroendocrine cells vs FFAR3 in enteric neurons and FFAR2 in enteric leukocytes. Endocrinology (2013) 154(10):3552–64. doi: 10.1210/en.2013-1142
156. Mishra S, Karunakar P, Taraphder S. Free fatty acid receptors 2 and 3 as microbial metabolite sensors to shape host health: pharmacophysiological view. Biomedicines (2017) 8:154. doi: 10.3390/biomedicines8060154
157. Melo-González F, Sepúlveda-Alfaro J, Schultz B, Suazo I, Boone D, Kalergis A, et al. Distal consequences of mucosal infections in intestinal and lung inflammation. Front Immunol (2022) 13:877533. doi: 10.3389/fimmu.2022.877533
158. Brown A, Goldsworthy S, Barnes A, Eilert M, Tcheang L, Daniels D, et al. The orphan G protein-coupled receptors GPR41 and GPR43 are activated by propionate and other short chain carboxylic acids. J Biol Chem (2003) 278(13):11312–9. doi: 10.1074/jbc.M211609200
159. Schlatterer K, Peschel A, Kretschmer D. Short-chain fatty acid and FFAR2 activation – a new option for treating infections? Front Cell Infect Microbiol (2021) 11:785833. doi: 10.3389/fcimb.2021.785833
160. Macia L, Tan J, Vieira A, Leach K, Stanley D, Luong S, et al. Metabolite-sensing receptors GPR43 and GPR109A facilitate dietary fibre-induced gut homeostasis through regulation of the inflammasome. Nat Commun (2015) 6:1–15. doi: 10.1038/ncomms7734
161. Lymperopoulos A, Suster M, Borges J. Short-chain fatty acid receptors and cardiovascular function. Int J Mol Sci (2022) 23(6):1–12. doi: 10.3390/ijms23063303
162. González-Bosch C, Boorman E, Zunszain P, Mann G. Short-chain fatty acids as modulators of redox signaling in health and disease. Redox Biol (2021) 47:1–11. doi: 10.1016/j.redox.2021.102165
163. Kespohl M, Vachharajani N, Luu M, Harb H, Pautz S, Wolff S, et al. The microbial metabolite butyrate induces expression of Th1- associated factors in cD4+ T cells. Front Immunol (2017) 8:1036. doi: 10.3389/fimmu.2017.01036
164. Gaudier E, Jarry A, Blottière H, De Coppet P, Buisine M, Aubert J, et al. Butyrate specifically modulates MUC gene expression in intestinal epithelial goblet cells deprived of glucose. Am J Physiol - Gastrointest Liver Physiol (2004) 287(6 50-6):1168–74. doi: 10.1152/ajpgi.00219.2004
165. Kaiko G, Ryu S, Koues O, Collins P, Solnica-Krezel L, Pearce E, et al. The colonic crypt protects stem cells from microbiota-derived metabolites. Cell (2016) 165(7):1708–20. doi: 10.1016/j.cell.2016.05.018
166. Donohoe D, Garge N, Zhang X, Sun W, O’Connell T, Bunger M, et al. The microbiome and butyrate regulate energy metabolism and autophagy. Cell Metab (2011) 13(5):517–26. doi: 10.1016/j.cmet.2011.02.018
167. Elce A, Amato F, Zarrilli F, Calignano A, Troncone R, Castaldo G, et al. Butyrate modulating effects on pro-inflammatory pathways in human intestinal epithelial cells. Beneficial Microbes (2017) 8(5):841–7. doi: 10.3920/BM2016.0197
168. El Hage R, Hernandez-Sanabria E, Calatayud M, Props R, Van De Wiele T. Propionate-producing consortium restores antibiotic-induced dysbiosis in a dynamic in vitro model of the human intestinal microbial ecosystem. Front Microbiol (2019) 10:1206. doi: 10.3389/fmicb.2019.01206
169. Su X, Zhao Y, Li Y, Ma S, Wang Z. Gut dysbiosis is associated with primary hypothyroidism with interaction on gut-thyroid axis. J Clin Endocrinol Metab (2020) 134(12):1521–35. doi: 10.1042/CS20200475
170. Vélez ML, Costamagna E, Kimura ET, Fozzatti L, Pellizas CG, Montesinos MM, et al. Bacterial lipopolysaccharide stimulates the thyrotropin-dependent thyroglobulin gene expression at the transcriptional level by involving the transcription factors thyroid transcription factor-1 and paired box domain transcription factor 8. Endocrinology (2006) 147(7):3260–75. doi: 10.1210/en.2005-0789
171. Gong B, Wang C, Meng F, Wang H, Song B, Yang Y, et al. Association between gut microbiota and autoimmune thyroid disease: a systematic review and meta-analysis. Front Endocrinol (2021) 12:774362. doi: 10.3389/fendo.2021.774362
172. Nsiah-Sefaa A, McKenzie M. Combined defects in oxidative phosphorylation and fatty acid β-oxidation in mitochondrial disease. Biosci Rep (2016) 36(2):1–19. doi: 10.1042/BSR20150295
173. Talley JT, Mohiuddin SS. Biochemistry, fatty acid oxidation. In: StatPearls. Treasure Island (FL): StatPearls Publishing (2020). Available at: http://www.ncbi.nlm.nih.gov/books/NBK556002/.
174. Sharpe A, McKenzie M. Mitochondrial fatty acid oxidation disorders associated with short-chain enoyl-CoA hydratase (ECHS1) deficiency. Cells (2018) 7(6):1–13. doi: 10.3390/cells7060046
175. Bultman SJ. Butyrate consumption of differentiated colonocytes in the upper crypt promotes homeostatic proliferation of stem and progenitor cells near the crypt base. Trans Cancer Res (2016) 5:S526–8. doi: 10.21037/tcr.2016.08.36
176. Scott N, Andrusaite A, Andersen P, Lawson M, Alcon-Giner C, Leclaire C, et al. Antibiotics induce sustained dysregulation of intestinal T cell immunity by perturbing macrophage homeostasis. Sci Trans Med (2018) 10(464):1–15. doi: 10.1126/scitranslmed.aao4755
177. Hoyles L, Snelling T, Umlai U, Nicholson J, Carding S, Glen R, et al. Microbiome–host systems interactions: protective effects of propionate upon the blood–brain barrier. Microbiome (2018) 6(1):1–14. doi: 10.1186/s40168-018-0439-y
178. Li Z, Yi CX, Katiraei S, Kooijman S, Zhou E, Chung CK, et al. Butyrate reduces appetite and activates brown adipose tissue via the gut-brain neural circuit. Gut (2018) 67(7):1269–79. doi: 10.1136/gutjnl-2017-314050
179. Bielczyk-Maczynska E. White adipocyte plasticity in physiology and disease. Cells (2019) 8(12):1–14. doi: 10.3390/cells8121507
180. Xiong Y, Miyamoto N, Shibata K, Valasek M, Motoike T, Kedzierski R, et al. Short-chain fatty acids stimulate leptin production in adipocytes through the G protein-coupled receptor GPR41. PNAS (2004) 101(4):1045–50. doi: 10.1073/pnas.2637002100
181. Kondo T, Kishi M, Fushimi T, Kaga T. Acetic acid upregulates the expression of genes for fatty acid oxidation enzymes in liver to suppress body fat accumulation. J Agric Food Chem (2009) 57(13):5982–6. doi: 10.1021/jf900470c
182. De Vadder F, Kovatcheva-Datchary P, Goncalves D, Vinera J, Zitoun C, Duchampt A, et al. Microbiota-generated metabolites promote metabolic benefits via gut-brain neural circuits. Cell (2014) 156(1–2):84–96. doi: 10.1016/j.cell.2013.12.016
183. Canfora E, van der Beek C, Jocken J, Goossens G, Holst J, Olde Damink S, et al. Colonic infusions of short-chain fatty acid mixtures promote energy metabolism in overweight/obese men: a randomized crossover trial. Sci Rep (2017) 7(1):1–12. doi: 10.1038/s41598-017-02546-x
184. Arpaia N, Campbell C, Fan X, Dikiy S, van der Veeken J, Deroos P, et al. Metabolites produced by commensal bacteria promote peripheral regulatory T-cell generation. Nature (2013) 504(7480):451–5. doi: 10.1038/nature12726
185. Furusawa Y, Obata Y, Fukuda S, Endo T, Nakato G, Takahashi D, et al. Commensal microbe-derived butyrate induces the differentiation of colonic regulatory T cells. Nature (2013) 504(7480):446–50. doi: 10.1038/nature12721
186. Atarashi K, Tanoue T, Oshima K, Suda W, Nagano Y, Nishikawa H, et al. Treg induction by a rationally selected mixture of clostridia strains from the human microbiota. Nature (2013) 500(7461):232–6. doi: 10.1038/nature12331
187. Smith P, Howitt M, Panikov N, Michaud M, Gallin CA, Bohlooly-Y M, et al. The microbial metabolites, short chain fatty acids, regulate colonic treg cell homeostasis. Science (2013) 341(6145):569–73. doi: 10.1126/science.1241165
188. Schulthess J, Pandey S, Capitani M, Rue-Albrecht K, Arnold I, Franchini F, et al. The short chain fatty acid butyrate imprints an antimicrobial program in macrophages. Immunity (2019) 50(2):432–45.e7. doi: 10.1016/j.immuni.2018.12.018
189. Millard A, Mertes P, Ittelet D, Villard F, Jeannesson P, Bernard J. Butyrate affects differentiation, maturation and function of human monocyte-derived dendritic cells and macrophages. Clin Exp Immunol (2002) 130(2):245–55. doi: 10.1046/j.0009-9104.2002.01977.x
190. Wang B, Morinobu A, Horiuchi M, Liu J, Kumagai S. Butyrate inhibits functional differentiation of human monocyte-derived dendritic cells. Cell Immunol (2008) 253(1–2):54–8. doi: 10.1016/j.cellimm.2008.04.016
191. Kim M, Qie Y, Park J, Kim C. Gut microbial metabolites fuel host antibody responses. Cell Host Microbe (2015) 20(2):202–14. doi: 10.1016/j.chom.2016.07.001
192. Park J, Kim M, Kang S, Jannasch A, Bruce Cooper J, Kim C. Short chain fatty acids induce both effector and regulatory T cells by suppression of histone deacetylases and regulation of the mTOR-S6K pathway. Mucosal Immunol (2015) 8(1):80–93. doi: 10.1038/mi.2014.44
193. Park J, Goergen C, HogenEsch H, Kim C. Chronically elevated levels of short-chain fatty acids induce T cell-mediated ureteritis and hydronephrosis. J Immunol (2016) 196(5):2388–400. doi: 10.4049/jimmunol.1502046
194. Singh N, Gurav A, Sivaprakasam S, Brady E, Padia R, Shi H, et al. Activation of Gpr109a, receptor for niacin and the commensal metabolite butyrate, suppresses colonic inflammation and carcinogenesis. Immunity (2014) 40(1):128–39. doi: 10.1016/j.immuni.2013.12.007
195. Chang PV, Hao L, Offermanns S, Medzhitov R. The microbial metabolite butyrate regulates intestinal macrophage function via histone deacetylase inhibition. Proc Natl Acad Sci USA (2014) 111(6):2247–52. doi: 10.1073/pnas.1322269111
196. Föh B, Buhre J, Lunding H, Moreno-Fernandez M, König P, Sina C, et al. Microbial metabolite butyrate promotes induction of IL-10+IgM+ plasma cells. PloS One (2022) 17(3 March):1–25. doi: 10.1371/journal.pone.0266071
197. Sanchez H, Moroney J, Gan H, Shen T, Im J, Li T, et al. B cell-intrinsic epigenetic modulation of antibody responses by dietary fiber-derived short-chain fatty acids. Nat Commun (2020) 11(1):1–19. doi: 10.1038/s41467-019-13603-6
198. Belizário JE, Napolitano M. Human microbiomes and their roles in dysbiosis, common diseases, and novel therapeutic approaches. Front Microbiol (2015) 6:1050(OCT). doi: 10.3389/fmicb.2015.01050
199. Levy M, Kolodziejczyk AA, Thaiss CA, Elinav E. Dysbiosis and the immune system. Nat Rev Immunol (2017) 17(4):219–32. doi: 10.1038/nri.2017.7
200. Koliada A, Syzenko G, Moseiko V, Budovska L, Puchkov K, Perederiy V, et al. Association between body mass index and Firmicutes/Bacteroidetes ratio in an adult Ukrainian population. BMC Microbiol (2017) 17(1):4–9. doi: 10.1186/s12866-017-1027-1
201. Stojanov S, Berlec A, Štrukelj B. The influence of probiotics on the firmicutes/bacteroidetes ratio in the treatment of obesity and inflammatory bowel disease. Microorganisms (2020) 8(11):1–16. doi: 10.3390/microorganisms8111715
202. Fredericks E, Theunissen R, Roux S. Short chain fatty acids and monocarboxylate transporters in irritable bowel syndrome. Turkish J Gastroenterol (2020) 31(12):840–7. doi: 10.5152/TJG.2020.19856
203. Yao Z, Zhao M, Gong Y, Chen W, Wang Q, Fu Y, et al. Relation of gut microbes and l-thyroxine through altered thyroxine metabolism in subclinical hypothyroidism subjects. Front Cell Infect Microbiol (2020) 10:495. doi: 10.3389/fcimb.2020.00495
204. Jackson M, Verdi S, Maxan M, Shin C, Zierer J, Bowyer R, et al. Gut microbiota associations with common diseases and prescription medications in a population-based cohort. Nat Commun (2018) 9(1):1–8. doi: 10.1038/s41467-018-05184-7
205. Dong T, Zhao F, Yuan K, Zhu X, Wang N, Xia F, et al. Association between serum thyroid-stimulating hormone levels and salivary microbiome shifts. Front Cell Infect Microbiol (2021) 11:603291. doi: 10.3389/fcimb.2021.603291
206. Yau W, Singh B, Lesmana R, Zhou J, Sinha R, Wong K, et al. Thyroid hormone (T3) stimulates brown adipose tissue activation via mitochondrial biogenesis and MTOR-mediated mitophagy. Autophagy (2019) 15(1):131–50. doi: 10.1080/15548627.2018.1511263
207. Yau W, Yen P. Thermogenesis in adipose tissue activated by thyroid hormone. Int J Mol Sci (2020) 21(8):1–16. doi: 10.3390/ijms21083020
208. Sinha R, Singh B, Yen P. Direct effects of thyroid hormones on hepatic lipid metabolism. Nat Rev Endocrinol (2018) 14(5):259–69. doi: 10.1038/nrendo.2018.10
209. Du F, Kuang H, Duan B, Liu D, Yu X. Effects of thyroid hormone and depression on common components of central obesity. J Int Med Res (2019) 47(7):3040–9. doi: 10.1177/0300060519851624
210. Khatiwada S, Sah S, Rajendra K, Baral N, Lamsal N. Thyroid dysfunction in metabolic syndrome patients and its relationship with components of metabolic syndrome. Clin Diabetes Endocrinol (2016) 2(1):1–5. doi: 10.1186/s40842-016-0021-0
211. Di Domenico M, Pinto F, Quagliuolo L, Contaldo M, Settembre G, Romano A, et al. The role of oxidative stress and hormones in controlling obesity. Front Endocrinol (2019) 10:540. doi: 10.3389/fendo.2019.00540
212. Mahdavi M, Amouzegar A, Mehran L, Madreseh E, Tohidi M, Azizi F. Investigating the prevalence of primary thyroid dysfunction in obese and overweight individuals: Tehran thyroid study. BMC Endocr Disord (2021) 21(1):1–11. doi: 10.1186/s12902-021-00743-4
213. Jin F, Zhang J, Shu L, Han W. Association of dietary fiber intake with newly-diagnosed type 2 diabetes mellitus in middle-aged Chinese population. Nutr J (2021) 20(1):1–8. doi: 10.1186/s12937-021-00740-2
214. Beretta MV, Bernaud FR, Nascimento C, Steemburgo T, Rodrigues TC. Higher fiber intake is associated with lower blood pressure levels in patients with type 1 diabetes. Arch Endocrinol Metab (2018) 62(1):40–7. doi: 10.20945/2359-3997000000008
215. Sanna S, van Zuydam NR, Mahajan A, Kurilshikov A, Vich Vila A, Võsa U, et al. Causal relationships among the gut microbiome, short-chain fatty acids and metabolic diseases. Nat Genet (2019) 51(4):600–5. doi: 10.1038/s41588-019-0350-x
216. CDC. Prevalence of obesity and severe obesity among adults: united states 2017-2018. NCHS (2020) 360:1–8.
217. Moreno-Navarrete JM, Fernandez-Real JM. The gut microbiota modulates both browning of white adipose tissue and the activity of brown adipose tissue. Rev Endocrine Metab Disord (2019) 20(4):387–97. doi: 10.1007/s11154-019-09523-x
218. Al-Lahham S, Roelofsen H, Priebe M, Weening D, Dijkstra M, Hoek A, et al. Regulation of adipokine production in human adipose tissue by propionic acid. Eur J Clin Invest (2010) 40(5):401–7. doi: 10.1111/j.1365-2362.2010.02278.x
219. Walczak K, Sieminska L. Obesity and thyroid axis. Int J Environ Res Public Health (2021) 18(18):1–23. doi: 10.3390/ijerph18189434
220. Fröhlich E, Wahl R. Microbiota and thyroid interaction in health and disease. Trends Endocrinol Metab (2019) 30(8):479–90. doi: 10.1016/j.tem.2019.05.008
221. ADA. American Diabetes association. 5. lifestyle management: standards of medical care in diabetes-2019. Diabetes Care (2019) 42(1):S46–60. doi: 10.2337/dc19-S005
222. DGFA. Dietary guidelines for americans 2015-2020. dietary guidelines for americans Vol. 47. Washington, D.C, US: U.S. Department of Health and Human Services and U.S. Department of Agriculture. (2015) p. 245–51. doi: 10.1097/NT.0b013e31826c50af
223. Yin XQ, An YX, Yu CG, Ke J, Zhao D, Yu K. The association between fecal short-chain fatty acids, gut microbiota, and visceral fat in monozygotic twin pairs. Diabetes Metab Syndr Obesity: Targets Ther (2022) 15:359–68. doi: 10.2147/DMSO.S338113
224. Jiang W, Yu X, Kosik R, Song Y, Qiao T, Tong J, et al. Gut microbiota may play a significant role in the pathogenesis of graves’ disease. Thyroid (2021) 31(5):810–20. doi: 10.1089/thy.2020.0193
225. Saluja M, Pyarsabadi P, Jelia S, Chittora S, Swami Y, Vimlani H. Study of thyroid dysfunction in metabolic syndrome and association with its components. Curr Med Res Pract (2018) 8(1):3–7. doi: 10.1016/j.cmrp.2017.11.010
226. Kim K, Yao Y, Ju S. Short chain fatty acids and fecal microbiota abundance in humans with obesity: a systematic review and meta-analysis. Nutrients (2019) 11(10):1–14. doi: 10.3390/nu11102512
227. Brechmann T, Sperlbaum A, Schmiegel W. Levothyroxine therapy and impaired clearance are the strongest contributors to small intestinal bacterial overgrowth: results of a retrospective cohort study. World J Gastroenterol (2017) 23(5):842–52. doi: 10.3748/wjg.v23.i5.842
228. Wang B, Xu Y, Hou X, Li J, Cai Y, Hao Y, et al. Small intestinal bacterial overgrowth in subclinical hypothyroidism of pregnant women. Front Endocrinol (2021) 12:604070. doi: 10.3389/fendo.2021.604070
229. Lauritano B, Gabrielli M, Scarpellini E, Lupascu A, Laginestra A, Novi M, et al. Association between hypothyroidism and small intestinal bacterial overgrowth. J Clin Endocrinol Metab (2007) 92(11):4180–4. doi: 10.1210/jc.2007-0606
230. Patil A. Link between hypothyroidism and small intestinal bacterial overgrowth. Indian J Endocrinol Metab (2014) 18(3):307–9. doi: 10.4103/2230-8210.131155
231. Jiang W, Lu G, Gao D, Lv Z, Li D. The relationships between the gut microbiota and its metabolites with thyroid diseases. Front Endocrinol (2022) 13:943408. doi: 10.3389/fendo.2022.943408
232. Janett-Pellegri C, Wildisen L, Feller M, Del Giovane C, Moutzouri E, Grolimund O, et al. Prevalence and factors associated with chronic use of levothyroxine: a cohort study. PloS One (2021) 16:1–14. doi: 10.1371/journal.pone.0261160
233. Virili C, Antonelli A, Santaguida MG, Benvenga S, Centanni M. Gastrointestinal malabsorption of thyroxine. Endocrine Rev (2018) 40(1):118–36. doi: 10.1210/er.2018-00168
234. Cai Y, Xu Y, Ban Y, Li J, Sun Z, Zhang M, et al. Plasma lipid profile and intestinal microflora in pregnancy women with hypothyroidism and their correlation with pregnancy outcomes. Front Endocrinol (2022) 12:792536(January). doi: 10.3389/fendo.2021.792536
235. Wang B, Xu Y, Zhang M, Zhang J, Hou X, Li J, et al. Oral and intestinal microbial features in pregnant women with hypothyroidism and their correlations with pregnancy outcomes. Am J Physiol Endocrinol Metab (2020) 319(6):E1044–52. doi: 10.1152/AJPENDO.00234.2020
236. Wu M, Chi C, Yang Y, Guo S, Li T, Gu M, et al. Dynamics of gut microbiota during pregnancy in women with TPOAb-positive subclinical hypothyroidism: a prospective cohort study. BMC Pregnancy Childbirth (2022) 22(1):1–14. doi: 10.1186/s12884-022-04923-5
237. Granado-Serrano A, Martín-Garí M, Sánchez V, Riart Solans M, Berdún R, Ludwig I, et al. Faecal bacterial and short-chain fatty acids signature in hypercholesterolemia. Sci Rep (2019) 9(1):1–13. doi: 10.1038/s41598-019-38874-3
238. Gaibani P, D’Amico F, Bartoletti M, Lombardo D, Rampelli S, Fornaro G, et al. The gut microbiota of critically ill patients with COVID-19. Front Cell Infect Microbiol (2021) 11:670424. doi: 10.3389/fcimb.2021.670424
239. Macy J, Ljungdahl L, Gottschalk G. Pathway of succinate and propionate formation in bacteroides fragilis. J Bacteriol (1978) 134(1):84–91. doi: 10.1128/jb.134.1.84-91.1978
240. Chen J, Wang R, Li X, Wang R. Bifidobacterium longum supplementation improved high-fat-fed-induced metabolic syndrome and promoted intestinal reg I gene expression. Exp Biol Med (2011) 236(7):823–31. doi: 10.1258/ebm.2011.010399
241. Wu G, Chen J, Hoffmann C, Bittinger K, Chen Y, Keilbaugh S, et al. Linking long-term dietary patterns with gut microbial enterotypes. Science (2011) 334(6052):105–8. doi: 10.1126/science.1208344
242. Park S, Kim M, Bae J. Blautia faecis sp. nov., isolated from human faeces. Int J Syst Evol Microbiol (2013) 63:599–603. doi: 10.1099/ijs.0.036541-0
243. Kovatcheva-Datchary P, Nilsson A, Akrami R, Lee YS, De Vadder F, Arora T, et al. Dietary fiber-induced improvement in glucose metabolism is associated with increased abundance of prevotella. Cell Metab (2015) 22(6):971–82. doi: 10.1016/j.cmet.2015.10.001
244. Chen T, Long W, Zhang C, Liu S, Zhao L, Bruce R. Fiber-utilizing capacity varies in prevotella- versus bacteroides- dominated gut microbiota. Sci Rep (2017) 7(2594):1–7. doi: 10.1038/s41598-017-02995-4
245. Leite A, Rodrigues N, Gonzaga M, Paiolo J, de Souza C, Stefanutto N, et al. Detection of increased plasma interleukin-6 levels and prevalence of prevotella copri and bacteroides vulgatus in the feces of type 2 diabetes patients. Front Immunol (2017) 8:1107. doi: 10.3389/fimmu.2017.01107
246. Leiva-Gea I, Sánchez-Alcoholado L, Martín-Tejedor B, Castellano-Castillo D, Moreno-Indias I, Urda-Cardona A, et al. Gut microbiota differs in composition and functionality between children with type 1 diabetes and MODY2 and healthy control subjects: a case-control study. Diabetes Care (2018) 41(11):2385–95. doi: 10.2337/dc18-0253
247. Liu X, Mao B, Gu J, Wu J, Cui S, Wang G, et al. Blautia–a new functional genus with potential probiotic properties? In: Gut microbes, vol. Vol. 13. China: Taylor & Francis (2021). p. 1–21. doi: 10.1080/19490976.2021.1875796
248. Kircher B, Woltemate S, Gutzki F, Schlüter D, Geffers R, Bähre H, et al. Predicting butyrate- and propionate-forming bacteria of gut microbiota from sequencing data. Gut Microbes (2022) 14(1):1–37. doi: 10.1080/19490976.2022.2149019
249. Michail S, Lin M, Frey M, Fanter R, Paliy O, Hilbush B, et al. Altered gut microbial energy and metabolism in children with non-alcoholic fatty liver disease. FEMS Microbiol Ecol (2015) 91(2):1–9. doi: 10.1093/femsec/fiu002
250. de Andrade P, Giovani P, Araujo D, de Souza A, Pedroni-Pereira A, Kantovitz K, et al. Shifts in the bacterial community of saliva give insights on the relationship between obesity and oral microbiota in adolescents. Arch Microbiol (2020) 202(5):1085–95. doi: 10.1007/s00203-020-01817-y
251. Mirzaei R, Afaghi A, Babakhani S, Sohrabi M, Hosseini-Fard S, Babolhavaeji K, et al. Role of microbiota-derived short-chain fatty acids in cancer development and prevention. Biomed Pharmacother (2021) 139:111619. doi: 10.1016/j.biopha.2021.111619
252. Park T, Im J, Kim A, Lee D, Jeong S, Yun C, et al. Short-chain fatty acids inhibit the biofilm formation of streptococcus gordonii through negative regulation of competence-stimulating peptide signaling pathway. J Microbiol (2021) 59:1142–9. doi: 10.1007/s12275-021-1576-8
253. Larsen JM. The immune response to prevotella bacteria in chronic inflammatory disease. Immunology (2017) 151(4):363–74. doi: 10.1111/imm.12760
254. de la Cuesta-Zuluaga J, Mueller N, Álvarez-Quintero R, Velásquez-Mejía E, Sierra J, Corrales-Agudelo V, et al. Higher fecal short-chain fatty acid levels are associated with gut microbiome dysbiosis, obesity, hypertension and cardiometabolic disease risk factors. Nutrients (2019) 11(1):1–16. doi: 10.3390/nu11010051
255. Ishaq H, Mohammad I, Guo H, Shahzad M, Hou Y, Ma C, et al. Molecular estimation of alteration in intestinal microbial composition in hashimoto’s thyroiditis patients. Biomed Pharmacother (2017) 95:865–74. doi: 10.1016/j.biopha.2017.08.101
256. Zhao F, Feng J, Li J, Zhao L, Liu Y, Chen H, et al. Alterations of the gut microbiota in hashimoto’s thyroiditis patients. Thyroid (2018) 28(2):175–86. doi: 10.1089/thy.2017.0395
257. Liu S, An Y, Cao B, Sun R, Ke J, Zhao D. The composition of gut microbiota in patients bearing hashimoto’s thyroiditis with euthyroidism and hypothyroidism. Int J Endocrinol (2020) 2020:1–9. doi: 10.1155/2020/5036959
258. Liu J, Qin X, Lin B, Cui J, Liao J, Zhang F, et al. Analysis of gut microbiota diversity in hashimoto’s thyroiditis patients. BMC Microbiol (2022) 22(1):318. doi: 10.1186/s12866-022-02739-z
259. Wang J, Qin J, Li Y, Cai Z, Li S, Zhu J, et al. A metagenome-wide association study of gut microbiota in type 2 diabetes. Nature (2012) 490(7418):55–60. doi: 10.1038/nature11450
260. Wu F, Guo X, Zhang J, Zhang M, Ou Z, Peng Y. Phascolarctobacterium faecium abundant colonization in human gastrointestinal tract. Exp Ther Med (2017) 14:3122–6. doi: 10.3892/etm.2017.4878
261. He Y, Wu W, Wu S, Zheng H, Li P, Sheng H, et al. Linking gut microbiota, metabolic syndrome and economic status based on a population-level analysis. Microbiome (2018) 6(172):1–11. doi: 10.1186/s40168-018-0557-6
262. Hul M, Le T, Prifti E, Carlota M, Paquot A. From correlation to causality : the case of subdoligranulum. GUT Microbes (2020) 12(1):1–13. doi: 10.1080/19490976.2020.1849998
263. Tsubokawa M, Nishimura M, Mikami T, Ishida M, Hisada T, Tamada Y. Association of gut microbial genera with heart rate variability in the general Japanese population: the iwaki cross-sectional research study. Metabolites (2022) 12(8):1–11. doi: 10.3390/metabo12080730
264. Derrien M, Vaughan E, Plugge C, de Vos W. Akkermansia municiphila gen. nov., sp. nov., a human intestinal mucin-degrading bacterium. Int J Syst Evol Microbiol (2004) 54(5):1469–76. doi: 10.1099/ijs.0.02873-0
265. Li W, Li L, Yang F, Hu Q, Xiong D. Correlation between gut bacteria phascolarctobacterium and exogenous metabolite α-linolenic acid in T2DM: a case-control study. Ann Transl Med (2022) 10(19):0–3. doi: 10.21037/atm-22-3967
266. Yatsunenko T, Rey F, Manary M, Trehan I, Dominguez-Bello M, Contreras M, et al. Human gut microbiome viewed across age and geography. Nature (2012) 486(7402):222–7. doi: 10.1038/nature11053
267. Wutthi-in M, Cheevadhanarak S, Yasom S, Kerdphoo S, Thiennimitr P, Phrommintikul A, et al. Gut microbiota profiles of treated metabolic syndrome patients and their relationship with metabolic health. Sci Rep (2020) 10(1):1–11. doi: 10.1038/s41598-020-67078-3
268. Zhang Q, Hu W, Deng Y, Wan J, Wang Y, Jin P. Dysbiosis of gut microbiota and decreased propionic acid associated with metabolic abnormality in cushing’s syndrome. Front Endocrinol (2023) 13:1095438. doi: 10.3389/fendo.2022.1095438
269. Murri M, Leiva I, Gomez-Zumaquero J, Tinahones F, Cardona F, Soriguer F, et al. Gut microbiota in children with type 1 diabetes differs from that in healthy children: a case-control study. BMC Med (2013) 11(1):46. doi: 10.1186/1741-7015-11-46
270. Vatanen T, Franzosa E, Schwager R, Tripathi S, Arthur T, Vehik K, et al. The human gut microbiome in early-onset type 1 diabetes from the TEDDY study. Nature (2018) 562(7728):589–94. doi: 10.1038/s41586-018-0620-2
271. Markowiak-Kopec P, Slizewska K. The effect of probiotics on the production of short-chain fatty acids by human intestinal microbiome. Nutrients (2020) 12(1107):1–23. doi: 10.3390/nu12041107
272. Ishaq H, Mohammad I, Shahzad M, Ma C, Raza M, Wu X, et al. Molecular alteration analysis of human gut microbial composition in graves’ disease patients. Int J Biol Sci (2018) 14(11):1558–70. doi: 10.7150/ijbs.24151
273. Zheng D, Liao H, Chen S, Liu X, Mao C, Zhang C, et al. Elevated levels of circulating biomarkers related to leaky gut syndrome and bacterial translocation are associated with graves’ disease. Front Endocrinol (2021) 12:796212. doi: 10.3389/fendo.2021.796212
274. Han Z, Cen C, Ou Q, Pan Y, Zhang J, Huo D, et al. The potential prebiotic berberine combined with methimazole improved the therapeutic effect of graves’ disease patients through regulating the intestinal microbiome. Front Immunol (2022) 12:826067. doi: 10.3389/fimmu.2021.826067
275. Mallick H, Franzosa E, Mclver L, Banerjee S, Sirota-Madi A, Kostic A, et al. Predictive metabolomic profiling of microbial communities using amplicon or metagenomic sequences. Nat Commun (2019) 10(1):1–11. doi: 10.1038/s41467-019-10927-1
276. Cornejo-Pareja I, Ruiz-Limón P, Gómez-Pérez A, Molina-Vega M, Moreno-Indias I, Tinahones F. Differential microbial pattern description in subjects with autoimmune-based thyroid diseases: a pilot study. J Pers Med (2020) 10(192):1–13. doi: 10.3390/jpm10040192
277. Zhou L, Li X, Ahmed A, Wu D, Liu L, Qiu J, et al. Gut microbe analysis between hyperthyroid and healthy individuals. Curr Microbiol (2014) 69(5):675–80. doi: 10.1007/s00284-014-0640-6
278. Borthakur A, Anbazhagan AN, Kumar A, Raheja G, Singh V, Ramaswamy K, et al. The probiotic lactobacillus plantarum counteracts TNF-α-induced downregulation of SMCT1 expression and function. Am J Physiol - Gastrointestinal Liver Physiol (2010) 299(4):1–18. doi: 10.1152/ajpgi.00279.2010
279. Duncan S, Hold G, Harmsen H, Stewart C, Flint H. Growth requirements and fermentation products of fusobacterium prausnitzii, and a proposal to reclassify it as faecalibacterium prausnitzii gen. nov., comb. nov. Int J Syst Evol Microbiol (2002) 52(6):2141–6. doi: 10.1099/00207713-52-6-2141
280. Xu Z, Jiang W, Huang W, Lin Y, Chan F, Ng S. Gut microbiota in patients with obesity and metabolic disorders[[/amp]]mdash; a systematic review. Genes Nutr (2022) 17(1):1–18. doi: 10.1186/s12263-021-00703-6
281. Schwiertz A, Taras D, Schäfer K, Beijer S, Bos N, Donus C, et al. Microbiota and SCFA in lean and overweight healthy subjects. Obesity (2009) 18(1):190–5. doi: 10.1038/oby.2009.167
282. Vital M, Howe A, Tiedje J. Revealing the bacterial butyrate synthesis pathways by analyzing (meta)genomic data. MBio (2014) 5(2):1–11. doi: 10.1128/mBio.00889-14
283. Higuchi B, Rodrigues N, Gonzaga M, Paiolo J, Stefanutto N, Omori W, et al. Intestinal dysbiosis in autoimmune diabetes is correlated with poor glycemic control and increased interleukin-6: a pilot study. Front Immunol (2018) 9:1689. doi: 10.3389/fimmu.2018.01689
284. Chen Z, Radjabzadeh D, Chen L, Kurilshikov A, Kavousi M, Ahmadizar F, et al. Association of insulin resistance and type 2 diabetes with gut microbial diversity a microbiome-wide analysis from population studies. JAMA Network Open (2021) 4(7):1–13. doi: 10.1001/jamanetworkopen.2021.18811
285. Louis P, Young P, Holtrop G, Flint H. Diversity of human colonic butyrate-producing bacteria revealed by analysis of the butyryl-CoA:acetate CoA-transferase gene. Environ Microbiol (2010) 12(2):304–14. doi: 10.1111/j.1462-2920.2009.02066.x
286. Shimizu J, Kubota T, Takada E, Takai K, Fujiwara N, Arimitsu N, et al. Propionate-producing bacteria in the intestine may associate with skewed responses of IL10-producing regulatory T cells in patients with relapsing polychondritis. PloS One (2018) 13(9):1–17. doi: 10.1371/journal.pone.0203657
287. Qian X, Si Q, Lin G, Zhu M, Lu J, Zhang H, et al. Bifidobacterium adolescentis is effective in relieving type 2 diabetes and may be related to its dominant core genome and gut microbiota modulation capacity. Nutrients (2022) 14(12):1–18. doi: 10.3390/nu14122479
288. Gonzalez-Garcia R, McCubbin T, Navone L, Stowers C, Nielsen L, Marcellin E. Microbial propionic acid production. Fermentation (2017) 3(2):1–20. doi: 10.3390/fermentation3020021
289. Kunc M, Gabrych A, Witkowski JM. Microbiome impact on metabolism and function of sex, thyroid, growth and parathyroid hormones. Acta Biochim Polonica (2016) 63(2):189–201. doi: 10.18388/abp.2015_1093
290. Trefely S, Lovell C, Snyder N, Wellen K. Compartmentalised acyl-CoA metabolism and roles in chromatin regulation. Mol Metab (2020) 38:1–18. doi: 10.1016/j.molmet.2020.01.005
291. Garcia-Villalba P, Jimenez-Lara AM, Castillo AI, Aranda A. Histone acetylation influences thyroid hormone and retinoic acid-mediated gene expression. In: DNA AND CELL BIOLOGY, vol. Vol. 16. Spain: Mary Ann Liebert, Inc (1997).
292. Samuels H, Stanley F, Casanova J, Shao T. Thyroid hormone nuclear receptor levels are influenced by the acetylation of chromatin-associated proteins*. J Biol Chem (1980) 255(6):2499–508. doi: 10.1016/S0021-9258(19)85921-5
293. Ortiz-Caro J, Montiel F, Pascual A, Aranda A. Modulation of thyroid hormone nuclear receptors by short-chain fatty acids in glial C6 cells ROLE OF HISTONE ACETYLATION*. J Biol Chem (1986) 261(30):13997–4004. doi: 10.1016/S0021-9258(18)66971-6
294. Mitsuhashis T, Uchimura H, Takaku F. THE JOURNAL OF BIOLOGICAL CHEMISTRY n-butyrate increases the level of thyroid hormone nuclear receptor in non-pituitary cultured cells. J Biol Chem (1987) 262:3993–9. doi: 10.1016/S0021-9258(18)61301-8
295. Nishii Y, Ichikawa K, Miyamoto T, Takeda T, Kobayashi M, Suzuki S, et al. N-butyrate responsive enhances induction nuclear protein of thyroid hormone. Endocrine J (1993) 40(5):515–21. doi: 10.1507/endocrj.40.515
296. Vanderpump MPJ. The epidemiology of thyroid disease. Br Med Bull (2011) 99(1):39–51. doi: 10.1093/bmb/ldr030
297. Ihnatowicz P, Drywien M, Wator P, Wojsiat J. The importance of nutritional factors and dietary management of hashimoto’s thyroiditis. In: Annals of agricultural and environmental medicine, vol. Vol. 27. Poland: Institute of Agricultural Medicine (2020). p. 184–93. doi: 10.26444/aaem/112331
298. Gratton J, Phetcharaburanin J, Mullish B, Williams H, Thursz M, Nicholson J, et al. Optimized sample handling strategy for metabolic profiling of human feces. Anal Chem (2016) 88(9):4661–8. doi: 10.1021/acs.analchem.5b04159
299. Park N, Kim M, Lee W, Lee M, Hong J. An in situ extraction and derivatization method for rapid analysis of short-chain fatty acids in rat fecal samples by gas chromatography tandem mass spectrometry. Anal Methods (2017) 9(15):2351–6. doi: 10.1039/c7ay00168a
300. Primec M, Mičetić-Turk D, Langerholc T. Analysis of short-chain fatty acids in human feces: a scoping review. Anal Biochem (2017) 526:9–21. doi: 10.1016/j.ab.2017.03.007
301. Ribeiro W, Vinolo M, Calixto L, Ferreira C. Use of gas chromatography to quantify short chain fatty acids in the serum, colonic luminal content and feces of mice. Bio-Protocol (2018) 8(22):1–11. doi: 10.21769/bioprotoc.3089
302. Song H, Lee H, Kim S, Back S, Yoo H. A facile profiling method of short chain fatty acids using liquid chromatography-mass spectrometry. Metabolites (2019) 9(9):1–11. doi: 10.3390/metabo9090173
303. Kim K, Lee Y, Chae W, Cho J. An improved method to quantify short-chain fatty acids in biological samples using gas chromatography–mass spectrometry. Metabolites (2022) 12(6):4–15. doi: 10.3390/metabo12060525
304. Wang H, Wang C, Guo L, Zheng Y, Hu WH, Dong T, et al. Simultaneous determination of short-chain fatty acids in human feces by HPLC with ultraviolet detection following chemical derivatization and solid-phase extraction segmental elution. J Separation Sci (2019) 42(15):2500–9. doi: 10.1002/jssc.201900249
305. Zhang S, Wang H, Zhu MJ. A sensitive GC/MS detection method for analyzing microbial metabolites short chain fatty acids in fecal and serum samples. Talanta (2019) 196(December 2018):249–54. doi: 10.1016/j.talanta.2018.12.049
306. Hauser A, Eisenmann P, Muhle-Goll C, Luy B, Dötsch A, Graf D, et al. Efficient extraction from mice feces for NMR metabolomics measurements with special emphasis on SCFAs. Metabolites (2019) 9(3):1–19. doi: 10.3390/metabo9030055
307. Lemay J, Yamamoto M, Kroezen Z, Shanmuganathan M, Ly R, Hart L, et al. Lyophilized fecal short-chain fatty acid and electrolyte determination by capillary electrophoresis with indirect UV detection for assessment of pediatric inflammatory bowel disease. J Pharm Biomed Anal (2021) 192:113658. doi: 10.1016/j.jpba.2020.113658
308. Kim H, Kwon J, Choi S, Ahn Y. Method development for the quantitative determination of short chain fatty acids in microbial samples by solid phase extraction and gas chromatography with flame ionization detection. J Anal Sci Technol (2019) 10(1):2–7. doi: 10.1186/s40543-019-0184-2
309. Scortichini S, Boarelli M, Silvi S, Fiorini D. Development and validation of a GC-FID method for the analysis of short chain fatty acids in rat and human faeces and in fermentation fluids. J Chromatogr B: Anal Technol Biomed Life Sci (2020) 1143(September 2019):121972. doi: 10.1016/j.jchromb.2020.121972
310. Furuhashi T, Sugitate K, Nakai T, Jikumaru Y, Ishihara G. Rapid profiling method for mammalian feces short chain fatty acids by GC-MS. Anal Biochem (2018) 543:51–4. doi: 10.1016/j.ab.2017.12.001
311. Rahman M, Diantini A, Fattah M, Barliana M, Wijaya A. A highly sensitive, simple, and fast gas chromatography–mass spectrometry method for the quantification of serum short-chain fatty acids and their potential features in central obesity. Anal Bioanal Chem (2021) 413(27):6837–44. doi: 10.1007/s00216-021-03639-3
312. Fu Z, Jia Q, Zhang H, Kang L, Sun X, Zhang M, et al. Simultaneous quantification of eleven short-chain fatty acids by derivatization and solid phase microextraction - gas chromatography tandem mass spectrometry. J Chromatogr A (2022) 1661:462680. doi: 10.1016/j.chroma.2021.462680
313. Inoue H, Takayama K, Takahara C, Tabuchi N, Okamura N, Narahara N, et al. Determination of short-chain fatty acids in mouse feces by highperformance liquid chromatography using 2-nitrophenylhydrazine as a labeling reagent. Biol Pharm Bull (2019) 42(5):845–9. doi: 10.1248/bpb.b18-01017
314. Kotani A, Miyaguchi Y, Kohama M, Ohtsuka T, Shiratori T, Kusu F. Determination of short-chain fatty acids in rat and human feces by high-performance liquid chromatography with electrochemical detection. Anal Sci (2009) 25(8):1007–11. doi: 10.2116/analsci.25.1007
315. Liebisch G, Ecker J, Roth S, Schweizer S, Öttl V, Schött HF, et al. Quantification of fecal short chain fatty acids by liquid chromatography tandem mass spectrometry–investigation of pre-analytic stability. Biomolecules (2019) 9(4):1–13. doi: 10.3390/biom9040121
316. Fu H, Zhang Q, Huang X, Ma Z, Zheng X, Li S, et al. A rapid and convenient derivatization method for quantitation of short-chain fatty acids in human feces by ultra-performance liquid chromatography/tandem mass spectrometry. Rapid Commun Mass Spectrometry (2020) 34(9):1–14. doi: 10.1002/rcm.8730
317. Cuervo A, Salazar N, Ruas-Madiedo P, Gueimonde M, González S. Fiber from a regular diet is directly associated with fecal short-chain fatty acid concentrations in the elderly. Nutr Res (2013) 33(10):811–6. doi: 10.1016/j.nutres.2013.05.016
318. Salazar N, Dewulf E, Neyrinck A, Bindels L, Cani P, Mahillon J, et al. Inulin-type fructans modulate intestinal bifidobacterium species populations and decrease fecal short-chain fatty acids in obese women. Clin Nutr (2015) 34(3):501–7. doi: 10.1016/j.clnu.2014.06.001
319. Wildt S, Nordgaard-Lassen I, Bendtsen F, Rumessen J. Metabolic and inflammatory faecal markers in collagenous colitis. Eur J Gastroenterol Hepatol (2007) 19(7):567–74. doi: 10.1097/MEG.0b013e328058ed76
320. Worthley D, Le Leu R, Whitehall V, Conlon M, Christophersen C, Belobrajdic D, et al. A human, double-blind, placebo-controlled, crossover trial of prebiotic, probiotic, and synbiotic supplementation: effects on luminal, inflammatory, epigenetic, and epithelial biomarkers of colorectal cancer. Am J Clin Nutr (2009) 90(3):578–86. doi: 10.3945/ajcn.2009.28106
321. Bouhnik Y, Raskine L, Champion K, Andrieux C, Penven S, Jacobs H, et al. Prolonged administration of low-dose inulin stimulates the growth of bifidobacteria in humans. Nutr Res (2007) 27(4):187–93. doi: 10.1016/j.nutres.2007.01.013
322. Weir T, Manter D, Sheflin A, Barnett B, Heuberger A, Ryan E. Stool microbiome and metabolome differences between colorectal cancer patients and healthy adults. PloS One (2013) 8(8):1–10. doi: 10.1371/journal.pone.0070803
323. Zheng X, Qiu Y, Zhong W, Baxter S, Su M, Li Q, et al. A targeted metabolomic protocol for short-chain fatty acids and branched-chain amino acids. Metabolomics (2013) 9(4):818–27. doi: 10.1007/s11306-013-0500-6
324. Han J, Lin K, Sequeira C, Borchers C. An isotope-labeled chemical derivatization method for the quantitation of short-chain fatty acids in human feces by liquid chromatography-tandem mass spectrometry. Analytica Chimica Acta (2015) 854:86–94. doi: 10.1016/j.aca.2014.11.015
325. Choi S, Son J, Kim N, Kim Y, Nam R, Park J, et al. Changes in cecal microbiota and short-chain fatty acid during lifespan of the rat. J Neurogastroenterol Motil (2021) 27(1):134–46. doi: 10.5056/JNM20148
326. Köhling H, Plummer S, Marchesi J, Davidge K, Ludgate M. The microbiota and autoimmunity: their role in thyroid autoimmune diseases. Clin Immunol (2017) 183:63–74. doi: 10.1016/j.clim.2017.07.001
Keywords: thyroid disorders, metabolic diseases, gut microbiota, dysbiosis, metabolism and endocrinology, Short-chain Fatty Acids (SCFAs)
Citation: Mendoza-León MJ, Mangalam AK, Regaldiz A, González-Madrid E, Rangel-Ramírez MA, Álvarez-Mardonez O, Vallejos OP, Méndez C, Bueno SM, Melo-González F, Duarte Y, Opazo MC, Kalergis AM and Riedel CA (2023) Gut microbiota short-chain fatty acids and their impact on the host thyroid function and diseases. Front. Endocrinol. 14:1192216. doi: 10.3389/fendo.2023.1192216
Received: 23 March 2023; Accepted: 23 May 2023;
Published: 30 June 2023.
Edited by:
Jieying Liu, Peking Union Medical College Hospital (CAMS), ChinaReviewed by:
Xin Qi, Central South University, ChinaCopyright © 2023 Mendoza-León, Mangalam, Regaldiz, González-Madrid, Rangel-Ramírez, Álvarez-Mardonez, Vallejos, Méndez, Bueno, Melo-González, Duarte, Opazo, Kalergis and Riedel. This is an open-access article distributed under the terms of the Creative Commons Attribution License (CC BY). The use, distribution or reproduction in other forums is permitted, provided the original author(s) and the copyright owner(s) are credited and that the original publication in this journal is cited, in accordance with accepted academic practice. No use, distribution or reproduction is permitted which does not comply with these terms.
*Correspondence: Claudia A. Riedel, Q2xhdWRpYS5yaWVkZWxAdW5hYi5jbA==
Disclaimer: All claims expressed in this article are solely those of the authors and do not necessarily represent those of their affiliated organizations, or those of the publisher, the editors and the reviewers. Any product that may be evaluated in this article or claim that may be made by its manufacturer is not guaranteed or endorsed by the publisher.
Research integrity at Frontiers
Learn more about the work of our research integrity team to safeguard the quality of each article we publish.